CaO2–Cu2O micromotors accelerate infected wound healing through antibacterial functions, hemostasis, improved cell migration, and inflammatory regulation†
Received
8th October 2023
, Accepted 30th November 2023
First published on 1st December 2023
Abstract
During the wound tissue healing process, the relatively weak driving forces of tissue barriers and concentration gradients lead to a slow and inefficient penetration of bioactive substances into the wound area, consequently showing an impact on the effectiveness of deep wound healing. To overcome these challenges, we constructed biocompatible CaO2–Cu2O “micromotors”. These micromotors reacted with the fluids at the wound site, releasing oxygen bubbles and propelling particles deep into the wound tissue. In vitro experimental results revealed that these micromotors not only exhibited antibacterial and hemostatic functions but also facilitated the migration of dermal fibroblasts and vascular endothelial cells, while modulating the inflammatory microenvironment. A methicillin-resistant Staphylococcus aureus infected full-thickness-wound model was created in rats, in which CaO2–Cu2O micromotors markedly expedited the wound healing process. Specifically, CaO2–Cu2O provided a sterile microenvironment for wounds and increased the amounts of M1-type macrophages during infection and inflammation. During the proliferation and remodeling stages, the amount of M1 macrophages gradually decreased, while the amount of M2 macrophages increased, and CaO2–Cu2O did not prolong the inflammatory period. Furthermore, the introduction of a regenerated silk fibroin (RSF) film on the wound surface successfully enhanced the therapeutic effects of CaO2–Cu2O against the infected wound. The combined application of oxygen-producing CaO2–Cu2O micromotors and a RSF film demonstrates significant therapeutic potential and emerges as a promising candidate for the treatment of infected wounds.
1. Introduction
Skin is the vital external covering of the human body,1 and although damaged skin can recover, the healing process can be retarded by pathogen infection and external forces.2,3 In recent years, various types of wound dressings containing bioactive molecules have been exploited for accelerating skin regeneration.4 The idea is that therapeutic compounds would diffuse from the dressing into the wound site. However, their diffusion into deeply damaged tissues is still limited because the tissue acts as a barrier, and the driving force, which depends only on the concentration gradient, is too weak. Thus, the activity of these bioactive molecules has mainly been confined to the superficial epidermal layer of a wound.5–7 For deep wounds, the therapeutic effects of traditional wound dressings are far from satisfactory in preventing pathogen invasion and promoting tissue regeneration.6,8–10
Artificial ‘motors’ have been designed to convert an internal or external state, such as acidic pH, a thermal gradient, or a magnetic field, into mechanical motion that can produce a reaction like deep tissue penetration. One type of motor utilizes the propulsive force of a constantly generated gas, such as O2, NO, or CO2, which makes it independent of an external force to produce the motion. We have recently synthesized mesoporous manganese oxide nanoparticles, which could decompose H2O2 into O2 and propel drug-loaded nanoparticles deep into colon tumor tissues.11 We hypothesized that the strong resistance of wound tissues to particle penetration might be overcome by gas bubbles generated from chemical reactions that also serve as the driving force for rapidly deploying therapeutic substances into deep wounds.12 It is known that CaO2 particles can rapidly react with water to produce large amounts of O2 bubbles, and the released Ca2+ can accelerate the blood clotting system to promote hemostasis.13,14 Cu+ has been reported to inhibit bacterial growth and accelerate angiogenesis to promote tissue regeneration.15–17 When combined with a ‘gas-powered’ micromotor, the metal complex is expected to be a promising therapeutic platform for healing infected wounds. Presently, for the treatment of deep wounds, numerous recently developed antibacterial particles, including nanoenzymes and a combination of photothermal therapy and simulated enzyme catalysis, exhibit outstanding antibacterial effectiveness and tissue repair capabilities.18–20 In contrast to certain metallic or precious metal nanoenzymes, the preparation process for CaO2–Cu2O does not necessitate any specialized equipment, resulting in lower costs while still achieving desirable therapeutic effects.
