DOI:
10.1039/D4TA03835E
(Paper)
J. Mater. Chem. A, 2024,
12, 21971-21986
Multifunctional self-refrigerated multivariate {GdLn} (Ln = Dy, Tb, Tb/Eu) metal–organic frameworks†
Received
3rd June 2024
, Accepted 18th July 2024
First published on 18th July 2024
Abstract
Multivariate metal–organic frameworks (MOFs) containing multiple lanthanide ions present a compelling avenue for developing versatile materials with tailored properties. In this work, we synthesized “self-refrigerated” multifunctional carborane-based MOFs of formula unit {[(GdLn)3(mCB-L)4(NO3)(DMF)x]n·Solv} strategically combining Gd3+ ions, known for their ability to achieve large magnetocaloric effects (MCE), with various lanthanides (Ln = Dy, Tb, Eu, Tb/Eu) intended to act as Single Molecule Magnet (SMM) or/and luminescent units. The intricate magnetic, thermal, and optical properties of these multivariate Ln-MOFs were unraveled through a comprehensive characterization employing dc and ac magnetometry, X-ray Absorption Spectroscopy (XAS), X-ray Magnetic Circular Dichroism (XMCD), and luminescence measurements. Element-selective XAS-XMCD technique proved instrumental in elucidating the magnetic properties of the individual lanthanides, and their contribution to the macroscopic properties of the MOFs. We demonstrate that Gd1.5Ln1.5 (Ln = Tb, Dy) MOFs exhibit multifunctionality, incorporating MCE, field-induced magnetic relaxation dominated by the anisotropic ion, and green emission for Ln = Tb. Conversely, Gd1.5Ln1.5 (Ln = Eu, Eu/Tb) MOFs display MCE, field-induced SMM behavior associated with Gd, and red/yellow luminescent emission for Ln = Eu and Eu/Tb substitutions, respectively. Our findings significantly contribute to our understanding of “complex magnetic molecular materials” and set a pathway for the design of multifunctional multi-lanthanide MOFs endowed with tailored properties for various technological applications.
Introduction
Metal–organic frameworks (MOFs) have emerged as a versatile class of materials, showcasing unprecedented structural and compositional tunability that enables a wide range of applications in fields such as catalysis,1 gas storage2 and separation, drug delivery, magnetism,3–5etc. The deliberate incorporation of two or more metals into a single crystalline phase has given rise to multi-metallic multivariate (MTV) MOFs, unveiling additive or synergistic effects that sometimes surpass the individual contributions of their constituent elements.6–8 These materials have been extensively explored for their unique properties, particularly in areas such as biomedical applications, catalysis, luminescent sensing, and more.6,7 While multi-metallic MTV MOFs incorporating up to ten different transition metal ions have been well-studied for their specific characteristics,8–13 a notable gap exists in our understanding of corresponding multi-lanthanide MOFs.14 Remarkably, existing research primarily focuses on the luminescence properties of these mixed lanthanide-containing MOFs, with less attention paid so far to magnetic phenomena.15–17
Lanthanide MOFs (Ln MOFs) stand out as a distinct class of compounds featuring appealing optical, electronic, and magnetic properties resulting from 4f electrons. This makes them attractive for many applications, including luminescence,18,19 anticounterfeiting,20 sensors,21–23 switching,24 thermometry,25 magnetic refrigeration,26–29 conductive MOFs30 and information storage and processing,31,32 among others.
Indeed, the significant anisotropy of Ln(III) ions makes them excellent candidates for designing single-molecule magnets (SMMs), exhibiting magnetic stability and quantum-tunneling effects.33–35 Recently, mononuclear SMMs with huge thermal activation energies close to 2000 K,36 and open hysteresis loops above 80 K (ref. 37) have been demonstrated. Simultaneously, Ln(III) ions provide avenues for encoding molecular qubits for quantum computing.38 The molecular platform offers the possibility to prepare reproducible qubits, at the limit of miniaturization, and push the scaling up, by encoding multiple qubits in one ion,39,40 or by coupling the electronic spin with the nuclear spin.41,42 The spatially ordered structures of MOFs present the potential to construct high-density information storage devices, incorporating SIMs as classical memory units in the nodes,22,24,43–49 and also “quMOFs” of organized qubit arrays for quantum computing.50,51
On the other hand, Ln-MOFs are good candidates for ultra-low temperature cooling based on the magnetocaloric effect (MCE).26–29 This effect exploits the entropy changes that result in the heating or cooling of the material when applying an external magnetic field at constant pressure. Magnetic refrigeration is an energy-efficient and environmentally friendly cooling technique that represents a significant advancement in sustainable cooling technology, particularly in the cryogenic regime, by eliminating the need for environmentally harmful refrigerants. In recent years, significant advancements have been made in magnetocaloric materials across various temperature ranges,52 crucial for applications such as hydrogen liquefaction53–55 and cryogenic refrigeration.56,57 Recent reviews have highlighted the exceptional MCE performance of rare-earth based magnetocaloric materials,58 including oxides,59 intermetallics,60 amorphous/high-entropy alloys, coordination polymers and MOFs.28,29 Gd(III) ions, with S = 7/2, L = 0, J = 7/2, are ideal for obtaining a large MCE, since maximum entropy can be obtained from Smax = Rln(2S + 1). Therefore, most MOFs reported for magnetic refrigeration are based on Gd, although proposals have been put forward to use anisotropic lanthanides to optimize the MCE performance of Ln-MOFs under lower magnetic field changes61 and extend the operating temperature above 4 K.62–64
Interestingly, some Ln-MOFs can simultaneously incorporate several properties at the same time, such as magnetism along with luminescence,17,65,66 or proton conductivity.67,68 Moreover, the synthesis of multivariate mixed lanthanide MOFs can open new avenues for the development of multifunctional materials, combining different properties, or presenting new synergistic effects.
For instance, in lighting application, Ln(III) ions are known for their high color purity and significant quantum yields, particularly for those emitting red (Eu3+) and green (Tb3+) light.20,66,69 Mixed Ln-MOFs have been frequently employed to tailor color emission by incorporating two or three Ln(III) ions in various proportions (commonly Eu/Tb,20,70,71 but also other combinations such as La/Eu,72 Gd/Eu,70,72–74 Gd/Tb,70,73,74 Gd/Sm,74 Eu/Gd/Tb,75–77 or Ce/Eu/Tb,73) and to achieve white-light emission.73,77 This is often accomplished by combining Eu/Tb chromophores with blue-emitting ligands.72,75–77 Moreover, mixed Ln-MOFs containing combinations like Eu/Tb,78–81 Eu/Gd,82 or Eu/Tb/Gd,77 have been used to produce highly sensitive, ratiometric luminescent temperature sensors effective across a wide range of temperatures,83 extending up to physiological levels.80–82
Meanwhile, in the field of magnetic refrigeration, mixed MOFs incorporating Gd and Tb with moderate ferromagnetic interactions have proven advantageous for improving MCE properties under low fields (<2 T), attainable by permanent magnets.62 Furthermore, mixed Gd/Dy MOFs exhibited a shift of the maximum entropy change peak −ΔSm towards higher temperatures with increasing the contents of Dy, while maintaining substantial −ΔSm values, providing a route to expand the temperature range of magnetic cooling.64
Remarkably, in a recent work, we demonstrated the possibility of synthesizing multi-metallic multivariate (MTV) MOFs incorporating up to eight different rare-earth (RE) cations.15 We emphasize that examples of multi-metallic MOFs with more than three 4f ions had never been reported before, due to compositional segregation issues during crystallization. This milestone was achieved thanks to the use of a 3D based carborane linker, mCB-L = 1,7-di(4-carboxyphenyl)-1,7-dicarba-closo-dodecaborane, which proved to be essential for the incorporation of different-sized RE cations.15 Icosahedral carboranes represent a commercially accessible group of boron-rich clusters known for their remarkable stability.84–86 They possess high hydrophobicity85–91 and unconventional electronic structure, and are considered as inorganic 3D “aromatic” moieties.92–94 Carborane-derived MOFs have been documented in literature,95–105 and investigations, including our own, have demonstrated that integrating hydrophobic carborane linkers into MOF structures enhances their water stability.106–109 This advancement has facilitated the development of aqueous inks for use in anti-counterfeiting and barcoding applications,20 as well as MOF materials capable of trapping and degrading pesticides present in water.110
Significantly, our innovative carborane-based approach enables the creation of multi-metallic MOFs with any desired combination of lanthanide ions, facilitating the synthesis of multifunctional materials with tailored properties, and the investigation of novel “compositionally complex magnetic materials” with unexplored phenomena. In this work, we exploit this strategy to prepare “self-refrigerated” carborane-based MOFs of formula unit {[(GdLn)3(mCB-L)4(NO3)(DMF)x]n·Solv}, where we combine Gd3+ ion, which is optimum to achieve large magnetocaloric effect (MCE), with various lanthanides (Ln = Dy, Tb, Eu, Tb/Eu) to provide added functionalities as SMM units or/and luminescent units. A comprehensive characterization, combining dc and ac magnetometry, X-ray Absorption Spectroscopy (XAS) and X-ray Magnetic Circular Dichroism (XMCD), along with luminescence measurements, will be used to provide insights into the intricate magnetic, thermal, and optical properties of these novel multivariate MOFs. Element-selective XAS-XMCD synchrotron technique will be crucially utilized to probe the magnetic properties of individual ions and elucidate how the overall magnetic and MCE properties depend on the constituent elements. We will discuss the multifunctional properties achieved for each GdLn MOF, depending on the lanthanide composition. Despite the increasing interest in multivariate MOFs,7 applications of magnetic multi-lanthanide MOFs have been largely unexplored. Through this work, we aim to contribute to the holistic understanding of these complex magnetic materials, and lay the foundation for future advancements in the discovery of multifunctional multi-lanthanide MOFs, holding promise for various technological applications.
Experimental section
Synthesis
All chemicals were of reagent-grade quality. They were purchased from commercial sources and used as received. 1,7-di(4-carboxyphenyl)-1,7-dicarba-closo-dodecaborane ligand (mCBH2L) was synthesized by a slight modification of a literature procedure.111
Synthesis of {[(Ln)3(mCB-L)4(NO3)(DMF)x]n·Solv} (Ln = Gd/Eu, Gd1.5Eu1.5; Gd/Tb, Gd1.5Tb1.5; Gd/Dy, Gd1.5Dy1.5; Gd/Eu/Tb, GdEuTb). These compounds were synthesized following a previously described procedure.15 In a typical preparation, mCB-L (0.03 mmol) and Ln(NO3)3 (0.02 mmol; Ln = Gd and Eu or Tb or Dy or Eu and Tb) were added to a mixture of DMF (0.5 mL)/methanol (1.5 mL)/H2O (0.3 mL) and sonicated until complete dissolution of all reagents. The above mixture was transferred to an 8-dram vial and heated at 95 °C in an oven for 48 h. Needle like white crystals were collected and washed with DMF. Yield based on the Ln ions: 61% for Gd1.5Eu1.5, 63% for Gd1.5Tb1.5, 58% for Gd1.5Dy1.5, 62% for GdEuTb. IR (ATR; selected bands; cm−1): 2601 (BH); 1658 (C
O from DMF); 1590 (C
O from carboxylate). {[(Gd0.48Eu0.52)3(mCB-L)4(NO3)(DMF)x]n·Solv} (Gd1.5Eu1.5). ICP(wt%): Gd(8.24 ± 0.1), Eu(8.76 ± 0.1). {[(Gd0.48Tb0.52)3(mCB-L)4(NO3)(DMF)x]n·Solv} (Gd1.5Tb1.5). ICP(wt%): Gd(9.4 ± 0.1), Tb(10.6 ± 0.1). {[(Gd0.47Dy0.53)3(mCB-L)4(NO3)(DMF)x]n·Solv} (Gd1.5Dy1.5). ICP(wt%): Gd(8.8 ± 0.1), Dy(10.36 ± 0.1). {[(GdEuTb)3(mCB-L)4(NO3)(DMF)x]n·Solv} (GdEuTb). ICP(wt%): Gd(6.6 ± 0.1), Eu(7.0 ± 0.1), Tb(5.8 ± 0.1).
