Effects of matrix viscoelasticity on cell–matrix interaction, actin cytoskeleton organization, and apoptosis of osteosarcoma MG-63 cells†
Received
30th August 2023
, Accepted 25th November 2023
First published on 11th December 2023
Abstract
Many recent reports have shown the effects of viscoelasticity of the extracellular matrix on the spreading, migration, proliferation, survival and cell–matrix interaction of mesenchymal stem cells and normal cells. However, the effect of matrix viscoelasticity on the behavior of tumor cells is still in the state of preliminary exploration. To this aim, we prepared a viscoelastic hydrogel matrix with a storage modulus of about 2 kPa and a loss modulus adjustable from 0 to 600 Pa, through adding linear alginate and regulating the compactness of a polyacrylamide covalent network. Overall, the addition of viscous components inhibited the apoptosis of osteosarcoma MG-63 cells, while it promoted their spreading and proliferation and in particular led to a well-developed cytoskeleton organization. However, with the increase of the viscous fraction, this trend was reversed, and the apoptosis of MG-63 cells gradually increased with gradually decreased spreading and proliferation, accompanied by a surprising manner change of the cytoskeleton from fusiform cells dominated by focal adhesion to dendritic cells dominated by pseudopodia. Besides the upregulation of MAPK, Ras, Rap1 and PI3K-Akt pathways commonly involved in mechanotransduction, the upregulation of the Wnt pathway and inhibited endoplasmic reticulum stress-mediated apoptosis were observed for the viscous matrix with a low loss modulus. The high viscosity matrix showed additional involvement of Hippo and NF-kappa B signaling pathways related to the cell–matrix interaction, with downregulation of the endoplasmic reticulum stress pathway and upregulation related to mitochondrial organization. Our study would provide insight into the effect of viscosity on fundamental behaviors of tumor cells and might have important implications in designing antitumor materials.
1. Introduction
Cells sense mechanical cues in the actual physiological environment through joint/adhesion to other cells/extracellular matrix.1–3 It has been widely reported that the elastic modulus of the matrix profoundly affects the behavior of various cell types, including tumor cells.4–6 Studies have shown that the soft matrix with a low storage modulus could inhibit the adhesion of tumor cells to the matrix and the organization of the actin cytoskeleton and promote cell apoptosis, whereas the hard matrix with a high storage modulus promoted the spreading, survival and malignant phenotype of tumor cells by providing enough stress for cell pulling.7–14 Taking advantage of the regulating effect of physical cues, researchers also developed anti-cancer materials to assist tumor treatment.15
Besides the elastic component, the viscous component is another critical factor that would affect mechanotransduction and cell behavior. In fact, in the actual physiological environment, the extracellular matrix is not purely elastic, but also has a certain degree of viscosity, that is, the so-called viscoelasticity.16,17 Some recent works have shown that matrices with viscosity could facilitate the spreading, migration, proliferation and survival of stem cells and normal cells by promoting/altering the cell adhesion/remodeling to the matrix.18–21 For example, matrices with viscosity could promote spreading and proliferation of fibroblasts and mesenchymal stem cells (MSCs) and osteogenic differentiation of MSCs by affecting adhesion-ligand binding, myosin contraction and mechanical aggregation of adhesion ligands.18,19 This enhancement of cell–matrix interaction also changed the cell area, nuclear area, focal adhesion area, focal adhesion tension and YAP/TAZ subcellular localization of human mesenchymal stem cells.20,21
The above studies were important for the work of damaged tissue repair and regeneration, but the research focusing on the effect of matrix viscosity on the behavior of tumor cells was still in a pioneering state. Recent studies have shown that human hepatoma Huh7 cells behave differently from normal human hepatocytes on the viscoelastic matrix.22 Specifically, on the viscoelastic matrix, the spreading area and motility of Huh7 cells were larger than those of normal human hepatocytes, and Huh7 cells had more long protrusions. The stress relaxation and creeping behaviors have also been reported to affect the spreading, migration and proliferation of a variety of normal cells and tumor cells.23–25
Moreover, most of the currently reported so-called viscoelastic matrices were in fact viscoplastic.26 The deformation of viscoplastic matrices upon stress was irreversible, which led to problems such as a localized concentration of adherent ligands, which made these matrices impossible to fully simulate the effect of the actual extracellular matrix on cell behavior.26 Studies have shown that the focal adhesion of 3t3 cells on the viscoelastic matrix was less than that on the viscoplastic matrix, although the cells spread well on both matrices.21,26
Therefore, based on previous studies, we prepared a viscoelastic matrix with a storage modulus of about 2 kPa and a loss modulus controllable from 1 to 600 Pa at a relevant frequency of 1 rad s−1 for cell mechanosensing by adjusting the compactness of the covalent network of polyacrylamide and doping linear sodium alginate as the viscous component. In this work, we explored in detail the effects of the viscoelasticity matrix on the apoptosis, spreading, proliferation and cell–matrix interaction of tumor cells (osteosarcoma MG-63). Using mRNA sequencing technology, we tried to establish the relationship and roadmap between the cell–matrix interaction, actin cytoskeleton organization, signaling pathway and cell behavior mediated by the viscoelastic matrix for MG-63 cells. This work would augment the understanding of the potential mechanism of the regulating effect of the viscoelastic matrix on the behaviors of tumor cells and provide insight for designing anti-tumor materials.
2. Materials and methods
2.1. Preparation of PAM/Alg/PDA gels
First, 40% acrylamide, 2% bis-acrylamide, 5% alginate, UP H2O, tetramethylethylenediamine (TEMED) and 10% ammonia persulfate (APS) solutions were mixed thoroughly in the proportions and order corresponding to those in Table S1, ESI.† Then, 3 ml of the mixture was transferred to a 6-well plate and set for 4 h to allow the reaction to be fully carried out. After 4 h, the samples were placed under UV light overnight to obtain a sterile gel. Then, the gels were immersed in a filtered dopamine solution and shaken on a shaker for 3 h to improve the cell adhesion properties of the gel surface. The concentration of the dopamine solution was 2 mg ml−1 and the solvent was Tris buffer with a pH value of 8.5. Finally, the gels were stored in a sterile and moist environment. In addition, all raw materials were from Sigma. All raw materials were autoclaved and sterilized using a needle filter or UV-sterilized before use.
2.2. Rheological characterization and surface topography characterization
The rheological properties of the gels were measured using a modular intelligent optical rotational rheometer (Anton Paar, MCR302). The mixed solution of 1 ml was deposited on the measuring platform of the rheometer, and the measuring rotor was lowered to form a solution plate with a diameter of 25 mm and a thickness of 2 mm, and the excess liquid was aspirated. Then, the rheological properties of the gels were measured by the previously published method,27,28 and the temperature was set at 37 °C. The frequency used for measuring the gel formation time was 1 rad s−1. The storage and loss modulus of the gel were measured at a shear strain of 0.5%. The shear strain used to perform stress relaxation was 10%. Creep generation tests were performed by giving a shear stress of 200 Pa.
