A Ti3C2 MXene-integrated near-infrared-responsive multifunctional porous scaffold for infected bone defect repair†
Received
13th July 2023
, Accepted 28th September 2023
First published on 6th October 2023
Abstract
Infected bone defect repair has long been a major challenge in orthopedic surgery. Apart from bacterial contamination, excessive generation of reactive oxygen species (ROS), and lack of osteogenesis ability also threaten the defect repair process. However, few strategies have been proposed to address these issues simultaneously. Herein, we designed and fabricated a near-infrared (NIR)-responsive, hierarchically porous scaffold to address these limitations in a synergetic manner. In this design, polymethyl methacrylate (PMMA) and polyethyleneimine (PEI) were used to fabricate the porous PMMA/PEI scaffolds via the anti-solvent vapor-induced phase separation (VIPS) process. Then, Ti3C2 MXenes were anchored on the scaffolds through the dopamine-assisted co-deposition process to obtain the PMMA/PEI/polydopamine (PDA)/MXene scaffolds. Under NIR laser irradiation, the scaffolds were able to kill bacteria through the direct contact-killing and synergetic photothermal effect of Ti3C2 MXenes and PDA. Moreover, MXenes and PDA also endowed the scaffolds with excellent ROS-scavenging capacity and satisfying osteogenesis ability. Our experimental results also confirmed that the PMMA/PEI/PDA/MXene scaffolds significantly promoted new bone formation in an infected mandibular defect model. We believe that our study provides new insights into the treatment of infected bone defects.
1. Introduction
Bone defects are typically caused by trauma, infection, tumor, and developmental abnormalities, and remain major challenges in orthopedic surgery.1 To satisfy the needs of bone defect repair, numerous efforts have been made in recent decades to develop varying bone substitute biomaterials. Three-dimensional (3D) porous scaffolds are promising candidates for bone defect repair because they can simulate the extracellular matrix,2 and the interconnected pore structure can facilitate cellular activity (such as proliferation, adhesion, and migration), nutrient transportation, and cell–cell interaction.3,4 However, there are still some limitations associated with 3D porous scaffolds in clinical practice. For instance, infection often accompanies bone defects when they are caused by trauma or diseases.5 In addition, the process of scaffold implantation may also cause infection because the surfaces of biomaterials may provide adhesion strongholds for bacteria.6 When infection occurs, conventional treatment methods usually include antibiotic therapy and removal of necrotic bone and contaminated bone grafts.7 Such processes significantly prolong hospitalization periods, resulting in considerable suffering and burden for both individual patients and the healthcare system. Moreover, in response to injury and infection, the immune system initiates inflammation to protect the tissues from more severe damage.8 As signaling molecules and inflammatory mediators, reactive oxygen species (ROS) are crucial to the progression of inflammation.9 However, when the production of ROS exceeds the intracellular antioxidant defense capacity, the healing process of the damaged tissues will be irreversibly impeded.10–12 Considering these reasons, the development of 3D porous scaffolds with the spatiotemporal abilities of antibacterial, ROS scavenging, and osteogenesis to satisfy the needs of bone defect repair is imperative.
To meet the antibacterial needs, researchers usually functionalize bone substitute biomaterials with antibiotics, antimicrobial peptides,13 Ag, Cu, and their oxides.14 However, the overuse of antibiotics and antimicrobial peptides will potentially induce bacterial resistance, and Ag, Cu, and their oxides may cause biosafety problems.15 Considering these problems, bone substitute biomaterials that can fight infection in new ways are urgently needed.
Recently, photothermal therapy (PTT), based on near-infrared (NIR) light irradiation, has been considered a promising treatment in clinical medicine because of its nonresistance to bacteria, minimal invasiveness, remote controllability, and immediate therapeutic effects.16 In the presence of photothermal agents (PTAs), the light energy of NIR lasers can be transformed into heat energy, killing bacteria through hyperthermia-induced membrane rupture and thus resulting in irreversible bacterial destruction.17,18 However, when PTAs are administered systematically or injected locally, some side effects, such as systemic toxicity and instability, are likely to occur.19 Fortunately, 3D porous scaffolds can serve as platforms for PTAs to remain stable in the defect sites, and function preciously and repeatedly, thereby overcoming the associated limitations.19
MXenes are increasingly popular two-dimensional (2D) nanomaterials that possess negligible biotoxicity20 and excellent bioactivities. Because of their robust NIR light absorption capacity and high photothermal conversion efficiency (PCE), MXenes are recognized as promising PTAs and are used widely in the researches of PTT.21 In addition, the unique structure of MXenes endows them with high hydrophilicity, protein adsorption capacity, and good electrical conductivity,22,23 which promote cell-matrix and intercellular interactions, indicating that MXenes have great application potential in biomedicine. For instance, many previous studies have confirmed that MXenes can promote osteogenesis in mesenchymal stem cells.24 Besides, the role of MXenes in antibacterial,25–27 anti-tumor,28,29 and ROS scavenging30–32 has also been confirmed by previous studies.
Polydopamine (PDA) has been used extensively in the biomedical field given its outstanding biocompatibility, high hydrophilicity, and diverse modification potential.33,34 In addition, PDA also exhibits high PCE and ROS scavenging capacities.35,36 Therefore, it is a feasible approach to load MXenes onto 3D porous scaffolds with the help of PDA to simultaneously realize anti-infection, ROS scavenging, and osteogenesis at the defect sites.
Herein, we designed and fabricated an NIR-responsive multifunctional porous polymethyl methacrylate (PMMA)/polyethyleneimine (PEI)/PDA/MXene scaffold for bone defect repair. The hierarchical porous structure of the scaffold was derived from the micro-phase separation between PMMA and PEI during the anti-solvent vapor-induced phase separation (VIPS) process.37 VIPS is one of the most efficient techniques to fabricate porous scaffolds, because it can produce scaffolds with tunable porous structures with accessible equipment via simple processes.38 PMMA and PEI are suitable for the VIPS system, because of the interaction between them and the obvious difference in their water solubility. Moreover, as an FDA-approved material, PMMA has long been used in the clinical treatment of bone defects because of its excellent characteristics such as good mechanical strength and high biocompatibility.39 After preparation of the 3D porous PMMA/PEI scaffolds, MXenes were anchored onto the scaffolds via the PDA-assisted co-deposition process40 to obtain the PMMA/PEI/PDA/MXene scaffolds. The scaffolds we prepared through the above simple and controllable methods possess excellent PTT antibacterial, ROS scavenging, and osteogenic capacities, making them promising alternatives in the treatment of infected bone defects. We believe that our research has the potential to provide new ideas for the fabrication of multifunctional biomaterials and the treatment of infected bone defects.
2. Materials and experiments
2.1 Materials
Ti3AlC2 powder (200 mesh, 98%) was purchased from 11 Technology Co. Ltd (Changchun, China). Lithium fluoride (LiF, 98%), PEI (Mw = 10
000), ascorbic acid, cetylpyridinium chloride, and pentobarbital sodium were supplied by Aladdin Chemistry Co. Ltd (Shanghai, China). PMMA (Altuglas, HF1-7, PH03331) was obtained from SAL Petrochemical Co. Ltd (Suzhou, China). Dopamine hydrochloride (HCl-DA) powder and tris[hydroxymethyl]-aminomethane (TRIS) were procured from Adamas-Beta Co. Ltd (Shanghai, China). N,N-Dimethylformamide (DMF, 99%) and hydrochloride (HCl, 36%) were obtained from Paini (Zhengzhou, China). Ethanol (99.7%) was provided by Kelong Chemical Co. Ltd (Chengdu, China).
Phosphate buffer saline (PBS), 2.5% glutaraldehyde, 4% paraformaldehyde, Alizarin red S staining solution, and radioimmunoprecipitation assay (RIPA) buffer were acquired from Servicebio (Wuhan, China). Dulbecco's modified Eagle medium (DMEM) and fetal bovine serum (FBS) were provided by Gibco (NY, USA). Cell counting kit-8 (CCK-8), 50 IU mL−1 penicillin/0.05 mg mL−1 streptomycin bicinchoninic acid (BCA) assay kit and 4′,6-diamidino-2-phenylin-dole (DAPI) were sourced from Biosharp (Hefei, China). The live/dead staining kit and fluorescein 5-isothiocyanate (FITC)-phalloidin were obtained from Abbkine (USA). β-Glycerophosphate was supplied by Yuanye (Shanghai, China), and dexamethasone was purchased from Macklin (Shanghai, China). Lactate dehydrogenase (LDH) cytotoxicity assay kit, nitroblue tetrazolium chloride/5-bromo-4-chloro-3-indolyl phosphate toluidine salt (NBT/BCIP) staining kit, alkaline phosphatase (ALP) assay kit, and ROS assay kit, were sourced from Beyotime (Shanghai, China). 2,2-Diphenyl-1-picrylhydrazyl (DPPH) and 2,2-azino-bis-3-ethylbenzothiazoline-6-sulfonic acid (ABTS) free radical scavenging capacity assay kits were procured from Solarbio (Beijing, China). The RNA-Quick Purification Kit was sourced from YiShan Biotech (Shanghai, China). The PrimeScript RT reagent Kit and TB Green Premix Ex Taq II (Tli RNaseH Plus) kit were provided by TaKaRa (Japan).
2.2 Synthesis of Ti3C2 MXenes
First, Ti3AlC2 (1 g) was added to an etching solution comprising LiF (1 g) and 9 M HCl (20 mL), followed by etching at 35 °C for 24 h. Subsequently, the combination was washed with deionized water and subjected to repeated centrifugation until the pH value of the supernatant exceeded 6. Following that, the residue was redispersed into deionized water and underwent ice-bath coupled sonication treatment for 60 min. Afterward, the dark-green supernatants were collected after centrifugation. Finally, Ti3C2 MXenes were obtained after freeze-drying and then stored at 4 °C for further applications.
2.3 Preparation of PMMA/PEI (PME) scaffolds
3D porous PME scaffolds were fabricated via the VIPS method. Briefly, a blend of PMMA/PEI (wt/wt, 7/1) was thoroughly dissolved in DMF solvent at a concentration of 160 g L−1. Subsequently, the solution was decanted into a cylindrical Teflon mold (Φ 15 mm, 10 mm), followed by transferring the setup into a chamber with constant temperature and humidity conditions (30 °C, 80% relative humidity [RH]) and kept there for 12 h. Next, the setup was submerged in a cold-water bath for another 12 h to obtain the PME scaffolds.
2.4 Preparation of PME/PDA/MXene and PME/PDA scaffolds
The PME/PDA/MXene scaffolds were prepared through the one-pot dopamine-assisted co-deposition strategy. In brief, 100 mg of HCl-DA powder and 75 mg of Ti3C2 MXenes were added to 50 mL of 10 mM TRIS solution. Subsequently, the PME scaffolds were submerged in the solution and stirred for 24 h to obtain the PME/PDA/MXene scaffolds. The scaffolds were then rinsed several times to remove any unreacted materials.
