DOI:
10.1039/D4TA03492A
(Paper)
J. Mater. Chem. A, 2024,
12, 21864-21872
Interface engineering and oxygen vacancies derived from plasma-treated Cu2O synergistically enhancing electrocatalytic CO2-to-C2+ conversion†
Received
20th May 2024
, Accepted 15th July 2024
First published on 16th July 2024
Abstract
Electrocatalytic CO2 reduction (ECR) into value-added chemicals and fuels helps tackle the challenges of the energy crisis and global warming. However, this strategy relies heavily on the rational design of catalysts with high selectivity and activity towards C2+ products. Herein, we introduce a dual-engineering strategy using plasma-treated Cu2O to synergistically enhance the material's catalytic performance for CO2-to-C2+ conversion. We demonstrate that well-controlled plasma reduction treatment in an Ar/H2 atmosphere can yield stable Cu2O–Cu catalysts (Cu2O–Ar/H2) with Cu0/Cu+ interfaces, abundant grain boundaries, and a high density of oxygen vacancies. Cu2O–Ar/H2 delivers an impressive 81.2% faradaic efficiency for C2+ products at an industrial current density of 100 mA cm−2. Performance comparisons show that plasma pre-reduction treatment samples outperform the in situ reduced Cu2O sample during ECR. Theoretical calculations reveal that the well-defined Cu0/Cu+ interfaces optimize intermediate adsorption and the oxygen vacancies provide multiple active sites for C–C coupling. This work establishes a correlation between plasma treatment-generated active sites and high C2+ product selectivity. Our work also demonstrates that this facile, scalable, standardized and controllable material preparation method can effectively promote the large-scale application of high-activity ECR catalysts.
1 Introduction
Electrocatalytic CO2 reduction (ECR) into high-value chemicals and feedstocks using renewable electricity offers an elegant solution for closing the carbon cycle, addressing global environmental issues, and meeting growing energy demands.1,2 However, the potential of this technology heavily relies on the development of highly active and selective electrocatalysts.3,4 Although other metals such as Ag and Sn have achieved highly selective C1 products, such as CO and formate,5,6 achieving high selectivity for C2+ products on Cu-based catalysts remains highly challenging due to their sluggish multiple proton-coupled electron transfer (PCET) processes in C–C coupling and the competitive hydrogen evolution reaction (HER).7 Therefore, there is an urgent need to develop highly efficient and active catalysts with scalable synthesis methods that can enhance selectivity towards C2+ products.
To enhance ECR to C2+ conversion selectivity, substantial efforts have focused on the rational design of copper-based catalysts, including morphology,8 oxide-derived copper (OD-Cu),9 interfacial structures,10,11 and alloying12,13 strategies. In particular, OD-Cu has been reported with excellent C2+ selectivity,4 garnering widespread research interest. However, for OD-Cu, the active Cu+ species inevitably undergoes electrochemical reduction to bulk metallic Cu at high current densities,14 leading to poor selectivity for C2+ products. This poses a significant challenge for the effective utilization of OD-Cu. Thus, unconventional and feasible strategies for stabilizing the active Cu+ species at reduction potentials during actual CO2 reduction processes is necessary. Research results indicate that abundant nanograin boundaries can enhance the stability of catalyst morphology and Cu0/Cu+ interfaces at high polarization and high current density, preventing catalyst reconstruction and improving the catalytic stability.15 Moreover, nanograin boundaries and the Cu0/Cu+ interface can increase *CO adsorption strength, promoting CO–CO coupling toward C2+ products.16,17 Therefore, constructing intricate structures assembled with abundant nanograin boundaries and Cu0/Cu+ interfaces in Cu-based catalysts is promising for achieving efficient ECR to C2+ products.
In addition, oxygen vacancies, as a well-known defect, are widely employed to tailor the properties of catalysts.18–20 For example, due to their weakly bound electrons, oxygen vacancies serve as excellent Lewis base sites to enhance the binding affinities of key intermediates (such as *CO and *COH), thereby promoting the production of C2.21 Moreover, a higher concentration of oxygen vacancies near the active sites is favorable for activating CO2 molecules, lowering the reaction barrier for targeted products.22 Therefore, integrating oxygen vacancies with grain boundaries and interface engineering is likely to modulate the surface electronic structure and concentration of active sites, accelerating reaction kinetics. However, effectively constructing oxygen vacancies and interface structures on the catalyst surface is highly challenging. A deeper understanding of the synergistic interaction between oxygen vacancies and interface structures will provide valuable guidance for the rational design of catalysts.
Plasma treatment, as a facile and scalable technique, has been introduced to selectively activate catalysts, due to its ability to rapidly alter the surface chemical state of catalysts at room temperature,23 create defect structures,24 or embed heteroatoms25 to improve reactivity. For example, Cu surfaces treated with O2 plasma exhibit higher ethylene selectivity than those treated with H2 plasma. The activity enhancement may originate from the presence of Cu+.26 Cuenya's group reported that O2 plasma treatment gives rise to specific defect sites and stable subsurface oxygen species inside Cu nanocubes, which are key to achieving high activity and ethylene selectivity.27 Additionally, Kang's group indicated that oxygen-plasma-assisted nitrogen doping on CuO can achieve high C2+ product selectivity, with enhanced activity attributed to the oxygen vacancies and grain boundary defects generated by N2 plasma radicals on CuO.24 These studies suggest that plasma treatment, as an effective surface treatment method, can precisely control active sites under different atmospheres.28,29 Nevertheless, whether plasma treatment can be used to synergistically construct oxygen vacancies and Cu+/Cu0 interface structures on the metal oxide catalyst surface has yet to be investigated.