In the present study, we synthesized composite CaO2–Cu2O micromotors by a sol–gel method (Fig. 1). These micromotors rapidly reacted with the tissue fluid to release O2 bubbles, effectively impelling the particles into the deeper layers of damaged skin. The metal ions released from the CaO2–Cu2O microparticles exerted their bioactivities to kill bacteria, modulate macrophage polarization, facilitate collagen generation, promote angiogenesis, and accelerate cell migration, which accelerated the healing process in the deeply infected wound. With the aid of this novel delivery platform, the functional metal ions can heal both the epidermis and the underlying dermis. As an additional benefit, regenerated silk fibroin (RSF) films were applied to cover the wound for protection. A synergistic effect was observed in wound healing by promoting collagen deposition and inducing angiogenesis.
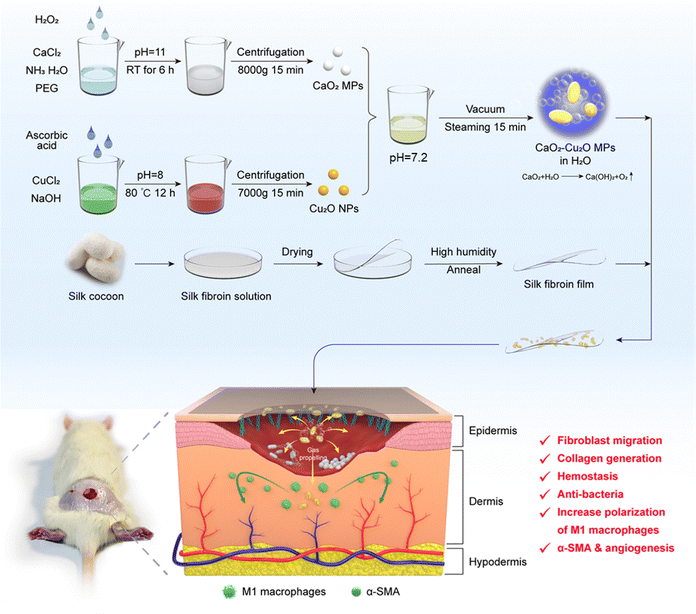 |
| Fig. 1 Illustration of the synthesis of CaO2–Cu2O micromotors with gas-propulsion ability and synergy between CaO2–Cu2O micromotors and the RSF film on an infected wound. CaO2–Cu2O micromotors can permeate the tissue and accelerate the healing process of an infected wound by promoting hemostasis and fibroblast migration, killing bacteria, maintaining macrophage polarization, facilitating collagen generation, and stimulating angiogenesis. | |
2. Materials and methods
2.1. Materials
3-(4,5-Dimethyl-2-thiazolyl)-2,5-diphenyl-2H-tetrazolium bromide (MTT) and diamidino-2-phenylindole (DAPI) were supplied by Invitrogen (Eugene, USA). Lipopolysaccharide (LPS) was obtained from Sigma-Aldrich (St. Louis, USA). Bovine serum albumin (BSA) and Triton X-100 were supplied by Aladdin (Shanghai, China). Silkworm cocoons were provided by the State Key Laboratory of Resource Insects (Southwest University, China). Hematoxylin–eosin (H&E) staining kit was bought from Solarbio (Beijing, China). Mannose Receptor C Type 1 (MRC-1) rabbit polyclonal antibodies and FITC-labeled goat anti-rabbit IgG (H + L) were supplied by Neobioscience Co. Ltd (Shenzhen, China). CuCl2 and CaCl2 were provided by General-reagent (Shanghai, China).
2.2. Synthesis of CaO2–Cu2O micromotors
2.2.1. Synthesis of CaO2.
CaCl2 (300 mg) was directly dissolved in double-distilled water (3 mL). Subsequently, PEG200 (12 mL) and NH3·H2O (2.5 mL, 25%) were added and stirred at room temperature for 5 h. Finally, the pH value of the mixture solution was adjusted to 11.5 to yield a CaO2 sol by adding a NaOH solution (40 mg mL−1). The CaO2 sol was obtained by centrifugation (8000g, 20 min), re-suspended with anhydrous ethanol and stored for future use.