Instruments and characterization
Attenuated total reflection Fourier transformed infrared (ATR-FTIR) spectra were recorded using a PerkinElmer Spectrum One spectrometer equipped with a Universal ATR sampling accessory. Spectra were collected with 2 cm−1 spectral resolution in the 4000–650 cm−1 range. Elemental analyses were obtained by using a Thermo (Carlo Erba) Flash 2000 Elemental Analyser, configured for wt% CHN. Powder X-ray Diffraction (PXRD) was recorded at room temperature on a Siemens D-5000 diffractometer with Cu Kα radiation (λ = 1.5418 Å, 35 kV, 35 mA, increment = 0.02°). Inductively Coupled Plasma – Mass Spectrometry (ICP-MS) measurements were carried out in an Agilent ICP-MS 7700x apparatus. Scanning Electron Microscopy (SEM) (QUANTA FEI 200 FEGESEM) and optical microscopy (Olympus BX52) were used to monitor the morphology and color changes at various conditions. Solid state UV-visible spectra were obtained on a UV-vis-NIR V-780 spectrophotometer equipped with operational range of 200–1600 nm.
Dc magnetometry measurements in the temperature range 1.8 to 300 K were collected using a Quantum Design MPMS SQUID equipped with a 5 T magnet. Experiments were conducted on a powder samples embedded in Daphne oil to prevent grain orientation. Ac susceptibility measurements in the range between 1.8–9.0 K, at μ0Hac = 4.1 × 10−4 T, μ0Hdc = 0–2.5 T in the range of frequencies between f = 0.1–1000 Hz were determined in the same SQUID magnetometer. Additional ac susceptibility measurements in the 10–10000 Hz range were carried out in a Quantum Design PPMS.
X-ray absorption spectroscopy (XAS) and X-ray magnetic circular dichroism (XMCD) experiments across the M4,5 edges of Gd, Tb, Eu and Dy were performed at BOREAS beamline in ALBA synchrotron. The powdered samples were crashed on an indium foil and placed at the top of the cold finger. Measurements were performed at temperatures ranging between base temperature, 3.4 K ± 0.5 K, and 18 K. All spectra were recorded using Total Electron Yield (TEY) detection mode, with a 90% circularly polarized light. The XMCD (μ−–μ+) and XAS (μ+ + μ−/2) spectra at 6 T were determined from eight X-ray absorption spectra measured under right-handed (μ+) and left-handed (μ−) circular polarizations. XMCD(H) cycles were performed by following the resonant M5 peak while sweeping the magnetic field between 6 T and −6 T at a rate of 2 T min−1.
Luminescent emission spectra were obtained with PTI Quantamaster 300 fluorimeter, putting the solid powder in a custom-made holder and setting the holder plane at 45° with the direction of the incident light and the optical path towards the detector. All spectra were obtained irradiating with a continuous wave Xe lamp at λexc = 280 nm. Lifetime measurements were obtained with the same fluorimeter, but exciting at 280 nm with a pulsed Xe lamp (100 Hz, 2 μs integration time). Absolute luminescence quantum yields (Φ) of solid-state samples under continuous wave excitation (λexc = 280 nm) were determined using the Quantum Yield Fluorimeter Hamamatsu C9920-02G, equipped with an integrating sphere, connected to the lamp with an optical fiber, at room temperature in the air. Φ values were calculated based on the number of photons absorbed and emitted by the sample. A detailed measurement procedure can be found in a previous report.112 Reported overall Φ values are averages of at least three independent determinations.
Results and discussion
Syntheses, structural and compositional characterization of the {GdLn} MOFs
{[(Gd0.48Eu0.52)3(mCB-L)4(NO3)(DMF)x]n·Solv} (Gd1.5Eu1.5), {[(Gd0.48Tb0.52)3(mCB-L)4(NO3)(DMF)x]n·Solv} (Gd1.5Tb1.5), {[(Gd0.47Dy0.53)3(mCB-L)4(NO3)(DMF)x]n·Solv} (Gd1.5Dy1.5) and {[(GdEuTb)3(mCB-L)4(NO3)(DMF)x]n·Solv} (GdEuTb) were obtained as colorless crystals by solvothermal reactions in a mixture of DMF/methanol/H2O at 95 °C for 48 h (see Experimental section for details). Powder X-ray diffraction (PXRD) data for all the synthesized {GdLn} MOFs (Fig. 1a) reveal these materials are isostructural with the mono- and multi-metal MOFs of the same family (see ESI Fig. S1†).15,20 Thus, we only briefly describe here the main structural characteristics to facilitate the understanding of the magnetic properties of the newly reported mixed MOFs. Fig. 1b shows a view of the 3D structure for this family of MOFs, while a detailed description of the single crystal structure of previously published mono-metallic MOFs can be seen in Fig. S2.† The structure for these lanthanide family of carborane MOFs consists of 1D-chains of Ln cations along the b axis that are connected through the carborane dicarboxylic linkers to form the observed 3D structures (Fig. 1a and S2†). It is important to remark that the 1D-chains of Ln cations are formed by the repetition of three crystallographically non-equivalent Ln(III) ions (Ln1, Ln2, Ln3; indicated in Fig. 1b), each with different coordination environments. Three different Ln⋯Ln distances (Ln1⋯Ln2, 4.6–4.7 Å; Ln2⋯Ln3, 5.3 Å; Ln1⋯Ln3, 4.6 Å)15,20 are found in the 1D-chains as a consequence of the non-equivalent positions of the three Ln ions. There can be found two distinct inter-chain distances in the 3D structure due to the arrangement of the carborane linkers (ca. 11 Å and 17 Å; Fig. S2†).
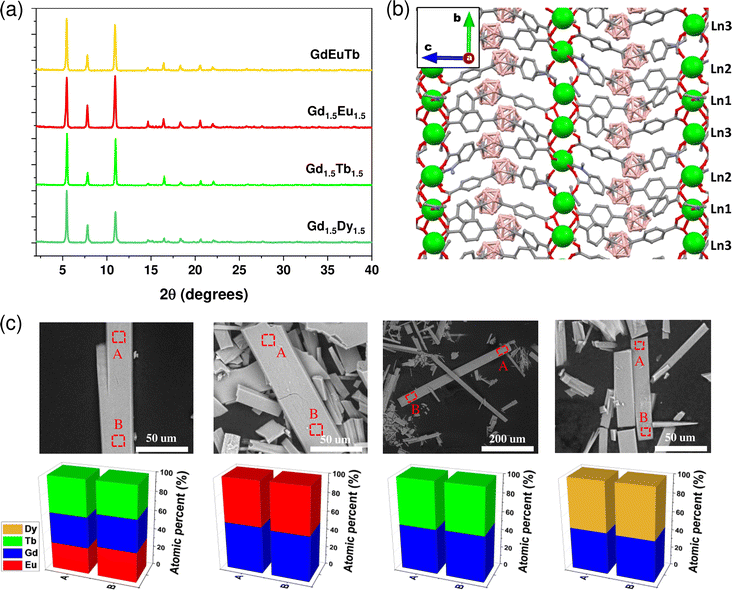 |
| Fig. 1 Structural characterization of mixed {GdLn} MOFs. (a) Experimental Powder X-ray diffraction patterns for GdEuTb (yellow), Gd1.5Eu1.5 (red), Gd1.5Tb1.5 (green) and Gd1.5Dy1.5 (dark green). (b) A view of the extended 3D framework for this MOF family along the a-axis, showing the Ln 1D-chains running along the b-axis, formed by three sites (Ln1, Ln2, Ln3) with non-equivalent coordination environments and the carborane linker mCB-L; Colour code: Ln (green), O (red), B (orange), C (grey); N (blue); H atoms and DMF molecules are omitted for clarity. (c) SEM images (from left to right) of GdEuTb, Gd1.5Eu1.5, Gd1.5Tb1.5 and Gd1.5Dy1.5 and EDX analysis of point scans of the Ln metals on the indicated positions (A and B) in the crystals. | |
Analyses of the {GdLn} MOFs crystals by Scanning electron microscopy (SEM)/Energy dispersive X-ray (EDX) spectroscopy show a similar morphology to those previously reported (Fig. 1c).15,20 In addition, EDX analysis at various points in the crystals confirm an equimolar distribution of Ln ions, ruling out compositional segregation and being consistent with multi-metallic MTV MOF composition.14,15
Magneto-thermal properties
The dc magnetic properties and MCE of all {GdLn} MOFs were characterized by SQUID magnetometry. For each MOF, we measured the temperature dependence of the susceptibility-temperature product at 0.1 T, χT(T), and the field-dependence of the magnetization, M(H), at different temperatures between 1.8 K and 12 K. From the later, the temperature dependence of the entropy change, −ΔSm, for different applied magnetic field changes ΔB = (Bf − 0) between Bf = 0.5–5 T was determined, following the Maxwell method.113 Results are summarized in Fig. 2.