In this study, we employ plasma treatment under different atmospheres as a dual-engineering strategy to couple oxygen vacancies and interface structures, aiming to enhance the activity of the ECR catalysts. Plasma treatment in an Ar/H2 environment results in the reduction of grain-boundary-rich Cu2O, creating active interfaces of Cu0/Cu+ interface structures and abundant oxygen vacancies. Compared to original Cu2O and Cu2O treated under an Ar/O2 atmosphere (Cu2O–Ar/O2), the coordinated interaction between Cu0/Cu+ and oxygen vacancies from Cu2O–Ar/H2 contributes to enhanced catalytic activity for C2+ products. DFT calculations indicate that Cu2O–Ar/H2 is more favorable for *OHCCO intermediate adsorption, promoting the conversion of C2+ products. As a result, the Cu2O–Ar/H2 sample achieves a faradaic efficiency of 81.4% for C2+ products at a current density of 100 mA cm−2. This study reveals the mechanism by which plasma treatment enhances catalyst activity, advancing the development of ECR to C2+ products.
2 Experimental section
2.1 Materials
A Cu2O target was purchased from AJA International Inc, USA. Potassium hydroxide (KOH) and ultrapure water (18 MΩ cm) were obtained from the University of Waterloo Chemical Store. Carbon paper (Freudenberg H15C13) and a Fumasep FAB-PK-130 membrane were purchased from a fuel cell store, USA. Argon and CO2 gas were provided by Linde Gas, Canada.
2.2 Synthesis of electrocatalysts
2.2.1 Synthesis of Cu2O nanostructures.
The carbon paper was cut into pieces of size 6 cm × 4 cm and then gently (low power) sonicated in acetone and IPA for 5 minutes each to remove any unbound materials or surface impurities. The substrates were then dried with an N2 blower and heated at 80 °C for 30 minutes to remove any solvent residue. After that, Cu2O was sputter-deposited on the substrates. For sputtering, the base pressure of the deposition chamber was ∼2 × 10−7 torr, and the Cu2O target was pre-sputtered for 30 min at 200 W. After that, the clean substrates were soaked at 70 °C for 30 minutes inside the deposition chamber and then sputtered for 90 minutes at 200 W power (DC bias: 380 V), keeping the Ar and O2 flow fixed at 12 sccm and 0.3 sccm, respectively with a 3 mTorr chamber pressure during deposition.
2.2.2 Plasma treatment.
The substrates were again cut into small pieces of size 2 cm × 2 cm and then treated with two different plasmas under different atmospheres, keeping all other parameters the same. For plasma treatment, the chamber was flushed with Ar gas three times before each treatment, and the chamber pressure was maintained at 100 mTorr and with an RIE and ICP power of 100 W each. Two different plasmas were created as per the set parameters with different gas flows as follows: O2 plasma: O2 flow 45 sccm, Ar flow 5 sccm; and H2 plasma: H2 flow 15 sccm, Ar flow 35 sccm.
2.3
Ex situ material characterization
Scanning electron microscopy (SEM, Hitachi S4800, Japan) images were taken with a working accelerating voltage of 10 kV. Glancing-incidence X-ray diffraction (GIXRD) spectra were collected on a MRD diffractometer (PANalytical X'Pert Pro, Netherlands) with Cu Kα radiation (1.54 Å) at an incidence angle of 0.3°. X-ray photoelectron spectroscopy (XPS) measurements were conducted (Thermo-VG Scientific ESCALab 250, USA) using microprobes with a monochromatic Al Kα X-ray source (1486.6 eV). High resolution TEM images (Zeiss Libra 200 MC, German) were collected with an acceleration voltage of 200 kV. The XAS measurements were conducted at the 06ID-1 Hard X-ray MicroAnalysis (HXMA) beamline of the Canadian Light Source (CLS) operated at 2.9 GeV with a constant current of 220 mA. The measurements at the copper K-edge were performed in fluorescence mode using a Ge detector. Proton nuclear magnetic resonance (H-NMR) spectroscopy was run on a Bruker Avance III 500 MHz, USA. The reduced products were evaluated on a gas chromatograph (Shimadzu, GC-2014) with a Carbonxen® 1000 column and a CarbonPLOT column for a flame ionization detector (FID) and thermal conductivity detector (TCD), respectively.
2.4 Electrochemical measurements
For the ECR test, all electrochemical tests were performed using an electrochemical workstation (Gamry Reference 3000) with iR compensation at room temperature. The performance evaluation of electrochemical CO2 reduction is conducted in flow cell setups. A prepared sample on carbon paper, Ni foam, and Ag/AgCl are used as the working electrode, counter electrode, and reference electrode, respectively. A gas-tight three-chamber flow cell is equipped with a Fumasep FAB-PK-130 anion exchange membrane (AEM), and 1 M KOH serves as the cathode and anode circulating electrolyte with a flow rate of 18 mL min−1. The flow rate of CO2 gas is set to 30 sccm. The ECR catalytic activities were evaluated using the potentiostatic technique for 1000 s. The gas products were analyzed using a Shimadzu GC-2014, while the liquid products were characterized using a Bruker 500 MHz Nuclear Magnetic Resonance (NMR) spectrometer with an internal reference prepared with D2O and DMSO.
All potentials in this work were measured against the Ag/AgCl reference electrode and converted into reversible hydrogen potential (RHE) using the equation below:
E(vs. RHE) = E(vs. Ag/AgCl) + 0.059 × pH + 0.197 |
All the potentials are relative to the RHE unless stated otherwise.
