A novel magnetically guided, oxygen propelled CoPt/Au nanosheet motor in conjugation with a multilayer hollow microcapsule for effective drug delivery and light triggered drug release†
Received
18th August 2023
, Accepted 15th November 2023
First published on 27th November 2023
Abstract
In recent years, nanomotors have been developed and attracted extensive attention in biomedical applications. In this work, a magnetically-guided oxygen-propelled CoPt/gold nanosheet motor (NSM) was prepared and used as an active self-propelled platform that can load, transfer and control the release of drug carrier to cancer cells. As a drug carrier, the microcapsules were constructed by the layer-by-layer (LbL) coating of chitosan and carboxymethyl cellulose layers, followed by incorporation of gold and magnetite nanoparticles. Doxorubicin (DOX) as an anti-cancer drug was loaded onto the synthesized microcapsules with a loading efficiency of 77%. The prepared NSMs can deliver the DOX loaded magnetic multilayer microcapsule to the target cancer cell based on the catalytic decomposition of H2O2 solution (1% v/v) via guidance from an external magnetic force. The velocity of NSM was determined to be 25.1 μm s−1 in 1% H2O2. Under near-infrared irradiation, and due to the photothermal effect of the gold nanoparticles, the proposed system was found to rapidly release more drugs compared to that of an internal stimulus diffusion process. Moreover, the investigation of cytotoxicity of NSMs and multilayer microcapsules clearly revealed that they have negligible side effects over all the concentrations tested.
1. Introduction
The development of new technologies is required to facilitate the delivery of pharmaceutical agents across all areas of the body, including those not accessible using traditional drug delivery systems.1 In recent years, synthetic nanomotors capable of converting chemical or external energy into mechanical motion were shown to have considerable potential for different functions, including water purification,2–4 biosensing,5–7 drug delivery,8–10 nano-surgery11 and imaging.12,13 Among these, the significant advancements in targeted drug delivery have been very promising. In fact, nanomotors as self-propelled platforms are essential for the design of drug delivery systems. Generally, nanomotors as nanosized devices were found to convert various forms of energy into mechanical motion.14,15
The nanomotors are classified as chemically propelled16,17 and nonchemically propelled18,19 motors, based on the potential for picking up, transporting and releasing therapeutic agents to the diseased tissues at a desired time and location in a comfortable and controllable way. Consequently, the efficiency of treatment is increased, while the side effects of the highly toxic drugs are reduced.20–23 Meanwhile, the navigation of nanomotors can be performed using a variety of external sources including, light, ultrasound, and magnetic fields.24
In recent years, Shamsipur et al. have published a number of US patents on the synthesis and characterization of various self-propelled nano/micromotors with different applications. For example, an aptamer-modified self-propelled nanomotor (MnO2-PEI/Ni/Au) was synthesized and used for transportation of human promyelocytic leukemia cells (HL-60) from human serum samples.25 They also reported a template-less self-propelled micromotor based on metallic gold and cobalt sandwiched polytryptophan for use in environmental remediation.26 Furthermore, for the first time, Shamsipur et al. synthesized the oxygen-propelled magnetically-guided nanosheet motors (NSMs) using a simple and rapid method.27 Here, the propulsion method of the nanosheet motors is based on the surface catalytic decomposition of hydrogen peroxide.28–30 Although the catalytic performance of these NSMs against degradation of organic pollutants has been investigated, there is no report on their utilization in drug delivery applications, including the transport and release of loading in a controlled manner.
Thus, in this work, we provide details of the performance of these catalytic NSMs for smart drug delivery. The preliminary results revealed that they can readily pick-up the biocompatible drug carriers and transport them towards the targeted cancer cells present. It should be noted that many drug delivery systems with specific properties including gels,31,32 polymeric micelles,33,34 liposomes,35,36 and colloids37 have already been developed as drug carriers. Among these, the multilayer microcapsules were found to be advantageous for controlled drug delivery.38–41 It should be noted that the microcapsules are fabricated using a layer-by-layer method via alternately adsorbing polyelectrolytes with opposite charges onto the colloidal templates, which can be dissolved later on.42 In this regard, the natural polysaccharides, due to their biocompatibility and biodegradability, are introduced as the best candidates for biomedical applications.43–45 Also, the multilayer microcapsules can load the drug and release it in a controlled manner under a specific external stimulus, such as temperature,46 electricity,47,48 ultrasound,49,50 light or laser irradiation51,52 using the magnetic fields53,54 to achieve an improved treatment outcome. Among these, the laser irradiation, particularly in the near-infrared (NIR) light region (800–1200 nm), is more suited for the microcapsule opening, as it can be easily transmitted through the body, due to its lower absorption and scattering in tissues with fine spatial control over intensity.55 Usually, NIR light triggered microcapsules are developed by incorporating gold and silver nanoparticles (NPs) or organic dyes into the walls of capsules.56,57
Herein, the calcium carbonate core was constructed as follows. First, chitosan (CS) and carboxymethylcellulose (CMC), as two biocompatible and biodegradable polyelectrolytes, were alternatively deposited onto the core, according to the principles of the LbL assembly. Two sorts of NPs including magnetite (Fe3O4) and gold (Au) NPs were incorporated into the microcapsule shells by the LbL procedure for different functions. As a result, the microcapsules could be magnetically loaded onto the NSMs. In our studies, we immobilized DOX, as one of the most effective anti-cancer drugs, and a model drug within the layers of the microcapsule.
It should be noted that DOX is a broad-spectrum anti-cancer drug and it has attracted much attention worldwide for more than forty years. Due to the low tumor selectivity of DOX, its therapeutic effects are limited and, thus, the toxicity of DOX toward both normal and tumor cells is increased.58,59 Specifically, the useful clinical application of DOX has been limited by the hypersensitivity and its cardiotoxicity.60 Accordingly, the introduction of an effective and controllable DOX drug delivery system has been of critical importance.
In this work, we used the novel NSM prepared previously by Shamsipur et al. This self-propelled NSM has coupling of unique properties of gold (Au) with a plate structure, magnetic cobalt (Co), and a catalytic platinum (Pt) as CoPt NPs. The self-propulsion of NSMs was performed by catalytic decomposition of hydrogen peroxide (H2O2 present at a low concentration of 1% v/v) into water and oxygen bubbles. Also, the cobalt segment present allows the NSM to be precisely guided and controlled using an external magnetic field. So, the prepared NSMs have the potential to pick up (by magnetic attraction) and transport DOX loaded multilayer microcapsules directly to cancerous tissues. As a result, the therapeutic efficacy improved and removed the systemic side effects of highly toxic drugs.
Also, due to the presence of Au NPs, we can release the drug into the cancerous tissue under the influence of laser light in a controlled manner.
The mentioned processes were performed in a microfluidic channel. Also, to solve the problem of toxicity of the synthesized nanocarrier, we examined the cytotoxicity of NSMs and the synthesized multilayer microcapsules in the presence and the absence of NIR laser irradiation. The results clearly revealed that our proposed nanosystem shows many of the suitable characteristics for ideal biomedical destined nanomotors, such as rapid autonomous motion, versatility, and stimuli-responsive controlled drug release. In fact, the NSM together with the DOX loaded magnetic multilayer microcapsule are an optimal drug delivery system with the best performance.