The preparation process of the PME/PDA scaffolds was similar to the abovementioned procedure, except that no Ti3C2 MXenes were added to the mixture of HCl-DA and TRIS.
2.5 Material characterization
The structural attributes of Ti3C2 MXene nanosheets were identified using transmission electron microscopy (TEM, JEOL, Japan). The thickness of Ti3C2 MXene nanosheets was measured using atomic force microscopy (AFM, Bruker, USA). The crystalline organization of Ti3C2 MXenes was determined using X-ray diffraction (XRD, Rigaku, Japan) analysis. The surface structures of the PME, PME/PDA, and PME/PDA/MXene scaffolds were examined using field emission scanning electron microscopy (FE-SEM, ThermoFisher, MA, USA). The elemental distribution on the surfaces of the various scaffolds was studied using the energy-dispersive spectrometer (EDS) mounted on the SEM. The elemental composition on the surfaces of diverse scaffolds was determined using X-ray photoelectron spectroscopy (XPS, XSAM800, Kratos, UK). Fourier transform infrared (FTIR) spectra were captured using an FTIR spectrometer (ThermoFisher) in transmission mode within a wavenumber range of 400–4000 cm−1. The water contact angle on the surface was assessed using a drop shape analysis system (DSA25S, Germany).
2.6 Photothermal effect
The photothermal performance of the PME, PME/PDA, and PME/PDA/MXene scaffolds was characterized using an 808 nm NIR laser (Beijing Blueprint Photoelectric Technology Co. Ltd) and an infrared thermal imaging camera (Fluke, Ti450 Pro, WA, USA). Briefly, different scaffolds (Φ 15 mm, 1 mm) were fixed to the bottom of 24-well culture plates with 500 μL of PBS and irradiated using an NIR laser (808 nm, 0.65 W cm−2, 600 s). The thermal images were recorded every 30 s.
The photothermal performance of the PME/PDA/MXene scaffolds under different laser power densities (0.23, 0.43, and 0.65 W cm−2) was also investigated. Other experimental conditions were the same as before.
The pulsed irradiation test was carried out on the PME/PDA/MXene scaffolds with 300 s irradiation (laser-on) followed by a 300 s cooling period (laser-off). The on–off cycle was repeated five times. The thermal images were captured every 60 s.
2.7 Antibacterial analysis
2.7.1 Bacteria preparation and NIR laser treatment.
Staphylococcus aureus (S. aureus, ATCC 6538) and Escherichia coli (E. coli, ATCC 25922), as representative Gram-positive and Gram-negative bacteria, respectively, were used to evaluate the antibacterial efficacy of various scaffolds under different conditions with or without NIR laser irradiation. The bacteria were cultured in Luria-Bertani medium. The PME, PME/PDA, and PME/PDA/MXene scaffolds were co-incubated with 500 μL bacterial suspension (1 × 104 CFU per mL) at 37 °C for 24 h. Afterward, the samples were treated with or without NIR lasers (808 nm, 0.65 W cm−2, 600 s) and then subjected to subsequent experimental procedures.
2.7.2 Antibacterial efficiency.
To measure the antibacterial performance of various scaffolds under different conditions, the plate counting method was used. The bacteria incubated without any scaffold were marked as the control group. After co-incubation with bacteria and treatment with or without NIR laser irradiation, the samples were washed gently and immersed in 500 μL PBS before being sonicated for 1 min, which was intended to dislodge the bacteria adhering to the scaffolds. Subsequently, the bacterial suspension was subjected to a 10-fold dilution, and 10 μL of the resulting suspension was evenly distributed over the agar plates. Next, the plates were incubated at 37 °C for 24 h. Afterward, the agar plates were photographed and the colony-forming units (CFU) were counted. The antibacterial rate was calculated using the following equation:
Antibacterial ratio (%) = (N0 − Ne)/N0 × 100%, |
where Ne signifies the CFU in the experimental group, and N0 represents the CFU in the control group.
2.7.3 Bacterial morphology.
The morphology of the co-incubated bacteria in different groups was observed using FE-SEM (Inspect F50, FEI, OR, USA) after fixing (2.5% glutaraldehyde, 4 °C, overnight), air-drying, and gold-sputtering.
2.7.4 LDH cytotoxicity assay.
The extent of bacterial membrane damage was evaluated using an LDH cytotoxicity assay kit following the manufacturer's instructions. Briefly, the culture solution from various groups was mixed with the LDH working solution and incubated for 30 min before the optical density (OD) values at 490 nm were read on a microplate reader (MQX200, BioTEK, VT, USA).
2.8 Cytocompatibility studies
2.8.1 Cells isolation and culture.
All experiments involving animals were sanctioned by the Research Ethics Committee of West China School of Stomatology and carried out in compliance with global standards for animal wellbeing. The femurs of 3-week-old Sprague-Dawley rats (sourced from Ensiweier Bioscience, Chengdu, China) were utilized to extract rat bone marrow mesenchymal stem cells (rBMSCs). The obtained cells were cultivated in complete DMEM supplemented with 10% (v/v) FBS and 50 IU mL−1 penicillin/0.05 mg mL−1 streptomycin. The incubation condition was 37 °C, 5% CO2, and 95% RH. The rBMSCs were used between passages 2 and 4.
2.8.2 Cell morphology.
The morphology of rBMSCs incubated with different scaffolds was observed via FE-SEM (Inspect F50, FEI) and confocal laser scanning microscopy (CLSM, Nikon, AXR, Japan), separately. In short, rBMSCs were seeded in 24-well culture plates (5 × 104 cells per well) with PME, PME/PDA, or PME/PDA/MXene scaffolds. After 36 h, the scaffolds were observed using FE-SEM observation after fixing (2.5% glutaraldehyde, 4 °C, overnight), air-drying, and gold-sputtering. For CLSM observation, the cells were stained with DAPI (blue) and FITC-phalloidin (green) after being co-incubated with different scaffolds for 36 h, and then observed using CLSM.
2.8.3 Cell proliferation assay.
The proliferation of rBMSCs cultured with various scaffolds was assessed by CCK-8 assay and Ki-67 staining. The CCK-8 assay was performed using a CCK-8 kit in accordance with the manufacturer's instructions. Briefly, rBMSCs were cultivated with different scaffolds in 24-well plates at a density of 5 × 104 cells per well. After culturing for 1, 3, and 5 days, the medium was replaced with 10% CCK-8 solution and then incubated at 37 °C for another 30 min. After a 5 min agitation on a shaker, the medium was transferred into a 96-well plate, and the OD values at 450 nm were recorded using a microplate reader. For the Ki-67 staining, rBMSCs after co-incubation with different scaffolds for 48 h were fixed and permeabilized before staining with anti-Ki67 antibody (Abcam, ab16667). The results were assessed with flow cytometry, and Mean Fluorescence Intensity (MFI) were evaluated using the FlowJo software version 10.8 (Becton, Dickinson and Company, NJ, USA).
2.8.4 Cell viability assay.
The viability of rBMSCs cultured with various scaffolds was assessed using a live/dead staining kit in accordance with the instructions provided by the manufacturer. Briefly, rBMSCs were cultured with the PME, PME/PDA, or PME/PDA/MXene scaffolds in 12-well plates (8 × 104 cells per well). Cells incubated without any scaffold were used as control groups. On days 1, 3, and 5 post-incubation, the medium was substituted with the working solution (2 μM calcein-AM and 8 μM propidium iodide [PI]) and incubated for another 30 min. The fluorescent images of the cells were obtained using an inverted fluorescence microscope (DMi8, Leica, Germany).
2.9 Osteo-differentiation studies
2.9.1 Material extract preparation.
As the PME/PDA and PME/PDA/MXene scaffolds appear brown and black, respectively, scaffold extracts were used in the osteogenic staining experiments to avoid the interference of the colors of the scaffolds themselves. The scaffold extracts were prepared by soaking different sterilized scaffolds in DMEM at a solid/liquid ratio of 100 mg mL−1. Then, the mixture was incubated at 37 °C for 24 h. Next, the supernatant was passed through 0.22 μm micropore filters (Millex GP, Merck Millipore, MA, USA) for sterilization and preserved at 4 °C for later use.
2.9.2 Osteogenic induction culture of rBMSCs.
Initially, rBMSCs were cultivated in complete DMEM. After one day, the culture medium was substituted with osteogenic inducing medium composed of complete DMEM, enriched with 10% FBS, 50 IU mL−1 penicillin/0.05 mg mL−1 streptomycin, 5 mM β-glycerophosphate, 50 μg mL−1 ascorbic acid, and 100 nM dexamethasone. For the staining procedures, DMEM was replaced with equivalent volumes of scaffold extracts. The medium was replaced every 72 h.
2.9.3 ALP staining and quantitative ALP assay.
For ALP staining analysis, the rBMSCs were incubated for 7 and 14 days and then fixed with 4% paraformaldehyde for 15 min before being washed gently. Next, ALP staining was performed using an NBT/BCIP staining kit according to the manufacturer's instructions. Photographs were taken for further analysis.
For the quantitative ALP assay, RIPA buffer was used to lyse the rBMSCs after incubation for 7 and 14 days.
Subsequently, the ALP assay kit was used to determine the ALP levels. The generation of p-nitrophenol, which could reflect the content of ALP, was quantified based on OD405
nm. To normalize the ALP activity levels, the protein levels were measured using the BCA assay kit.
2.9.4 Alizarin red staining and semi-quantitative analysis.
For Alizarin red staining, rBMSCs were fixed with 4% paraformaldehyde for 15 min after 14 and 21 day incubation periods, separately. Following gentle rinses, the cells were stained with ARS solution. Images were subsequently captured for detailed analysis.
For semi-quantitative evaluation, 10% cetylpyridinium chloride was added to the stained wells for 20 min, and then the OD readings of the supernatant were recorded at a wavelength of 562 nm.
2.9.5 Quantitative real time polymerase chain reaction (RT-qPCR) analysis.
Subsequently, the mRNA expression levels of various osteogenesis-associated genes, such as ALP, type I collagen (COL1), bone morphogenetic protein 2 (BMP2), and runt-related transcription factor 2 (RUNX2) were investigated via RT-qPCR. Glyceraldehyde-3-phosphate dehydrogenase (GAPDH) was used as a reference gene for normalization. After a co-incubation period of 7 or 14 days with varying scaffolds, total RNA was extracted using the RNA-Quick Purification Kit. Thereafter, the total RNA was transcribed into cDNA with the assistance of a PrimeScript RT reagent Kit, processed on an ABI Veriti 96-Well Thermal Cycler (ThermoFisher). The subsequent real-time polymerase chain reactions were carried out using the TB Green Premix Ex Taq II kit, operated on a QuantStudio3 Real-Time PCR System (ThermoFisher). Expression levels of the genes were determined with the 2-ΔΔCT method. Table 1 presents the primers for the specific genes.