2.5 DFT calculation details
All calculations were performed using the Vienna ab initio simulation package with the Perdew–Burke–Ernzerhof (PBE) generalized gradient approximation (GGA) exchange–correlation functional.30 A plane wave cutoff energy of 450 eV was employed. Spin polarization and van der Waals corrections in the models were accounted for using the projector augmented wave (PAW)31 method and DFT-D3,32 respectively. The convergence criteria for the total energy and forces were set to 1 × 10−5 eV and 0.03 eV Å−1, respectively. The k-point sampling was performed using a 3 × 3 × 1 gamma scheme. A vacuum layer of 15 Å was introduced to eliminate interactions between the layers in the model. A three-layer Cu2O (111) surface was constructed to model the Cu2O sample, where the oxygen vacancy on the Cu2O (111) surface is represented by removing a coordinated oxygen atom (Fig. S11a†). The Cu2O (111)/Cu (111) structure was obtained by adding two layers of Cu (111) under the Cu2O (111) surface, a four-layer slab model, to model the Cu2O–Ar/H2 sample (Fig. S11b†). The VASPsol implicit solvation model was used to study the solvation effect.33 As shown in Table S3†, taking two key intermediates of the potential-determining steps, *CO and *CHO, as examples, there is little difference in the reaction energies considering the solvation effect or not, and consequently the solvation effect is not considered.
3 Results and discussion
3.1 Synthesis and ex situ characterization of electrocatalysts
The material preparation process, involving both magnetron sputtering and plasma treatment, is illustrated in Fig. 1a. Initially, Cu2O catalysts are fabricated on a gas diffusion electrode (GDE) via magnetron sputtering using a Cu2O target. Subsequently, plasma treatments on Cu2O catalysts are conducted under reducing (Ar/H2) and oxidizing (Ar/O2) atmospheres, wherein highly reactive Ar/H2 and Ar/O2 active particles in the plasma state bombard the surface of Cu2O, causing further reduction or oxidation. This would create different structural defects in the resulting Cu2O–Ar/H2 and Cu2O–Ar/O2. This facile and scalable material preparation method makes it feasible to produce large-scale gas diffusion electrode (GDE)-compatible high-activity ECR catalysts.
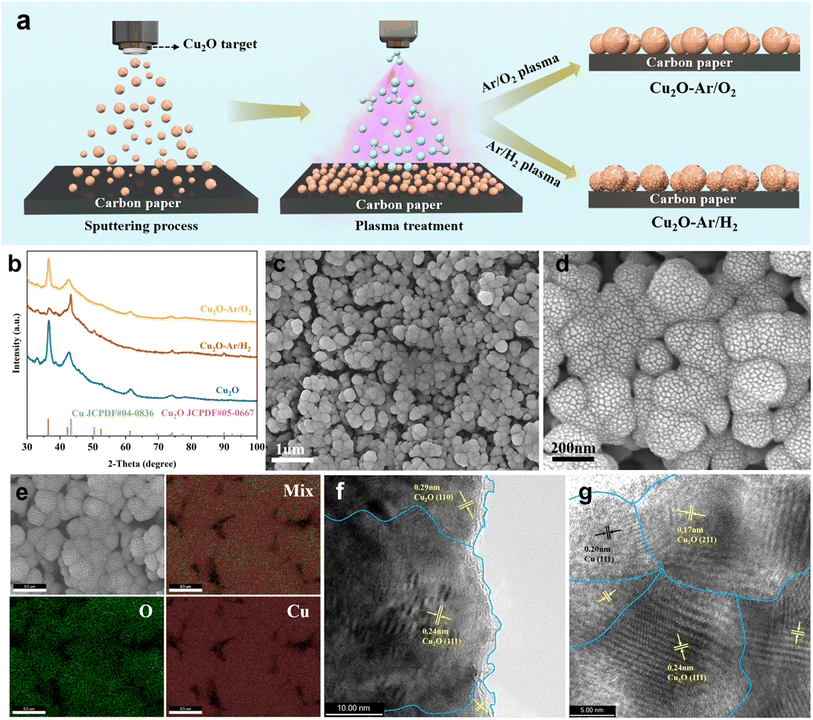 |
| Fig. 1 Synthesis and structural characterization of electrocatalysts. (a) The schematic illustration of the as-synthesized catalysts. (b) GIXRD spectra of the as-prepared electrocatalysts; (c and d) SEM images of Cu2O–Ar/H2; (e) SEM-EDS elemental mapping images of Cu2O–Ar/H2; (f and g) HRTEM images of Cu2O (f) and Cu2O–Ar/H2 (g). | |
The composition and structural characteristics of the synthesized samples were investigated using grazing incidence X-ray diffraction (GIXRD). Diffraction peaks attributed to cuprite (Cu2O JCPDF#05-0667) are observed in the Cu2O and Cu2O–Ar/O2 samples (Fig. 1b), indicating that Ar/O2 plasma treatment does not reduce the original Cu2O. However, the mixed phase of Cu (JCPDF#04-0836) and Cu2O in the Cu2O–Ar/H2 sample suggests that Ar/H2 plasma treatment partially reduced Cu2O to metallic copper (Fig. 1b), forming interface structures between Cu and Cu2O. The scanning electron microscope (SEM) images reveal that Cu2O nanoparticles are in situ grown on carbon paper using magnetron sputtering (Fig. S1a and b†). The Ar/H2 (Fig. 1c, d, S1c and d†) and Ar/O2 (Fig. S1e and f†) plasma treatments do not visibly affect the morphology of Cu2O NPs. SEM-EDS elemental mapping images show a uniform distribution of Cu and O in the Cu2O (Fig. S2a†) and Cu2O–Ar/O2 (Fig. S2b†) samples, while SEM-EDS images (Fig. 1e) confirm that Ar/H2 plasma treatment does not completely reduce Cu2O to Cu, as oxygen signals are still detected. Correspondingly, in terms of elemental composition, compared to pristine Cu2O (Fig. S4†), the oxygen content in Cu2O–Ar/H2 (Fig. S3†) is significantly reduced, while the oxygen content in Cu2O–Ar/O2 (Fig. S5†) remains relatively unchanged. High-resolution transmission electron microscopy (HRTEM) images reveal the presence of abundant grain boundary structures in the as-synthesized samples (Fig. 1f and g). For the original Cu2O, lattice spacings of 0.29 nm and 0.24 nm are attributed to the (110) and (111) planes of Cu2O34 (Fig. 1f and S6a and b†), respectively. However, after plasma reduction treatment on the Cu2O–Ar/H2 sample, the grain boundary structures observed at 0.17 nm for Cu2O (211), 0.20 nm for Cu (111), and 0.24 nm for Cu2O (111) indicate the existence of Cu0/Cu+ interface structures35,36 (Fig. 1g and S7a†). This suggests that partial reduction of Cu atoms in Cu2O nanoparticles has occurred under plasma reduction treatment, forming an interface between Cu0 and Cu+ within the Cu2O lattice. Such interface structures may facilitate the formation of active sites and the generation of oxygen vacancies, thereby enhancing catalytic performance. In contrast, for the Cu2O–Ar/O2 sample, only the grain boundary structures of Cu2O (111) and (110) are observed, with no presence of metallic Cu (Fig. S7b†). This indicates that the plasma oxidation process does not create the unique interface structure seen in the Cu2O–Ar/H2 sample.