After freeze-drying for 48 h, the surface of the gel was sputtered with gold and examined by SEM (S8400, Hitachi Ltd, Tokyo, Japan).
2.3. Cell culture
MG-63 human osteosarcoma cells (Cell Bank of Type Culture Collection of Chinese Academy of Sciences, Shanghai, China) were cultured in α-MEM medium (Gibco) with 10% fetal bovine serum (FBS, Gibco) and 1% penicillin–streptomycin antibiotics (Gibco) under conventional cell culture conditions. When MG-63 cells were cultured to a sub-confluent state, the cells were used for passaging and seeding. Moreover, unless otherwise specified below, cells were seeded at a density of 2 × 104 cells per cm2 and the culture time was 2 days.
2.4. Flow cytometry and in situ fluorescence assay
The apoptosis rate of MG-63 cells was examined by flow cytometry (BD, LSRfortessa) and in situ fluorescence assay using annexin V-FITC/PI double-staining assay kit instructions (BD Bioscience). The 10 minutes of staining with annexin V-FITC was followed by 5 minutes of staining with propidium iodide (PI). After this, flow cytometry was used for flow analysis and confocal microscopy (Carl Zeiss, LSM880 Airyscan with STEDYCON) was used for in situ fluorescence assay.
2.5. Cell morphology assay and cell proliferation assay
Fluorescein diacetate (FDA, Sigma) was used for fluorescence staining of MG-63 cells, and the morphology of the cells was observed and photographed under an inverted microscope (Leica, DMI4000B). In addition, the morphology of MG-63 cells at 8, 24 and 48 h was observed and obtained using the bright field mode of the inverted microscope and the morphological parameters of the cells were quantified using the ImageJ software. The formula “circularity = (4π × S)/C2” was used to calculate the circularity and the formula “aspect ratio = MaxFeret/MinFeret” was used to calculate the aspect ratio.
The proliferation of MG-63 cells cultured on different viscoelastic matrices for two days was evaluated using a cell counting kit-8 (cck8, Sigma) solution according to the instructions for use, and the absorbance (OD value) was tested using an enzyme marker (BIOTEK, Synergy H1) with an excitation light wavelength of 450 nm.
2.6. Cytoskeleton staining
After 4% paraformaldehyde (Sigma) fixation for 15 min, the cytoskeletons and nuclei of MG-63 cells were stained using phalloidin (Sigma) for 40 min and 4,5-diamidino-2-phenylindole (DAPI, Sigma) for 10 min, respectively. Washing with PBS was performed 3 times between each operation. Afterwards, a confocal microscope was used to observe and record the cytoskeletal state of MG-63 cells.
2.7. Immunofluorescence staining
The primary antibodies used were the anti-integrin β1 recombinant rabbit monoclonal antibody and anti-vinculin recombinant rabbit monoclonal antibody, respectively, and the secondary antibodies were the iFluor™ 488 conjugated goat anti-rabbit IgG goat polyclonal antibody, both from Huabio Technology, Ltd (Hangzhou, China). The primary antibodies were fixed with 4% paraformaldehyde, permeabilized with 0.1% Triton X-100 (Sigma) and sealed with 4% bovine serum albumin (BSA, Sigma), with PBS as the solvent. The primary antibodies were diluted 1
:
200 and secondary antibodies were diluted 1
:
750, with 4% BSA as the solvent. Complete protection from light was required when cells were incubated with secondary antibodies. Finally, observation and photography were performed under a confocal microscope.
2.8. mRNA sequencing and analysis
After being cultured on different viscoelastic matrices for 2 days, MG-63 cells were digested with trypsin without ethylene diamine tetraacetic acid (EDTA). The 3 × 105 digested cells were collected and centrifuged at low speed for 5 min. 1 ml of Trizol was added to each sample to protect the mRNA of the cells. After this, the samples were placed in liquid nitrogen for 10 min. Then, the samples were quickly transferred to a refrigerator at −80 °C for storage. Finally, these samples were sequenced to obtain the expression of mRNA on different viscoelastic matrices at Genergy Bio-Technology, Ltd (Shanghai, China). The differential expression analysis was obtained through online website processing (https://metascape.org, https://www.kegg.jp, https://www.bioinformatics.com.cn and https://cn.string-db.org).
2.9. Statistical analysis
The data were expressed as mean ± standard deviation. Three replicates were used in the experiments. Statistical analysis was performed using the T-test: paired two-sample mean analysis for groups between two and using one-way ANOVA for groups more than two, and a P value < 0.05 was considered statistically significant for the results. Statistically similar data sets were represented by “ns”. (*p < 0.05, **p < 0.01 and ***p < 0.001).
3. Results
3.1. Preparation of tunable viscoelastic matrices by a simple synthetic method
By adjusting the ratio of acrylamide, bis-acrylamide and linear alginate to regulate the tightness of the polyacrylamide covalent network and the proportion of viscous components (Fig. 1(A)), we prepared a viscoelastic matrix with adjustable storage modulus (G′) and loss modulus (G′′), respectively. The G′ and G′′ of different viscoelastic matrices simultaneously increased with time at the same time, and tended to be stable at 1200 s (Fig. 1(B)). In addition, the G′ and G′′ of different viscoelastic matrices also increased gradually with the increase of frequency (Fig. 1(C)). At a frequency of 1 rad s−1, the storage modulus of these viscoelastic matrixes was about 2 kPa, and the loss moduli were 1, 100, 200 and 600 Pa, respectively (Fig. 1(D)). Hereafter, these viscoelastic matrices would be denoted as LM 1, LM 100, LM 200, and LM 600, respectively. Except for the LM 1 matrix, the stress relaxation test of these viscoelastic matrixes showed typical viscoelastic solid characteristics, and the stress gradually decreased to a platform value with the extension of time (Fig. 1(E)). For LM 100 and LM 200 matrices, it took about 10 s to reach this plateau, while for the LM 600 matrix it took about 100 s. The LM 1 matrix showed typical elastic matrix characteristics, and the stress did not change with time. The LM 600 matrix was selected as a typical example for creep testing. The LM 600 matrix exhibited obvious viscous creep and the deformation of the matrix returned completely after the stress was withdrawn (Fig. 1(F)), indicating viscoelastic rather than viscoplastic behavior. The SEM observations showed that the incorporation of viscous components increased the complexity of the matrix surface, and the porosity and pore size of the matrix surface increased with the increase of viscoelasticity (Fig. 1(G)). In addition, the surface of the matrices turned dark brown (Fig. S1(A), ESI†), as a result of surface modification with polydopamine (Fig. 1(G)).