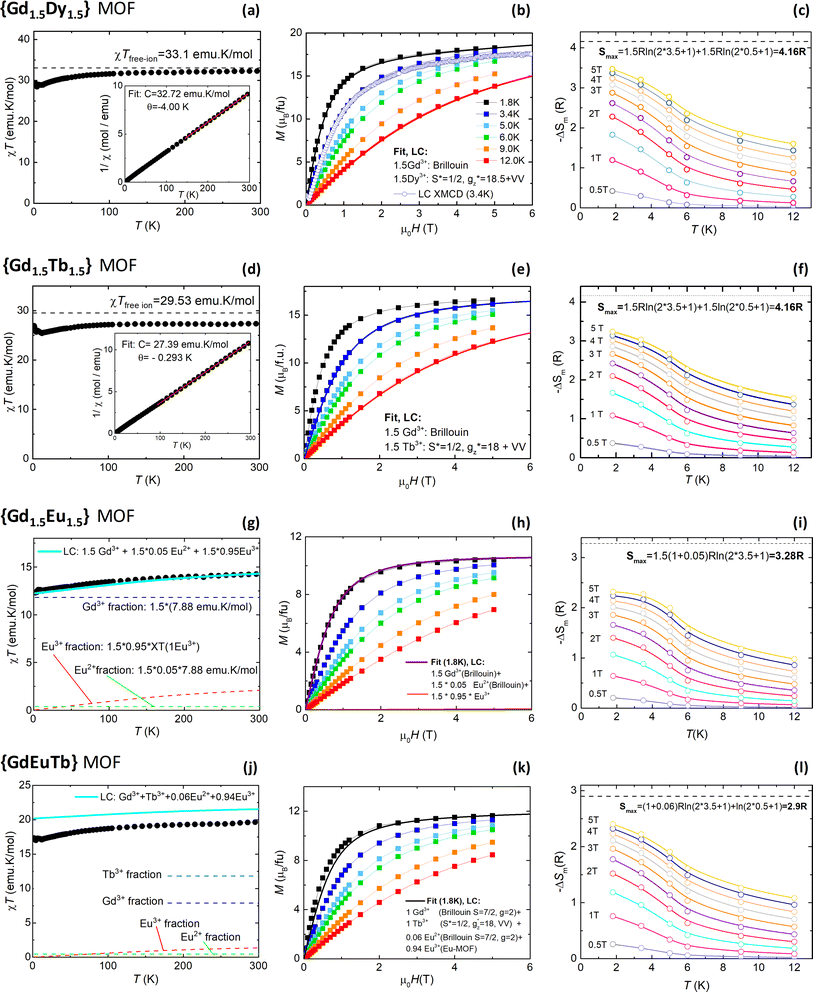 |
| Fig. 2 Dc magnetometry and MCE properties for {GdLn} MOFs (Gd1.5Dy1.5, Gd1.5Tb1.5, Gd1.5Eu1.5 and GdEuTb). (Left column: a, d, g and j): temperature dependence of the susceptibility product, χT, at 0.1 T; (central column: b, e, h and k): magnetization as a function of the applied magnetic field for different temperatures between 1.8–12 K; (right column: c, f, i and l): change of magnetic entropy as a function of the temperature for selected changes of applied magnetic field ΔB = Bf − 0. | |
For Gd1.5Dy1.5 MOF the χT product at 300 K reaches a value of 33.1 emu K mol−1, close to the expected value for 1.5 Gd3+ and 1.5 Dy3+ ions per f.u., where the theoretical saturation for each ion, given by χTfree ion = gJ2J(J + 1)/8 (gJ the Landé constant and J the total angular momentum), is 7.9 emu K mol−1 (Gd3+) and 14.1 emu K mol−1 (Dy3+), see Fig. 2a. Upon decreasing the temperature, the χT product decreases down to 28.48 emu K mol−1 at 3.8 K, and then slightly increases for lower temperatures reaching a value of 29.45 emu K mol−1 at 1.8 K, evidencing the existence of weak intrachain ferromagnetic (FM) interactions. In the homonuclear Dy MOF analogue, such intrachain Ln–Ln interactions were also found to be FM,114 estimated of the order
, and practically negligible for isostructural Gd MOF.115 On the other hand, the linear fit of the 1/χ plot at high temperatures yields a Curie–Weiss temperature of θ = −4.00 K, evidencing overall antiferromagnetic (AF) interactions, possibly of dipolar origin.
The M(H) curves at all temperatures measured for Gd1.5Dy1.5 MOF could be reasonably fit using a weighted linear combination of the magnetization predictions for Gd (using a Brillouin model with S = 7/2, g = 2), and the M(H) curves for Dy within an Ising model, with effective spin S* = 1/2,
,
, a van Vleck contribution MVV = H·0.19 μB T−1 and negligible interactions (J* = 0) (Fig. 2b).
The magnetic entropy change −ΔSm(T, ΔB) obtained for Gd1.5Dy1.5 for selected field changes ΔB are shown in Fig. 2c. For practical purposes it is useful to discuss results obtained for Bf = 2 T, which is easily attained with permanent magnets in magnetic refrigerator applications. Thus, for ΔB = 2–0 T the maximum value is found −ΔSmaxm = 2.28 R (7.90 J kg−1 K−1) at 1.8 K. This is a 55% of the maximum magnetic entropy available for this mixed Gd1.5Dy1.5 MOF: Smax = 1.5Rlog(2·3.5 + 1) + 1.5Rlog(2·0.5 + 1) = 4.16 R.
In the case of Gd1.5Tb1.5 MOF, the χT product at 300 K saturates to 29.53 emu K mol−1, approaching the expected value for the combination of 1.5 Gd3+ ions and 1.5 Tb3+ ions (χTfree ion for Tb = 11.8 emu K mol−1), see Fig. 2d. Similar to previous Gd1.5Dy1.5 MOF, the χT(T) product decreases upon cooling down to 25.42 emu K mol−1 at 12 K, and then slightly raises reaching 26.95 emu K mol−1 at 1.8 K, again pointing to the existence of weak intra-chain FM interactions. Homonuclear Tb MOF counterpart also presented weak FM intra-chain interactions.114
For Gd1.5Tb1.5 MOF the M(H) isotherms could be fit using a weighted linear combination of the Brillouin prediction for Gd, and the M(H) curves for Tb, again within an Ising model, with effective spin S* = 1/2,
,
, a smaller van Vleck contribution MVV = H·0.35 μB T−1 and negligible interactions (Fig. 2e).
The magnetic entropy change −ΔSm(T, ΔB) obtained for Gd1.5Tb1.5 from its magnetization isotherms, is shown in Fig. 2f. For ΔB = 2 T it reaches a maximum value of −ΔSmaxm = 2.098 R (7.28 J kg−1 K−1) at 1.8 K, that is, a 50.4% of the maximum magnetic entropy available (Smax = 4.16 R).
In the case of Gd1.5Eu1.5 MOF, the χT product does not saturate at high temperatures, owing to the Europium contribution. Moreover, the saturation of the M(H, 1.8 K) at 5 T is above the predicted value for 1.5 Gd3+ ions (10.5 μB), and given that Eu3+ is non-magnetic, it implies this MOF contains a small fraction of Eu2+ (S = 7/2) magnetic ions. Indeed, both the saturation of the χT(T) plot and the M(H, 1.8 K) curve could be well reproduced considering a 5% of Eu2+ of all Eu ions. The presence of Eu2+ in these synthesized MOFs arises from impurities in the precursor salts,15 and the Eu2+/Eu3+ ratio can be further increase upon X-ray irradiation (vide infra XMCD section).
The magnetic entropy change −ΔSm(T, ΔB) obtained for Gd1.5Eu1.5, see Fig. 2i, reaches −ΔSmaxm = 1.4 R (4.84 J kg−1 K−1) at 1.8 K for a ΔB = 2 T, that is a 43% of the maximum magnetic entropy available (including the small Eu2+ contribution): Smax ≈ (1.5 + 0.05·1.5)Rlog(2·3.5 + 1) = 3.28 R. For GdEuTb MOF, like in the Gd1.5Eu1.5 instance discussed above, the χT(T) and M(H, 1.8 K) curves reflect the presence of a small fraction of Eu2+ ions, see Fig. 2j and k, and both curves can be reasonably reproduced considering a linear combination of the theoretical curves for 1 Tb3+, 0.94 Eu3+, and 0.06 Eu2+ ions. For GdEuTb the determined magnetic entropy change −ΔSm(T, ΔB) reaches −ΔSmaxm = 1.52 R (4.94 J kg−1 K−1) at 1.8 K for a ΔB = 2 T (Fig. 2l), that is a 52% of the maximum available magnetic entropy, Smax ≈ (1 + 0.06)Rlog(2·3.5 + 1) +Rln(2·0.5 + 1) = 2.9 R.
XAS and XMCD characterization
To investigate the magnetic properties and spectroscopic characteristics of the individual ions within the mixed {GdLn} MOF we used element-selective X-ray absorption spectroscopy (XAS) and X-ray magnetic circular dichroism (XMCD) technique. We performed XAS and XMCD measurements across the M4,5 edges of Gd and Ln = Dy, Tb, Eu ions at 3.4 K and 6 T in each {GdLn} MOF. The orbital (mL), spin (ms) and total magnetic moment (mTOT = mL + ms) for each ion were determined from the XAS-XMCD spectra using the corrected sum rules for lanthanides116,117 (see Table S1†). The field-dependence of the total magnetic moment, mtot(H), for each Ln ion was determined by following the intensity of the XMCD(H) peak at the M5 edge between −6 T and 6 T, and scaling the curve with the value of mTOT obtained at 6 T.
Fig. 3 summarizes results for Gd1.5Dy1.5 and Gd1.5Tb1.5, while Fig. 4 displays results for Gd1.5Eu1.5 and GdEuTb. In all compounds, the Gadolinium XAS spectrum exhibits the expected features for trivalent Gd(III), see Fig. 3a, d, 4a and d: the M5 edge shows a shoulder at 1182.4 eV, a main peak at 1184.7 and two satellites at 1189.3 and 1192.0 eV, whereas M4 edge exhibits two peaks at 1213.92, 1215.6 eV and a shoulder at 1218.6 eV. The Gd XMCD spectrum across M5 displays a main negative peak at 1184.85 eV and M4 edge displays a positive pre-peak (1213.07 eV), two main peaks (1214.07, 1215.6 eV) a shoulder at 1216.52 eV and satellite at 1218.93 eV.