2. Experimental
2.1. Materials
Chitosan (CS, with low molecular weight), carboxymethyl cellulose (CMC), hydrogen tetrachloroaurate trihydrate (HAuCl4·3H2O), ferric chloride hexahydrate (FeCl3·6H2O) and ferrous chloride tetrahydrate (FeCl2·4H2O) were obtained from Sigma-Aldrich. Citric acid (C6H8O7), ethylenediamine tetra acetic acid disodium salt (EDTA), sodium hydroxide (NaOH), sodium chloride (NaCl), ammonium hydroxide (NH4OH), ammonium chloride (NH4Cl), boric acid (H3BO3), cobalt(II) chloride (CoCl2), platinum chloride (PtCl4), sodium carbonate (Na2CO3), glutaraldehyde (GA) and calcium chloride (CaCl2) were purchased from Merck.
2.2. Preparation of CoPt/gold nanosheet motors (NSMs)
The NSMs were synthesized using a method described previously.61 The Au electrode was oxidized at a potential of 6 V (versus Ag|AgCl saturated KCl) in PBS solution (0.5 M, pH 7.0) for 20 min, followed by reduction at a potential of −0.3 V for 5 min to prepare the nanofilm of Au. In the next stage, the Au nanofilm electrode was placed in a solution of NH4Cl (1.0 M) and H3BO3 (0.1 M) at pH 4.5 containing solutions of CoCl2 (0.1 mM) and Na2PtCl6 (0.02 mM) and reduced at a potential of −1.15 V for 20 min. The obtained electrode was washed with water for 10 s, then immersed in distilled water (2 mL). At the end, the obtained electrode was sonicated for 5 min to separate the synthesized NSMs. The solution was finally centrifuged at 2000 rpm and the separated products were used for the following studies.
2.3. Motion analysis of NSM
NSM solution with a concentration 0.002 mg mL−1 was ultrasonicated and mixed with H2O2 solution (1%). Then the prepared solution was transferred to a capillary microslide (0.1 mm thickness, 2 mm width, and 50 mm length). The movement was recorded for 30 s, and each second contained 30 frames s−1. The position (x–y coordinates) of 20 NSM is determined in each frame. The mean-square displacements (MSD) between the frames for each NSM were estimated by applying eqn 1 and the average velocity of each NSM was determined. | 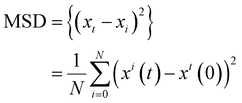 | (1) |
N: number of averaged NSM; xi and xt vector positions of NSM at different times.62
2.4. Preparation of citrate modified Fe3O4 nanoparticles
The Fe3O4 NPs modified with citric acid were prepared using a co-precipitation method as described previously.63 25 mL of 0.1 M FeCl2·4H2O and 0.2 M FeCl3·6H2O with a stoichiometric ratio of 1
:
2 were dissolved in distilled water in a water bath (60 °C) under a nitrogen atmosphere with vigorous stirring. To start the formation of the Fe3O4 NPs, NH4OH solution of 25% (5 mL) was added. Then, 1 g of citric acid (CA) solution was added into the above solution while the temperature was increased to 95 °C and maintained for one hour. The surface of the prepared Fe3O4NPs was covered with a negative charge. The resulting black precipitate was decanted with an external magnet and washed several times with distilled water.
2.5. Synthesis of gold nanoparticles (Au NPs)
The Au NPs were synthesized using the Kimura et al. method.64,65 HAuCl4 (20.8 mmol L−1, 0.5 mL) was dissolved in distilled water (20 mL) and heated until boiling, then 1 mL of 0.5% citrate solution was added under vigorous stirring. After a defined time of 60 min, the color of the solution was gradually altered to wine red, clarifying the formation of Au NPs.
2.6. Synthesis of the magnetic multilayer microcapsules
The core of the microcapsules, i.e., CaCO3 microparticles doped with CMC (CaCO3. CMC), was synthesized using the following method; the Na2CO3 (0.025 M, 100 mL) and Ca(NO3)2 (0.025 M, 100 mL) solutions were mixed with CMC solution (5%, 2 mL).63,66 After vigorous agitation with a magnetic stirrer for 30 s, the precipitate CaCO3 microparticles were obtained by sedimentation and washed with deionized water three times, and then dried in air. Finally, the CaCO3 (CMC) hybrid microparticles were obtained.
The LbL microcapsules were synthesized by alternative adsorption of CS and CMC onto the CaCO3 (CMC) colloidal template from polyelectrolyte solutions (2 mg mL−1) containing 0.5 M NaCl. After each layer of coating, the microparticles were separated by centrifugation (10
000 rpm, 1 min), followed by washing with 0.5 M NaCl solution (pH 5.0) three times. After 4 CS/CMC bilayers with CS as the outermost layer, citrate stabilized Fe3O4 NPs and citrate stabilized Au NPs from a 2 mg mL−1 suspension, were adsorbed, replacing negatively charged CMC layers in the third, fourth and fifth, and sixth bilayers, respectively. After completing the multilayer assembly, a glutaraldehyde (GA) solution (1%) was added to the solution of microcapsules and stirred at room temperature for 12 h to crosslink the CS polymer chains.67 The calcium carbonate core was removed with EDTA (0.2 M) at pH 7.0 to obtain the magnetic hollow microcapsules.67–69 The overall structure of the hollow microcapsule was as follows:
(CS/CMC)4 (CS/Fe3O4NPs)2 (CS/AuNP)2 (CS/CMC)4 CS. |
2.7. Analytical studies of loading DOX into the magnetic multilayer microcapsules
The syntheses of the multilayer microcapsules mentioned in the previous section were performed, except that before adding GA, we added 10 mL of the DOX drug with different concentrations (20–150 μg mL−1) and various pH values (2 to 9) to the synthesized multilayer microcapsule (0.1 mg) and incubated it for 24 h, then GA solution (1%) was added and stirred at room temperature for 12 h to crosslink the CS polymer chains. The calcium carbonate core was then removed with 0.2 M EDTA solution at pH 7.0.
The drug-loaded microcapsules were separated using a magnet and the amount of free DOX in the supernatant solution was quantified spectrophotometrically at the absorption maximum of λmax = 485 nm of the drug. The calibration curve of the DOX solution (A = 0.0199C + 0.002) showed a linear concentration range (2–30 μg mL−1). The DOX loading efficiency (LE) and drug loading content (LC) were also measured using the following formulas:
|  | (2) |
|  | (3) |
2.8.
In vitro release experiments of DOX from magnetic multilayer microcapsules
The influences of pH and NIR irradiation (780 nm) on the in vitro drug release from magnetic multilayer microcapsules were studied. The characteristic pH in tumor tissue is 5.0 (acidic) and in normal tissue is about 7.4. 1 mg of DOX loaded microcapsules were then dispersed in 10 mL buffer solution (0.005 M, pH = 5.0 and 7.4) incubated at 37 °C with gentle shaking without or with NIR irradiation of 200 mW output power at certain releasing time intervals. The amount of drug released over time was recorded using a UV-Vis spectrophotometer at 485 nm, and the concentration of DOX was calculated using a corresponding standard calibration curve.
2.9.