X-ray photoelectron spectroscopy (XPS) was used to reveal changes in surface composition and chemical states of Cu2O samples treated with plasma under different reaction atmospheres. For the Cu2O and Cu2O–Ar/H2 samples, the Cu 2p XPS spectrum can be deconvoluted into two peaks at 932.4 and 933.9 eV, corresponding to Cu+/Cu0 and Cu2+ species21,37 (Fig. S8†), respectively. However, compared to the samples treated with Ar/H2 plasma reduction, those treated with Ar/O2 plasma only exhibit features of Cu2+ species (Fig. S8†), indicating that the surface Cu2O has been further oxidized. The Cu Auger LMM spectrum is employed to further confirm the valence state of the Cu species. For the original Cu2O sample, the surface Cu is mainly in the monovalent and divalent Cu species.38 After plasma reduction treatment, some of the surface Cu is reduced to metallic Cu,39 showing the coexistence of zero-valent, monovalent, and divalent Cu species (Fig. 2a). For Cu2O and Cu2O–Ar/H2, CuO originates from the inevitable oxidation of Cu species when exposed to air. Nevertheless, for Cu2O–Ar/O2, the presence of divalent copper on the surface results from the plasma oxidation treatment (Fig. 2a). Regarding the O 1s XPS spectrum, every O 1s curve is asymmetric and can be fitted into three components: lattice oxygen from Cu2O (OL(Cu+)) at 530.3 eV or CuO (OL(Cu2+)) at 529.5 eV,40 adsorbed oxygen (Oad) at 531.5 eV, and adsorbed water (H2Oad) at 533.5 eV41,42 (Fig. 2b). The ratio between Oad and OL can be used to evaluate the amount of surface oxygen vacancies.43 It can be observed that the oxygen vacancy content follows this order: Cu2O–Ar/H2 (3.95) > Cu2O (2.54) > Cu2O–Ar/O2 (0.97), indicating that Cu2O–Ar/H2 has a higher density of surface oxygen vacancies compared to its other two counterparts.
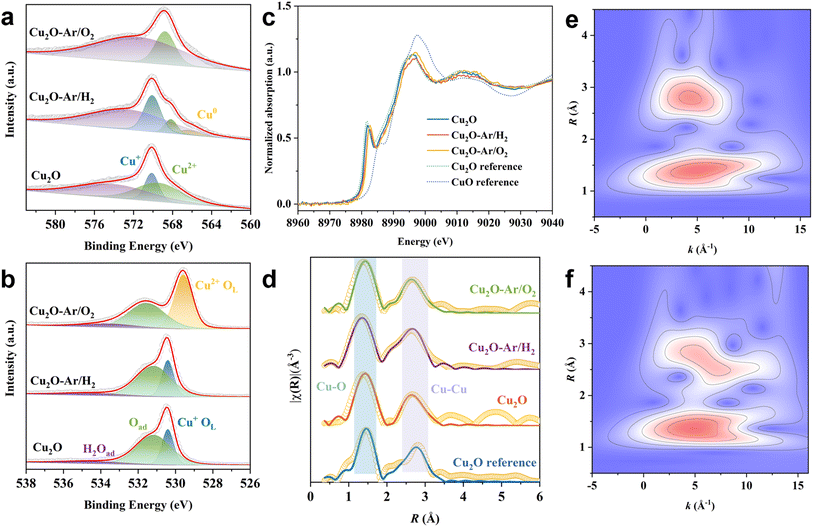 |
| Fig. 2
Ex situ X-ray spectroscopic analysis of electrocatalysts. (a and b) Cu LMM Auger spectra (a) and O 1s XPS spectra (b) of the as-prepared catalysts; (c) Cu K-edge XANES spectra of Cu2O, Cu2O–Ar/H2 and Cu2O–Ar/O2 samples and corresponding references (Cu2O, and CuO). (d) Cu K-edge EXAFS experimental and fitting spectra of Cu2O, Cu2O–Ar/H2 and Cu2O–Ar/O2 catalysts and corresponding references (Cu2O). (e and f) Wavelet transforms for the k3-weighted EXAFS signals of Cu2O reference (e) and Cu2O–Ar/H2 (f). | |
Synchrotron-based X-ray absorption Near-Edge Structure (XANES) spectra and Extended X-ray Absorption Fine Structure (EXAFS) spectra were recorded to further study the chemical state and local coordination environment of copper sites. Compared to Cu2O and CuO references, the XANES spectra of the as-synthesized samples display characteristics of Cu2O (Fig. 2c). Although plasma reduction or oxidation treatments could affect the oxidation state of surface Cu atoms, the bulk phase of the catalysts retains attributes of Cu2O. On the other hand, fitting in the R-space of EXAFS spectra of the as-prepared samples was then conducted to quantify changes in scattering paths (Fig. 2d and Table S1†). For the original Cu2O sample, a Cu–O coordination number of 1.90 at 1.84 Å and Cu–Cu coordination number of 13.1 at 3.01 Å can be observed, similar to the Cu2O reference, indicating the characteristic properties of Cu2O. After plasma reduction or oxidation treatment, different Cu–O coordination numbers of 1.77 at 1.79 Å for Cu2O–Ar/H2 and 1.65 at 1.84 Å for Cu2O–Ar/O2 can be obtained, indicating that plasma treatment results in structural changes around Cu. For Cu2O–Ar/H2, the reduced Cu–O coordination number