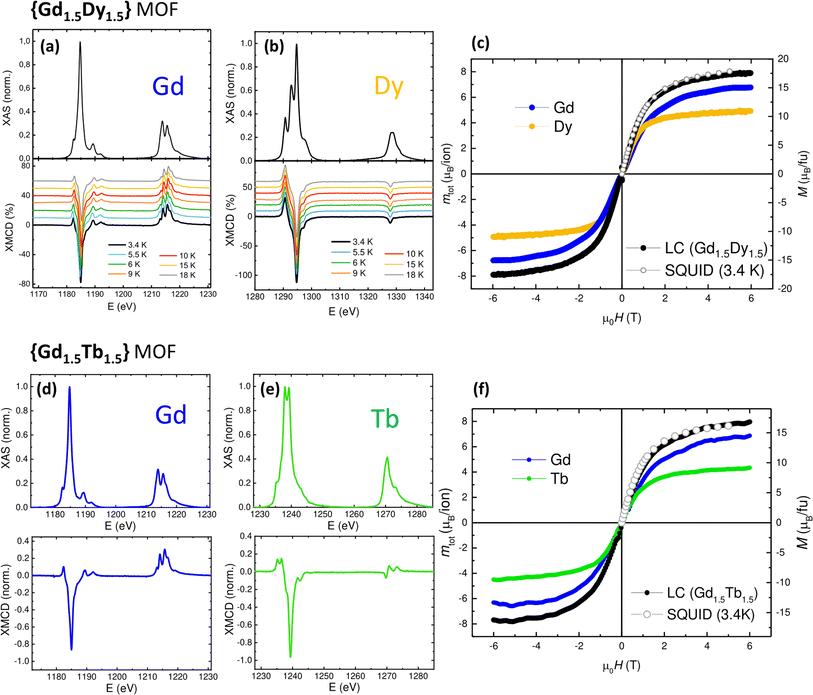 |
| Fig. 3 XAS and XMCD characterization. Gd1.5Dy1.5: normalized, background-subtracted XAS (top) and XMCD (bottom) spectra measured across the M4,5 edge of Gd (a) and Dy (b) at 6 T and indicated temperatures, between 3.4 K and 18 K; (c) field-dependence of the total magnetic moment, mTOT(H)/ion, obtained for Gd3+ and Dy3+, and magnetic moment per formula unit, at 3.4 K. Open symbols show, for comparison, the magnetization curve M(H) of the mixed MOF determined by SQUID at the same temperature; Gd1.5Tb1.5: XAS and XMCD (6 T and 3.4 K) across the M4,5 edges of Gd (d) and Tb (e); (f) mTOT(H)/ion obtained for Gd3+ and Tb3+, calculated magnetic moment per formula unit at 3.4 K (black filled symbols), and SQUID determined M(H) (open symbols). | |
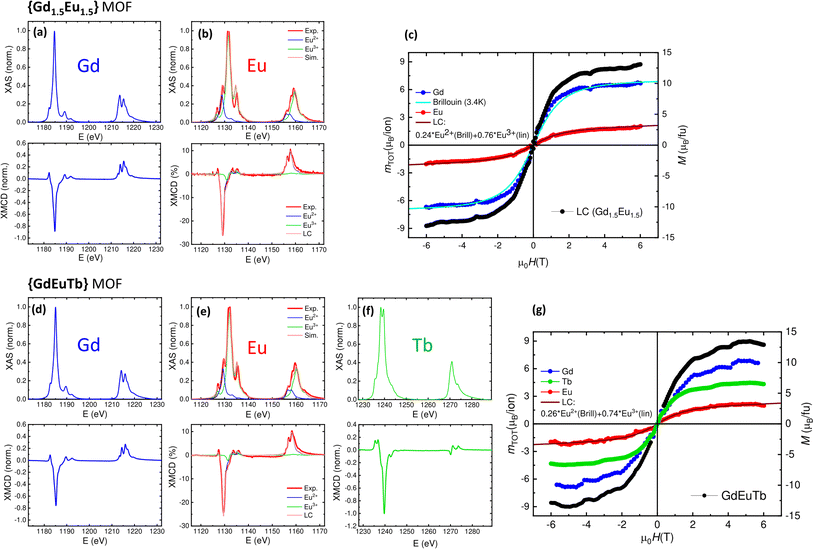 |
| Fig. 4 XAS and XMCD characterization. Gd1.5Eu1.5: normalized, background-subtracted XAS (top) and XMCD (bottom) spectra measured at 6 T at 3.4 K across the M4,5 edge of Gd (a) and (b) Eu (also shown, the simulated spectra for Eu2+ and Eu3+); (c) field-dependence of the total magnetic moment, mTOT(H)/ion, obtained for Gd3+ and Eu, and calculated magnetic moment per formula unit, at 3.4 K. GdEuTb: XAS and XMCD (6 T at 3.4 K) across the M4,5 edges of Gd (d), (e) Eu (also shown, simulated spectra for Eu2+ and Eu3+), and (f) Tb; (g) mTOT(H)/ion obtained for Gd3+, Eu and Tb3+, and calculated magnetic moment per formula unit, at 3.4 K. | |
In Gd1.5Dy1.5, the XAS and XMCD spectra measured at Dysprosium M4,5 edges are typical for Dy(III) (Fig. 3b). The XAS spectrum displays two pre-peaks (1296.71, 1292.88 eV), a main peak (1294.7 eV) at M5 and a shoulder (1297.16 eV), while M4 includes two close peaks (1328.1–1328.9 eV). The XMCD spectra consists of negative peaks, across M5 a main peak at 1294.7 eV and a minor 1294.16 eV, and a small peak at M4 (1327.8 eV). Fig. 3c shows the magnetic field dependence of the total magnetic moment per ion, mTOT(H), obtained at 3.4 K for Gd3+ and Dy3+ in Gd1.5Dy1.5. They coincide with the M(H) curves measured separately in the homonuclear Gd and Dy-MOFs.114 Furthermore, we calculated the magnetic moment per formula unit, mGd1.5Dy1.5 (H), by taking a weighted linear combination (LC) of the mTOT(H) for each ion: mGd1.5Dy1.5 = 1.5·mTOT(H)/ion(Gd3+) + 1.5·mTOT(H)/ion(Dy3+). This calculated value is in excellent agreement with the magnetization curve, M(H), measured by SQUID magnetometry for the mixed Gd1.5Dy1.5 MOF, at the same temperature (3.4 K).
As for Gd1.5Tb1.5, the XAS and XMCD spectra at Terbium M4,5 edges show the typical feature expected for Tb(III) (Fig. 3e). The XAS spectrum exhibits a pre-peak (1235.19 eV) and two main peaks (1237.99 eV) and shoulder (1242.99 eV) in the M5 region, while M4 consists of a peak at 1270.53 eV and shoulder (1273.2 eV). The XMCD spectra contain a primary negative peak at 1239.3 eV in the M5 region and a minor peak at 1242.37 eV, and three features in the M4 region (at 269.65, 1270.6, 1273.25 eV). Fig. 3f displays the mTOT(H)/ion curves measured at 3.4 K for both Gd3+ and Tb3+ ions in Gd1.5Tb1.5. Similar to the above described compound, these curves align, respectively, with the M(H) curves previously measured in the isostructural pure Gd and Tb MOFs.114,115 Also, the magnetic moment per formula unit, calculated as the weighted LC of the ionic mTOT(H) contributions: mGd1.5Tb1.5 = 1.5·mTOT(H)/ion(Gd3+) + 1.5·mTOT(H)/ion(Tb3+), is in very good agreement with the magnetization SQUID-determined M(H, 3.4 K) curve for Gd1.5Tb1.5.
In the case of Gd1.5Eu1.5, the XAS and XMCD spectra of Europium reveal the coexistence of two valence states, Eu3+ and Eu2+. Notably, the experimental spectra could be successfully reproduced considering the linear combination (LC) of the XAS-XMCD spectra calculated using Ligand Field Multiplet (LFM) simulations, implemented in Quanty–Crispy, for both Eu2+ and Eu3+, in a ratio of 24–76% (refer to Fig. 4b). The presence of both Eu2+ and Eu3+ ions had been already inferred from magnetometric measurements of the mixed MOF (as discussed above, see Fig. 2g and h), and is here confirmed through the application of the XAS-XMCD element-selective technique. However, it is noted that the Eu2+/Eu3+ ratio determined by the synchrotron measurements is higher than that obtained through SQUID (Eu2+/Eu3+ = 0.05/0.95). The reason for this discrepancy may be attributed to the propensity of Eu in these carborane-based MOF to undergo photoreduction upon soft X-ray irradiation, not causing molecular damage.115 For Europium, the XMCD signal arises from the magnetic Eu2+ ions, while the XAS absorption spectrum is associated to all Eu ions. Thus, we calculated the “averaged” magnetic moment per Eu ion in the sample, applying the sum rules with an averaged number holes nh = 7.8, taking into account the experimentally determined ratio Eu2+(Eu3+) = 0.24(0.76), and the number of holes of each one, nh = 7 (Eu2+), 8 (Eu3+). The mTOT(H)/ion curves determined at 3.4 K both for Gd and Eu ions, together with the magnetic moment calculated per Gd1.5Eu1.5 formula unit are shown in Fig. 4c. The mTOT(H)/ion curve obtained for Gd3+ is in agreement with the Brillouin prediction at 3.4 K, while the mTOT(H)/ion per average Eu coincides with theoretical curve, calculated as the LC of 24% of Eu2+ ions (following a Brillouin function) and 76% of Eu3+.
In trinuclear GdEuTb MOF the XAS and XMCD spectra of Europium again show the coexistence of Eu3+ and Eu2+, in a ratio of Eu2+/Eu3+ = 0.26/0.74, estimated from LFM simulations (Fig. 4e), very similar to that found in the above discussed Gd1.5Eu1.5 instance. The XAS-XMCD spectra across the M4,5 edge of Terbium (Fig. 4f) corresponds to Tb(III) and are similar to the spectra described for Tb dinuclear Gd1.5Tb1.5. The mTOT(H)/ion curves at 3.4 K obtained for Gd, Eu and Tb ions, along the magnetic moment calculated per GdEuTb formula unit are plotted in Fig. 4g. Once again, the mTOT(H)/ion curve obtained for Gd3+ aligns with the Brillouin prediction at 3.4 K, the mTOT(H)/ion for Tb3+ is similar to the curve measured for Tb-MOF and Tb in dinuclear Gd1.5Tb1.5, and the mTOT(H)/ion per Eu coincides with the theoretical curve, calculated as a weighted combination of Eu2+ and Eu3+ contribution, with the experimentally determined ratio.
MCE at atomic and macroscopic level
XMCD measurements were further used to characterize the MCE performance at atomic level, and compare it with the global MCE observed for the mixed MOF. The procedure is illustrated in Fig. 5, for the case of Gd1.5Dy1.5. XMCD(H) cycles at different fixed temperatures between 3.4 K and 18 K were measured separately for Gd3+ and Dy3+. XAS-XMCD spectra at 6 T and each fixed temperature were performed (see Fig. 3a and b), to scale the XMCD(H) curves and obtain mTOT(H)/ion isotherms both for Gd3+ (Fig. 5a) and Dy3+ (Fig. 5c). These figures show also, for comparison, the M(H, T) curves for Gd3+ ions, according to Brillouin predictions (Fig. 5a, lines), and M(H) isotherms measured by SQUID for Dy-MOF (Fig. 5c, lines). Maxwell method was then used to obtain, separately, the magnetic entropy change curves, −ΔSm(T) for different ΔB values, for Gd3+ (Fig. 5b) and Dy3+ (Fig. 5d). Despite the error committed in the determination of −ΔSm(T) from XMCD, associated to some thermal drift at intermediate temperatures, and the differentiation steps involved in Mawell method, the obtained cationic −ΔSm(T) are close to the expected curves for homonuclear Gd-MOF and Dy-MOFs (also represented as continuous lines in Fig. 5b and d, for comparison purposes).
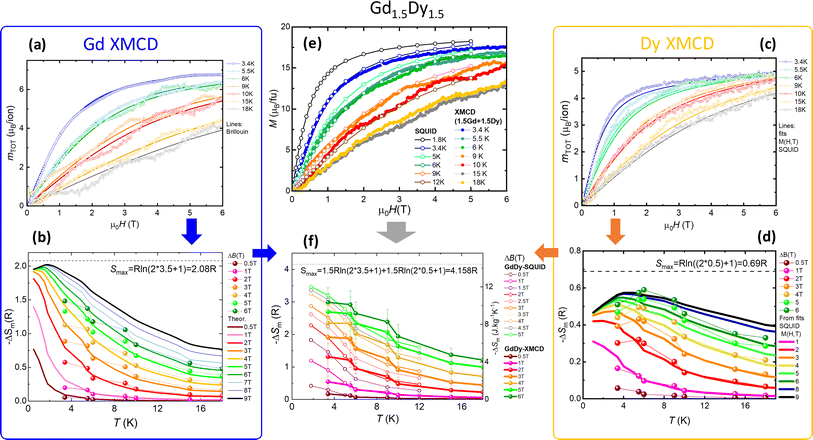 |
| Fig. 5 MCE at atomic and macroscopic level. Lateral panels: mTOT(H)/ion curves determined by XMCD at different temperatures for Gd3+ (a) and Dy3+ (c), and derived magnetic entropy change curves, −ΔSm(T, H), for Gd3+ (b) and Dy3+ (d). Central panel: (e) magnetization per formula unit as a function of field at different temperatures, M(H, T), obtained as the linear combination of XMCD-obtained mTOT(H)/ion curves (bold symbols) and measured by SQUID (open symbols); (f) total magnetic entropy change −ΔSm(T, H) per formula unit, calculated as the linear combination of the individual cationic contributions (bold symbols). For comparison, the −ΔSm(T, H) obtained from the M(T, H) curves of Gd1.5Dy1.5 MOF are shown (open symbols). In (b), (d) and (f) the dotted lines indicate the maximum available entropy. | |
The total magnetic moment per Gd1.5Dy1.5 formula unit, obtained from the LC of mTOT(H)/ion contributions at different temperatures is shown in Fig. 5e (bold symbols), along the M(H, T) curves obtained by magnetometry (open symbols). A good agreement is observed for those curves measured in similar T conditions.