2.2.2. Synthesis of Cu2O.
CuCl2 (34 mg) and ascorbic acid (440 mg) were simultaneously dissolved in 25 mL double-distilled water, respectively. The obtained CuCl2 solution was stirred at 80 °C for 10 min, and the ascorbic acid solution was slowly added to the CuCl2 solution. The mixture with a pH value of 8 (adjusted by adding 40 mg mL−1 NaOH solution) was incubated at 80 °C for 12 h. The Cu2O solution was centrifuged (7000g, 15 min) to obtain the Cu2O sol. The Cu2O solution was dialyzed and obtained by centrifugation (7000g, 10 min).
2.2.3. Synthesis of CaO2–Cu2O micromotors.
CaO2–Cu2O micromotors were synthesized by the blending of Cu2O particles and CaO2 particles in a mass ratio of 5/1. The final pH was 7.2 when CaO2 particles were combined with Cu2O particles under wet conditions. Initially, the prepared Cu2O solution was slowly added into the CaO2–ethanol suspension and stirred for 2 h. The CaO2–Cu2O micromotors were collected by centrifugation and re-suspended in anhydrous ethanol three times to wash residual organic solvents. The micromotors were obtained after vacuum spin steaming to remove the ethanol. The concentrations of CaO2 and Cu2O in micromotors were quantified by inductively coupled plasma mass spectrometry (ICP-MS, Agilent, Palo Alto, USA).
2.3.
In vitro phenotypic polarization of macrophages
RAW 264.7 macrophages were cultured in a 12-well plate (2 × 105 cells per well) for 12 h. Subsequently, the cells were induced by LPS for 12 h and co-cultured with different particle suspensions (50 μg mL−1, 500 μL) for 24 h. After fixation with paraformaldehyde and washing, the cells were successively incubated with primary rabbit polyclonal antibodies (iNOS and MRC-1, respectively) and goat anti-rabbit antibodies (FITC and Cy3, respectively). Meanwhile, cell nuclei were stained with DAPI. The stained macrophages were observed by super-resolution laser scanning confocal microscopy (SRLSCM, Olympus Corporation, Tokyo, Japan).
2.4. Antibacterial activity of CaO2–Cu2O micromotors
Antibacterial experiments were carried out with Escherichia coli (E. coli), Staphylococcus aureus (S. aureus), and methicillin resistant Staphylococcus aureus (MRSA). The bacterial solution (6 × 108 CFU mL−1, 100 μL) was added to 10 mL LB medium at 37 °C for 6 h on a shaker. Then, it was incubated with different concentrations of CaO2–Cu2O micromotors (0.2, 0.4, 0.8, 1.6, and 3.2 mg mL−1). The OD values (600 nm) were measured using a microplate reader (Tecan Shanghai, China), and the bactericidal rate can be calculated according to the following equation:
where An, A1, A2, and A3 represent the OD values of bacterial suspensions for bacteria with micro-particle treatment, bacteria without treatment, LB media with particles and blank LB media, respectively.
Bacterial live/dead staining experiments were performed by double staining with DAPI and PI. After co-incubation for 30 min, E. coli, S. aureus, and MRSA were observed by SRLSCM (Olympus Corporation, Tokyo, Japan). Besides, the bacteria were gathered, fixed, gradient dehydrated, dried, and subsequently imaged using a SEM and a transmission electron microscope (TEM, Hitachi, Tokyo, Japan).
Simultaneously, the antibacterial effects of CaO2, Cu2O, and CaO2–Cu2O were assessed and compared by the plate dilution method. Essentially, diverse particulate compounds were co-cultivated with bacteria (1 × 106 CFU mL−1). Following this, the mixture was suitably diluted, and 100 μL of the bacterial solution was uniformly applied to agar plates. Subsequently, the solution was incubated at 37 °C for 12 h. Ultimately, photographs of the resulting colony units were acquired for subsequent analyses.
2.5.