and similar Cu–Cu coordination number compared to the Cu2O reference indicate the presence of oxygen vacancies. A wavelet transform was employed to elucidate the coordination environment near the Cu atoms and confirm the atomic distribution of Cu in all samples. As shown in Fig. 2e, for the Cu2O reference, a strong wavelet transform signal focused at around 5 Å−1 originating from Cu–O coordination is observed, while another intense signal at the same focus can be attributed to Cu–Cu coordination. The wavelet transform contour plot of the CuO reference exhibits only Cu–O coordination features (Fig. S9a†). In contrast, the original Cu2O sample exhibits similar peak intensities and positions to the Cu2O reference (Fig. S9b†), indicating their resemblance in structures. However, after plasma reduction or oxidation treatment, differing first shell Cu–O and second shell Cu–Cu scattering distributions suggest variations in the local coordination environment around Cu between Cu2O–Ar/H2 and Cu2O–Ar/O2 (Fig. 2fvs.Fig. S9c†).
3.2 Electrochemical CO2 reduction performance
The ECR performance of the as-synthesized catalysts was evaluated in a flow cell using an alkaline electrolyte (1 M KOH). All catalysts exhibit a similar reduced product distribution, with CO, methane, and formic acid as the main C1 products and ethylene, ethanol, acetic acid, and isopropanol as the main C2+ products (Fig. 3a, S10a and b†). For all samples, the H2 FE increases with increasing cathodic potentials, suggesting that at higher negative potentials, the competitive hydrogen evolution reaction (HER) suppresses the production of C2+ products. Therefore, all samples demonstrate higher C2+ product selectivity at lower potentials (−0.65 or −0.7 VRHE). The Cu2O sample shows a relatively high C2+ FE (70.47%) at −0.65 VRHE, but as the potentials increase, the C2+ FE continues to decrease to 39% at −0.75 VRHE (Fig. 3b). Increasing the cathode potentials induces the further reduction of Cu2O to metallic Cu. Since oxidative Cu species act as the main active sites for C2+ products, the reduction in these active species on the catalyst surface results in a decrease in the selectivity for C2+ products. This indicates that in situ reduction of Cu2O cannot enhance catalytic activity at higher cathodic potentials, possibly due to the inherent Cu2O grain boundary structure's inability to stabilize active Cu+ species. Although plasma oxidation treatment (Ar/O2) raises the oxidation state of Cu2O, achieving a C2+ FE of 77.14% at low potential (−0.65 VRHE), it still fails to stabilize active Cu+ species at higher potentials (−0.7 and −0.75 VRHE), leading to decreased activity (Fig. 3b). However, for the Cu2O–Ar/H2 sample, the C2+ FE is lower than that of Cu2O and Cu2O–Ar/O2 at −0.65 VRHE, but at −0.7 VRHE, the C2+ FE reaches 81.2% with a current density of ∼100 mA cm−2. Moreover, at higher potentials (−0.75 VRHE), the C2+ FE of Cu2O–Ar/H2 also exceeds that of Cu2O and Cu2O–Ar/O2 (Fig. 3b). This suggests that the Cu0/Cu+ interface structure and high density of oxygen vacancies obtained through plasma reduction treatment (Ar/H2) can effectively stabilize active Cu species during ECR, enhancing C2+ selectivity. Considering the impact of plasma treatment on catalytic activity of C2+ products, a comparison is made with other plasma-treated catalysts in terms of C2+ FE (Fig. 3c and Table S2†). The results show that catalytic activity of Cu2O–Ar/H2 exceeds that of most plasma-treated samples, demonstrating excellent ECR performance in terms of the C2+ product pathway.