Finally, the macroscopic magnetic entropy change −ΔSm(T, H) per formula unit, calculated as the LC of the individual cationic contributions obtained for Gd and Dy, is shown in Fig. 5f (bold symbols). The obtained curves are in reasonably agreement with the −ΔSm(T, H) directly determined from the M(T, H) curves of Gd1.5Dy1.5 MOF (shown in Fig. 5f as open symbols), within the associated errors of the procedure. These results showcase two aspects. On the one hand, they underscore the potential of XMCD technique for investigating the individual MCE performance of distinct ions within magnetically complex materials. On the other, they reveal that in these mixed {GdLn} MOFs, the MCE is the sum of the separate ions' contributions, indicating an additive behavior.
Dynamic magnetic properties
To characterize the spin magnetic relaxation properties of the synthesized {GdLn} MOFs, we performed ac susceptibility measurements as a function of the frequency, temperature and magnetic field. Fig. 6 (left) shows the out-of-phase component of the susceptibility as a function of the frequency, at constant μ0H = 0.3 T and different temperatures between 1.8 K–20 K, χ′′(f, T), while Fig. 6 (right) plots the χ′′(f, H) curves, at fixed T = 1.8 K and different applied magnetic fields in the 0–1 T range. From these data, the spin relaxation time for the different relaxation processes observed were obtained. Fig. 6g and h show, respectively, the dependence of the relaxation time with the inverse of the temperature, τ(1/T), and the magnetic field, τ(H), obtained for the different process in all studied {GdLn} MOFs. Results are discussed in the light of measurements previously reported for homonuclear Gd, Tb and Dy MOFs.114,115
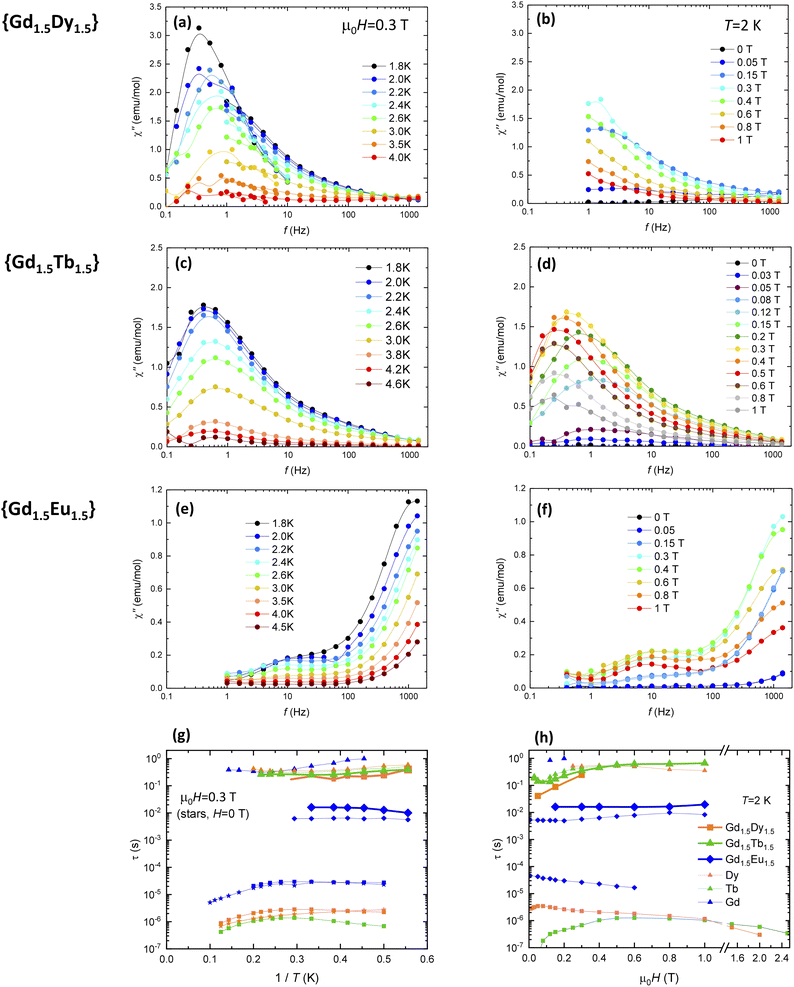 |
| Fig. 6 Spin magnetic relaxation of mixed {GdLn} MOFs. (Left: a, c, e and g): imaginary component of the ac susceptibility as a function of the frequency, χ′′(f), at μ0H = 0.3 T, and (right: b, d, f and h): at T = 2 K and different applied magnetic fields. (Bottom): (g) magnetic relaxation time as a function of the inverse temperature (1/T) at μ0H = 0.3 T and (h) as a function of the applied magnetic field at T = 2 K, for the different relaxation processes observed. For comparison, relaxation times previously reported for homonuclear Gd, Tb and Dy-MOFs are shown (open symbols).114,115 Additional relaxation results for {GdTbEu} MOF are shown in Fig. S4.† | |
For Gd1.5Dy1.5 MOF, under the application of a μ0H = 3 T magnetic field, a slow relaxation process at f < 1 Hz, and a second process is hinted at the highest measured f = 1 kHz (Fig. 6a and b). Both the χ′′(f, T, 0.3 T) and χ′′(f, H, 2 K) resemble the curves measured for the homonuclear analogue Dy-MOF, though the curves in the mixed MOF are slightly shifted toward lower frequencies. The relaxation peaks associated to Gd are not observed, possibly masked by the much larger intensity of the Dy ones.
Similarly, in the case of Gd1.5Tb1.5 MOF the χ′′(f, T, 0.3 T) and χ′′(f, H, 2 K) curves display a single very low frequency peak (Fig. 6b and c), with τ(1/T), and τ(H) dependencies similar to those previously observed for homonuclear Tb-MOF, which can be associated to a direct process. Like in the previous case, relaxation is dominated by the anisotropic ion, and the processes associated to Gd are not observed.
In contrast, in Gd1.5Eu1.5 MOF, the χ′′(f, T, H) behavior (Fig. 6d and e) is very similar to that previously observed for Gd-MOF,115 and evidences the single-ion relaxation of the Gd ions in the mixed MOF through multiple processes. The first relaxation slow process has relaxation time values in the order of τ1 ≈ 0.1 to 1 s. The τ1(1/T) dependence at 0.3 T could be fit to an Arrhenius law with an activation energy of U/kB = 12.1 K, of the same order as the levels splitting. The second slowest relaxation process, with a T and H independent relaxation time constant τ2 ≈ 0.016 s may be associated to a direct process. The third, temperature-dependent relaxation process (τ3) may be ascribed to a Resonant Phonon Trapping (RPT) mechanism.118–120
Finally, the dynamic behavior of trinuclear GdTbEu MOF (Fig. S4†) is very similar to that of previously discussed Gd1.5Tb1.5, i.e. it is dominated by the intensity of Tb relaxation.
Optical properties
The absorption spectra of the new {GdLn} MOFs exhibit a slight broadening of the UV band, akin to that observed for the free ligand mCB-L and reminiscent of the UV absorption bands in previous mono- and multi-metallic MOFs (Fig. S3†). The emission spectra in the visible region for powdered samples of all the studied mixed {GdLn} MOFs under light irradiation (λex = 280 nm; air atmosphere; RT) are shown in Fig. 7 and Table S2.† No blue emission originating from the ligand is detected. The spectra of Gd1.5Dy1.5, Gd1.5Tb1.5 and Gd1.5Eu1.5 show solely the characteristic emission peaks for, respectively, Dy3+ (λemmax = 572 nm), Tb3+ (λemmax = 542 nm, deriving from 5D4 → 7FJ (J = 6–3)) and Eu3+ (λemmax = 614 nm, 5D0 → 7FJ; J = 1–4),20 while the spectrum of trinuclear GdEuTb includes the peaks of both Eu3+ and Tb3+, with resulting emission color ranging from green (Gd1.5Tb1.5), red (Gd1.5Eu1.5), to greenish (Gd1.5Dy1.5) and yellow (GdEuTb), see Fig. 7 (insets) and S5.† In the case of the tri-metallic GdEuTb MOF, the emitted color is due to the combination of the two extreme colors (green and red) for the individual Tb3+ and Eu3+ lanthanides, respectively (Fig. 7).15 No emission is observed from Gd. This fact is justified by the fact that the calculated triplet state (T1) energy of mCB-L is not enough to sensitize the Gd. The measured quantum yield (QY) values (Table S2†) of all MOFs are 0.2% (Gd1.5Dy1.5), 7.3% (Gd1.5Tb1.5), 4.2% (Gd1.5Eu1.5) and 4.5% (GdEuTb). The emission of the Tb/Eu-containing MOFs (Gd1.5Tb1.5, Gd1.5Eu1.5, GdEuTb), are dominated by Tb3+ and Eu,3 but have significantly lower QY than the values previously reported for carborane-based pure Tb (49.8%), Eu (20.5%) and mixed Tb0.5Eu0.5 (42.5%) MOFs.20 We speculate that the energy of the excited carboranes next to the Gd ions is lost through non-radiative pathways, not contributing to the sensitization of the Tb, Eu or Dy atoms, reducing the overall MOF quantum yield. Mono-exponential fitting of the slower part of the decay curves was carried out to obtain the longest luminescence lifetimes, while avoiding possible background interferences (Fig. S6†). The obtained lifetimes for the Gd1.5Dy1.5 (37.3 μs), Gd1.5Tb1.5 (872.5 μs), Gd1.5Eu1.5 (739.1 μs) and GdEuTb (744.1 for Eu3+, 867.1 for Tb3+) are nearly identical to those of the individual Dy-MOF (41.7 μs), Tb-MOF (849.7 μs) and Eu-MOF (739.0 μs),20 suggesting no energy transfer (ET) occurs between Ln ions. Since no rise is observed in the first part of the decay signal, we conclude that no sensitization from one lanthanide to the other is occurring, nor even from the non-emitting Gd, as consequence of the fact that it was not sensitized by the carborane. Furthermore, contrarily to that observed in previous carborane-based binary Eu/Tb MOFs, in the trinuclear GdEuTb, there is no ET between Eu and Tb ions, indicating that the inclusion of Gd separating the ions hinders the energy transfer.