In vitro cytotoxicity test
First, the HeLa cells, derived from cervical cancer, were seeded at 2.0 × 103 cells per well in Dulbecco's MEM supplemented with fetal bovine serum (10%), penicillin (50 units mL−1) and streptomycin (50 μg mL−1). The cell cultures were incubated for 24 h in 5% CO2 and 95% air at 37 °C. Then, specified concentrations of DOX and the synthesized microcapsules (4–32 μg mL−1) were dissolved in cells and incubated for another 48 h. To investigate the photothermal effects, the cells were irradiated with NIR light (200 mW) for 15 min before 48 h incubation. After this time, MTT (5 mg mL−1 in PBS) was added to the cells for another 4 h. Finally, MTT-containing supernatant was removed and 100 μL of dimethyl sulfoxide (DMSO) was added to the cells and the absorbance was measured at 570 nm.
2.10. Laser-induced DOX release in HeLa cells
HeLa cells were seeded and incubated at 37 °C for 24 h. Free DOX and DOX loaded magnetic multilayer microcapsules (32 μg mL−1) were added to the cells, irradiated with NIR laser (780 nm) for 15 min and incubated for 4 h. Fluorescent dye (Hoechst 33342) was added into the cells and the fluorescence images were obtained using fluorescence microscopy.
2.11. Characterization
The morphology of the NSMs and microcapsules was observed with scanning electron microscopy (SEM) (Philips, Model XL-30). TEM images were obtained with a transmission electron microscope (Philips CM120) at an accelerating voltage of 120 kV. The structural analysis of the synthesized microcapsules was investigated using a Fourier transform infrared spectrophotometer (FTIR, Bruker, ALPHA, Germany) over the range of 400–4000 cm−1. The specific surface area and the pore size distribution of the CaCO3 (CMC) microparticles were measured using the Brunauer–Emmett–Teller method with N2 adsorption desorption at −196 °C and a surface analyzer model (BEL Japan).
The UV-Visible absorption spectra were evaluated using a UV-Vis. spectrophotometer (Agilent 8453, Santa Clara, CA, USA), equipped with a thermostated cell. Particle size distributions of NPs and zeta-potential values of the microcapsules were measured at a pH value of 5.0 with a Zeta-sizer (Malvern Instruments Ltd). The electrochemical studies were performed using a μAutolab electrochemical system (Eco-Chemise, Utrecht, and The Netherlands) equipped with GPES and FRA 4.9 software. A three-electrode assembled cell was employed, consisting of a gold disk electrode (3.0 mm diameter) as the working electrode, a platinum-wire as the counter electrode and an Ag|AgCl|saturated KCl electrode as the reference electrode. The movement of the NSMs and the ability of these NSMs to pick up and transport the drug carrier were investigated using an optical microscope (Olympus IX71) coupled to a digital camera (Olympus DP72). Fluorescence images were recorded using fluorescence microscopy (micros, Austria). A near-infrared (NIR) laser with a power of 200 mW at a wavelength of 780 nm was used. All reagents were of analytical grade.
3. Results and discussion
3.1. Synthesis of CoPt/gold nanosheet nanomotors (NSMs)
The synthesis of NSMs was performed with a simple and template-less method. Navigation of the NSMs can be carried out with hydrogen peroxide solution (1%) as the fuel.61 Characterization of the NSMs was carried out and the results are shown in the ESI,† Fig. S1. The NSMs have a gold plate structure (average thickness about ∼70 nm) on which CoPt alloy nanoparticles (average size about ∼35 nm) are deposited. Detailed information was provided in previous work.
In the absence of fuel (0% H2O2), the movement is Brownian-type, the diffusive behaviour could be observed, and MSD shows a linear correlation with Δt (MSD = 4DΔt). As shown in Fig. 1, the MSD curve of the NSM showed a nonlinear and parabolic shape according to the equation (4D)Δt + (v2)(Δt2),70 indicating directional movement and an autonomous bubble propulsion mechanism. The propulsion velocity was determined to be 25.1 μm s−1.
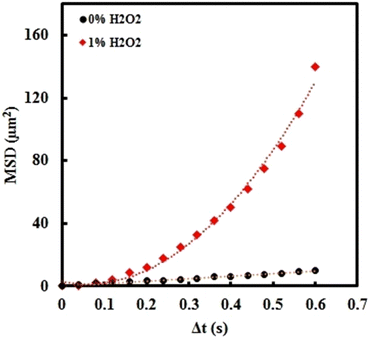 |
| Fig. 1 Mean squared displacement as a function of time interval for the NSMs at 0% and 1% H2O2. | |
3.2. Preparation and characterization of the hollow magnetic multilayer microcapsules
Multilayer microcapsules were prepared by the alternative coating of various layers of CS and CMC, magnetite and Au NPs on CaCO3 microparticles with the LbL method. During the microparticle synthesis, negatively charged CMC, as a biocompatible polymer, was added for controlling the crystallization of CaCO3 microparticles. By adding the CMC, the CaCO3 microparticles with a smaller particle size distribution and improved biocompatibility were prepared. SEM images (Fig. S2a and b, ESI†) show that the CaCO3 (CMC) microparticles have a regular spherical shape and were found to be ∼500 nm and exhibited an uneven surface (Fig. S2c, ESI†).
The XRD pattern (Fig. S2d, ESI†) of CaCO3 (CMC) microparticles shows distinct diffraction peaks relating to the vaterite phase.
The pore size distribution of CaCO3 (CMC) was determined by the Barrett–Joyner–Halenda method from the desorption branch of the isotherm and is shown in Fig. S3a (ESI†). It is obvious that the mean pore diameter of the microparticles is 5.6 nm. Nitrogen adsorption–desorption measurements (Figu. S3b, ESI†) indicate a surface area of 128.4 m2 g−1 which is much higher than that of CaCO3 microparticles without CMC (8.8 m2 g−1).69,71 Due to the absorption of CMC polymer molecules on the surface of carbonate microcrystals, they are loosely packed, and thus possess a larger surface area.
In the next stage, the CaCO3 (CMC) microparticles were coated with 12 bilayers of CS, CMC, Fe3O4 and Au NPs. The optimal pH value of CS and CMC solutions was kept at 5.0, because at this pH, both polymers are highly charged.68 For thermal therapy applications, the Au NPs were loaded into the microcapsule,69 which resulted in Au NPs with sizes ranging from 8–13 nm based on TEM and DLS measurements (Fig. S4a and b, ESI†). The Au NPs exhibited a maximum UV-Vis absorption peak at 560 nm (Fig. S4c, ESI†). Because of the coating of sodium citrate on Au NPs, their zeta-potential was observed at −24.5 mV.
Also, to remotely control and transfer the magnetic multilayer microcapsules to the desired site using a magnetic field, the Fe3O4 NPs were synthesized and introduced as a middle layer of biodegradable microcapsules. The Fe3O4 NPs synthesized using the co-precipitation method revealed a spherical shape with a mean diameter of about 50–100 nm, as confirmed by SEM images and DLS measurements (Fig. S5a and b, ESI†), and were successfully coated with citrate (as verified by the FTIR spectra (Fig. S5c, ESI†)). The multilayer buildup between the Au NPs/CS and Fe3O4 NPs/CS was carried out using the electrostatic interactions between the anionic citrate groups on NPs (Au and Fe3O4) and the cationic amino groups of the CS. After deposition of 14 bilayers on the surface of the CaCO3 (CMC) microparticles, the core was removed with EDTA solution.
For most cancer cell membranes, the surfaces usually show a negative charge, as the outer layer of the microcapsules modified with CS possess a good affinity toward cancer cells.72,73 The LbL deposition of oppositely charged polyelectrolytes was verified by the zeta-potential variations. As shown in Fig. S6 (ESI†), the zeta-potential of the bare CaCO3 (CMC) microparticles was −23.0 mV. The alternating charge potential variations implied successful deposition of polyelectrolytes. Subsequently, the magnetic multilayer microcapsules could be significantly stabilized by crosslinking the CS layer with GA followed by core removal with EDTA solution to obtain hollow microcapsules.