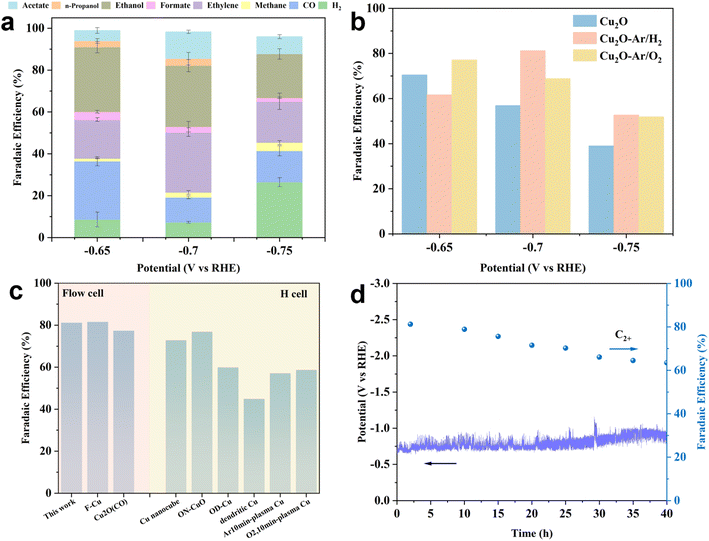 |
| Fig. 3 ECR performance of the as-prepared catalysts. (a) The reduced product distribution of CO2 for Cu2O–Ar/H2 at different cathodic potentials under ECR. (b) The FEs for C2+ products on the as-synthesized samples. (c) Comparisons of the FE values for C2+ products between Cu2O–Ar/H2 and other recently reported plasma-treated Cu-based catalysts. The summary of the specific ECR performance of catalysts is presented in Table S1.† (d) The stability test of Cu2O–Ar/H2 at a current density of 100 mA cm−2 using a flow cell. | |
A long-term stability test was conducted to evaluate the practical applicability of the catalyst. Considering the C2+ product selectivity and industrial-relevant current densities, Cu2O–Ar/H2 was selected to run stability tests in a flow cell with 1 M KOH at a constant current of 100 mA cm−2 (Fig. 3d). The results show that the ECR system could operate stably for 40 h, with a gradual decrease in C2+ FE from an initial 81.2% to 63.5%. This indicates that Cu2O–Ar/H2 has significant potential for industrial-scale ECR to C2+ product conversion as it can be used as a standardized process.
3.3 DFT calculations
To better understand how interface structures and oxygen vacancies enhance the activity towards C2+ products, we conducted DFT calculations with ethylene as the representative C2+ product. As shown in Fig. S11,† there are Cu triangles due to oxygen vacancies, of which both vertex and edge Cu atoms can be active sites. The project density of states (PDOS) shows that the Cu-d states of vertex atoms are always closer to the Fermi level than those of edge atoms (Fig. S12†), so CO2 adsorption begins on these sites. For ethylene production, the adsorbed *CO2 is reduced to *CO first and then hydrogenated to *CHO on vertex Cu atoms, and subsequently the C–C coupling occurs, *CHO + *CO → *CHOCO, on both vertex and edge Cu atoms (Fig. 4a). The C–C coupling step is always endothermic but is weaker than the hydrogenation of *CO to *CHO, and the potential-determining step (PDS) is thus *CO → *CHO, with the free energy changes of the PDS of 0.70 and 0.91 eV for Cu2O–Ar/H2 and Cu2O, respectively. Fig. 4b shows the PDOS of vertex Cu atoms in Cu2O–Ar/H2 and Cu2O, and we can find that the Cu-d states of Cu2O–Ar/H2 are more far to the Fermi level than those of Cu2O, which leads to different adsorption strengths of *CO. Electron accumulation between active Cu sites and the C atom of *CO can be found in the charge density difference plots (Fig. 4c and d), validating strong interactions between *CO and catalysts. The Bader charge analysis suggests that 0.10 |e| is transferred from Cu2O–Ar/H2 to *CO, which is smaller than that on Cu2O (0.16 |e|), in accordance with the more unactive Cu-d states of Cu2O–Ar/H2 shown in Fig. 4b. This leads to weaker *CO adsorption on Cu2O–Ar/H2 with Eads-CO = −1.58 eV, while Eads-CO = −1.83 eV for Cu2O. In short, multiple active sites induced by oxygen vacancies make the C–C coupling easier than the *CO hydrogenation and weaker *CO adsorption on Cu2O–Ar/H2 related to interface engineering makes the *CO hydrogenation easier than that on Cu2O.
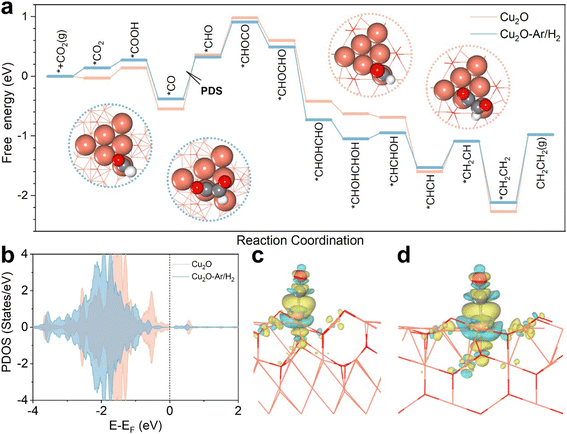 |
| Fig. 4 DFT investigations. (a) Free energy profile of ethylene production on Cu2O–Ar/H2 and Cu2O, including the *CHO and *CHOCO structures. (b) PDOS of the Cu-d states of vertex Cu atoms in Cu2O–Ar/H2 and Cu2O. Charge density differences of *CO on (c) Cu2O–Ar/H2 and (d) Cu2O with an isovalue of 0.001 e A−3, where yellow and cyan regions denote electron accumulation and depletion, respectively. | |
4 Conclusions
In summary, a facile, scalable, and standardized magnetron sputtering method combined with plasma surface treatment was developed to controllably construct high-activity catalysts for C2+ products. Cu2O with rich grain boundaries, upon plasma reduction treatment, displays a well-defined Cu0/Cu+ interface structure with abundant oxygen vacancies. The enhanced activity stems from the synergistic interaction of the interface structure and oxygen vacancies. Compared to the original Cu2O and Cu2O further treated with plasma oxidation, pre-plasma reduction treatment of Cu2O effectively prevents the loss of active sites during in situ reduction of ECR. This work reveals the real active sites created by plasma treatment during the ECR process, effectively promoting the industrial-scale application of ECR.