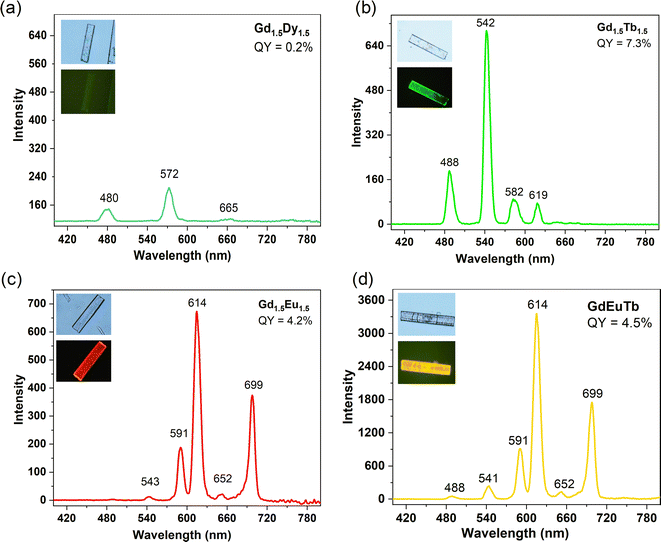 |
| Fig. 7 Luminescent properties of mixed {GdLn} MOFs. Solid-state emission spectra of Gd1.5Dy1.5 (a), Gd1.5Tb1.5 (b), Gd1.5Eu1.5(c) and GdEuTb (d) under continuous-wave irradiation (λexc = 280 nm) at room temperature. (Insets): Quantum yields (QY) and optical microscopy images of the corresponding crystals under white and the corresponding luminescence images. | |
Discussion
Finally, we discuss the multifunctional properties of the new carborane-based mixed {GdLn} MOFs, in view of the above described magneto-thermal and optical characterization results. All {GdLn} compounds exhibit MCE, with entropy change values −ΔSm at 1.8 K and ΔB = 2–0 T, in decreasing order, of 7.90, 7.28, 4.94 and 4.84 J kg−1 K−1 for Gd1.5Dy1.5, Gd1.5Tb1.5, GdEuTb and Gd1.5Eu1.5. Although, as expected, these values are smaller than those of homonuclear Gd MOF, the mixed MOFs have interesting added functionalities. Both Gd1.5Tb1.5 and Gd1.5Dy1.5 MOFs exhibit field-induced slow relaxation of the magnetization arising from the anisotropic Ln = Dy and Tb SMM units, associated to a direct process. Since the relaxation time associated to this mechanism is essentially field-independent above 2 T, the MCE may be used to refrigerate the SMM units. However, it should be noted that for the cooling procedure, a change of magnetic field ΔB = Bf − Bi with Bi ≠ 0 should be used, since no relaxation is observed in the absence of field (Fig. 6h). In addition, Gd1.5Tb1.5 displays green luminescent emission, while Gd1.5Dy1.5 MOF's emission is greenish but its quantum yield is quenched. On the other hand, Gd1.5Eu1.5 exhibits slow magnetic relaxation arising from Gd, with a relaxation time τ ∼ 0.02 s that could be maintained upon a refrigeration cycling under ΔB = Bf − Bi with Bi > 2 T, and displays red luminescence. Finally, GdEuTb MOF exhibits MCE along yellow luminescent emission.
Conclusions
The synthesis and full structural, magneto-thermal and optical properties of new carborane-based mixed MOFs {[(GdLn)3(mCB-L)4(NO3)(DMF)x]n·Solv} incorporating Ln = Dy, Tb, or Tb/Eu have been reported. The use of the mCB-L ligand allows the synthesis of isostructural multivariate MOFs combining any tailored combination of lanthanides with desired ratios, and therefore is ideal for the preparation of multifunctional materials. Herein we explored the potentiality of combining Gd, providing the largest change of entropy for magnetocalorics, with different lanthanides introducing added functionalities. We demonstrate that Gd1.5Ln1.5 (Ln = Tb, Dy) constitute multifunctional MOFs incorporating MCE, field-induced slow relaxation of the magnetization, and green emission, in the case of Ln = Tb. Conversely, Gd1.5Ln1.5 (Ln = Eu, Eu/Tb) exhibit MCE, field-induced slow relaxation of the magnetization for Ln = Gd, and red/yellow luminescent emission for Ln = Eu and Eu/Tb substitutions, respectively. Interestingly, the coexistence of MCE and SMM properties would permit “self-refrigeration” of the magnetic units, using an applied magnetic field change with non-zero smallest field. Although in these MOFs magnetic bifunctionality was not observed, the concept of self-refrigerated nanomagnets in mixed MOFs is worth pursuing.
Previous studies of mixed Gd/Ln MOFs had focused either on the luminescent or magnetocaloric properties of the compounds. Our comprehensive study of the optical, thermal, dc and ac magnetic properties has allowed us to establish how the macroscopic properties of the {GdLn} MOFs benefit from those of the constituting elements. Macroscopic magnetometry measurements allowed characterizing the magnetic and MCE behaviour of the mixed MOFs, while element-selective XAS-XMCD was crucial for analyzing the magnetic properties of the individual Ln ions. The use of this rarely employed technique for MOF characterization allowed us to show how global MCE properties emerge from the contributions of constituent lanthanides. This novel methodology holds promise to advance research of other multimetallic magnetocaloric materials, including high-entropy (HE) alloys, with significant potential for energy-efficient refrigeration.
We have shown that in these carborane-based mixed MOFs the static magnetic and MCE properties results from the additive contribution of the constituent Ln elements. The dynamic properties of Gd1.5Ln1.5 (Ln = Dy, Tb, Tb/Eu) are dominated by the anisotropic Ln = Tb, Dy, while those of Gd1.5Eu1.5 reflect the relaxation of the magnetic Gd ion. On the other hand, color tuning ranging from green (Gd1.5Tb1.5), red (Gd1.5Eu1.5), to greenish (Gd1.5Dy1.5) and yellow (GdEuTb) was achieved through the combination of lanthanide ions, although quantum yield efficiency was diminished with respect to that of homonuclear MOFs due to the presence of Gd ion.
In conclusion, our innovative synthetic approach for preparing multi-lanthanide MOFs not only expands the horizons for investigating “complex magnetic materials”, but also paves the way for developing multifunctional MOFs endowed with tailored or unforeseen properties. Specifically, {GdLn} MOFs offer the attractive possibility of organizing self-refrigerated SMM memory units and “qubits”, for high density information storage and quantum computing applications, requiring operation at cryogenic temperatures.
At present, Artificial Intelligence (AI) algorithms are already being exploited in the realm of high-entropy alloys, comprising five or more metals, to predict materials with novel synergistic properties.121,122 Looking ahead, we anticipate the application of AI in uncovering new multi-lanthanide MOFs that exhibit compelling characteristics. However, this Machine Learning approach is contingent on the prior synthesis and thorough characterization of a substantial number of MOFs encompassing various combinations of lanthanides. Our work stands as a milestone in this promising direction, laying the foundation for future advancements in the discovery of innovative multi-lanthanide MOFs with remarkable properties.
Data availability
Data supporting this article have been included as part of the ESI.†
Author contributions
ZL, JGP synthesis, XRD, SEM-EDX, luminescent investigation and data curation; CR luminescence resources and writing; AA magnetometry resources, EB investigation and data curation; EB MCE and XAS-XMCD investigation and data curation; EB writing – original draft; JGP writing – review; EB conceptualization; EB and JGP supervision, resources, project administration. All authors have given approval to the final version of the manuscript.
Conflicts of interest
There are no conflicts to declare.
Acknowledgements
This work was financially supported by MINECO (PID2022-138492NB-I00 and PID2022-136892NB-I00), the Generalitat de Catalunya (2021/SGR/00442), and the Gobierno de Aragón (RASMIA E12-23). EB and JGP acknowledges financial support from the State Investigation Agency, through the Severo Ochoa Programme for Centres of Excellence in R&D (CEX2023-001263-S). Zhen Li acknowledges the China Scholarship Council (CSC) for his PhD grant (201808310071). Authors would like to acknowledge the use of Servicio General de Apoyo a la Investigación-SAI, Universidad de Zaragoza. XAS and XMCD experiments were performed at the BOREAS beamline of the ALBA synchrotron with the support of ALBA staff (experiment number 2022085925).
References
- X. J. Wang, X. Zhang, R. Pandharkar, J. F. Lyu, D. Ray, Y. Yang, S. Kato, J. Liu, M. C. Wasson, T. Islamoglu, Z. Li, J. T. Hupp, C. J. Cramer, L. Gagliardi and O. K. Farha, ACS Catal., 2020, 10, 8995–9005 CrossRef CAS.
- D. Q. Yuan, D. Zhao, D. F. Sun and H. C. Zhou, Angew. Chem., 2010, 49, 5357–5361 CrossRef CAS PubMed.
- X. Zhang, V. Vieru, X. Feng, J.-L. Liu, Z. Zhang, B. Na, W. Shi, B.-W. Wang, A. K. Powell, L. F. Chibotaru, S. Gao, P. Cheng and J. R. Long, Angew. Chem., 2015, 54, 9861–9865 CrossRef CAS PubMed.
- K. Liu, X. Zhang, X. Meng, W. Shi, P. Cheng and A. K. Powell, Chem. Soc. Rev., 2016, 45, 2423–2439 RSC.
- G. Mínguez Espallargas and E. Coronado, Chem. Soc. Rev., 2018, 47, 533–557 RSC.
- R. E. Sikma, K. S. Butler, D. J. Vogel, J. A. Harvey and D. F. Sava Gallis, J. Am. Chem. Soc., 2024, 146, 5715–5734 CrossRef CAS PubMed.
- W. Jiang, C.-C. Liang and Y.-B. Zhang, Adv. Funct. Mater., 2024, 2308946 CrossRef.
- M. Viciano-Chumillas, X. Liu, A. Leyva-Pérez, D. Armentano, J. Ferrando-Soria and E. Pardo, Coord. Chem. Rev., 2022, 451, 214273 CrossRef CAS.
- C. Castillo-Blas, V. A. De la Peña-O’Shea, I. Puente-Orench, J. Romero de la Paz, R. Sáez-Puche, R. Gutierrez-Puebla and F. Gándara, Sci. Adv., 2017, 3, e1700773 CrossRef PubMed.
- K. L. S. Abednatanzi, P. G. Derakhshandeh, H. Depauw, F.-X. Coudert, H. Vrielinck and P. Van Der Voort, Chem. Soc. Rev., 2019, 48, 2535–2565 RSC.
- Y. Wang, Y.-Y. Xue, L.-T. Yan, H.-P. Li, Y.-P. Li, E.-H. Yuan, M. Li, S.-N. Li and Q.-G. Zhai, ACS Appl. Mater. Interfaces, 2020, 12, 24786 CrossRef CAS PubMed.
- L. J. Wang, H. Deng, H. Furukawa, F. Gándara, K. E. Cordova, D. Peri and O. M. Yaghi, Inorg. Chem., 2014, 53, 5881 CrossRef CAS PubMed.