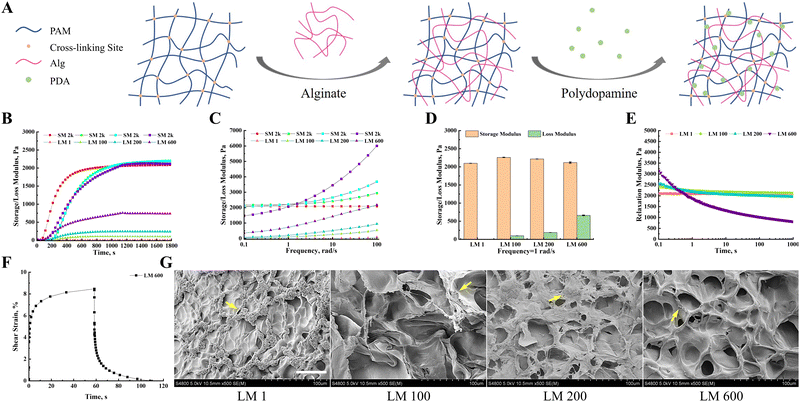 |
| Fig. 1 Preparation and characterization of the viscoelastic matrix. (A) Schematic diagrams of the viscoelastic matrix composed of slipperable linear alginate and a recoverable polyacrylamide elastic network. Cell adhesion sites were provided by polydopamine granules. (B) Evolution of the storage modulus (G′) and loss modulus (G′′) with time during the construction of the viscoelastic matrix. (C) Evolution of G′ and G′′ with frequency after the successful construction of the viscoelastic matrix. (D) The G′ and G′′ of the viscoelastic matrix at a cell sensing frequency of 1 rad s−1. (E) Stress relaxation tests for different viscoelastic matrices. (F) Creep test for the typical viscoelastic matrix LM600. (G) SEM was used to observe the surface morphology of viscoelastic matrices and polydopamine particle layers. The yellow arrows showed the polydopamine particles deposited on the surface of viscoelastic matrices. The scale bar was 50 μm. | |
3.2. The introduction of the viscous component increased the spreading and proliferation of MG-63 cells and decreased the apoptosis of MG-63 cells, but this trend decreased with the increase of the viscous fraction
The apoptosis results showed that the introduction of the viscous component overall reduced the apoptosis rate of MG-63 cells (Fig. 2(A)–(C)). For instance, the total apoptosis rate decreased from 27.14% (LM 1) to 14.94% (LM 100). Interestingly, with the increase of the viscous fraction, the apoptosis of MG-63 cells would gradually increase, for both early apoptosis and late apoptosis (Fig. 2(A)). A similar trend was observed in the in situ fluorescence assay, and the apoptosis rate of MG-63 cells decreased with the introduction of the viscous component (Fig. 2(C)), but increased gradually with the increase of the viscous fraction (Fig. 2(B)). In addition, the MTT assay showed that the viscoelastic matrixes were non-cytotoxic, with the average relative proliferation rate of MG-63 cells cultured on different viscoelastic matrixes and in different proportions of extracts was more than 80% (Fig. S1, ESI†).
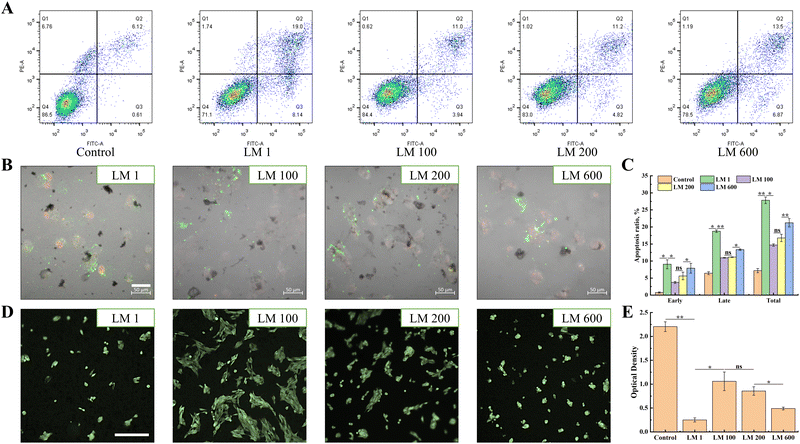 |
| Fig. 2 Effects of matrix viscoelasticity on the apoptosis, spreading and proliferation of MG-63 cells. (A) Flow cytometry and (B) in situ fluorescence assay were used to analyze the effects of matrix viscoelasticity on the apoptosis of MG-63 cells. The scale bar was 50 μm. (C) Quantitative analysis of flow cytometry results. (D) FDA assay and (E) cck8 assay were used to analyze the effect of matrix viscoelasticity on the spreading and proliferation of MG-63 cells. The scale bar was 250 μm. | |
Then, the FDA assay showed that MG-63 cells overall had better spread after the introduction of the viscous component (Fig. 2(D)). Moreover, the spreading of MG-63 cells gradually decreased with increasing matrix viscosity, with the LM 100 matrix showing the best spreading. It was worth noting that MG-63 cells grew and spread in aggregates on the viscoelastic matrix, but the degree of aggregation decreased with the increase of matrix viscosity. In addition, the proliferation of MG-63 cells showed the similar trend as spreading (Fig. 2(E)).
3.3. The spreading ability of MG-63 cells decreased with the increase of matrix viscoelasticity
In order to further investigate the response of MG-63 cells to the viscoelastic matrix, we also analyzed the morphological changes of MG-63 cells on the viscoelastic matrix with time using an inverted fluorescence microscope. The spreading of MG-63 cells increased with the introduction of the viscous component, while such an enhancement effect decreased with the increase of the viscous fraction (Fig. 3(A)). The LM 100 matrix reached about 40% spreading after 48 h (Fig. 3(B)), but very minimal spreading was observed with the LM 200 and LM 600 samples.