As shown in the SEM images (Fig. 2a and b), the prepared magnetic multilayer microcapsules exhibited spherical and smooth surface morphology. Meanwhile, because of the coating of CaCO3 (CMC) microparticles with multiple layers of the polyelectrolytes, the porous surface was changed to a smoother one. This is due to the adsorption of polymers with the opposite charges on top of each other, resulting in a low adhesion created between them. The SEM image of the hollow microcapsule (Fig. 2c) showed that the core of the microcapsule had been removed. BET analysis of the hollow multilayer microcapsule (Fig. S3c, ESI†) shows that the mean pore diameter is 3.6 nm. The porosity of the hollow multilayer microcapsule was about 42%.
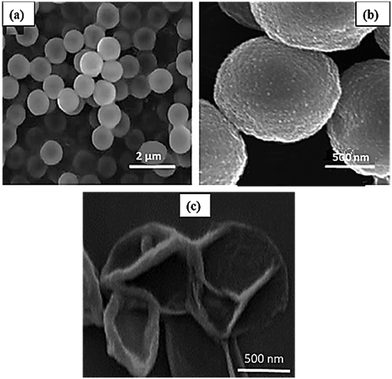 |
| Fig. 2 SEM images of (a) and (b) the synthesized magnetic multilayer microcapsules with two magnifications and (c) the hollow magnetic multilayer microcapsules. | |
The FTIR spectra of the synthesized microcapsules and their components are shown in Fig. 3. The spectral data obtained for the CaCO3 microparticles (Fig. 3a) revealed some broad absorption peaks at 1380, 1009, 877, and 746 cm−1, which are the characteristic modes of vibration for this molecule.72 Regarding the CS spectrum shown in Fig. 3b, the absorption band at 3450 cm−1 is related to N–H and O–H groups (stretching vibration) and the bands at 1649 and 1603 cm−1 are due to the amide I and II groups.74
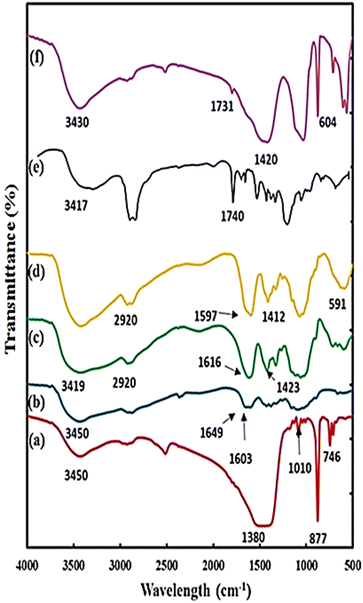 |
| Fig. 3 FT-IR spectra of (a) CaCO3 microparticles, (b) CS, (c) CMC, (d) hollow microcapsules, (e) pure DOX and (f) DOX loaded hollow microcapsules. | |
In the spectrum of CMC shown in Fig. 3c, the broadband at 3419 cm−1 is due to the O–H stretching vibration of the OH group and the band at 2920 cm−1 shows the C–H stretching vibration of the CH2 and CH3 groups. While the peaks at 1616 and 1423 cm−1 indicate the stretching vibrations of the COO group.75,76
For the magnetic multilayer microcapsules (Fig. 3d), the absorption of the C
O group of CMC at 1423 cm−1 and amide I and II bands for CS disappeared. Instead, new absorption peaks appearing at 1412 and 1597 cm−1 are the specific bands of the COO− and NH3+, respectively, which means strong electrostatic interactions between CMC and CS in the microcapsules.74 Meanwhile, the most notable specialty for the spectrum of magnetic multilayer microcapsules is the appearance of a strong peak at 591 cm−1, which is related to the Fe–O bond that confirms the incorporation of Fe3O4 NPs in the microcapsule shells.
3.3. Analytical studies of DOX loading into magnetic multilayer microcapsules for magnetic multilayer microcapsules
Here, the magnetic multilayer microcapsules were mixed with DOX solution. DOX with the positive charge was loaded into the multilayer microcapsules. The intensities of the UV-Vis absorption peak of DOX at 485 nm before and after the drug loading process are compared in Fig. 4a; the loading efficiency is as high as 75%.
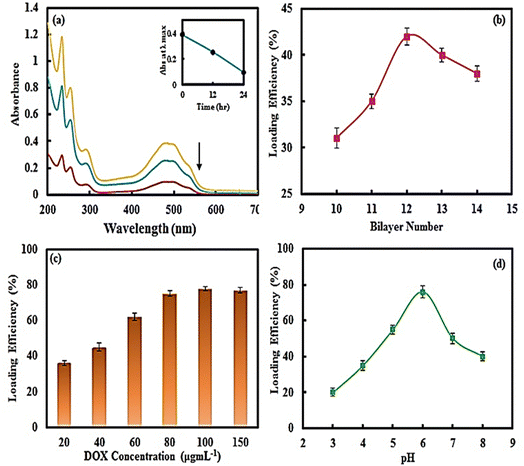 |
| Fig. 4 (a) UV-Vis absorbance spectra of DOX before and after the drug loading process. Inset: Variation of absorbance at λ max (485 nm) of DOX at different times. (b) Effect of the thickness of the layers on DOX loading efficiency. (c) The effect of different concentrations of DOX on the loading efficiency as the pH was kept constant at 5.0. (d) The effect of different pH values on DOX loading efficiency. | |
3.3.1. Effect of thickness of the layers on DOX loading efficiency.
The number of the deposited layers has a high impact on the amount of loaded drug into magnetic multilayer microcapsules. The influence of the thickness of layers on drug loading efficiency was investigated. Fig. 4b shows that the amount of drug loading efficiency is varied with the number of layers (as the concentration of DOX was kept constant at 50 μg mL−1). With the coating of 12 bilayers on the surface of microcapsules, the maximum loading efficiency of DOX was 42% and for less and more than 12 bilayers, the loading amount of DOX is reduced. As the number of layers increases, the interaction between drug and polymer backbone increases, However, for more than 12 bilayers, packing of the microcapsule layers occurs, which prevents more loading of the drug. Thus, 12 bilayers are preferred for microcapsule formation.
3.3.2. Effect of various concentrations of DOX on the DOX loading efficiency.
For investigation of the influence of DOX concentration on loading efficiency, the magnetic multilayer microcapsules (0.1 mg) were mixed with different concentrations of DOX solution (20–150 μg mL−1). As shown in Fig. 4c, the maximum loading efficiency and drug loading content of DOX into magnetic multilayer microcapsules are found to be 78% and 44%, respectively. When the DOX concentration increased up to 100 μg mL−1, the aggregation effect of DOX was more prominent. Moreover, when the microcapsule concentration was not sufficient to encapsulate the high concentration of the drug, the loading efficiency decreased.
3.3.3. Effect of varying pH values on the DOX loading efficiency.
The DOX loading efficiencies at different pH values of the magnetic multilayer microcapsule's solutions, over the range of 3.0 to 8.0, were determined (Fig. 4d). As is obvious from the results, the maximum amount of loaded DOX was obtained at pH 6.0. Thus, the drug loading experiments were recorded at pH 6.0.