Table 1 Primers (5′-3′) used for RT-qPCR
Genes |
Forward primer sequence |
Reverse primer sequence |
ALP
|
ATGTCTGGAACCGCACTGAACTG |
CGTGGTCAATCCTGCCTCCTTC |
COL1
|
GACAGGCGAACAAGGTGACAGAG |
GAGAGCCAGGAGAGCCAGGAG |
BMP2
|
AAGCGTCAAGCCAAACACAAACAG |
GGCATGGTTGGTGGAGTTCAGG |
RUNX2
|
TGCCCAGGCGTATTTCAG |
TGCCTGGCTCTTCTTACTGAG |
GAPDH
|
GGTGTGAACCATGAGAAGTATGA |
GGTGTGAACCATGAGAAGTATGA |
2.10 ROS scavenging studies
2.10.1 Free radical scavenging assay.
The DPPH and ABTS free radical quenching capacity assay kits were employed to individually assess the DPPH and ABTS radical scavenging capacity of the various scaffolds. The working solution was prepared according to the operating instructions. For the DPPH scavenging assay, the working solution was incubated with PME, PME/PDA, and PME/PDA/MXene scaffolds, respectively, in the dark for 30 min before OD515
nm was measured. The ABTS assay process was similar to that of the DPPH, except that the incubation time was 6 min, after which OD405
nm was measured.
2.10.2 Cellular ROS scavenging activity.
The reactive oxygen species assay kit (employing the DCFH-DA probe) was used to measure the cellular ROS neutralizing effect of various scaffolds. The rBMSCs were incubated alongside the PME, PME/PDA, and PME/PDA/MXene scaffolds in 24-well plates, with a cell density of 8 × 104 per well, over a 24 h period. Subsequently, the media was disposed of and the cells were rinsed thrice with DMEM. Following this, each well was treated with 50 nM of H2O2. After 30 min of incubation at 37 °C, 1 mM of DCFH-DA probe was added. After incubation for another 20 min, the cells were washed thrice. The ROS level was measured with an inverted fluorescence microscope (DMi8, Leica, Germany) and flow cytometer (ThermoFisher).
2.11
In vivo studies
2.11.1 Generation of infected mandibular defects and implantation surgery procedure.
Thirty-six male Sprague-Dawley rats (age: 12 weeks, weight: 400–450 g) purchased from Chengdu Dashuo Biological Institute were randomly assigned into three groups: Control, PME/PDA/MXene (−), and PME/PDA/MXene (+). Critical sized bone defects were created in the rat mandibles.
In brief, the animals were first anesthetized with intraperitoneal injections of pentobarbital sodium, dosed at 3.5 mg per 100 g of body weight. Following this, a surgical cut was carefully made in the skin. This was succeeded by dissecting the muscles layer-by-layer and the periosteum. The branches of facial nerves were protected from injury. A full-thickness 4 mm round bone defect was made on the mandible. For the control group, 10 μL of 1 × 108 CFU of S. aureus was directly injected into the defect areas. For the two experimental groups, the PME/PDA/MXene scaffolds were placed at the defect sites before the bacterial suspension was injected into the scaffolds. Next, the incision was sutured closed and the rats were housed in their cages without any antibiotic treatment. For the PME/PDA/MXene (+) group, the implanted sites were irradiated with an NIR laser (808 nm, 1.0 W cm−2, 600 s) on days 1, 2, and 3 after implantation.
After 7 days of surgery, four rats in each group were euthanized with an overdose of pentobarbital sodium injection. The mandibles and muscles surrounding the defects were harvested to evaluate the degree of infection and inflammation by histological analysis. The remaining eight rats in each group were sacrificed after 4 and 8 weeks of implantation, and the mandibles were also collected to evaluate inflammation (by histological analysis) and osteogenesis condition (by histological analysis and micro-CT evaluation). In addition, the main organs including the heart, liver, lung, kidney, and spleen were excised and collected from the experimental rats after 8 weeks of implantation for histological analysis. The harvested tissues were fixed in 4% polyformaldehyde before being subjected to micro-CT scanning and histological analysis.
2.11.2 Micro-CT analysis.
The micro-CT images of the mandibles harvested at the 4th and 8th week post-surgery were obtained using a micro-CT scanner (45 μCT; SCANCO Medical, Switzerland) at a resolution of 14.6 μm. Subsequently, the SCANCO Visualization software was used to reconstruct the 3D models of the mandibles as well as the defect sites, and the SCANCO Evaluation software was used to calculate the bone volume/total volume ratio (BV/TV).
2.11.3 Histological evaluation.
The harvested soft tissues (including organs and muscles) were subjected to dehydration using gradient ethanol solutions, embedded and fixed in 4% polyformaldehyde, and sectioned. Next, all the tissues were stained with hematoxylin and eosin (H&E). For muscle tissue harvested 7 days after the implantation, Gram staining was also conducted.
For the bone tissue, decalcification, paraffin embedding, and sectioning were carried out before H&E staining was performed. For the bone tissue harvested 4 and 8 weeks after surgery, Masson's trichrome staining was also performed.
In addition, bone samples collected 8 weeks post-surgery were subjected to immunohistochemistry (IHC) staining. After decalcification, embedding, and sectioning, the bone sample slices were stained with antibodies against ALP (Abcam, ab254113, 1
:
300) and osteocalcin (OCN) (Bio-Techne, MAB1419, 1
:
300).
Every Histopathological section was examined using a digital panoramic scanner (WS-10, Wisleap, China). ImageJ software was utilized for the semi-quantitative assessment of IHC staining results.
2.12 Statistical analysis
All biological tests were conducted at least three times, with the outcomes reported as mean ± standard deviation. Data analysis was performed with GraphPad Prism 9.0 software (GraphPad Software, CA, USA). When comparing two sets of data, statistical significance was determined using a one-tailed Student's t-test, while a one-way analysis of variance (ANOVA) with a Bonferroni's post-hoc correction was applied for comparing the averages of more than two groups. P < 0.05 was deemed significant, and P < 0.01 or < 0.001 was considered to indicate very high statistical significance.
3. Results and discussion
3.1 Fabrication and characterization of NIR-responsive 3D porous PME/PDA/MXene scaffolds
The schematic fabrication process of the 3D porous PME/PDA/MXene scaffolds was illustrated in Scheme 1. The TEM image (Fig. 1a) shows that after etching and ultrasonication, the nanosheets obtained had a typical 2D flake-like structure. Moreover, the AFM results (Fig. 1b) show that the nano-flake had a thickness of approximately 2 nm, illustrating the 2D single-layered nanostructures of the Ti3C2 MXenes we prepared. As shown in Fig. 1c, the XRD results further confirm the successful preparation of Ti3C2 nanosheets. The (002) characteristic peak of Ti3C2 appeared at 2θ = 5.7°, while the characteristic peak at 2θ ≈ 40° of Al from Ti3AlC2 was not evident, which is consistent with previous reports.41 The SEM and EDS images (Fig. 1d) show the surface morphologies of the PME, PME/PDA, and PME/PDA/MXene scaffolds. All the scaffolds exhibited a typical hierarchically porous structure, which consisted of macropores (approximately 100–300 μm) and micropores (approximately 2–5 μm). On the surface of the PME/PDA scaffolds, a uniformly rough surface formed by PDA nanoparticles could be observed, and the Ti3C2 MXene nanosheets were tightly attached to the surface of PDA nanoparticles. As shown in the EDS results (Fig. 1d), the Ti and F elements only exist in the PME/PDA/MXene scaffolds. As Ti and F elements are the characteristic elements of Ti3C2 MXenes, it can be deduced that Ti3C2 nanosheets were successfully co-deposited on the PME/PDA/MXene scaffolds. The XPS and FTIR curves further confirm these results. The complete XPS spectra (Fig. 1e) reveal that the main elements in the PME and PME/PDA scaffolds were C, O, and N. In comparison, the PME/PDA/MXene scaffolds display distinct peaks of Ti and F, originating from the surface functional groups of Ti3C2 MXenes. The FTIR spectra (Fig. 1f) show characteristic peaks at 3442 cm−1, 2921 cm−1, 1727 cm−1, and 1118 cm−1, which correspond respectively to the N–H stretching vibration and C–H stretching vibration of PEI,42 and the C
O stretching vibration and C–O stretching vibration of PMMA.43 After being coated with PDA, the characteristic peaks at 2360 cm−1, 1636 cm−1, and 663 cm−1, linked to the C
C vibration, skeleton vibration, and C–H out-plane blend vibration of the benzene ring, could be observed.65 In the PME/PDA/MXene group, the unique peak at 546 cm−1, attributed to the surface terminal group of –OH in Ti3C2 MXenes is also noted.44
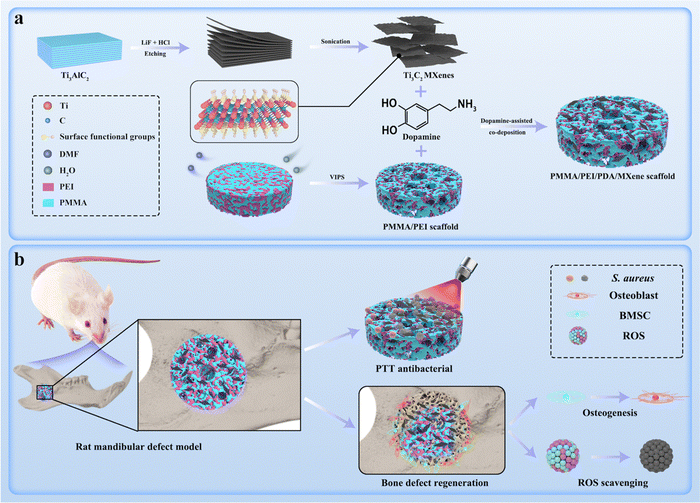 |
| Scheme 1 Schematic diagram of the PMMA/PEI/PDA/MXene scaffold preparation process (a) and its PTT antibacterial, ROS scavenging, and osteogenic abilities (b). | |
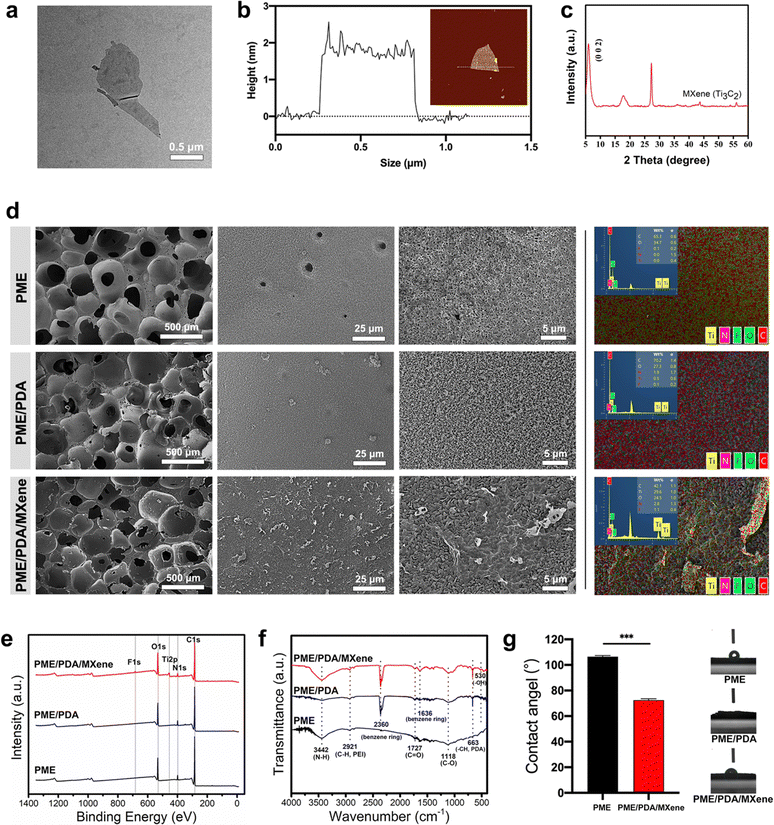 |
| Fig. 1 Characterization of Ti3C2 MXene nanosheets and the PME, PME/PDA, and PME/PDA/MXene scaffolds. (a) TEM image of Ti3C2 MXene nanosheets. (b) AFM image and the thickness curve of Ti3C2 MXene nanosheets. (c) XRD pattern of Ti3C2 MXenes. (d) SEM and EDS images of the PME, PME/PDA and PME/PDA/MXene scaffolds. (e) XPS spectra of various scaffolds. (f) FTIR spectra of different scaffolds (400–4000 cm−1 wavenumber). (g) Water contact angles of different scaffolds. *** represents P < 0.001. | |
Ti3C2 MXenes were anchored on the PME scaffolds through the dopamine-assisted co-deposition strategy by immersing the PME scaffolds into the mixing solution of TRIS-dopamine and Ti3C2 MXenes. In the alkaline environment, dopamine can self-polymerize to form PDA, which can firmly adhere to the PME scaffolds.45 Moreover, PEI can accelerate the deposition rate, because PDA can react with PEI via the Michael addition reaction.46 In addition, PEI can inhibit the self-polymerization process of PDA, resulting in uniform distribution of PDA nanoparticles on the scaffold surfaces.47 In addition, the catechol groups of PDA can combine with Ti–O bonds of Ti3C2 MXenes through chelation.48 Therefore, it is feasible to load Ti3C2 MXenes onto the PME scaffolds via the dopamine-assisted co-deposition strategy.