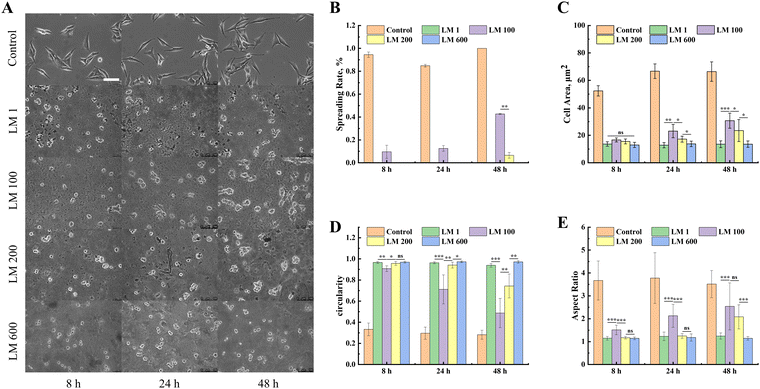 |
| Fig. 3 Evolution of MG-63 cell spreading with time on different viscoelastic matrices. (A) The spreading state of MG-63 cells was observed under an inverted fluorescence microscope at 0, 24 and 48 h. The scale bar was 100 μm. Quantitative analysis of the change of the (B) spreading rate, (C) cell area, (D) circularity and (E) aspect ratio of MG-63 cells on these matrices over time. | |
In order to quantify the spreading dynamics, we calculated the evolution of the cell area, circularity and aspect ratio of MG-63 cells over time. The introduction of the viscous component significantly improved the interaction between MG-63 cells and the matrix compared with the elastic matrix, while such a promotion effect decreased with the increase of the viscous fraction (Fig. 3(C)–(E)). Time also exerted certain influence on the spreading, in particular for the LM 100 and LM 200 samples. The quantitative statistics showed that the cell area measurements of MG-63 cells on all matrices were similar at 8 h, with a slightly lower circularity and higher aspect ratio for the LM 100 sample (Fig. 3(B)–(D)). However, the spreading ratio of MG-63 cells on the LM 100 matrix gradually increased from 9% to 42% as time increased from 8 h to 48 h (Fig. 3(E)), while the MG-63 cells maintained a low cell area, low aspect ratio and high roundness close to 1 on the LM 1 and LM 600 matrices, showing no improvements over the time.
3.4. The expressions of integrin β1 and vinculin increased and the organization of the actin cytoskeleton was altered in MG-63 cells with the increase of matrix viscoelasticity.
Fluorescence staining was further performed for integrin β1 and vinculin proteins that mediated the adhesion and spreading of MG-63 cells, as well as the actin cytoskeleton of the cells. In general, with the introduction of the viscous component and the increase of matrix viscoelasticity, the expressions of integrin β1 and vinculin in MG-63 cells showed small but gradual increases (Fig. 4(A)–(D)). Moreover, the moderate decrease of the vinculin expression was observed from 24 h to 48 h (Fig. 4(C) and (D)), while the expression of integrin β1 showed no significant difference in each group (Fig. 4(A) and (B)).
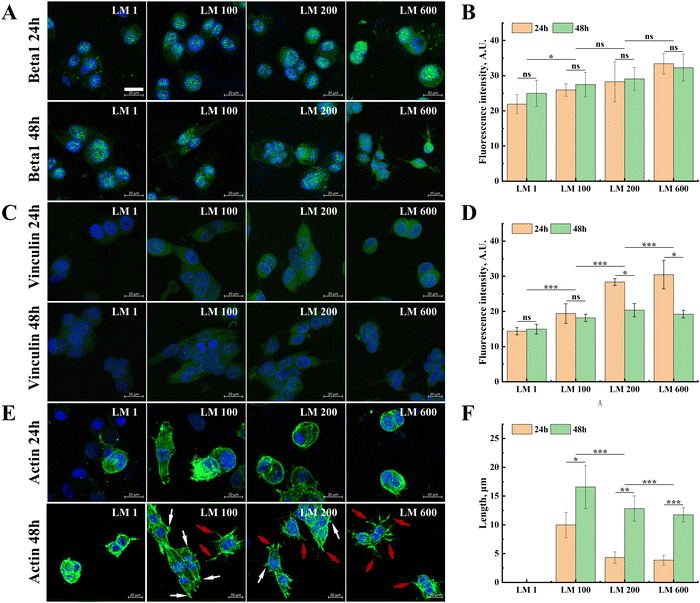 |
| Fig. 4 Effects of matrix viscoelasticity on the expression of integrin β1 and vinculin and cytoskeleton organization in MG-63 cells. (A) Staining and (B) quantitative analysis of integrin β1 expression in MG-63 cells on different viscoelastic substrates at 24 h and 48 h. (C) Staining and (D) quantitative analysis of vinculin expression in MG-63 cells on different viscoelastic substrates at 24 h and 48 h. (E) Cytoskeleton organization and (F) stress fiber length were affected by matrix viscoelasticity at 24 h and 48 h. The scale bar was 20 μm. | |
In contrast to the moderate modulatory effect of integrin β1 and vinculin, the introduction of the viscous component exerted a more profound effect on cytoskeleton organization (Fig. 4(E)). Compared with the elastic matrix LM 1, MG-63 cells on all viscoelastic matrixes have more developed stress fibers (Fig. 4(F)). It was also interesting to note that the fraction change of the viscous component seemed to lead to switching the manner of cytoskeleton organization, resulting in changes in the number and proportion of the focal adhesion (white) and pseudopodia (red), i.e. from fusiform cells dominated by focal adhesion to dendritic cells dominated by pseudopodia, despite that this change was well developed only at 48 h (Fig. 4(E)).
3.5. The mRNA sequencing analysis indicated that Ras/Rap1/PI3K-Akt/MAPK/Wnt pathways and NF-kappa B/Hippo pathways were involved in the regulation of the behavior of MG-63 cells on different viscoelastic matrices.
To understand the underlying mechanisms of how viscoelasticity affected cellular behaviors, we performed mRNA sequencing for MG-63 cells cultured on the plastic dish of the control group, the LM 1 matrix of the elastic group, the LM 100 matrix of the low viscosity group and the LM 600 matrix of the high viscosity group. Overall, we found a total of 404 genes with significant differences in expression related to the cell–matrix interaction among each group of samples in heat map analysis (Fig. S2A, ESI†).
First, the effect of the introduction of the viscous component was examined by comparing the LM 1 and LM 100 groups. GO analysis showed that those associated with the cell–matrix interaction were mostly upregulated in the biological process (BP), cellular component (CC) and molecular function (MF) in MG-63 cells on the LM 100 group (Fig. 5(A)). The processes intensively involved with the cell–matrix interaction and cytoskeleton organization, such as cell morphogenesis, cell adhesion, ameboidal-type cell migration, filopodium assembly and cytoskeletal protein binding, were upregulated. Meanwhile, those associated with endoplasmic reticulum stress were downregulated in BP and CC (Fig. 2(A), (C) and Fig. S2B, ESI†), which was consistent with the observed decreased apoptosis rate as a result of the introduction of the viscous component (Fig. 2).