To confirm the loading of DOX on magnetic multilayer microcapsules, the FTIR spectrum of DOX loaded magnetic CMC/CS multilayer microcapsules was also studied. For comparison, the FTIR spectrum of DOX is also presented (Fig. 3e). From Fig. 3e and f, the sharp peak around 1740 cm−1 in pure DOX (due to C
O) shifted to 1731 cm−1. Meanwhile, the broad peak at 3417 cm−1 (corresponding to OH stretching) became sharper and shifted to 3430 cm−1 after loading into the microcapsule. The absorption band at 1412 cm−1 (for COO−) in the spectrum of magnetic multilayer microcapsules shifted to 1420 cm−1 in the spectrum of DOX-loaded magnetic multilayer microcapsules, mainly due to its electrostatic interaction with amino groups (NH3+) in DOX. These results strongly support the fact that the DOX is substantially encapsulated in the magnetic multilayer microcapsules.
3.4. NIR- and pH-triggered release of DOX from the magnetic multilayer microcapsules
The DOX release from the magnetic multilayer microcapsules was studied at a physiological temperature of 37 °C. In addition, the influence of pH on the in vitro drug release was considered. It is well-known that the characteristic pH in tumor tissue is acidic, at about 5.0, and in normal tissue is about 7.4. Therefore, the release experiment was performed at a pH of 5.0 and 7.4.
3.4.1. Effect of pH on in vitro drug release.
As shown in Fig. 5a, the release rate was relatively slow and the drug release process continued for 24 hours. By comparing the release profiles at pH 5.0 and pH 7.4, it is noted that the DOX has a faster release rate at an acidic pH 5.0, thus confirming the pH-controlled drug release from the microcapsules. The results thus obtained confirmed that our proposed system is less toxic toward healthy tissues (pH 7.4) in the body, confirming the safety of the delivery system.77
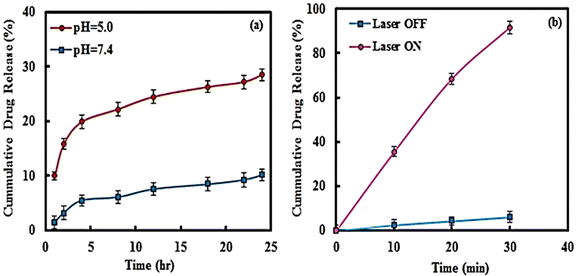 |
| Fig. 5 (a) Release of DOX at different pH values without NIR irradiation at 37 °C; the graph shows the sustained release of DOX under physiological conditions. (b) Photo-responsive release of DOX in the dark and under irradiation of NIR laser into the tumor tissue (pH 5.0). | |
3.4.2. Effect of NIR irradiation on in vitro drug release.
As the Au NPs possess high NIR light absorption coefficients, their loaded magnetic multilayer microcapsules have already been used as photothermal conversion agents for photothermal therapy.78 Fig. S7 (ESI†) shows the photothermal effects of water, Au NPs and an aqueous dispersion of magnetic multilayer microcapsule (1 mg) under NIR irradiation (780 nm) at a power density of 200 mW cm−2.
The temperature of the Au NPs and magnetic multilayer microcapsules increased within 15 min of irradiation with a 780 nm laser and reached 52 and 49 °C, respectively. In contrast, no temperature change was detected for water.
In the next step, the release of DOX from magnetic multilayer microcapsules under the NIR irradiation was studied. As illustrated in Fig. 5b, with increasing irradiation time, the release of DOX increased, so that after 15 min irradiation, the amount of drug released reached 90%. It should be noted that under dark conditions, only 10% of the drug is released after 15 min.
It should be noted that, under NIR irradiation, the temperature increased because of the photothermal effect of the Au NPs used, and the microcapsules will collapse which results in the release of DOX. According to the results thus obtained, the appropriate irradiation time for complete drug release was fixed as 15 min.
GA as a crosslinking agent, was added to the microcapsule solution, to increase the stability of the polyelectrolyte coated on the surface of the microcapsule. It should be noted that at higher temperatures, GA is unstable. In fact, with increasing temperature of the magnetic multilayer microcapsules under NIR laser irradiation, the aldehyde groups of GA degraded and, consequently, result in degradation of the magnetic multilayer microcapsule and successful release of the loaded drug.
It is very important to notice that our designed system resulted in a significant increase in the release of more drug, under external NIR irradiation compared to that over the internal stimulus (diffusion) condition. The thus obtained results clearly reveal the fact that the prepared magnetic multilayer microcapsule possesses a remarkable potential as a much more responsive and controllable drug delivery system.
3.5.
In vitro cytotoxicity analysis
Since the biocompatibility of the drug delivery systems is a prerequisite for their biomedical application, we carried out an in vitro cytotoxicity assay as follows. The HeLa cells were incubated at different concentrations of 4–32 μg mL−1 of the synthesized nanostructures and the results obtained are shown in Fig. 6. From the result shown in Fig. 6a, it is evident that the NSMs led to a negligible change in the cell viability, which is quite favorable in the physiological environments. It should be noted that the empty magnetic multilayer microcapsules exhibited non-or-low cytotoxicity toward the cells. The results thus obtained indicate that the hollow magnetic multilayer microcapsules are biocompatible at relatively high concentrations of up 32 μg mL−1. Interestingly, the DOX-loaded magnetic multilayer microcapsules could kill about 17–55% of the cancer cells at 24 h over the above-mentioned concentration range. The results are also quite understandable, due to the partial release of DOX from the magnetic multilayer microcapsules. To investigate the potential applications of the NSMs and magnetic multilayer microcapsules as magnetic/NIR-responsive drug carriers, their in vitro cytotoxicity under NIR irradiation was studied, and the corresponding results are presented in Fig. 6b. As is quite obvious, under 15 min NIR irradiation, the empty magnetic multilayer microcapsules significantly enhanced the cytotoxicity, over a range of 30–65% killing of the tumor cells over a concentration of 4–32 μg mL−1. In comparison, the control experiment with NIR irradiation for 15 min exhibited no cytotoxicity toward the HeLa cells (see Fig. S8, ESI†), indicating the photothermal effect in the presence of empty magnetic multilayer microcapsules.
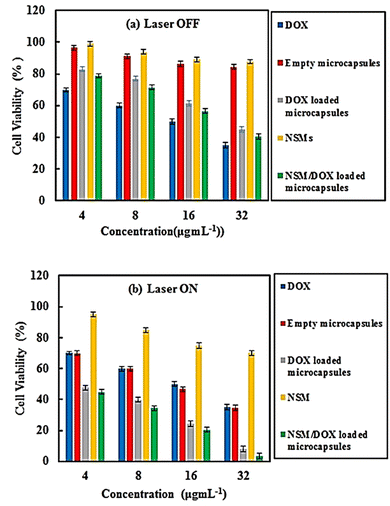 |
| Fig. 6 Viability of HeLa cells with DOX, empty and DOX loaded microcapsules, NSMs, and NSM/DOX loaded microcapsules (a) with and (b) without NIR laser irradiation (780 nm, 200 mw for 15 min). | |
Also, under 15 min NIR irradiation, the DOX-loaded magnetic multilayer microcapsules possessed a greater influence of 52–100% killing of the tumor cells over the same concentration range that is quite remarkable. It should be noted that the cytotoxicity when the DOX-loaded magnetic multilayer microcapsules and NSMs were used together (magnetic multilayer microcapsule/NSM) was nearly the same as or a little better than the cytotoxicity when the only DOX-loaded magnetic multilayer microcapsules were used. In fact the DOX-loaded magnetic multilayer microcapsules/NSMs can be a highly efficient nanocarriers for photo triggered drug release in cancer treatment.