The water contact angle test (Fig. 1g) was conducted to assess the hydrophilicity of the different scaffolds. Before surface modification, the PME scaffolds had a large water contact angle of 106.5°, indicating its hydrophobicity and biological inertness.49,50 After treatment with PDA, the PME/PDA scaffolds became highly hydrophilic (water contact angle = 0°). After loading with Ti3C2 MXenes, the contact angle increased to 72°, as the hydrophilicity of MXenes was not so strong as that of PDA, but the PME/PDA/MXenes scaffolds were still hydrophilic, which facilitated cell adhesion and diffusion.51
3.2
In vitro photothermal performance
Next, we explored the photothermal performance of the various scaffolds. Fig. 2a and b show that both the PME/PDA/MXene and the PME/PDA groups exhibit a good photothermal effect. After irradiation with NIR lasers (808 nm, 0.65 W cm−2, 10 min), the temperatures of the PME/PDA/MXene and PME/PDA groups increased from 21.5 °C to 54.0 °C and 43.2 °C, respectively, indicating that the PME/PDA/MXene scaffolds had a better photothermal performance than the PME group, which was derived from the synergetic photothermal effect of Ti3C2 MXenes and PDA. By contrast, no significant temperature change was observed in the PME group under the same condition, indicating a negligible photothermal effect.
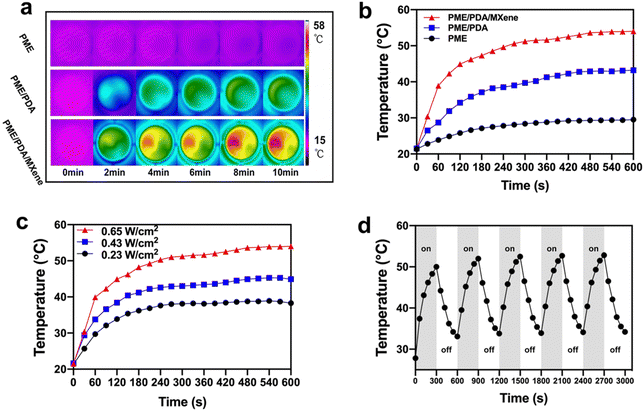 |
| Fig. 2 Photothermal performance of the PME, PME/PDA, and PME/PDA/MXene scaffolds. Infrared thermal images (a) and the corresponding heating curves (b) of the different scaffolds irradiated with NIR lasers (808 nm, 0.65 W cm−2, 600 s) in PBS solution. (c) Photothermal heating curves of the PME/PDA/MXene scaffolds under different power densities of NIR laser irradiation (0.65, 0.43, and 0.23 W cm−2) for 600 s in PBS solution. (d) Cyclic heating on/off profiles of the PME/PDA/MXene scaffolds for five cycles (laser-on: 300 s; laser-off: 300 s). | |
In addition, when irradiated with 808 nm lasers of different power densities (0.23, 0.43, and 0.65 W cm−2), the temperature changes in the PME/PDA/MXene group showed a power density-dependent performance (Fig. 2c). With the power density increasing from 0.23 to 0.65 W cm−2, the temperature of the PME/PDA/MXene group increased to 38.3 °C, 44.9 °C, and 54.0 °C within 10 min, respectively.
Furthermore, Fig. 2d depicts the photothermal stability of the PME/PDA/MXene scaffolds. The scaffolds were irradiated with 808 nm lasers for 300 s (laser-on), and then naturally cooled down for another 300 s (laser-off). After five cycles, the temperature increase shows no obvious attenuation, manifesting the robust and stable photothermal property of the PME/PDA/MXene scaffolds, which ensures a reliable PTT antibacterial performance once the bone defects are threatened by infection. The PME/PDA/MXene scaffolds with strong and tunable photothermal ability could provide adaptable heat energy to cope with bacterial infection effectively.
3.3
In vitro antibacterial ability
The antibacterial efficiency of the PME, PME/PDA, and PME/PDA/MXene scaffolds with or without NIR light irradiation was investigated via the plate counting method (Fig. 3a). The quantitative results of CFU counting and antibacterial ratio were also evaluated (Fig. 3b–d). In the absence of NIR light irradiation, the PME group exhibited negligible antibacterial efficiency against both S. aureus and E. coli. Such an inconspicuous antibacterial effect may come from PEI;52 however, the amount of residual PEI in the scaffolds was too small to generate a significant antibacterial effect. Similarly, after irradiation with NIR lasers, the PME group still showed a weak antibacterial ability because the PME scaffolds did not possess a photothermal conversion effect. However, both the PME/PDA and PME/PDA/MXene groups showed good antibacterial ability even without NIR laser irradiation. As previously reported, both PDA and Ti3C2 MXenes could destroy the structure of the bacterial cell membrane through direct contact.53,54 With NIR light irradiation, the PME/PDA and PME/PDA/MXene groups exhibited higher antibacterial efficiency against S. aureus (69.74% and 97.20%, respectively) and E. coli (72.58% and 96.25%, respectively) owing to their photothermal conversion ability, and the presence of Ti3C2 MXenes significantly enhanced the photothermal antimicrobial effect of the scaffolds. The so-called nanothermal blade effect of Ti3C2 MXenes made it possible for the PME/PDA/MXene scaffolds to effectively cope with bacterial infection.26
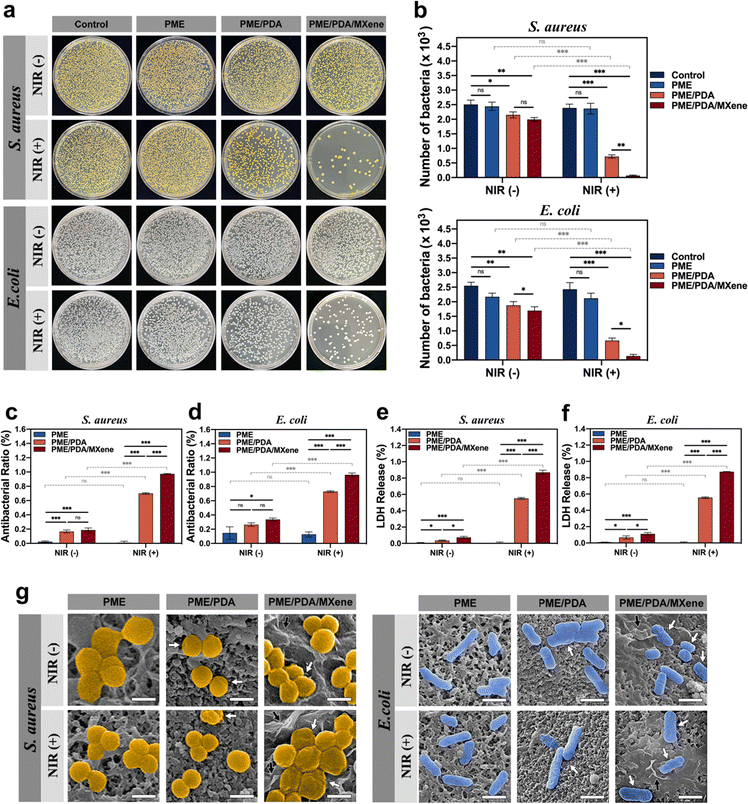 |
| Fig. 3 Antibacterial effects of the PME/PDA/MXene, PME/PDA, and PME scaffolds under various conditions. (a) Images of bacterial colony formation on different samples with or without the application of NIR lasers (808 nm, 0.65 W cm−2, 600 s). (b) Corresponding bacterial colony counts. The antibacterial proportion of different structures with or without NIR light treatment (808 nm, 0.65 W cm−2, 600 s) against (c) S. aureus and (d) E. coli. LDH release analysis of (e) S. aureus and (f) E. coli when co-cultured with various scaffolds with or without NIR light treatment. (g) SEM results of S. aureus and E. coli on different scaffolds with or without NIR light treatment (white arrows point to bacterial membrane damage; black arrows indicate Ti3C2 MXenes. Scale bar = 400 nm.) * denotes P < 0.05, ** denotes P < 0.01, and *** denotes P < 0.001. | |
Similar results could be found in the SEM images (Fig. 3g). Both S. aureus and E. coli. on the surfaces of the PME groups presented normal spherical or rod shapes with no sign of membrane folding irrespective of NIR light irradiation. By contrast, a small number of bacteria on the surfaces of the PME/PDA and PME/PDA/MXene groups showed slight shrinkage without NIR light irradiation, which is attributed to the direct contact-killing antibacterial effect of PDA and Ti3C2 MXenes. After irradiation with NIR lasers, the bacteria on the surfaces of the PME/PDA and PME/PDA/MXene groups showed severe deformation, and the morphology change in the PME/PDA/MXene group was worse.