In vitro clotting ability of CaO2–Cu2O micromotors
To investigate the in vitro blood clotting time, different particle suspensions (1.6 mg mL−1, 100 μL) were added to consecutive wells of a 96-well plate containing 50 μL blood. Each well was softly washed with PBS at predetermined time points to remove un-clotted blood (1, 2, 3, 4, 5, 6, 7 and 8 min). The time to form a homogeneous and stable clot in the wells was defined as the clotting time.
2.6.
In vivo wound healing assay
Female SD rats (260–300 g) were anesthetized and a full-thickness-wound model (diameter = 10 mm) was established on the back by a punch. Then, an MRSA suspension (2 × 108 CFU mL−1, 200 μL) was intramuscularly injected into the wound to construct an infected model. CaO2 particles, Cu2O particles, and CaO2–Cu2O micromotors were applied to treat the infected wound. Additionally, a group of rats were synergistically treated with CaO2–Cu2O micromotors and an RSF film. A digital camera recorded the wound area on days 0, 3, 7, and 14. The rats without treatment were defined as the control group. The wound closure percentage was obtained from the wound traces. On predetermined time points, the adjacent skins in the wound site and viscera organs were harvested and sectioned for staining to evaluate the therapy and biosafety further. The in vivo experiments were conducted in accordance with the ethical principles outlined by the Southwest University Animal Ethics Committee and the corresponding animal ethics licence was obtained (IACUC-20230512-11).
2.7.
In vivo distribution of CaO2–Cu2O micromotors
A full-thickness-wound model (diameter = 10 mm) was established on the back of BALB/c female mice (6–8 weeks old), and CaO2–Cu2O micromotors conjugated with coumarin (100 μL, 1.6 mg mL−1) were added to the wound. The tissue on the wound site was harvested after incubation for 2 h and subsequently sectioned to evaluate the distribution of particles. PS microspheres conjugated with rhodamine were set as the control group.
2.8. Statistical analysis
Data analysis was conducted using the Origin 8.0 software. Data are presented as mean ± standard error of the mean (S.E.M.). Student's t-test was applied to analyze the significant differences. The significant differences were presented as *p < 0.05, **p < 0.01, ***p < 0.001, and ns = no significance.
3. Results and discussion
3.1. Preparation and physicochemical characterization of CaO2–Cu2O micromotors
The size and stability of the CaO2–Cu2O micromotors are critical characteristics, as they affect the particle permeability and, consequently, the therapeutic effectiveness in the wound area.21 Dynamic light scattering measurements were carried out to determine the diameter of the particles. As shown in Fig. 2A, the average hydrodynamic size of CaO2–Cu2O micromotors was 1.2 μm, and the polydispersity index was 0.111, confirming their uniform diameter. The changes in the diameter of particles over time were determined to evaluate their stability in water (Fig. S1, ESI†). The average hydrodynamic size of CaO2 particles, Cu2O particles, and CaO2–Cu2O micromotors in deionized water was 3.4, 0.4, and 1.2 μm, respectively. The diameter of the Cu2O particles and CaO2–Cu2O micromotors showed no significant variation over time, while that of CaO2 particles dramatically varied during incubation in deionized water for 8 h, suggesting that CaO2 particles were hydrolyzed in water. Thus, the introduction of Cu2O signally improved the stability of CaO2 in the complex. We speculate that the Cu2O nanoparticles were scattered on the surface of CaO2 particles, which probably reduced the contact area between CaO2 and water. Thus, the stability of Cu2O–CaO2 composite particles was increased to some extent. SEM assessed the substructure of the CaO2–Cu2O micromotors. It revealed an ovate shape with a length of 1.1 μm and a width of 0.5 μm (Fig. 2B). The elementary composition of micromotors was established using an energy-dispersive spectrometer, and the corresponding results (Fig. S2, ESI†) revealed that the CaO2–Cu2O micromotors contained calcium, copper, and oxygen, which verified their successful synthesis. It was obvious that the Cu2O particles were evenly scattered on the surface of CaO2. The crystalline state of these particles was assessed by X-ray diffraction (XRD). As shown in Fig. 2C, CaO2 particles had a characteristic peak attributed to the (002) lattice planes, and Cu2O particles had peaks belonging to the (110) and (200) lattice planes, which were in agreement with published data.22,23 All the characteristic peaks of CaO2 and Cu2O particles were observed in the spectrum of the CaO2–Cu2O micromotors, proving the complexation of CaO2 and Cu2O. Additionally, these broad lattice peaks suggested that the CaO2 and Cu2O structures in the microparticles were poorly crystallized. In view of the alterable valence state of copper, X-ray photoelectron spectroscopy (XPS) was performed to determine it. The full XPS spectrum also confirmed the existence of calcium, copper, and oxygen in the CaO2–Cu2O micromotors (Fig. S3, ESI†). The high-resolution spectrum of copper was deconvoluted to investigate the valence state (Fig. 2D). These results indicated that the Cu 2p spectrum contained three peaks for Cu+ and four peaks for Cu2+. By calculating the ratio of the peak areas, we determined that the ratio of Cu+ to Cu2+ was about 2/1, confirming that Cu+ was the central state of copper in the micromotors.