3.6. Application of NSMs to drug delivery
3.6.1. Transport and release of magnetic multilayer microcapsules.
NSMs are important for targeted drug delivery. As shown in Video 1 in the ESI,† in 1% H2O2 solution, the NSMs move spontaneously by producing oxygen bubbles from the surface of NSMs (Fig. S9a–c, ESI†). The NSMs are also quickly moved toward a magnetic direction by applying an external magnet (Fig. S9d–f, ESI†). In this work, we verified the potential performance of the NSMs for controlled transportation of DOX-loaded magnetic multilayer microcapsules to cancer cells through a microchannel network, as schematically shown in Fig. 7A. The related experimental results are displayed in Fig. 7B (see the ESI,† Videos 2–4). Experiments were performed with HeLa cancer cells. Using H2O2 solution with a low concentration (1%), the HeLa cells survive for more than 90 min.20 Repeatedly washed NSMs and DOX loaded magnetic multilayer microcapsules were added to the PBS solution (pH 7.4) in the corresponding wells and the HeLa cells were seeded and cultured in the release well.
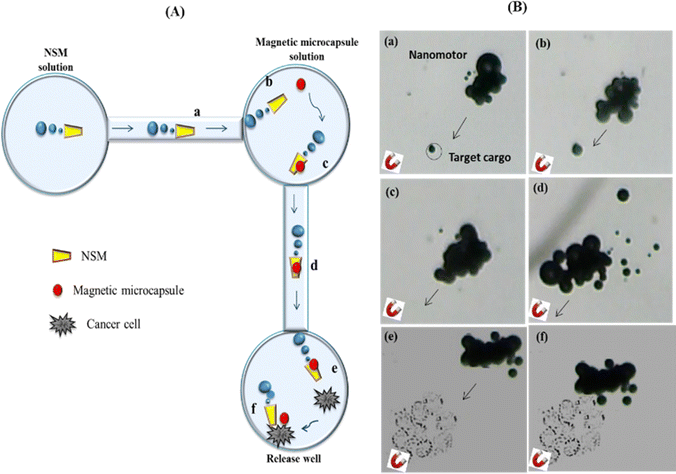 |
| Fig. 7 Pick-up and transport of a DOX loaded magnetic multilayer microcapsule to HeLa cells in a PDMS microchannel using NSM. (A) Scheme depicting the process as a NSM is magnetically navigated to a DOX loaded microcapsule (cargo) (a) and (b), followed by the capture of the DOX loaded microcapsule in the loading reservoir (c), transporting it through the channel (d), followed by approaching the HeLa cell (e) and sticking onto the HeLa cell (f) in PBS solution with 1% H2O2 at 37 ○C. (B). Time-lapse images illustrating each of the previous steps described in (A), as they are taken from Video 2 (ESI†), and the arrows show the moving direction of the NSM. | |
First, the NSMs magnetically approach the target DOX loaded magnetic multilayer microcapsules and can pick them up well with a cube-shaped Neodymium (NdFeB) magnet (0.5 Tesla) (a) and (b). When the NSM is close to the magnetic multilayer microcapsule, the microcapsule is attached by magnetic attraction and picked up (c) (see the ESI,† Video 2). After capturing the magnetic multilayer microcapsule, the NSM was navigated using a magnetic field along the channel (d) (see the ESI,† Video 3) (e). The DOX-loaded magnetic multilayer microcapsule/NSMs approached (f) and attached to the cancer cells in the corresponding well containing PBS solution with 1% H2O2 at 37 °C (see the ESI,† Video 4).
3.6.2. NIR-stimulated release studies of the drug from the magnetic multilayer microcapsules.
When the cargo loaded NSMs attached to the HeLa cells, the microcapsules could release the drug under the exposure of a NIR laser at 780 nm. Fig. 8 shows the results of DOX release from microcapsules at the cellular level at pH 5.0.
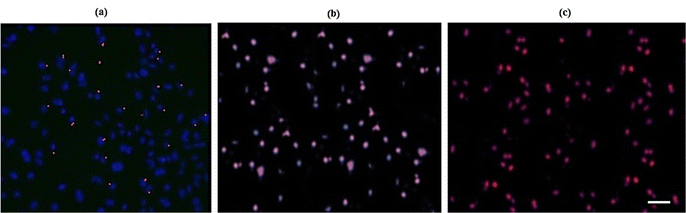 |
| Fig. 8 The fluorescence images of HeLa cells incubated with microcapsules containing Au NPs (a) before and (b) after NIR light irradiation at 780 nm at a power output of 200 mW, and (c) the HeLa cells incubated with pure DOX. HeLa cells counter-stained with Hoechst 33342 (blue). Magnification ×20. | |
For successfully combining HeLa cells with DOX loaded microcapsules, the HeLa cells were incubated with them for 12 h. As shown in Fig. 8, the blue fluorescence and red fluorescence are related to nuclear dyes Hoechst 33342 and DOX, respectively. At first, without laser irradiation, the red fluorescence and blue fluorescence did not overlap together because most of the DOX was attached to the microcapsules and didn’t enter the cell.
As a result, while the cells were exposed to a NIR laser, Au NPs absorb the light and the temperature of the microcapsule increased; thus, the microcapsule shell was ruptured and DOX leaked out of the microcapsule and entered into the cells. Consequently, with the triggered release of DOX under NIR irradiation, the red color from the fluorescence of DOX was seen and covered with the blue fluorescence of the dye. These results are significant for advanced drug delivery.
4. Conclusion
In this work, we studied the significant performance of a Co/Pt Au nanosheet (NSM) for smart drug delivery in a microchannel network. Magnetic and light-sensitive magnetic multilayer microcapsules that can integrate magnetic Fe3O4 and Au NPs into their walls were synthesized and used as carriers for an anti-cancer DOX drug. In this system, (1) the DOX-loaded multilayer magnetic microcapsule was picked up as cargo on the NSMs through magnetic interaction in the first reservoir, (2) the loaded NSMs self-propelled in H2O2 solution and navigated using a magnet to the well containing cancer cells, where the release of drug was performed and (3) the Au NPs incorporated into the microcapsule walls served as light-absorbing centers. The Au NPs allow the NIR triggered microcapsule to open based on a photothermal effect and remotely release the encapsulated drug. In this work, for the first time we investigated the cytotoxicity effects of the NSMs on HeLa cells. We also found that the NSMs do not exhibit toxicity effects on the viability of the HeLa cell. After NIR irradiation for 15 min, the DOX-loaded magnetic multilayer microcapsules/NSMs had a greater impact on killing the cancer cells, compared to the obtained results from cell viability in the absence of NIR irradiation. The prepared NSMs showed promising potential as an effective platform for fast delivery and triggered release of drugs in the biomedical field.
Conflicts of interest
There are no conflicts to declare.
References
-
Q. Ye and J. Sun, Nanorobotics and Nanodiagnostics in Integrative Biology and Biomedicine, Springer, 2022, pp. 15–34 DOI:10.1007/978-3-031-16084-4_2.