The LDH assay, which is widely used for monitoring the integrity of cell membranes,55,56 was carried out to further confirm the bacterial cell membrane damage in the different groups. When the cell membrane of bacteria is disrupted, LDH, which normally exists in the cytoplasm when the membrane is intact, will be released into the culture medium. Therefore, the LDH release rate is proportional to the degree of cell membrane damage and can be used to evaluate the extent of damage. As shown in Fig. 3e and f, the bacteria of the PME/PDA/MXene group under NIR laser irradiation experienced the most severe membrane destruction, followed by the PME/PDA scaffolds treated with NIR light. The PME/PDA/MXene and PME/PDA groups in the absence of NIR laser irradiation also exhibited slight damage. In contrast, nearly no LDH could be detected in the PME group irrespective of NIR laser irradiation. These findings are consistent with previous antibacterial experiment results.
Taken together, these results confirmed that the incorporation of Ti3C2 MXenes and PDA into our porous scaffolds could effectively kill both Gram-positive and Gram-negative bacteria by generating high local temperature through the synergetic photothermal effect of Ti3C2 MXenes and PDA. Our experiments also suggest that bacterial deaths were mainly due to high local temperature-induced bacterial envelope destruction, as well as the contact-active antibacterial effect of Ti3C2 MXenes and PDA. Such a nanothermal blade effect could kill bacteria efficiently, without inducing bacterial resistance or generating cytotoxicity.
3.4 Cytocompatibility assays
The cytocompatibility of the PME, PME/PDA, and PME/PDA/MXene scaffolds was evaluated using rBMSCs. The SEM images (Fig. 4a) show that rBMSCs attached to different scaffolds all exhibited flattened morphology. However, for cells on the PME/PDA and PME/PDA/MXene scaffolds, the cytoplasm extended further, and the number of lamellipodia was larger than in the PME scaffold. The CLSM images (Fig. 4a) also show consistent results. FITC-phalloidin and DAPI were used to stain cell cytoskeletons (green) and nuclei (blue), respectively. Compared with cells on the PME scaffolds, cells on the PME/PDA and PME/PDA/MXene scaffolds stretched further with more filopodia. Taken together, these results indicate that the surface modification of PDA and Ti3C2 MXenes facilitated rBMSCs adhesion.
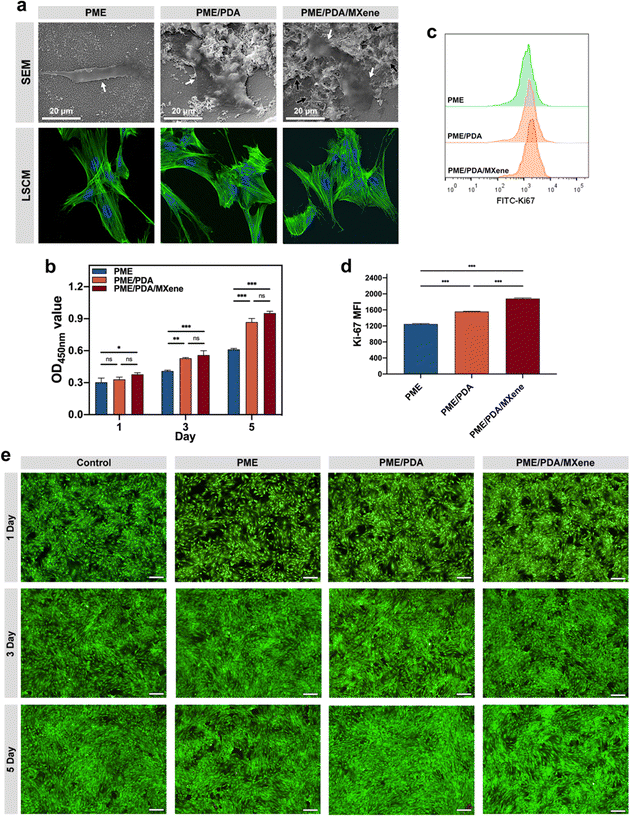 |
| Fig. 4
In vitro cytocompatibility assays. (a) SEM and CLSM images of rBMSCs cultured with the PME, PME/PDA, and PME/PDA/MXene scaffolds for 36 h. In SEM images, the white arrows indicate rBMSCs, and the black arrows denote Ti3C2 MXene nanosheets. (b) CCK-8 analysis of rBMSCs on different scaffolds at days 1, 3, and 5. (c) Flow cytometry results of Ki-67. (d) Statistical results of MFI for Ki-67. (e) Live/dead staining results of rBMSCs in different groups at days 1, 3, and 5 seen through a fluorescence microscope (scale bar = 250 μm). | |
Then, the proliferation of rBMSCs on various scaffolds was assessed by the CCK-8 assay (Fig. 4b) and Ki-67 staining (Fig. 4c and d). It is easy to notice from the CCK-8 results that cell proliferation in the different groups all exhibit time-dependent growth trends. The PME/PDA/MXene group showed the highest cell proliferation rate, while the PME group had the lowest, and the gap between them became more obvious over time. However, there was no significant difference between the PME/PDA and the PME/PDA/MXene groups, possibly due to an initial oversaturation of cells. Therefore, the Ki-67 staining experiment was further performed to verify the role of Ti3C2 MXenes in rBMSCs proliferation, as the expression level of Ki-67 can directly reflect the cell proliferation ability. The results show that rBMSCs cultured with the PME/PDA and the PME/PDA/MXene scaffolds displayed a significantly higher proliferation rate compared with that of the PME group. Moreover, compared with the PME/PDA group, the PME/PDA/MXene group showed a significantly stronger proliferation ability. These results confirm that both PDA and Ti3C2 MXenes could facilitate cell proliferation.
The above experimental outcomes revealed that the existence of PDA and Ti3C2 MXenes facilitated rBMSCs adhesion, growth, and proliferation. As previously mentioned, both PDA and Ti3C2 MXenes possess good hydrophilicity and biocompatibility. Additionally, both PDA nanoparticles and Ti3C2 MXenes could improve the surface roughness of the porous scaffolds on the nanoscale, facilitating the diffusion of water, oxygen molecules, and other bioactive substances on the surfaces of the scaffolds, which are essential to cellular growth and proliferation.57
Furthermore, the live/dead cells staining results (Fig. 4e and Fig. S1, ESI†) show consistent results. In this experiment process, live and dead cells were stained by PI as green and calcein-AM as red, respectively. It could be found that the percentage of red-stained dead cells was consistently low in all groups, even with a prolonged culture period. The results confirm the cytocompatibility of the PME, PME/PDA, and PME/PDA/MXene scaffolds.
3.5
In vitro osteogenic potential
Next, we explored the influences of different scaffolds on the osteogenic differentiation of rBMSCs. The ALP expression level was first investigated as it is an essential marker of osteogenesis at the early stage of differentiation. Fig. 5a shows that after 7 days of osteogenic induction, all groups exhibited purple staining, with the darkest shades in the PME/PDA/MXene group and the lightest in the PME group. As the staining shades are proportional to ALP content, we could infer that the PME/PDA/MXene scaffolds had the strongest osteogenic ability, while the PME scaffolds had the weakest. The same tendency was observed in the staining results after 14 days of incubation. The quantitative analysis of ALP content (Fig. 5b) further confirms these conclusions.
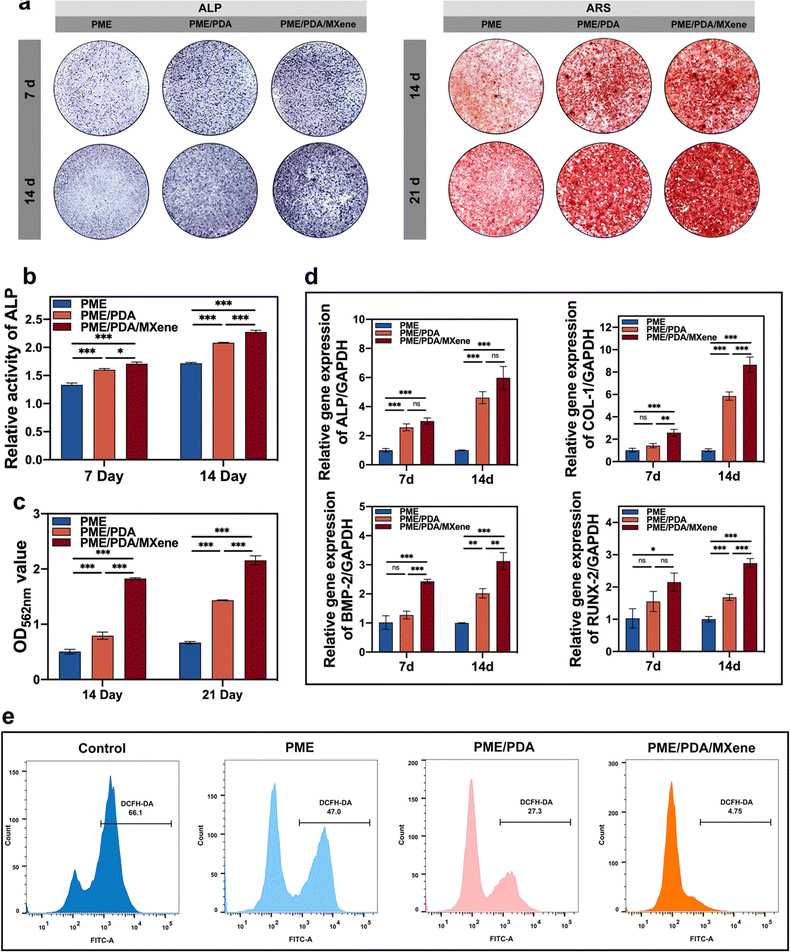 |
| Fig. 5
In vitro osteogenesis and ROS scavenging capacities. (a) ALP and ARS staining images of rBMSCs co-cultured with different scaffolds. (b) ALP expression levels of rBMSCs in different groups after culturing for 7 and 14 days. (c) Semi-quantitative assay of calcium nodules of different groups after 14 and 21 days of culturing. (d) Osteogenic gene expressions of rBMSCs after osteogenic induction for 7 and 14 days. (e) FCM analysis (FITC-A) of ROS scavenging of rBMSCs incubated with different scaffolds. * represents P < 0.05, ** represents P < 0.01, and *** represents P < 0.001. | |
Calcium deposition is an indication of osteogenesis at the late stage of differentiation. To visualize cellular mineralization, an ARS staining solution was applied, because it could chelate with the deposited calcium specifically, and exhibit a red color. Similar to the results of ALP expression, after incubation for 14 and 21 days, the largest and most intensive red calcium nodules appeared in the PME/PDA/MXene group (Fig. 5a), indicating its strongest mineralization capacity compared with the other groups. In contrast, the PME group had the lightest red staining, indicating the weakest mineralization ability. Semi-quantification detection (Fig. 5c) also supports these findings.