Data availability
The authors declare that all data supporting this study are available within the paper and ESI† files. Source data are provided upon request.
Author contributions
Y. A. W. conceived and supervised the project. Z. T. co-supervised the project. C. V. S. and L. Z. led the DFT calculations. S. J. and Y. Z. carried out the catalyst's synthesis. L. W. conducted characterization and performance tests of catalysts. X. Y. and C. Q. conducted the DFT calculations. S. J. carried out the XAS measurements. N. C. provided advice for the XAFS measurements. L. W., X. Y., S. J., C. V. S., Z. C. T., and Y. A. W. wrote the manuscript. All authors made comments and revised the manuscript.
Conflicts of interest
All the authors declare no competing interests.
Acknowledgements
Y. A. W. acknowledges the funding from the Government of Canada's New Frontiers Research Fund-Transformation CANSTOREnergy Project (NFRFT-2022-00197), the Natural Sciences and Engineering Research Council of Canada (NSERC) (RGPIN-2020-05903 and GECR-2020-00476), the Tang Family Chair in New Energy Materials and Sustainability, the Canadian Foundation for Innovation John R. Evans Leaders Fund (#41779), and the Ontario Research Fund for Small Infrastructure (#41779). Z. T. acknowledges the funding from the NSERC collaborative research and treating experience program (CREATE) and GCI Ventures Capital, Toronto. This research used the resources of the Canadian Light Source and its funding partners. The studies for hard XAS were performed at HXMA beamlines of the Canadian Light Source (CLS), a national research facility of the University of Saskatchewan, which is supported by the Canada Foundation for Innovation (CFI), the Natural Sciences and Engineering Research Council (NSERC), the National Research Council (NRC), the Canadian Institutes of Health Research (CIHR), and the Government of Saskatchewan. C. V. S. acknowledges support of the Natural Sciences & Engineering Research Council of Canada (NSERC), University of Toronto, and the Digital Research Alliance of Canada for enabling DFT simulations.
References
- C. P. O’Brien,
et al., CO2 Electrolyzers, Chem. Rev., 2024, 124, 3648–3693 CrossRef PubMed.
- L. Peng,
et al., Research advances in electrocatalysts, electrolytes, reactors and membranes for the electrocatalytic carbon dioxide reduction reaction, Acta Phys.–Chim. Sin., 2023, 39(12), 2302037 Search PubMed.
- Y. Jia,
et al., Cu-based bimetallic electrocatalysts for CO2 reduction, Adv. Powder Mater., 2022, 1(1), 100012 CrossRef.
- L. Fan,
et al., Strategies in catalysts and electrolyzer design for electrochemical CO2 reduction toward C2+ products, Sci. Adv., 2020, 6(8), eaay3111 CrossRef CAS PubMed.
- L. Fan,
et al., 1D SnO2 with Wire-in-Tube Architectures for Highly Selective Electrochemical Reduction of CO2 to C1 Products, Adv. Funct. Mater., 2018, 28(17), 1706289 CrossRef.
- S. Verma,
et al., The effect of electrolyte composition on the electroreduction of CO2 to CO on Ag based gas diffusion electrodes, Phys. Chem. Chem. Phys., 2016, 18(10), 7075–7084 RSC.
- W. Ma,
et al., Electrocatalytic reduction of CO2 and CO to multi-carbon compounds over Cu-based catalysts, Chem. Soc. Rev., 2021, 50(23), 12897–12914 RSC.
- M. Ma,
et al., Controllable hydrocarbon formation from the electrochemical reduction of CO2 over Cu nanowire arrays, Angew. Chem. Int. Ed., 2016, 55(23), 6680–6684 CrossRef CAS PubMed.
- C. W. Li,
et al., Electroreduction of carbon monoxide to liquid fuel on oxide-derived nanocrystalline copper, Nature, 2014, 508(7497), 504–507 CrossRef CAS PubMed.
- X. Chang,
et al., Tuning Cu/Cu2O interfaces for the reduction of carbon dioxide to methanol in aqueous solutions, Angew. Chem., 2018, 130(47), 15641–15645 CrossRef.
- X. Y. Zhang,
et al., Direct OC–CHO coupling towards highly C2+ products selective electroreduction over stable Cu0/Cu2+ interface, Nat. Commun., 2023, 14(1), 7681 CrossRef CAS.
- W. J. Dong,
et al., Grain Boundary Engineering of Cu–Ag Thin-Film Catalysts for Selective (Photo) Electrochemical CO2 Reduction to CO and CH4, ACS Appl. Mater. Interfaces, 2021, 13(16), 18905–18913 CrossRef CAS.
- Z. Chang,
et al., The tunable and highly selective reduction products on Ag@Cu bimetallic catalysts toward CO2 electrochemical reduction reaction, J. Phys. Chem. C, 2017, 121(21), 11368–11379 CrossRef CAS.
- J. Chen and L. Wang, Effects of the catalyst dynamic changes and influence of the reaction environment on the performance of electrochemical CO2 reduction, Adv. Mater., 2022, 34(25), 2103900 CrossRef CAS PubMed.
- Q. Wu,
et al., Nanograin-Boundary-Abundant Cu2O–Cu Nanocubes with High C2+ Selectivity and Good Stability during Electrochemical CO2 Reduction at a Current Density of 500 mA cm−2, ACS Nano, 2023, 17, 12884–12894 CrossRef CAS PubMed.
- Y. Yang,
et al., Operando studies reveal active Cu nanograins for CO2 electroreduction, Nature, 2023, 614(7947), 262–269 CrossRef CAS PubMed.
- X. Yuan,
et al., Controllable Cu0–Cu+ sites for electrocatalytic reduction of carbon dioxide, Angew. Chem., 2021, 133(28), 15472–15475 CrossRef.