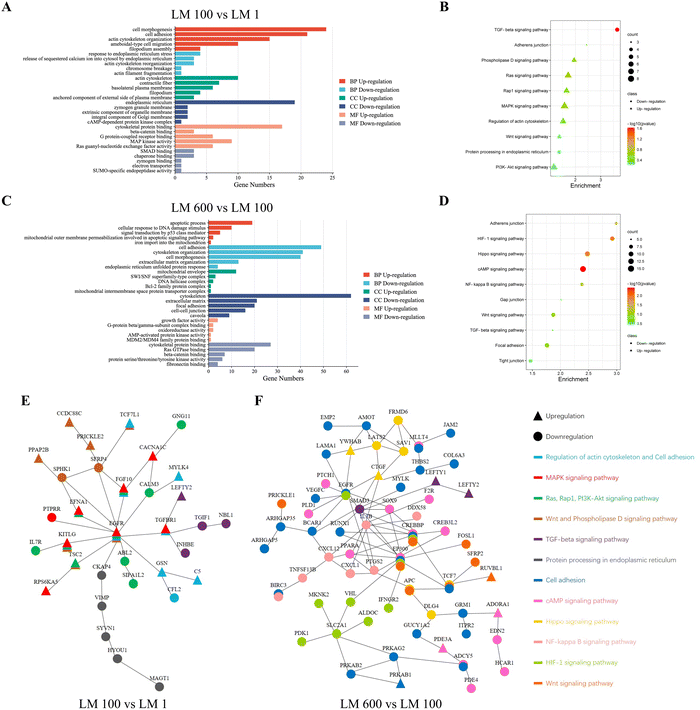 |
| Fig. 5 Regulation of cell adhesion and cytoskeleton organization by matrix viscoelasticity affected the apoptosis of MG-63 cells through the Ras/Rap1/PI3K-Akt/MAPK/Wnt pathway and NF-kappa B/Hippo pathway. Compared with the soft elastic matrix LM 1, (A) GO analysis showed that the cell–matrix interaction of MG-63 cells was upregulated while endoplasmic reticulum stress was downregulated, and (B) KEGG analysis showed that this change involved the Ras/Rap1/PI3K-Akt/MAPK/Wnt pathway on the low viscosity matrix LM 100. Compared with the low viscosity matrix LM 100, (C) GO analysis showed that the cell–matrix interaction of MG-63 cells was downregulated while the mitochondrial pathway was upregulated, and (D) KEGG analysis showed that this change also involved the NF-kappa B/Hippo pathway on the high viscosity matrix LM 600. (E) STRING analysis showed that the EGFR gene was the core gene when viscous components were introduced. (F) STRING analysis showed that MG-63 cells underwent a more complex regulation of signaling pathways with the increase of viscous components, in which EGFR, SMAD3, IL1B, CREBBP and EP300 were core genes. | |
Different trends were observed for the increase of the viscous fraction, as GO analysis indicated most downregulation in BP, CC and MF for the LM 600 matrix with high viscosity, compared to the LM 100 one with low viscosity. For instance, in contrast to the above observed trend with the LM 100 sample, those related to cell adhesion, cytoskeleton organization and extracellular matrix organization were downregulated in BP, CC and MF (Fig. 5(C)) for the LM 600 sample, i.e. with the further increase of the viscous fraction. On the other hand, for the LM 600 group, the processes associated with apoptosis and mitochondrial organization were upregulated in BP, CC and MF, which was consistent with the observed increased apoptosis rate with the increase of the viscous fraction (Fig. 2(A)–(C)).
Furthermore, KEGG analysis showed that Ras, Rap1, PI3K-Akt, MAPK and Wnt signaling pathways related to cell–matrix interaction were upregulated and protein processing in endoplasmic reticulum was downregulated in MG-63 cells on the viscoelastic matrix LM 100 compared to those on the LM 1 one (Fig. 5(B)). With the increase of the viscous fraction, the Hippo, Wnt and NF-kappa B signaling pathways related to cell–matrix interaction and HIF-1 and cAMP related to cell survival were downregulated in MG-63 cells on the LM 600 matrix compared to those on the LM 100 matrix (Fig. 5(D)). Moreover, it was noted that the TGF-beta signaling pathway was clearly involved, in particular for the LM 100 matrix, indicating the likely involvement of cytoskeleton organization and cell spreading (Fig. 5(B) and (D)).
Finally, through STRING analysis, it was inferred that the introduction of the viscous component affected the signaling pathway in two aspects (Fig. 5(E)). On the one hand, upregulation of cytoskeleton organization and cell adhesion would contribute to the upregulated MAPK, Ras, Rap1, PI3K-Akt and Wnt signaling pathways and downregulated endoplasmic reticulum stress, with a critical involvement of the EGFR gene (Fig. 5(E)). On the other hand, the TGF-beta signaling pathway was downregulated, due to likely strong meditation by the TGFBR1 gene (Fig. 5(E)). However, when the viscoelasticity of the matrix increased, changes in the cell–matrix interaction and the cytoskeleton organization of MG-63 cells seemed to lead to a much more complex signaling pathway or a protein-to-protein interaction network, correlated with the cell morphology and apoptosis, and the core of this network was the EGFR, SMAD3, IL1B, CREBBP and EP300 genes (Fig. 5(F)). This was consistent with the complex cell–matrix interaction and apoptosis pathways of MG-cells on the high viscosity matrix.