Next, we evaluated the osteogenesis-related gene expression levels of the different groups after osteogenic induction for 7 and 14 days. The results (Fig. 5d) show that the PME group had the lowest expression of ALP, COL1, BMP2 and RUNX2 after incubation for 7 and 14 days. After wrapping with PDA, the PME/PDA group had a higher expression level of the abovementioned genes. After loaded with Ti3C2 MXenes, gene expression level of the PME/PDA/MXene group was further improved. Compared with the PME scaffolds, the osteogenesis-related gene expression levels of the PME/PDA/MXene group were significantly higher after incubated for both 7 and 14 days, demonstrating that the PME/PDA/MXene group is superior to the others with respect to promoting osteogenic differentiation of rBMSCs.
Therefore, the above experimental results proved that the PME/PDA/MXene porous scaffolds possess the strongest osteogenic potential. As reported previously, 3D scaffolds with open and connected hierarchical porous structures could facilitate bone defect repair by promoting cellular activity and nutrient transport.3 In addition, PDA enhances cell proliferation and calcium deposition,58 thus facilitating the osteogenic process. Moreover, Ti3C2 MXenes were also reported to have superior osteogenic capacity.59 Therefore, the PME/PDA/MXene porous scaffolds we designed and fabricated possessed a strong osteogenic potential both theoretically and practically.
3.6
In vitro ROS scavenging ability
The DPPH and ABTS assays were performed to evaluate the radical scavenging ability of different scaffolds as they are the most commonly used representative free radicals in laboratory assays.60,61 As shown in Fig. S2 (ESI†), both PME/PDA and PME/PDA/MXene scaffolds possessed evident radical scavenging capacities compared with the PME group.
Next, the intracellular ROS-scavenging property was explored. After co-culturing with various scaffolds and stimulation by H2O2, the intracellular ROS level in rBMSCs was tested with the DCFH-DA probe. The results were subjected to fluorescence microscopy observation and flow cytometry (FCM) analysis, respectively. As shown in Fig. S2 (ESI†), the PME/PDA/MXene group exhibited the lowest fluorescence intensity, while the PME group showed the highest, constituting a sharp contrast. Similarly, the results of FCM (Fig. 5e) showed that the ROS positivity of the PME/PDA/MXene scaffolds was 4.75%, while that for the PME/PDA group was 27.3%, suggesting that both groups had a satisfying ROS scavenging capacity. However, the ROS positivity of the PME group was 47%, indicating a limited ROS scavenging ability.
PDA shows promising ROS scavenging capacity mainly because of the abundant phenolic groups on the surface.62 Therefore, PDA is frequently used in the treatment of inflammatory diseases such as osteoarthritis and periodontitis. For Ti3C2 MXenes, Ti species with an unsaturated valence state and their quasi-metallic property induced superior electron transfer ability, endowing Ti3C2 MXenes with efficient and broad-spectrum antioxidant capability.30
As mentioned previously, trauma and infection could lead to an increase in ROS generation.63 Furthermore, an important side effect of PTT is inflammation because the adjacent hyperthermia-induced necrotic cells release excessive ROS.64 As common triggers and important promoters, the excessive ROS could exacerbate and prolong the inflammation process, leading to a delayed healing process of the defect areas.65 According to the above-mentioned experiment results, the PME/PDA/MXene scaffolds could eliminate excessive ROS, thereby protecting cells from oxidative damage, which is beneficial for bone regeneration.
3.7
In vivo antibacterial capability and bone regeneration effect
Based on the aforementioned experimental results, we could tentatively confirm that among the three studied scaffolds, the PME/PDA/MXene scaffold possessed the best antibacterial, ROS scavenging, and osteogenic abilities in vitro. Therefore, the PME/PDA/MXene scaffolds were chosen for the following in vivo study on a rat model of infected mandibular defect to verify its PTT antibacterial and bone defect repair abilities. Fig. 6a illustrates the brief surgical implantation process and subsequent treatment and analysis procedures. For photothermal antibacterial treatment, NIR lasers (808 nm, 1.0 W cm−2, 600 s) were used in the PME/PDA/MXene (+) group on days 1, 2, and 3 after surgery. Fig. 6b and c exhibit the infrared thermal images and the corresponding heating curves of the PME/PDA/MXene (+) group upon NIR light irradiation.
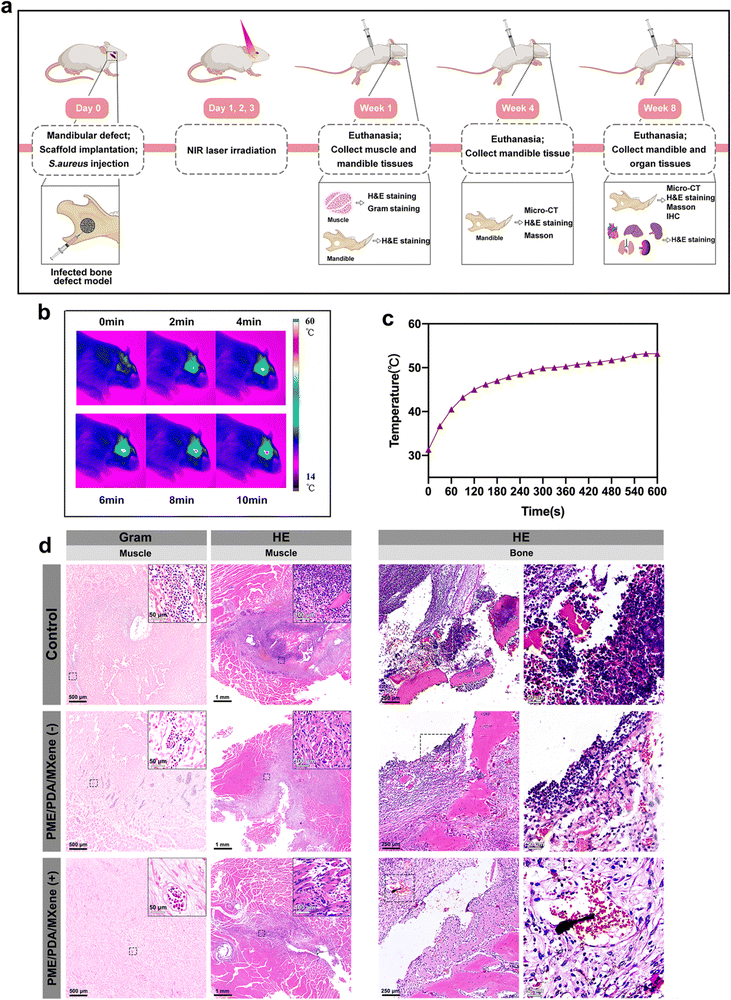 |
| Fig. 6
In vivo antibacterial ability 1 week after surgery. (a) Schematic of the surgical implantation process and subsequent treatment and analysis procedures for in vivo evaluation. Infrared thermal images (b) and the corresponding heating curves (c) of the PME/PDA/MXene (+) group upon NIR light irradiation (808 nm, 1.0 W cm−2) in vivo. (d) From left to right, Gram staining images reveal the bacterial distribution in the muscle tissue near the defects, H&E staining shows the degree of infection in the muscle tissue, and H&E staining indicating the degree of infection in the bone tissue. | |
One week after surgery, the infection and inflammation status were investigated. Gross observation results of the muscle tissue near the surgical sites are presented in Fig. S3 (ESI†). Not surprisingly, the control group had massive necrosis and pus. However, in the PME/PDA/MXene (−) group, the necrosis and pus were lessened, while no necrosis or pus could be observed in the PME/PDA/MXene (+) group.
After gross observation, the tissue was subjected to histological analysis. H&E and Gram staining were employed to evaluate the severity of infection and inflammation in the surrounding soft and hard tissues. As shown in the H&E staining images (Fig. 6d), signs of severe infection could be found in both the muscles and the bone tissue in the control group, with massive infiltration of basophilic inflammatory cells (including monocytes, neutrophils, and eosinophils) as well as eosinophilic structureless necrotic tissue formation. In the PME/PDA/MXene (−) group, such signs were mitigated, but still could not be ignored. However, the inflammatory state was negligible in the PME/PDA/MXene (+) group, and even osteoid formation could be observed. Similarly, the Gram staining results (Fig. 6d) of the muscle tissue show extensive bacterial infiltration in the control group, while in the PME/PDA/MXene (−) group, the bacterial count was decreased. In contrast, nearly no bacteria could be found in the PME/PDA/MXene (+) group. The above experimental results confirm the limited antibacterial and anti-inflammation ability of the PME/PDA/MXene scaffolds alone, and improved anti-infection capacity when they were subjected to NIR light irradiation.