- X. Zhao, Z. Xue, W. Chen, X. Bai, R. Shi and T. Mu, J. Mater. Chem. A, 2019, 7, 26238 RSC.
- R. L. Vasile, A. A. Godoy, I. Puente Orench, N. M. Nemes, V. A. de la Peña O'Shea, E. Gutiérrez-Puebla, J. L. Martínez, M. Á. Monge and F. Gándara, Chem. Mater., 2022, 34, 7029–7041 CrossRef CAS PubMed.
- Z. Li, X.-B. Li, M. E. Light, A. E. Carrillo, A. Arauzo, M. Valvidares, C. Roscini, F. Teixidor, C. Viñas, F. Gándara, E. Bartolomé and J. G. Planas, Adv. Funct. Mater., 2023, 33, 2307369 CrossRef CAS.
- R. L. Vasile, R. S. J. Silva, E. Céspedes, J. L. Martínez, E. Gutiérrez-Puebla, M. A. Monge and F. Gándara, Inorg. Chem., 2023, 62, 19741–19748 CrossRef CAS PubMed.
- E. Bartolomé, A. Arauzo, S. Fuertes, L. Navarro-Spreafica, P. Sevilla, H. F. Cortés, N. Settineri, S. J. Teat and E. C. Sañudo, Dalton Trans., 2023, 52, 7258–7270 RSC.
- Y. Cui, B. Chen and G. Qian, Coord. Chem. Rev., 2014, 273–274, 76–86 CrossRef CAS.
- Z. Qu, D. Wu, J. Jin, G. Yang and Y.-Y. Wang, J. Solid State Chem., 2022, 309, 123003 CrossRef CAS.
- Z. Li, R. Núñez, M. E. Light, E. Ruiz, F. Teixidor, C. Viñas, D. Ruiz-Molina, C. Roscini and J. G. Planas, Chem. Mater., 2022, 34, 4795–4808 CrossRef CAS PubMed.
- Y. Guo, Z. Han, H. Min, Z. Chen, T. Sun, L. Wang, W. Shi and P. Cheng, Inorg. Chem., 2021, 60, 9192–9198 CrossRef CAS PubMed.
- J.-M. Li, R. Huo, X. Li and H.-L. Sun, Inorg. Chem., 2019, 58, 9855–9865 CrossRef CAS PubMed.
- Q. Lin, W. Xie, Z. Zong, Z. Liu, Y. Sun and L. Liang, New J. Chem., 2021, 45, 7382–7389 RSC.
- S. Mohapatra, B. Rajeswaran, A. Chakraborty, A. Sundaresan and T. K. Maji, Chem. Mater., 2013, 25(25), 1673–1679 CrossRef CAS.
- D. B. Kanzariya, M. Y. Chaudharya and T. K. Pal, Dalton Trans., 2023, 52, 7383–7404 RSC.
- G. Lorusso, J. W. Sharples, E. Palacios, O. Roubeau, E. K. Brechin, R. Sessoli, A. Rossin, F. Tuna, E. J. L. McInnes, D. Collison and M. Evangelisti, Adv. Mater., 2013, 25, 4653–4656 CrossRef CAS PubMed.
- G. Lorusso, E. Natividad, M. Evangelisti and O. Roubeau, Mater. Horiz., 2019, 6, 144–156 RSC.
- M. Falsaperna and P. Saines, Dalton Trans., 2022, 51, 3394–3410 RSC.
- P. Konieczny, W. Sas, D. Czernia, A. Pacanowska, M. Fitta and R. Pełka, Dalton Trans., 2022, 51, 12762–12780 RSC.
- C.-L. Chen, C. Wang, X.-Y. Zheng, R. Zhang, Y. Xu, G.-L. Zhuang, L.-S. Long, L.-S. Zheng, X.-J. Kong and Y. Cao, J. Am. Chem. Soc., 2023, 145, 16983–16987 CrossRef CAS PubMed.
- E. Coronado, Nat. Rev. Mater., 2020, 5, 87–104 CrossRef.
- M. J. Graham, J. M. Zadrozny, M. S. Fataftah and D. E. Freedman, Chem. Mater., 2017, 29, 1885–1897 CrossRef CAS.
- N. Ishikawa, M. Sugita, T. Ishikawa, S. Y. Koshihara and Y. Kaizu, J. Am. Chem. Soc., 2003, 125, 8694–8695 CrossRef CAS PubMed.
-
E. Bartolomé, A. Arauzo, J. Luzón, J. Bartolomé and F. Bartolomé, in Handbook of Magnetic Materials, ed. E. Brück, Elsevier, 2017, pp. 1–289 Search PubMed.
-
L. Sorace and D. Gatteschi, Electronic Structure and Magnetic Properties of Lanthanide Molecular
Complexes, ed. R. Layfield and M. Murugesu, Wiley-VCH, 2015 Search PubMed.
- Z. Zhu, C. Zhao, T. Feng, X. Liu, X. Ying, X.-L. Li, Y.-Q. Zhang and J. Tang, J. Am. Chem. Soc., 2021, 143, 10077–10082 CrossRef CAS PubMed.
- F. S. Guo, B. M. Day, Y. C. Chen, M. L. Tong, A. Mansikkamäki and R. A. Layfield, Science, 2018, 362, 1400–1403 CrossRef CAS PubMed.
- A. Gaita-Ariño, F. Luis, S. Hill and E. Coronado, Nat. Chem., 2019, 11, 301–309 CrossRef PubMed.
- M. D. Jenkins, Y. Duan, B. Diosdado, J. J. García-Ripoll, A. Gaita-Ariño, C. Giménez-Saiz, P. J. Alonso, E. Coronado and F. Luis, Phys. Rev. B, 2017, 95, 064423 CrossRef.
- F. Luis, P. J. Alonso, O. Roubeau, V. Velasco, D. Zueco, D. Aguilà, J. I. Martínez, L. A. Barrios and G. Aromí, Commun. Chem., 2020, 3, 176 CrossRef CAS PubMed.
- M. Urdampilleta, S. Klyatskaya, M. Ruben and W. Wernsdorfer, Phys. Rev. B: Condens. Matter Mater. Phys., 2013, 87, 195412 CrossRef.
- C. Godfrin, A. Ferhat, R. Ballou, S. Klyatskaya, M. Ruben, W. Wernsdorfer and F. Balestro, Phys. Rev. Lett., 2017, 119, 187702 CrossRef CAS PubMed.
- C. Zhang, X. Ma, P. Cen, X. Jin, J. Yang, A. Y.-Q. Zhang, J. Ferrando-Soria, E. Pardo and X. Liu, Dalton Trans., 2020, 49, 14123–14132 RSC.
- L. Li, Y. Fang, S. Liu, M. Hu and W. Wang, J. Rare Earths, 2023, 41, 100–107 CrossRef CAS.
- C. Bai, C.-T. Li, H.-M. Hu, B. Liu, J.-D. Li and G. Xue, Dalton Trans., 2019, 48, 814–817 RSC.
- Z. Chen, B. Zhao, P. Cheng, X. Q. Zhao, W. Shi and Y. Song, Inorg. Chem., 2009, 48, 3493–3495 CrossRef CAS PubMed.
- T. Yang, S. Wang, C. Lin, X. Wang, B. Zhu and D. Wu, Dalton Trans., 2021, 50, 1293–1299 RSC.
- A. Calahorro, I. Oyarzabal, B. Fernandez, J. Seco, T. Tian and D. Fairen-Jimenez, Dalton Trans., 2016, 45, 591–598 RSC.
- R. Gao, F. Guo, N. Bai, Y. Wu, F. Yang and J. Liang, Inorg. Chem., 2016, 55, 11323–11330 CrossRef CAS PubMed.
- J. López-Cabrelles, L. Escalera-Moreno, Z. Hu, H. Prima-García, G. Mínguez-Espallargas, A. Gaita-Ariño and E. Coronado, Inorg. Chem., 2021, 60, 8575–8580 CrossRef PubMed.
- A. Urtizberea, E. Natividad, P. J. Alonso, M. A. Andrés, I. Gascón, M. Goldmann and O. Roubeau, Adv. Funct. Mater., 2018, 28, 1801695 CrossRef.
- Y. Zhang, W. Hao, C. Hu, X. Wang, X. Zhang and L. Li, Adv. Funct. Mater., 2023, 33, 2310047 CrossRef CAS.
- C. Romero-Muñiz, J. Y. Law, J. Revuelta-Losada, L. Moreno-Ramírez and V. Franco, Innovation Mater., 2023, 1, 100045 CrossRef.
- Y. Zhang, W. Hao, J. Shen, Z. Mo, T. Gottschall and L. Li, Acta Mater., 2024, 276, 120128 CrossRef CAS.
- P. W. Doheny, J. Chen, T. Gruner, F. M. Groscheb and P. J. Saines, J. Mater. Chem. A, 2023, 11, 26474–26480 RSC.
- Y. Zhang, Y. Tian, Z. Zhang, Y. Jia, B. Zhang, M. Jiang, J. Wang and Z. Ren, Acta Mater., 2022, 226, 117669 CrossRef CAS.
- Y. Zhang, J. Zhu, S. Li, Z. Zhang, J. Wang and Z. Ren, Sci. China Mater., 2022, 65, 1345 CrossRef CAS.
-
J. Y. Law and V. Franco, in Handbook on the Physics and Chemistry of Rare Earths, 2023, vol. 64, pp. 175–246 Search PubMed.
- L. Li and M. Yan, J. Mater. Sci. Technol., 2023, 136, 1–12 CrossRef CAS.
- L. Li and M. Yan, Alloys Compd., 2020, 823, 153810 CrossRef CAS.
- R. J. C. Dixey and P. J. Saines, Inorg. Chem., 2018, 57, 12543–12551 CrossRef CAS PubMed.
- P. J. Saines, J. A. M. Paddison, P. M. M. Thygesen and M. G. Tucker, Mater. Horiz., 2015, 2, 528–535 RSC.
- P. W. Doheny, S. J. Cassidy and P. J. Saines, Inorg. Chem., 2022, 61, 4957–4964 CrossRef CAS PubMed.
- S. Kim, R. Muhammad, K. Son and H. Oh, Inorg. Chem., 2023, 62, 2994–2999 CrossRef CAS PubMed.
- J. González, P. Sevilla, G. Gabarró-Riera, J. Jover, J. Echeverría, S. Fuertes, A. Arauzo, E. Bartolomé and E. C. Sañudo, Angew. Chem., 2021, 60, 12001–12006 CrossRef PubMed.
- E. Bartolomé, A. Arauzo, S. Herce, A. Palau, N. Mestres, S. Fuertes, P. Sevilla, N. S. Settineri, L. Navarro-Spreafico, J. González and E. C. Sañudo, Molecules, 2021, 26, 5503 CrossRef PubMed.
- F.-G. Chen, W. Xu, J. Chen, H.-P. Xiao, H.-Y. Wang, Z. Chen and J.-Y. Ge, Inorg. Chem., 2022, 61, 5388–5396 CrossRef CAS PubMed.