- B. Verma, S. P. Gumfekar and M. Sabapathy, Can. J. Chem. Eng., 2022, 100, 540–558 CrossRef CAS.
- X. Li, Y. Zhao, D. Wang and X. Du, Colloids Surf., A, 2023, 658, 130712 CrossRef CAS.
- S. Chang, Mater. Today Sustainability, 2022, 100196 CrossRef.
- A. Esmaeili and N. A. Hadad, Ceram. Int., 2015, 41, 7529–7535 CrossRef CAS.
- R. Maria-Hormigos, B. Jurado-Sánchez and A. Escarpa, Anal. Bioanal. Chem., 2022, 414, 7035–7049 CrossRef CAS.
- Y. Hu, Z. Li and Y. Sun, J. Colloid Interface Sci., 2022, 621, 341–351 CrossRef CAS.
- W. Gao, B. E.-F. de Ávila, L. Zhang and J. Wang, Adv. Drug Delivery Rev., 2018, 125, 94–101 CrossRef CAS PubMed.
- W. Zhang, Z. Zhang, S. Fu, Q. Ma, Y. Liu and N. Zhang, Chem. Phys. Mater., 2023, 2, 114–125 Search PubMed.
- Y. Sun, R. Pan, Y. Chen, Y. Wang, L. Sun, N. Wang, X. Ma and G. P. Wang, ACS Nanosci. Au, 2022, 3, 94–102 CrossRef PubMed.
- S. K. Srivastava, M. Medina-Sánchez, B. Koch and O. G. Schmidt, Adv. Mater., 2016, 28, 832–837 CrossRef CAS.
- X. Wei, M. Beltrán-Gastélum, E. Karshalev, B. Esteban-Fernández de Ávila, J. Zhou, D. Ran, P. Angsantikul, R. H. Fang, J. Wang and L. Zhang, Nano Lett., 2019, 19, 1914–1921 CrossRef CAS PubMed.
- C. M. Oral, M. Ussia, M. Urso, J. Salat, A. Novobilsky, M. Stefanik, D. Ruzek and M. Pumera, Adv. Healthcare Mater., 2023, 12, 2202682 CrossRef CAS.
- F. Peng, Y. Tu and D. A. Wilson, Chem. Soc. Rev., 2017, 46, 5289–5310 RSC.
- J. Dan, S. Shi, H. Sun, Z. Su, Y. Liang, J. Wang and W. Zhang, Crit. Rev. Food Sci. Nutr., 2022, 1–21 CrossRef PubMed.
- Y. Tu, F. Peng, X. Sui, Y. Men, P. B. White, J. C. van Hest and D. A. Wilson, Nat. Chem., 2017, 9, 480–486 CrossRef CAS PubMed.
- Y. Feng, M. An, Y. Liu, M. T. Sarwar and H. Yang, Adv. Funct. Mater., 2023, 33, 2209883 CrossRef CAS.
- H. Zhou, C. C. Mayorga-Martinez, S. Pané, L. Zhang and M. Pumera, Chem. Rev., 2021, 121, 4999–5041 CrossRef CAS PubMed.
- J. Li, C. C. Mayorga-Martinez, C. D. Ohl and M. Pumera, Adv. Funct. Mater., 2022, 32, 2102265 CrossRef CAS.
- D. Kagan, R. Laocharoensuk, M. Zimmerman, C. Clawson, S. Balasubramanian, D. Kang, D. Bishop, S. Sattayasamitsathit, L. Zhang and J. Wang, Small, 2010, 6, 2741–2747 CrossRef CAS.
- Z. Wu, Y. Wu, W. He, X. Lin, J. Sun and Q. He, Angew. Chem., Int. Ed., 2013, 52, 7000–7003 CrossRef CAS PubMed.
- B. Esteban-Fernández de Ávila, D. E. Ramírez-Herrera, S. Campuzano, P. Angsantikul, L. Zhang and J. Wang, ACS Nano, 2017, 11, 5367–5374 CrossRef.
- D. Xu, Y. Wang, C. Liang, Y. You, S. Sanchez and X. Ma, Small, 2020, 16, 1902464 CrossRef CAS.
- S. M. Beladi-Mousavi, B. Khezri, L. Krejcova, Z. K. Heger, Z. K. Sofer, A. C. Fisher and M. Pumera, ACS Appl. Mater. Interfaces, 2019, 11, 13359–13369 CrossRef CAS.
- M. A. Tabrizi, M. Shamsipur, R. Saber and S. Sarkar, Biosens. Bioelectron., 2018, 110, 141–146 CrossRef.
- N. Moradi, M. Shamsipur, A. Taherpour, N. Rahimdad and A. Pashabadi, ChemPlusChem, 2020, 85, 1129–1136 CrossRef CAS PubMed.
- M. A. Tabrizi and M. Shamsipur, RSC Adv., 2015, 5, 51508–51511 RSC.
- A. A. Solovev, Y. Mei, E. Bermúdez Ureña, G. Huang and O. G. Schmidt, Small, 2009, 5, 1688–1692 CrossRef CAS.
- J. Wang and K. M. Manesh, Small, 2010, 6, 338–345 CrossRef CAS.
- A. A. Solovev, S. Sanchez, M. Pumera, Y. F. Mei and O. G. Schmidt, Adv. Funct. Mater., 2010, 20, 2430–2435 CrossRef CAS.
- A. Prasannan, H.-C. Tsai and G.-H. Hsiue, React. Funct. Polym., 2018, 124, 40–47 CrossRef CAS.
-
P. Shrivastava, N. Vishwakarma, L. Gautam and S. P. Vyas, Smart Polymeric Nano-Constructs in Drug Delivery, Elsevier, 2023, pp. 129–150 Search PubMed.
- C.-Y. Su, J.-J. Liu, Y.-S. Ho, Y.-Y. Huang, V. H.-S. Chang, D.-Z. Liu, L.-C. Chen, H.-O. Ho and M.-T. Sheu, Eur. J. Pharm. Biopharm., 2018, 123, 9–19 CrossRef CAS PubMed.
- A. Guzmán Rodríguez, M. Sablón Carrazana, C. Rodríguez Tanty, M. J. Malessy, G. Fuentes and L. J. Cruz, Cancers, 2022, 15, 4 CrossRef.
- T. Zhang, S. Zhou, L. Hu, B. Peng, Y. Liu, X. Luo, X. Liu, Y. Song and Y. Deng, Drug Delivery Transl. Res., 2018, 8, 602–616 CrossRef CAS.
- P. Namakshenas and A. Mojra, Int. J. Mech. Sci., 2023, 237, 107818 CrossRef.
- S. Peers, A. Montembault and C. Ladaviere, Carbohydr. Polym., 2022, 275, 118689 CrossRef CAS PubMed.
- S. Heidari, M. Akhlaghi, M. Sadeghi, A. M. Kheirabadi, D. Beiki, A. E. Ardekani, A. Rouhollah, P. Saeidzadeh and R. Soleyman, J. Drug Delivery Sci. Technol., 2022, 73, 103477 CrossRef CAS.
- T. Kruk, K. Chojnacka-Górka, M. Kolasińska-Sojka and S. Zapotoczny, Adv. Colloid Interface Sci., 2022, 102773 CrossRef CAS.
- T. Bukreeva, T. Borodina and D. Trushina, Colloid J., 2022, 84, 621–632 CrossRef CAS.
- V. Kozlovskaya, A. Alford, M. Dolmat, M. Ducharme, R. Caviedes, L. Radford, S.