It is well known that fever is a cardinal response to infection to protect the host from attacks of invading pathogens.66 However, as mentioned previously, the increase in local temperature can also do harm to the surrounding normal tissue, leading to the side effect of inflammation.64 Therefore, PTT may be a double-edged sword, and we ought to find ways to avoid its side effects. First, the temperature should be controlled at an appropriate level. According to previous studies relating to PTT, temperatures below 53 °C will cause only minor heat damage to the surrounding normal tissue.67,68 In our in vivo experiments, the highest temperature used for PTT was also approximately 53 °C (Fig. 6b and c). In addition, as mentioned in Section 3.6, the ROS scavenging capacity of the PME/PDA/MXene scaffold can mitigate the inflammation in the implanted sites. The in vivo experiment results further suggest that in the PME/PDA/MXene (+) group, which had the highest temperature, the signs of inflammation were the slightest, indicating that the pros of PTT below 53 °C outweigh the cons when it comes to infected bone defect repair. Apart from the side effect of inflammation, another factor that may limit the effectiveness of PTT is the penetration depth of NIR light. In the rat mandibular defect model we used, the PTT antibacterial effect of the PME/PDA/MXene scaffolds under NIR laser irradiation was satisfactory. However, if the infected defect site is more deeply located, the limited penetration depth of NIR light may not provide an effective PTT antibacterial effect. Under such conditions, light in the second NIR window (NIR-II, 1000–1350 nm), which possesses higher tissue penetrability due to less light scattering, might be a better choice.69
After evaluation of PTT antibacterial effects, bone regeneration at 4 and 8 weeks post-operation was evaluated. Micro-CT scanning and reconstruction results were used to evaluate the new bone formation of each group. From the representative 3D reconstruction images (Fig. 7a) of the whole mandibles and the defect sites, we noted that after 4 weeks, only limited new bone formed in the defect sites of the control group, suggesting that infection and inflammation had impeded the osteogenesis process. However, the PME/PDA/MXene (−) group had more newly formed bone than that of the control group, which was on account of the limited antibacterial and ROS scavenging ability of the PME/PDA/MXene scaffolds. However, in the PME/PDA/MXene (+) group, the most amount of new bone could be observed, indicating that the PTT antibacterial ability of the PME/PDA/MXene scaffolds under NIR light irradiation could facilitate bone regeneration. The trend of new bone growth after 8 weeks exhibited similar trends, but the total mass of new bone was obviously more. The quantitative results of BV/TV also supported this conclusion.
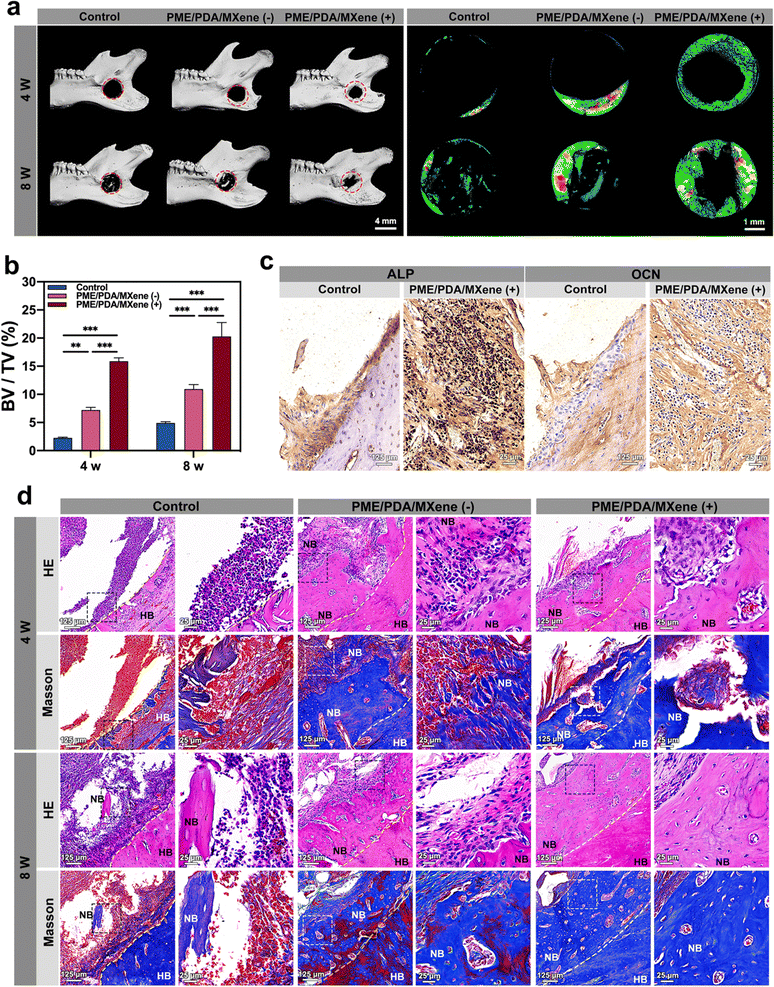 |
| Fig. 7
In vivo bone regeneration at postoperative 4 and 8 weeks. (a) Micro-CT 3D reconstruction images of the whole mandibles and the defect sites after 4 weeks and 8 weeks under different conditions. (b) Values of BV/TV of new bone tissue in the defect sites according to the micro-CT results. (c) Representative IHC staining images of ALP and OCN surrounding the defect area after implantation for 8 weeks. (Scale bar = 50 μm.) (d) H&E and Masson's trichrome staining images of different groups after 4 and 8 weeks (HB: host bone; NB: new bone; yellow dotted line: boundary between new bone and host bone). * represents P < 0.05, ** represents P < 0.01, and *** represents P < 0.001. | |
Images of H&E and Masson staining provided further confirmation of new bone formation and the infection and inflammation status of defect sites. As shown in Fig. 7d, the control group had the highest infiltration of inflammatory cells, with only limited newly formed bone. In the PME/PDA/MXene (−) group, the number of inflammatory cells decreased to some extent, and more new bone formation could be found. The PME/PDA/MXene (+) group had the most newly formed bone and the least infiltration of inflammatory cells, indicating that PTT could effectively kill bacteria, thereby promoting bone formation. The Masson staining results show consistent trends. Similarly, the IHC staining results of ALP and OCN in the PME/PDA/MXene (+) group exhibit a darker staining than that of the control group (Fig. 7c). The quantitative results of the average optical density (AOD) (Fig. S3b, ESI†) confirm significant differences between the two groups.
H&E staining results of the main organs (heart, liver, spleen, lung, and kidney) at the 8th week post-operation (Fig. S3c, ESI†) showed that no histological change could be found in any group, proving the biosafety of the PME/PDA/MXene scaffolds and NIR light treatment.
4. Conclusions
In this study, a Ti3C2 MXene-integrated, NIR-responsive, 3D hierarchically porous scaffold (PME/PDA/MXene) with excellent PTT antibacterial, ROS scavenging, and osteogenic abilities was successfully designed and fabricated. When irradiated with NIR lasers, the PME/PDA/MXene scaffolds showed satisfying PTT antibacterial effects by destroying the bacterial membrane. Moreover, the ROS scavenging ability further facilitated bone regeneration. The VIPS and dopamine-assisted co-deposition processes, which we used for scaffold manufacturing, are simple, controllable, cost-effective, and do not require high-end equipment. The photothermal antibacterial treatment is controllable, highly effective, and avoids inducing bacterial resistance. In contrast to prior studies that examined and utilized the antibacterial, ROS scavenging, or osteogenic properties of Ti3C2 MXenes independently, herein, a novel porous scaffold for bone defect regeneration that integrates these effects simultaneously was successfully developed. Our work not only offers new perspectives on the design of multifunctional biomaterials but also expand the potential applications of Ti3C2 MXenes in the field of biomedicine.
Author contributions
Linli Zhang: conceptualization, methodology, investigation, data curation, visualization, writing – original draft. Hui Zhang: conceptualization, methodology, investigation. Hongling Zhou: conceptualization, methodology, investigation. Yi Tan: investigation. Zhengmin Zhang: conceptualization, writing – review & editing. Wei Yang: conceptualization, methodology, writing – review & editing, funding acquisition, project administration. Lixing Zhao: conceptualization, methodology. Zhihe Zhao: conceptualization, methodology, funding acquisition, project administration.
Conflicts of interest
There are no conflicts to declare.
Acknowledgements
We acknowledge financial support from the National Natural Science Foundation of China (No. 32271416).
Notes and references
- M. Alonzo, F. Alvarez Primo, S. Anil Kumar, J. A. Mudloff, E. Dominguez, G. Fregoso, N. Ortiz, W. M. Weiss and B. Joddar, Curr. Opin. Biomed. Eng., 2021, 17, 100248 CrossRef CAS.
- Y. Jin, J. Zhou, X. Zhao, X. Zhang and Z. Su, J. Mater. Chem. B, 2022, 10, 9040–9053 RSC.
- H. Ma, J. Luo, Z. Sun, L. Xia, M. Shi, M. Liu, J. Chang and C. Wu, Biomaterials, 2016, 111, 138–148 CrossRef CAS.
- M. Lu, L. Sun, J. Yao, B. Zhao, Y. Liu, Z. Shao and X. Chen, J. Mater. Chem. B, 2022, 10, 6546–6556 RSC.
- W. Wang and K. W. K. Yeung, Bioact. Mater., 2017, 2, 224–247 Search PubMed.
- Z.-Y. Chen, S. Gao, Y.-W. Zhang, R.-B. Zhou and F. Zhou, J. Mater. Chem. B, 2021, 9, 2594–2612 RSC.
- D. P. Lew and F. A. Waldvogel, Lancet, 2004, 364, 369–379 CrossRef CAS PubMed.
- L. Chen, H. Deng, H. Cui, J. Fang, Z. Zuo, J. Deng, Y. Li, X. Wang and L. Zhao, Oncotarget, 2017, 9, 7204–7218 CrossRef.
- M. Mittal, M. R. Siddiqui, K. Tran, S. P. Reddy and A. B. Malik, Antioxid. Redox Signaling, 2014, 20, 1126–1167 CrossRef CAS.
- H. Kanzaki, S. Wada, T. Narimiya, Y. Yamaguchi, Y. Katsumata, K. Itohiya, S. Fukaya, Y. Miyamoto and Y. Nakamura, Front. Physiol., 2017, 8, 351 CrossRef.
- H. Wang, D. Wang, H. Huangfu, S. Chen, Q. Qin, S. Ren, Y. Zhang, L. Fu and Y. Zhou, Biomater. Sci., 2023, 11, 1335–1349 RSC.
- I. Ullah, Z. Hussain, S. Ullah, Q. ul ain Zahra, Y. Zhang, S. Mehmood, X. Liu, E. Kamya, M. W. Ghani, M. Mansoorianfar, Z. Wang, Z. Wang and R. Pei, J. Mater. Chem. B, 2023, 11, 5830–5845 RSC.
- N. Mookherjee, M. A. Anderson, H. P. Haagsman and D. J. Davidson, Nat. Rev. Drug Discovery, 2020, 19, 311–332 CrossRef CAS PubMed.
- Z. Wang, W. Hu, W. Wang, Y. Xiao, Y. Chen and X. Wang, Adv. Fiber Mater., 2023, 5, 107–129 CrossRef CAS.
- Y. Xu, S. Chen, Y. Zhang, C. Wu, L. Li, X. Hu, J. Zhang and Y. Wang, J. Mater. Chem. B, 2023, 11, 7069–7093 RSC.
- Z. Li, Y. Yang, J. Yao, Z. Shao and X. Chen, Mater. Sci. Eng., C, 2017, 79, 123–129 CrossRef CAS.