4. Discussion
By adding linear sodium alginate as a viscous component to the polyacrylamide elastic network, we obtained a viscoelastic matrix with adjustable elasticity and viscosity. The introduction of the viscous component allowed this matrix to take some time to reach a plateau value when subjected to mechanical force or deformation, while the presence of an elastic network allowed the matrix to recover from deformations due to exposure to mechanical forces within a certain period of time, as shown by creep and stress relaxation tests (Fig. 1(E) and (F)). This strategy prevented cells from widely aggregating adhesion ligands, unlike the viscoplastic matrix, which was more similar to the mechanical properties of the actual soft tissue.23
In this study, 2 kPa was selected as the elastic modulus since such a low storage modulus value would promote tumor cell apoptosis. The storage modulus of biological tissues is basically 2 kPa and above.17 In addition, the viscous fraction range of the PAM/Alg/PDA matrix covered a wide range. The LM 100, LM 200 and LM 600 matrices selected in this paper had the loss factor and viscoelasticity similar to those of lung tissue, liver tissue and brain tissue, respectively.17 These tissues are the most prone to tumor cell metastasis and colonization except for the bone tissue.29
In this work, the introduction of the viscous component overall increased the spreading, proliferation, adhesion and cytoskeleton organization of MG-63 cells, and decreased the apoptosis of MG-63 cells. Interestingly, it was noted that compared with other groups, MG-63 cells had more obvious spreading with time on the low viscosity matrix with a loss modulus of 100 Pa (Fig. 3), which meant that the tumor cells seemed to have better adaptability on the low viscosity matrix, which might be the result of the combination of viscoelastic properties such as loss modulus and stress relaxation caused by the introduction of the viscous component. The mRNA sequencing analysis showed that the introduction of the viscous component upregulated Ras, Rap1, PI3K-Akt, MAPK and Wnt signaling pathways by upregulating the cell–matrix interaction and cytoskeleton organization, and thus downregulated endoplasmic reticulum stress and apoptosis induced by the elastic matrix (G′ = 2 kPa, G′′ = 1 Pa). This is in agreement with the reports that the introduction of the viscous component and fast stress relaxation generally promoted the adhesion, spreading, survival and cytoskeleton organization of mesenchymal stem cells/normal cells.18,19,30,31 Moreover, in previous studies on the effects of the elastic matrix on tumor cells, Ras, Rap1 and PI3K-Akt signaling pathways were considered to be the main pathways affecting spreading, proliferation and apoptosis.32,33 For example, extracellular matrix elasticity could regulate the apoptosis of osteosarcoma cells through RAS signal cascade and mediate the malignant phenotype of normal breast epithelial cells.34,35 The observed additional involvement of MAPK and Wnt signaling pathways however suggest the somewhat different mechanism of mechanotransduction. The MAPK pathway was usually activated by upstream Rho family proteins and was involved in cytoskeletal organization and p53 protein regulation, and further affected DNA repair and apoptosis.36–38 The Wnt pathway is involved in many aspects of biologic processes including bone development and diseases39,40 and mechanotransduction41 and has been considered as an important target for cancer treatment.42
However, with the increase of the viscous fraction, the spreading, proliferation, adhesion and cytoskeleton organization of MG-63 cells gradually decreased, while apoptosis gradually increased, which was contrary to the previously reported effect of matrix viscoelasticity on the behavior of normal cells/mesenchymal stem cells, where a high loss modulus generally promoted the adhesion, spreading, survival and cytoskeleton organization.22,43,44 This suggested that tumor cells seemed to have a different response to the viscoelastic matrix than mesenchymal stem cells/normal cells. The mRNA sequencing analysis showed that the high viscosity matrix would downregulate Hippo, Wnt and NF-kappa B signaling pathways related to the cell–matrix interaction and HIF-1 and cAMP signaling pathways related to cell survival, compared with the low viscosity matrix, suggesting a potential link between the downregulation of the cell–matrix interaction and upregulation of apoptosis for MG-63 cells. Moreover, the increase of apoptosis of MG-63 cells seems to be related to the mitochondrial pathway, instead of the endoplasmic reticulum stress for the elastic matrix. For mesenchymal stem cells, the high viscoelasticity matrix has been reported to promote spreading, migration, and cytoskeleton organization through Rac1 and TGF-β signaling pathways.45,46 This suggested that the response of tumor cells to matrix viscoelasticity might be different from that of stem cells/normal cells, potentially affecting tumor development and metastasis processes.
Overall, the introduction of the viscous component exerted a more profound effect on cytoskeleton organization, exhibiting significantly more developed stress fibers for all viscoelastic matrixes (Fig. 4(E) and (F)). It was also interesting to note that the fraction change of the viscous component seemed to lead to switching the manner of cytoskeleton organization, i.e. from fusiform cells dominated by focal adhesion to dendritic cells dominated by pseudopodia, in particular at 48 h (Fig. 4(E)). In addition, it could be noted that the TGF-beta signaling pathway was downregulated on the low viscosity matrix, accompanied by good spreading and upregulation of cytoskeleton organization. The TGF-beta pathway is closely related to extracellular matrix organization, cell–matrix adhesion and cytoskeleton organization, and is activated by integrin-mediated adhesion.47,48 Usually in the late stage of tumor development, the TGF-beta pathway is upregulated and promotes tumor cell spreading, migration, EMT and invasion.49,50 The downregulation of the TGF-beta pathway and the upregulation of cytoskeleton organization on the low viscosity matrix was somewhat surprising, but the state of aggregation growth seemed to suggest the suppressed EMT and invasion and improved survival for the low viscosity sample, in contrast to the more invasive dendritic phenotype along with the higher apoptosis rate for the high viscosity sample. This might have important implications in migration-related invasiveness and malignancy of the tumor cells.
The summary of the effects of the introduction of the viscous component and the change of the viscous fraction on the cell–matrix interaction and apoptosis of tumor cells is illustrated in Fig. 6. Overall, the viscoelastic matrix with a low loss modulus reversed the inhibition of the elastic matrix on MG-63 cell adhesion and cytoskeleton organization by upregulating Ras, Rap1, PI3K-Akt, MAPK and Wnt signaling pathways, which promoted the spreading and proliferation of MG-63 cells, endowed MG-63 cells with fusiform shape, and inhibited endoplasmic reticulum stress and apoptosis of MG-63 cells (Fig. 6). On the other hand, the high viscosity matrix endowed MG-63 cells with the dendritic morphology by remodeling cell–matrix interactions, which downregulated cAMP, Hippo, HIF-1, Wnt and NF-kappa B signaling pathways, further inhibited cell spreading and proliferation and promoted mitochondrial pathway-dependent apoptosis, compared with the viscoelastic matrix with the low loss modulus.
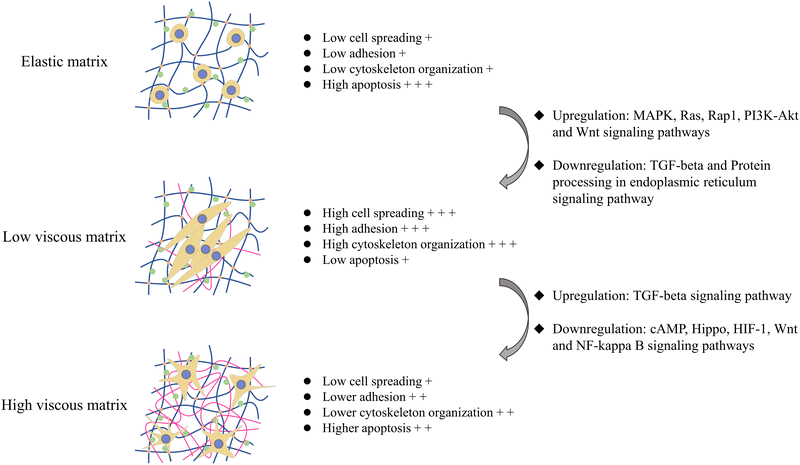 |
| Fig. 6 Schematic diagram of the effect of matrix viscoelasticity on MG-63 cells. MG-63 cells had low adhesion, low cytoskeleton organization, low cell spreading, low proliferation, high apoptosis, round cell morphology and dispersed growth on the elastic matrix. The introduction of viscous components promoted the cell–matrix interaction and cell survival by upregulating MAPK, Ras, Rap1, PI3K-Akt and Wnt signaling pathways and downregulating TGF-beta and protein processing in endoplasmic reticulum signaling pathways. However, MG-63 cells on the high viscosity matrix seemed to have less cell–matrix interaction and lose the survival advantage to some extent, showing dendritic morphology and downregulating cAMP, Hippo, HIF-1, Wnt and NF-kappa B signaling pathways and upregulating TGF-beta signaling pathway. The number of “+” indicated the degree of changes of different cellular responses, including cell spreading, adhesion, cytoskeletal organization, and apoptosis. | |
Overall, our study strongly suggested that the viscous properties also played a critical role in regulating the tumor cell responses in addition to the elastic properties of the matrix. Moreover, the fraction of the viscous component also played an important role in directing the cellular responses, in particular for the cytoskeleton organization and apoptosis. Our study would have implications in understanding the fundamental mechanisms of the regulating effect of viscosity on fundamental behaviors of tumor cells and optimal designing materials with antitumor efficacy.