E. Lapi and E. Kharlampieva, ACS Appl. Mater. Interfaces, 2020, 12, 56792–56804 CrossRef CAS.
- K. V. Jardim, A. F. Palomec-Garfias, B. Y. G. Andrade, J. A. Chaker, S. N. Báo, C. Márquez-Beltrán, S. E. Moya, A. L. Parize and M. H. Sousa, Mater. Sci. Eng., C, 2018, 92, 184–195 CrossRef CAS.
- X. Zhu, J. Shi, H. Ma, R. Chen, J. Li and S. Cao, Mater. Sci. Eng., C, 2019, 99, 1236–1245 CrossRef CAS.
- Q. Meng, S. Zhong, Y. Gao and X. Cui, Int. J. Biol. Macromol., 2022, 220, 878–891 CrossRef CAS PubMed.
- Q. Meng, S. Zhong, J. Wang, Y. Gao and X. Cui, Carbohydr. Polym., 2023, 300, 120265 CrossRef CAS.
- D. Zheng, A. Ramos-Sebastian, W. S. Jung and S. H. Kim, Composites, Part B, 2022, 230, 109551 CrossRef CAS.
- A. Baba, P. Taranekar, R. R. Ponnapati, W. Knoll and R. C. Advincula, ACS Appl. Mater. Interfaces, 2010, 2, 2347–2354 CrossRef CAS.
- G. Liu, S. Xu, Y. Liu, Y. Gao, T. Tong, Y. Qi and C. Zhang, Adv. Funct. Mater., 2020, 30, 1909886 CrossRef CAS.
- A. Alford, M. Rich, V. Kozlovskaya, J. Chen, J. Sherwood, M. Bolding, J. Warram, Y. Bao and E. Kharlampieva, Adv. Therapeutics, 2018, 1, 1800051 CrossRef.
-
L. Tu, Z. Liao, Z. Luo, Y. L. Wu, A. Herrmann and S. Huo, 2021.
- Y. Rui, B. Pang, J. Zhang, Y. Liu, H. Hu, Z. Liu, S. Ama Baidoo, C. Liu, Y. Zhao and S. Li, Artif. Cells, Nanomed., Biotechnol., 2018, 46, 15–24 CrossRef CAS.
- D. Chen, Z. Jin, B. Zhao, Y. Wang and Q. He, Adv. Mater., 2021, 33, 2008089 CrossRef CAS.
- D. Luo, R. N. Poston, D. J. Gould and G. B. Sukhorukov, Mater. Sci. Eng., C, 2019, 94, 647–655 CrossRef CAS PubMed.
- C. Takei, K. Mori, T. Oshizaka and K. Sugibayashi, Chem. Pharm. Bull., 2022, 70, 50–51 CrossRef CAS.
- T. Zhou, S. Xie, C. Zhou, Y. Chen, H. Li, P. Liu, R. Jiang, L. Hang and G. Jiang, ACS Appl. Bio Mater., 2022, 5, 3841–3849 CrossRef CAS PubMed.
- Z. Qi, J. Shi, Z. Zhang, Y. Cao, J. Li and S. Cao, Mater. Sci. Eng., C, 2019, 104, 109889 CrossRef CAS PubMed.
- Z. Wu, J. Shi, P. Song, J. Li and S. Cao, Int. J. Biol. Macromol., 2021, 183, 870–879 CrossRef CAS.
- J. Li, S. Ying, H. Ren, J. Dai, L. Zhang, L. Liang, Q. Wang, Q. Shen and J.-W. Shen, Int. J. Pharm., 2020, 580, 119241 CrossRef CAS.
- M. D. A. Paskeh, H. Saebfar, M. K. Mahabady, S. Orouei, K. Hushmandi, M. Entezari, M. Hashemi, A. R. Aref, M. R. Hamblin and H. L. Ang, Life Sci., 2022, 298, 120463 CrossRef CAS.
- S. Peter, S. Alven, R. B. Maseko and B. A. Aderibigbe, Molecules, 2022, 27, 4478 CrossRef CAS.
- M. Amouzadeh Tabrizi and M. Shamsipur, RSC Adv., 2015, 5, 51508–51511 RSC.
- P. Diez, E. Lucena-Sanchez, A. Escudero, A. Llopis-Lorente, R. Villalonga and R. Martinez-Manez, ACS Nano, 2021, 15, 4467–4480 CrossRef CAS.
- C. Zheng, Y. Ding, X. Liu, Y. Wu and L. Ge, Int. J. Pharm., 2014, 475, 17–24 CrossRef CAS PubMed.
- S. Chen and K. Kimura, Langmuir, 1999, 15, 1075–1082 CrossRef CAS.
- Y. Wu, J. Frueh, T. Si, H. Möhwald and Q. He, Phys. Chem. Chem. Phys., 2015, 17, 3281–3286 RSC.
- J. Tripathy and A. M. Raichur, Colloids Surf., B, 2013, 101, 487–492 CrossRef CAS PubMed.
- A. Yashchenok, B. Parakhonskiy, S. Donatan, D. Kohler, A. Skirtach and H. Mohwald, J. Mater. Chem. B, 2013, 1, 1223–1228 RSC.
- N. A. N. Hanafy, M. L. De Giorgi, C. Nobile, R. Rinaldi and S. Leporatti, Beni-Suef University Journal of Basic and Applied Sciences, 2015, 4, 60–70 CrossRef.
- C. Peng, Q. Zhao and C. Gao, Colloids Surf., A, 2010, 353, 132–139 CrossRef CAS.
- W. Duan, M. Ibele, R. Liu and A. Sen, Eur. Phys. J. E, 2012, 35, 1–8 CrossRef.
- D. V. Volodkin, N. I. Larionova and G. B. Sukhorukov, Biomacromolecules, 2004, 5, 1962–1972 CrossRef CAS PubMed.
- J. Tang, B. Kong, H. Wu, M. Xu, Y. Wang, Y. Wang, D. Zhao and G. Zheng, Adv. Mater., 2013, 25, 6569–6574 CrossRef CAS.
- G. Pasut, F. Canal, L. Dalla Via, S. Arpicco, F. M. Veronese and O. Schiavon, J. Controlled Release, 2008, 127, 239–248 CrossRef CAS.
- S. Yan, J. Zhu, Z. Wang, J. Yin, Y. Zheng and X. Chen, Eur. J. Pharm. Biopharm., 2011, 78, 336–345 CrossRef CAS.
- K. Madhusudana Rao, B. Mallikarjuna, K. Krishna Rao, M. Prabhakar, K. Chowdoji Rao and M. Subha, Polym. Bull., 2012, 68, 1905–1919 CrossRef CAS.
- A. J. Braihi, S. I. Salih, F. A. Hashem and J. K. Ahmed, Int. J. Mater. Sci. Appl., 2014, 3, 363–369 Search PubMed.
-
Q. Zhao, B. Han, Z. Wang, C. Gao, C. Peng and J. Shen, Nanomedicine in Cancer, Jenny Stanford Publishing, 2017, pp. 317–345 Search PubMed.
- H. Chen, Y. Di, D. Chen, K. Madrid, M. Zhang, C. Tian, L. Tang and Y. Gu, Nanoscale, 2015, 7, 8884–8897 RSC.
|
This journal is © The Royal Society of Chemistry 2024 |
Click here to see how this site uses Cookies. View our privacy policy here.