- Y. Yang, L. Ma, C. Cheng, Y. Deng, J. Huang, X. Fan, C. Nie, W. Zhao and C. Zhao, Adv. Funct. Mater., 2018, 28, 1705708 CrossRef.
- J. Li, X. Liu, L. Tan, Z. Cui, X. Yang, Y. Liang, Z. Li, S. Zhu, Y. Zheng, K. W. K. Yeung, X. Wang and S. Wu, Nat. Commun., 2019, 10, 4490 CrossRef PubMed.
- R. Sun, H. Chen, L. Sutrisno, N. Kawazoe and G. Chen, Sci. Technol. Adv. Mater., 2021, 22, 404–428 CrossRef CAS.
- S. M. George and B. Kandasubramanian, Ceram. Int., 2020, 46, 8522–8535 CrossRef CAS.
- J. Li, Z. Li, X. Liu, C. Li, Y. Zheng, K. W. K. Yeung, Z. Cui, Y. Liang, S. Zhu, W. Hu, Y. Qi, T. Zhang, X. Wang and S. Wu, Nat. Commun., 2021, 12, 1224 CrossRef CAS PubMed.
- X. Qu, Y. Guo, C. Xie, S. Li, Z. Liu and B. Lei, ACS Nano, 2023, 17, 7229–7240 CrossRef CAS.
- S. H. Lee, M. S. Kang, S. Jeon, H. J. Jo, S. W. Hong, B. Kim and D.-W. Han, Heliyon, 2023, 9, e14490 CrossRef CAS.
- J. Zhang, S. Tang, N. Ding, P. Ma and Z. Zhang, Nanoscale Adv., 2023, 5, 2921–2932 RSC.
- K. Rasool, K. A. Mahmoud, D. J. Johnson, M. Helal, G. R. Berdiyorov and Y. Gogotsi, Sci. Rep., 2017, 7, 1598 CrossRef.
- F. Seidi, A. Arabi Shamsabadi, M. Dadashi Firouzjaei, M. Elliott, M. R. Saeb, Y. Huang, C. Li, H. Xiao and B. Anasori, Small, 2023, 19, 2206716 CrossRef CAS.
- J. Chen, W. Fu, F.-L. Jiang, Y. Liu and P. Jiang, J. Mater. Chem. B, 2023, 11, 702–715 RSC.
- F. Duan, Q. Jia, G. Liang, M. Wang, L. Zhu, K. J. McHugh, L. Jing, M. Du and Z. Zhang, ACS Nano, 2023, 17, 11290–11308 CrossRef CAS PubMed.
- H. Zhu, X. Zhang, Q. Wang, J. Deng, Z. Zhang, X. Zhang, J. Cao and B. He, J. Mater. Chem. B, 2022, 10, 10083–10096 RSC.
- H. Geng, Y. Ren, G. Qin, T. Wen, Q. Liu, H. Xu and W. He, RSC Adv., 2022, 12, 11128–11138 RSC.
- J. Liu, W. Lu, X. Lu, L. Zhang, H. Dong and Y. Li, Nano Res., 2022, 15, 2558–2566 CrossRef.
- X. Zhao, L.-Y. Wang, J.-M. Li, L.-M. Peng, C.-Y. Tang, X.-J. Zha, K. Ke, M.-B. Yang, B.-H. Su and W. Yang, Adv. Sci., 2021, 8, e2101498 CrossRef.
- H. Wu, C. Zhao, K. Lin and X. Wang, Front. Bioeng. Biotechnol., 2022, 10, 952500 CrossRef PubMed.
- J. Zeng, C. Gu, X. Geng, K. Lin, Y. Xie and X. Chen, Biomaterials, 2023, 297, 122122 CrossRef CAS.
- J. Ma, J. Li, X. Wang, M. Li, W. Teng, Z. Tao, J. Xie, Y. Ma, Q. Shi, B. Li and Saijilafu, Adv. Healthcare Mater., 2023, 12, 2202377 CrossRef CAS.
- F. Xu, M. Li, Z. Que, M. Su, W. Yao, Y. Zhang, B. Luo, Y. Li, Z. Zhang and J. Tian, J. Mater. Chem. B, 2023, 11, 3453–3472 RSC.
- X. Tan and D. Rodrigue, Polymers, 2019, 11, 1160 CrossRef PubMed.
- R. Zeinali, L. J. del Valle, J. Torras and J. Puiggalí, Int. J. Mol. Sci., 2021, 22, 3504 CrossRef CAS.
- K. Yu, B. Liang, Y. Zheng, A. Exner, M. Kolios, T. Xu, D. Guo, X. Cai, Z. Wang, H. Ran, L. Chu and Z. Deng, Theranostics, 2019, 9, 4192–4207 CrossRef CAS.
- H. Li, B. Jiang and J. Li, Adv. Colloid Interface Sci., 2021, 295, 102489 CrossRef CAS.
- A. C. Khot, T. D. Dongale, J. H. Park, A. V. Kesavan and T. G. Kim, ACS Appl. Mater. Interfaces, 2021, 13, 5216–5227 CrossRef CAS PubMed.
- W. Bu, X. Xu, Z. Wang, N. Jin, L. Liu, J. Liu, S. Zhu, K. Zhang, R. Jelinek, D. Zhou, H. Sun and B. Yang, ACS Appl. Mater. Interfaces, 2020, 12, 50287–50302 CrossRef CAS.
- C. Li, J. Sun, K. Shi, J. Long, L. Li, Y. Lai and L. Qin, J. Mater. Chem. B, 2020, 8, 4575–4586 RSC.
- X. Zhao, X.-J. Zha, L.-S. Tang, J.-H. Pu, K. Ke, R.-Y. Bao, Z. Liu, M.-B. Yang and W. Yang, Nano Res., 2020, 13, 255–264 CrossRef CAS.
- X. Meng, J. Zhang, J. Chen, B. Nie, B. Yue, W. Zhang, Z. Lyu, T. Long and Y. Wang, J. Mater. Chem. B, 2020, 8, 10190–10204 RSC.
- W.-Z. Qiu, H.-C. Yang and Z.-K. Xu, Adv. Colloid Interface Sci., 2018, 256, 111–125 CrossRef CAS.
- Y. Zhang, S. Huang, B. Mei, X. Tian, L. Jia and W. Zhu, Sci. Total Environ., 2023, 876, 162841 CrossRef CAS.
- J. E. Heckler, G. R. Neher, F. Mehmood, D. B. Lioi, R. Pachter, R. Vaia, W. J. Kennedy and D. Nepal, Langmuir, 2021, 37, 5447–5456 CrossRef CAS PubMed.
- K.-Y. Law, J. Phys. Chem. Lett., 2014, 5, 686–688 CrossRef CAS PubMed.
- S. Sankar, B. N. Nair, T. Suzuki, G. M. Anilkumar, M. Padmanabhan, U. N. S. Hareesh and K. G. Warrier, Sci. Rep., 2016, 6, 22732 CrossRef CAS.
- L. Ponsonnet, K. Reybier, N. Jaffrezic, V. Comte, C. Lagneau, M. Lissac and C. Martelet, Mater. Sci. Eng., C, 2003, 23, 551–560 CrossRef.
- S. Maity, D. Gaur, B. Mishra, N. C. Dubey and B. P. Tripathi, J. Colloid Interface Sci., 2023, 642, 129–144 CrossRef CAS PubMed.
- K. Rasool, M. Helal, A. Ali, C. E. Ren, Y. Gogotsi and K. A. Mahmoud, ACS Nano, 2016, 10, 3674–3684 CrossRef CAS PubMed.
- Y. Fu, L. Yang, J. Zhang, J. Hu, G. Duan, X. Liu, Y. Li and Z. Gu, Mater. Horiz., 2021, 8, 1618–1633 RSC.
- L. Pan, J. Liu and J. Shi, ACS Appl. Mater. Interfaces, 2017, 9, 15952–15961 CrossRef CAS PubMed.
- R. Nie, Y. Sun, H. Lv, M. Lu, H. Huangfu, Y. Li, Y. Zhang, D. Wang, L. Wang and Y. Zhou, Nanoscale, 2022, 14, 8112–8129 RSC.
- X. Mi, Z. Su, Y. Fu, S. Li and A. Mo, Biomed. Mater., 2022, 17, 035002 CrossRef PubMed.
- S. Huang, N. Liang, Y. Hu, X. Zhou and N. Abidi, BioMed Res. Int., 2016, 2016, 2389895 Search PubMed.
- J. Zhang, Y. Fu and A. Mo, Int. J. Nanomed., 2019, 14, 10091–10103 CrossRef CAS.
- M. Gangwar, M. K. Gautam, A. K. Sharma, Y. B. Tripathi, R. K. Goel and G. Nath, Sci. World J., 2014, 2014, e279451 Search PubMed.
- Y. Zhang, G. Li, X. Zhang and L. Lin, J. Mater. Chem. B, 2022, 10, 4575–4587 RSC.
- J. Shen, W. Shi, G. Liu, W. Zhuang, K. Wang, Y. Wang, K. Shou, W. Wu, X. Liu, Q. Fan and L. Zhang, J. Mater. Chem. B, 2023, 11, 5777–5785 RSC.
- M. Shao, A. Bigham, S. Yousefiasl, C. K. Y. Yiu, Y. R. Girish, M. Ghomi, E. Sharifi, S. Sezen, E. Nazarzadeh Zare, A. Zarrabi, N. Rabiee, A. C. Paiva-Santos, S. Del Turco, B. Guo, X. Wang, V. Mattoli and A. Wu, Small, 2023, 19, 2207057 CrossRef CAS.
- D. Liu, X. Dai, W. Zhang, X. Zhu, Z. Zha, H. Qian, L. Cheng and X. Wang, Biomaterials, 2023, 292, 121917 CrossRef CAS PubMed.
- X. Bao, J. Zhao, J. Sun, M. Hu and X. Yang, ACS Nano, 2018, 12, 8882–8892 CrossRef CAS.
- S. S. Evans, E. A. Repasky and D. T. Fisher, Nat. Rev. Immunol., 2015, 15, 335–349 CrossRef CAS.
- L. Ma, Y. Zhou, Z. Zhang, Y. Liu, D. Zhai, H. Zhuang, Q. Li, J. Yuye, C. Wu and J. Chang, Sci. Adv., 2020, 6, eabb1311 CrossRef CAS.
- R. Nie, Y. Sun, H. Lv, M. Lu, H. Huangfu, Y. Li, Y. Zhang, D. Wang, L. Wang and Y. Zhou, Nanoscale, 2022, 14, 8112–8129 RSC.
- Y. Chu, X.-Q. Xu and Y. Wang, J. Phys. Chem. Lett., 2022, 13, 9564–9572 CrossRef CAS.
|
This journal is © The Royal Society of Chemistry 2024 |
Click here to see how this site uses Cookies. View our privacy policy here.