5. Conclusions
In this work, we prepared a matrix with adjustable viscoelasticity and found that the osteosarcoma MG-63 cells on the viscoelastic matrix exhibited somewhat different behaviors from those on the elastic matrix and were also different from the normal cells/mesenchymal stem cells on the viscoelastic matrix. Compared with the soft elastic matrix, MG-63 cells had a large number of focal adhesion and pseudopodia on the low viscosity matrix, and obtained fusiform cell morphology, higher spreading, higher proliferation and lower apoptosis. However, with the increase of viscoelasticity of the matrix, the focal adhesion of MG-63 cells gradually disappeared, the pseudopodia became developed and the cell morphology became dendritic shaped, while cell spreading and proliferation decreased and apoptosis increased. The change of the cell–matrix interaction and cytoskeleton organization mediates the effects of the viscoelastic matrix on the spreading, proliferation and apoptosis of MG-63 cells. In addition to the Ras, Rap1, and PI3K-Akt signaling pathways that were commonly present in elasticity regulated effects on tumor cells, signaling pathways such as MAPK, Wnt, Hippo, TGF-beta and NF-kappa B played important roles in the viscoelastic matrix regulation of MG-63 cell spreading and apoptosis. In addition, the apoptosis of MG-63 cells on the high viscosity matrix seemed to be dominated by the mitochondrial pathway, compared with the endoplasmic reticulum stress pathway which was dependent on apoptosis induced by the soft elastic matrix. In general, our work would contribute to the design of materials that simulate extracellular matrix mechanical cues and improve the understanding of cell–matrix interaction and mechanical transduction in tumorigenesis and development.
Conflicts of interest
There are no conflicts of interest to declare.
Acknowledgements
This work was supported by the National Natural Science Foundation of China (no. 31971257 and 32271397) and the Natural Science Foundation of Sichuan Province (no. 2022NSFSC0360).
References
- D. E. Discher, P. Janmey and Y. L. Wang, Science, 2005, 310, 1139–1143 CrossRef CAS PubMed.
- S. H. Kim, J. Turnbull and S. Guimond, J. Endocrinol., 2011, 209, 139–151 CAS.
- C. T. Mierke, Rep. Prog. Phys., 2019, 82, 064602 CrossRef CAS PubMed.
- A. Miyazawa, S. Ito, S. Asano, I. Tanaka, M. Sato, M. Kondo and Y. Hasegawa, Biochem. Biophys. Res. Commun., 2018, 495, 2344–2349 CrossRef CAS PubMed.
- E. Y. Tokuda, J. L. Leight and K. S. Anseth, Biomaterials, 2014, 35, 4310–4318 CrossRef CAS PubMed.
- J. Y. Park, S. J. Yoo, E. J. Lee, D. H. Lee, J. Y. Kim and S. H. Lee, BioChip J., 2010, 4, 230–236 CrossRef CAS.
- A. J. McKenzie, S. R. Hicks, K. V. Svec, H. Naughton, Z. L. Edmunds and A. K. Howe, Sci. Rep., 2018, 8, 7228 CrossRef PubMed.
- Y. T. Peng, Z. Y. Chen, Y. C. He, P. Li, Y. Chen, X. Y. Chen, Y. Jiang, X. Qin, S. Li, T. T. Li, C. H. Wu, H. Yang, F. M. You and Y. Y. Liu, Cancer Lett., 2022, 524, 245–258 CrossRef CAS PubMed.
- R. W. Tilghman, C. R. Cowan, J. D. Mih, Y. Koryakina, D. Gioeli, J. K. Slack-Davis, B. R. Blackman, D. J. Tschumperlin and J. T. Parsons, PLoS One, 2010, 5, e12905 CrossRef PubMed.
- S. Prauzner-Bechcicki, J. Raczkowska, E. Madej, J. Pabijan, J. Lukes, J. Sepitka, J. Rysz, K. Awsiuk, A. Bernasik, A. Budkowski and M. Lekka, J. Mech. Behav. Biomed. Mater., 2015, 41, 13–22 CrossRef PubMed.
- A. Parandakh, A. Anbarlou, M. Tafazzoli-Shadpour, A. Ardeshirylajimi and M. M. Khani, Colloids Surf., B, 2019, 173, 194–201 CrossRef CAS PubMed.
- W. T. Chiu, Y. H. Wang, M. J. Tang and M. R. Shen, J. Cell. Physiol., 2007, 212, 401–410 CrossRef CAS PubMed.
- R. J. Jerrell and A. Parekh, Biomaterials, 2016, 84, 119–129 CrossRef CAS PubMed.
- R. S. Stowers, A. Shcherbina, J. Israeli, J. J. Gruber, J. L. Chang, S. Nam, A. Rabiee, M. N. Teruel, M. P. Snyder, A. Kundaje and O. Chaudhuri, Nat. Biomed. Eng., 2019, 3, 1009–1019 CrossRef PubMed.
- J. Y. Wei, J. Yao, M. C. Yan, Y. Xie, P. Y. Liu, Y. C. Mao and X. Li, Acta Biomater., 2022, 150, 34–47 CrossRef CAS PubMed.
- A. Elosegui-Artola, Curr. Opin. Cell Biol., 2021, 72, 10–18 CrossRef CAS PubMed.
- O. Chaudhuri, J. Cooper-White, P. A. Janmey, D. J. Mooney and V. B. Shenoy, Nature, 2020, 584, 535–546 CrossRef CAS PubMed.