- H. Li,
et al., Oxygen vacancy structure associated photocatalytic water oxidation of BiOCl, ACS Catal., 2016, 6(12), 8276–8285 CrossRef CAS.
- H. Li,
et al., Oxygen vacancy-mediated photocatalysis of BiOCl: reactivity, selectivity, and perspectives, Angew. Chem. Int. Ed., 2018, 57(1), 122–138 CrossRef CAS PubMed.
- X. Li,
et al., Strategies for enhancing electrochemical CO2 reduction to multi-carbon fuels on copper, Innov. Mater., 2023, 1(1), 100014 CrossRef.
- Z. Gu,
et al., Oxygen vacancy tuning toward efficient electrocatalytic CO2 reduction to C2H4, Small Methods, 2019, 3(2), 1800449 CrossRef.
- Y. Wang,
et al., Defect and interface engineering for aqueous electrocatalytic CO2 reduction, Joule, 2018, 2(12), 2551–2582 CrossRef CAS.
- J.-J. Zou,
et al., Control of the metal – support interface of NiO-loaded photocatalysts via cold plasma treatment, Langmuir, 2006, 22(5), 2334–2339 CrossRef CAS PubMed.
- D. G. Park,
et al., Increasing CO binding energy and defects by preserving Cu oxidation state via O2-plasma-assisted N doping on CuO enables high C2+ selectivity and long-term stability in electrochemical CO2 reduction, ACS Catal., 2023, 13(13), 9222–9233 CrossRef CAS.
- Y. Zhou,
et al., Dopant-induced electronic structure
modification of HOPG surfaces: implications for high activity fuel cell catalysts, J. Phys. Chem. C, 2010, 114(1), 506–515 CrossRef CAS.
- H. Mistry,
et al., Highly selective plasma-activated copper catalysts for carbon dioxide reduction to ethylene, Nat. Commun., 2016, 7(1), 1–9 Search PubMed.
- D. Gao,
et al., Plasma-activated copper nanocube catalysts for efficient carbon dioxide electroreduction to hydrocarbons and alcohols, ACS Nano, 2017, 11(5), 4825–4831 CrossRef CAS PubMed.
- P. Chen,
et al., In situ reconfiguration of plasma-engineered copper electrodes towards efficient electrocatalytic hydrogenation, Catal. Sci. Technol., 2022, 12(12), 4032–4039 RSC.
- F. Scholten,
et al., Plasma-modified dendritic Cu catalyst for CO2 electroreduction, ACS Catal., 2019, 9(6), 5496–5502 CrossRef CAS.
- J. P. Perdew,
et al., Generalized Gradient Approximation Made Simple, Phys. Rev. Lett., 1996, 77(18), 3865–3868 CrossRef CAS.
- G. Kresse and J. Furthmüller, Efficiency of ab initio total energy calculations for metals and semiconductors using a plane-wave basis set, Comput. Mater. Sci., 1996, 6(1), 15–50 CrossRef CAS.
- S. Grimme,
et al., A consistent and accurate ab initio parametrization of density functional dispersion correction (DFT-D) for the 94 elements H-Pu, J. Chem. Phys., 2010, 132(15), 154104 CrossRef.
- K. Mathew,
et al., Implicit solvation model for density-functional study of nanocrystal surfaces and reaction pathways, J. Chem. Phys., 2014, 140, 084106 CrossRef PubMed.
- C. Chen,
et al., The in situ study of surface species and structures of oxide-derived copper catalysts for electrochemical CO2 reduction, Chem. Sci., 2021, 12(16), 5938–5943 RSC.
- Z. Zhou,
et al., Enhanced CO2 Electroreduction to Multi-Carbon Products on Copper via Plasma Fluorination, Adv. Sci., 2024, 2309963 CrossRef CAS PubMed.
- C. Ma,
et al., A novel core/shell cuprous oxide-based structure with improved microwave absorbing and antibacterial performance, J. Clean. Prod., 2022, 378, 134419 CrossRef CAS.
- Y.-H. Zhang,
et al., Oxygen vacancies in concave cubes Cu2 O-reduced graphene oxide heterojunction with enhanced photocatalytic H2 production, J. Mater. Sci.: Mater. Electron., 2019, 30, 7182–7193 CrossRef CAS.
- I. Platzman,
et al., Oxidation of polycrystalline copper thin films at ambient conditions, J. Phys. Chem. C, 2008, 112(4), 1101–1108 CrossRef CAS.
- Y. Yang,
et al., Nitrogen-doping boosts *CO utilization and H2O activation on copper for improving CO2 reduction to C2+ products, Green Energy Environ., 2023 Search PubMed , in press.
- Y. Wang,
et al., Synthesis of porous Cu2 O/CuO cages using Cu-based metal–organic frameworks as templates and their gas-sensing properties, J. Mater. Chem. A, 2015, 3(24), 12796–12803 RSC.
- L. Xue,
et al., Unveiling the reaction pathway on Cu/CeO2 catalyst for electrocatalytic CO2 reduction to CH4, Appl. Catal., B, 2022, 304, 120951 CrossRef CAS.
- Q. T. Trinh,
et al., Synergistic application of XPS and DFT to investigate metal oxide surface catalysis, J. Phys. Chem. C, 2018, 122(39), 22397–22406 CrossRef CAS.
- Z. Wang,
et al., Surface oxygen vacancies on Co3 O4 mediated catalytic formaldehyde oxidation at room temperature, Catal. Sci. Technol., 2016, 6(11), 3845–3853 RSC.
|
This journal is © The Royal Society of Chemistry 2024 |
Click here to see how this site uses Cookies. View our privacy policy here.