- X.-Q. Ji, R. Sun, J. Xiong, H.-L. Sun and S. Gao, J. Mater. Chem. C, 2021, 9, 15858–15867 RSC.
- A. Arauzo, L. Gasque, S. Fuertes, C. Tenorio, S. Bernès and E. Bartolomé, Dalton Trans., 2020, 49, 13671–13684 RSC.
- H. Brunckova, E. Mudra, L. Rocha, E. Nassar, W. Nascimento, H. Kolev, M. Lisnichuk, A. Kovalcikova, Z. Molcanova, M. Strečkova and L. Medvecky, Inorganics, 2021, 9, 77 CrossRef CAS.
- X. Fan, S. Freslon, C. Daiguebonne, G. Calvez, L. Le Pollès, K. Bernot and O. Guillou, J. Mater. Chem. C, 2014, 2, 5510–5525 RSC.
- A. E. Psalti, D. Andriotou, S. A. Diamantis, A. Chatz-Giachia, A. Pournara, M. J. Manos, A. Hatzidimitriou and T. Lazarides, Inorg. Chem., 2022, 61, 11959–11972 CrossRef CAS PubMed.
- Y. Zheng, K. Liu, X. Sun, R. Guan, H. Su, H. You and C. Qi, CrystEngComm, 2015, 17, 2321 RSC.
- T. Alammar, I. Z. Hlova, S. Gupta, A. Biswas, T. Ma, L. Zhou, V. Balema, V. K. Pecharsky and A.-V. Mudring, New J. Chem., 2020, 44, 1054 RSC.
- H. Brunckova, E. Mudra, L. Rocha, E. Nassar, W. Nascimento, H. Kolev, A. Kovalcikova, Z. Molcanova, M. Podobova and L. Medvecky, Appl. Surf. Sci., 2021, 542, 148731 CrossRef CAS.
- J. Rong, W. Zhang and J. Bai, RSC Adv., 2016, 6, 103714 RSC.
- Y. Yang, L. Chen, F. Jiang, M. Yu, X. Wan, B. Zhangab and M. Hong, J. Mater. Chem. C, 2017, 5, 1981 RSC.
- K. Song, W. Xiao, M. He, J. Yu, Y. Bai and Y. Guan, J. Photochem. Photobiol., A, 2020, 389, 112194 CrossRef CAS.
- T. Xia, W. Cao, L. Guan, J. Zhang, F. Jiang, L. Yua and Y. Wan, Dalton Trans., 2022, 51, 5426–5433 RSC.
- A. Cadiau, C. D. S. Brites, P. M. F. J. Costa, R. A. S. Ferreira, J. Rocha and L. D. Carlos, ACS Nano, 2013, 7, 7213–7218 CrossRef CAS PubMed.
- D. Zhao, X. Rao, J. Yu, Y. Cui, Y. Yang and G. Qian, Inorg. Chem., 2015, 54, 11193–11199 CrossRef CAS PubMed.
- T. Xia, J. Wang, K. Jiang, Y. Cui, Y. Yang and G. Qian, Chin. Chem. Lett., 2018, 29, 861–864 CrossRef CAS.
- Y. Zhao and D. Li, J. Mater. Chem., 2020, 8, 12739–12754 CAS.
- B. P. Dash, R. Satapathy, J. A. Maguire and N. S. Hosmane, New J. Chem., 2011, 35, 1955–1972 RSC.
- M. Scholz and E. Hey-Hawkins, Chem. Rev., 2011, 111, 7035–7062 CrossRef CAS PubMed.
- F. Issa, M. Kassiou and L. M. Rendina, Chem. Rev., 2011, 111, 5701–5722 CrossRef CAS PubMed.
- J. Plesek, Chem. Rev., 1992, 92, 269–278 CrossRef CAS.
-
R. N. Grimes, Carboranes, Academic Press, 2016 Search PubMed.
-
F. Teixidor and D. E. Kaufmann, Science of Synthesis: Houben-Weyl Methods of Molecular Transformations, Georg Thieme Verlag, Stuttgart, 5th edn, 2015, vol. 6 Search PubMed.
- S. Fujii, MedChemComm, 2016, 7, 1082–1092 RSC.
- J. F. Valliant, K. J. Guenther, A. S. King, P. Morel, P. Schaffer, O. O. Sogbein and K. A. Stephenson, Coord. Chem. Rev., 2002, 232, 173–230 CrossRef CAS.
- J. Poater, C. Viñas, I. Bennour, S. Escayola, M. Solà and F. Teixidor, J. Am. Chem. Soc., 2020, 142, 9396–9407 CrossRef CAS PubMed.
- J. Poater, M. Solà, C. Viñas and F. Teixidor, Angew. Chem., 2014, 53, 12191–12195 CrossRef CAS PubMed.
- J. Poater, C. Viñas, M. Solà and F. Teixidor, Nat. Commun., 2022, 13, 3844 CrossRef CAS PubMed.
- K. B. Idrees, K. O. Kirlikovali, C. Setter, H. Xie, H. Brand, B. Lal, F. Sha, C. S. Smoljan, X. Wang, T. Islamoglu, L. K. Macreadie and O. K. Farha, J. Am. Chem. Soc., 2023, 145, 23433–23441 CrossRef CAS PubMed.
- L. K. Macreadie, K. B. Idrees, C. S. Smoljan and O. K. Farha, Angew. Chem., Int. Ed., 2023, 62, e202304094 CrossRef CAS PubMed.
- O. K. Farha, A. M. Spokoyny, K. L. Mulfort, M. F. Hawthorne, C. A. Mirkin and J. T. Hupp, J. Am. Chem. Soc., 2007, 129, 12680–12681 CrossRef CAS PubMed.
- O. K. Farha, A. M. Spokoyny, K. L. Mulfort, S. Galli, J. T. Hupp and C. A. Mirkin, Small, 2009, 5, 1727–1731 CrossRef CAS PubMed.
- S.-L. Huang, Y.-J. Lin, W.-B. Yu and G.-X. Jin, Chempluschem, 2012, 77, 141–147 CrossRef CAS.
- Y.-S. Bae, A. M. Spokoyny, O. K. Farha, R. Q. Snurr, J. T. Hupp and C. A. Mirkin, Chem. Commun., 2010, 46, 3478–3480 RSC.
- I. Boldog, P. J. Bereciartua, R. Bulánek, M. Kučeráková, M. Tomandlová, M. Dušek, J. Macháček, D. De Vos and T. Baše, CrystEngComm, 2016, 18, 2036–2040 RSC.
- Y.-S. Bae, O. K. Farha, A. M. Spokoyny, C. A. Mirkin, J. T. Hupp and R. Q. Snurr, Chem. Commun., 2008, 4135 RSC.
- S.-L. Huang, L.-H. Weng and G.-X. Jin, Dalton Trans., 2012, 41, 11657–11662 RSC.
- D. J. Clingerman, W. Morris, J. E. Mondloch, R. D. Kennedy, A. A. Sarjeant, C. Stern, J. T. Hupp, O. K. Farha and C. A. Mirkin, Chem. Commun., 2015, 51, 6521–6523 RSC.
- D. J. Kennedy, R. D. Krungleviciute, V. Clingerman, J. E. Mondloch, Y. Peng, C. E. Wilmer, A. A. Sarjeant, R. Q. Snurr, J. T. Hupp, T. Yildirim, O. K. Farha and C. A. Mirkin, Chem. Mater., 2013, 25, 3539–3543 CrossRef.
- L. Gan, E. Andres-Garcia, G. Mínguez Espallargas and J. G. Planas, ACS Appl. Mater. Interfaces, 2023, 15, 5309–5316 CrossRef CAS PubMed.
- Z. Li, D. Choquesillo-Lazarte, J. Fraile, C. Viñas, F. Teixidor and J. G. Planas, Dalton Trans., 2022, 51, 1137–1143 RSC.
- L. Gan, A. Chidambaram, P. G. Fonquernie, M. E. Light, D. Choquesillo-Lazarte, H. Huang, E. Solano, J. Fraile, C. Viñas, F. Teixidor, J. A. R. Navarro, K. C. Stylianou and J. G. Planas, J. Am. Chem. Soc., 2020, 142, 8299–8311 CrossRef CAS PubMed.
- S. Rodríguez-Hermida, M. Y. Tsang, C. Vignatti, K. C. Stylianou, V. Guillerm, J. Pérez-Carvajal, F. Teixidor, C. Viñas, D. Choquesillo-Lazarte, C. Verdugo-Escamilla, I. Peral, J. Juanhuix, A. Verdaguer, I. Imaz, D. Maspoch and J. Giner Planas, Angew. Chem., Int. Ed., 2016, 55, 16049–16053 CrossRef PubMed.
- L. Gan, M. T. Nord, J. M. Lessard, N. Q. Tufts, A. Chidambaram, M. E. Light, H. Huang, E. Solano, J. Fraile, F. Suárez-García, C. Viñas, F. Teixidor, K. C. Stylianou and J. G. Planas, J. Am. Chem. Soc., 2023, 145, 13730–13741 CrossRef CAS PubMed.
-
M. A. Fox, Icosahedral Carborane Derivatives, Durham thesis, Durham University, 1991 Search PubMed.
- M. Shang, S. Liang, N. Qu, H. Lian and J. Lin, Chem. Mater., 2017, 29, 1813–1829 CrossRef CAS.
- F. Moro, V. Corradini, M. Evangelisti, R. Biagi, V. De Renzi, U. del Pennino, J. C. Cezar, R. Inglis, C. J. Milios and E. K. Brechin, Nanoscale, 2010, 2, 2698–2703 CAS.
- Z. Li, A. Arauzo, J. G. Planas and E. Bartolomé, Dalton Trans., 2024, 53, 8969–8979 RSC.
-
E. Bartolomé and J. Giner Planas, 2024, manuscript in preparation.
-
S. Tripathi, XMCD investigation at M4,5 edges of the rare earth elements in high-performance permanent magnet, PhD thesis, Max-Planck-Institut für Intelligente Systeme, Stuttgart, 2018 Search PubMed.
- B. Thole, P. Carra, F. Sette and G. van der Laan, Phys. Rev. Lett., 1992, 68, 1943–1946 CrossRef CAS PubMed.
- R. R. Schenker, M. N. Leuenberger, G. Chaboussant, D. Loss and H. U. Güdel, Phys. Rev. B: Condens. Matter Mater. Phys., 2005, 72, 184403–184413 CrossRef.
- D. L. Huber, Phys. Rev. B: Solid State, 1965, 139, A1684.15.08.2013 Search PubMed.
- A. Arauzo, A. Lazarescu, S. Shova, E. Bartolomé, R. Cases, J. Luzón, J. Bartolomé and C. Turta, Dalton Trans., 2014, 43, 12342–12356 RSC.
- C. Wen, Y. Zhang, C. Wang, D. Xue, Y. Bai, S. Antonov, L. Dai and T. Lookman, Acta Mater., 2019, 170, 109–117 CrossRef CAS.
- G. Hart, T. Mueller, C. Toher and S. Curtarolo, Nat. Rev. Mater., 2021, 6, 730–755 Search PubMed.
|
This journal is © The Royal Society of Chemistry 2024 |
Click here to see how this site uses Cookies. View our privacy policy here.