- O. Chaudhuri, L. Gu, D. Klumpers, M. Darnell, S. A. Bencherif, J. C. Weaver, N. Huebsch, H. P. Lee, E. Lippens, G. N. Duda and D. J. Mooney, Nat. Mater., 2016, 15, 326–334 CrossRef CAS PubMed.
- S. Nam, R. Stowers, J. Z. Lou, Y. Xia and O. Chaudhuri, Biomaterials, 2019, 200, 15–24 CrossRef CAS PubMed.
- S. C. Tang, H. Ma, H. C. Tu, H. R. Wang, P. C. Lin and K. S. Anseth, Adv. Sci., 2018, 5, 1800638 CrossRef PubMed.
- I. A. Marozas, K. S. Anseth and J. J. Cooper-White, Biomaterials, 2019, 223, 119430 CrossRef CAS PubMed.
- K. Mandal, Z. Gong, A. Rylander, V. B. Shenoy and P. A. Janmey, Biomater. Sci., 2020, 8, 1316–1328 RSC.
- F. Y. Lin, C. Y. Chang, H. Nguyen, H. D. Li, M. L. Fishel and C. C. Lin, Mater. Today Bio, 2023, 19, 100576 CrossRef CAS PubMed.
- M. Najmina, M. Ebara, T. Ohmura and K. Uto, Int. J. Mol. Sci., 2022, 23, 14637 CrossRef CAS PubMed.
- D. L. Liu, H. Zhang, X. F. Dong, L. Sang and M. Qi, Front. Bioeng. Biotechnol., 2022, 10, 959409 CrossRef PubMed.
- E. E. Charrier, K. Pogoda, R. G. Wells and P. A. Janmey, Nat. Commun., 2018, 9, 449 CrossRef PubMed.
- N. Huebsch, P. R. Arany, A. S. Mao, A. S. Mao, D. Shvartsman, O. A. Ali, S. A. Bencherif, J. Rivera-Feliciano and D. J. Mooney, Nat. Mater., 2010, 9, 518–526 CrossRef CAS PubMed.
- X. H. Zhao, N. Huebsch, D. J. Mooney and Z. G. Suo, J. Appl. Phys., 2010, 107, 063509 CrossRef PubMed.
- S. E. S. Leary, A. W. Wozniak, C. A. Billups, J. R. Wu, V. McPherson, M. D. Neel, B. N. Rao and N. C. Daw, Cancer, 2013, 119, 2645–2653 CrossRef CAS PubMed.
- J. Z. Lou, R. Stowers, S. M. Nam, Y. Xia and O. Chaudhuri, Biomaterials, 2018, 154, 213–222 CrossRef CAS PubMed.
- S. C. Tang, H. Ma, H. C. Tu, H. R. Wang, P. C. Lin and K. S. Anseth, Adv. Sci., 2018, 5, 1800638 CrossRef PubMed.
- O. Chaudhuri, S. T. Koshy, C. B. da Cunha, J. W. Shin, C. S. Verbeke, K. H. Allison and D. J. Mooney, Nat. Mater., 2014, 13, 970–978 CrossRef CAS PubMed.
- H. H. Lin, H. K. Lin, I. H. Lin, Y. W. Chiou, H. W. Chen, C. Y. Liu, H. I. C. Harn, W. T. Chiu, Y. K. Wang, M. R. Shen and M. J. Tang, Oncotarget, 2015, 6, 20946–20958 CrossRef PubMed.
- H. Deng, X. D. Shu, Y. Wang, J. W. Zhang, Y. Yin, F. Wu and J. He, Cell Biochem. Biophys., 2023, 81, 839–850 CrossRef CAS PubMed.
- O. Chaudhuri, S. T. Koshy, C. B. da Cunha, J. W. Shin, C. S. Verbeke, K. H. Allison and D. J. Mooney, Nat. Mater., 2014, 13, 970–978 CrossRef CAS PubMed.
- J. M. Kyriakis and J. Avruch, Physiol. Rev., 2001, 81, 807–869 CrossRef CAS PubMed.
- J. M. Salvador, J. D. Brown-Clay and A. J. Fornace, Gadd45 stress sensor genes, Adv. Exp. Med. Biol., 2013, 793, 1–19 CrossRef CAS PubMed.
- J. C. Yue and J. M. Lopez, Int. J. Mol. Sci., 2020, 21, 2346 CrossRef CAS PubMed.
- J. J. Guan, J. Y. Zhang, S. C. Guo, H. Y. Zhu, Z. Z. Zhu, H. Y. Li, Y. Wang, C. Q. Zhang and J. Chang, Biomaterials, 2015, 55, 1–11 CrossRef CAS PubMed.
- H. Clevers and R. Nusse, Cell, 2012, 149, 1192–1205 CrossRef CAS PubMed.
- L. F. Bonewald and M. L. Johnson, Bone, 2008, 42, 606–615 CrossRef CAS PubMed.
- R. Nusse and H. Clevers, Cell, 2017, 169, 985–999 CrossRef CAS PubMed.
- A. R. Cameron, J. E. Frith and J. J. Cooper-White, Biomaterials, 2011, 32, 5979–5993 CrossRef CAS PubMed.
- A. R. Cameron, J. E. Frith, G. A. Gomez, A. S. Yap and J. J. Cooper-White, Biomaterials, 2014, 35, 1857–1868 CrossRef CAS PubMed.
- O. Chaudhuri, L. Gu, D. Klumpers, M. Darnell, S. A. Bencherif, J. C. Weaver, N. Huebsch, H. P. Lee, E. Lippens, G. N. Duda and D. J. Mooney, Nat. Mater., 2016, 15, 326–334 CrossRef CAS PubMed.
- A. R. Cameron, J. E. Frith and J. J. Cooper-White, Biomaterials, 2011, 32, 5979–5993 CrossRef CAS PubMed.
- R. Samarakoon, M. Goppelt-Struebe and P. J. Higgins, Cell. Signalling, 2010, 22, 1413–1419 CrossRef CAS PubMed.
- S. Assinder and N. Cole, Med. Hypotheses, 2011, 76, 802–804 CrossRef CAS PubMed.
- D. R. Principe, J. A. Doll, J. Bauer, B. Jung, H. G. Munshi, L. Bartholin, B. Pasche, C. Lee and P. J. Grippo, J. Natl. Cancer Inst., 2014, 106, djt369 CrossRef PubMed.
- I. Fabregat, J. Moreno-Caceres, A. Sanchez, S. Dooley, B. Dewidar, G. Giannelli and P. ten Dijke, FEBS J., 2016, 283, 2219–2232 CrossRef CAS PubMed.
|
This journal is © The Royal Society of Chemistry 2024 |
Click here to see how this site uses Cookies. View our privacy policy here.