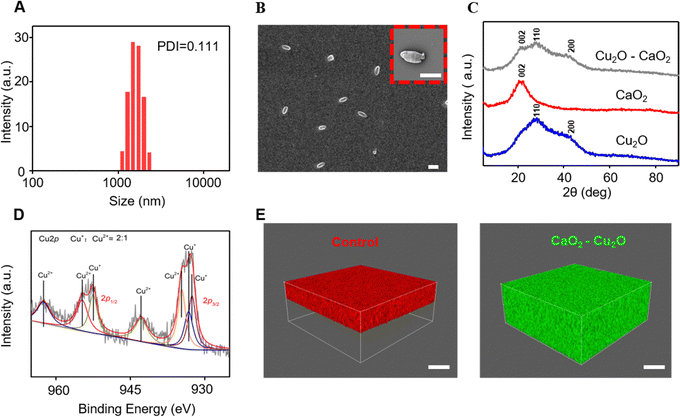 |
| Fig. 2 Characterization of CaO2–Cu2O micromotors. (A) Size distribution profiles. (B) SEM images; scale bar = 1 μm. (C) XRD spectra of Cu2O particles, CaO2 particles, and CaO2–Cu2O micromotors. (D) High-resolution XPS spectrum of Cu in CaO2–Cu2O micromotors with deconvolution. (E) and (F) Three-dimensional distribution of PS particles (red) and CaO2–Cu2O micromotors (green) in the simulated tissue fluid; scale bar = 10 μm. | |
Deep wound tissue is challenging to treat because of its poor accessibility to therapeutic compounds released from wound dressing, which usually results in skin loss and scar tissue formation.24–26 Therefore, bioactive substances with enhanced permeability to enter into the deeper wound tissue are crucial for healing. The CaO2–Cu2O micromotors react with H2O to release O2, and a train of O2 bubbles can be observed after dispersing the particles in water, as presented in Video S1 (ESI†). The oxygen bubbles can propel the micromotors deep into the damaged tissues. The oxygen production is directly linked to the calcium peroxide concentration. Controlling the oxygen levels involves precisely managing the CaO2 ratio in the composite particles. However, in terms of control, CaO2–Cu2O, being non-targeted, releases oxygen in all directions, lacking a specific directional movement. To verify this hypothesis, we designed a permeation experiment in which hydroxyethyl cellulose hydrogels were applied to simulate the tissue fluid with strong resistance to permeation. The polystyrene (PS) microspheres labeled with a red fluorophore were used as the control group. As shown in Fig. 2E, the PS were mainly distributed on top of the simulated tissue fluid. For comparison, CaO2–Cu2O micromotors labeled with a green fluorescent tag filled the whole simulated tissue fluid for several minutes (Fig. 2F). These results demonstrate that the micromotors can penetrate the deeper areas of the simulated tissue fluid with the assistance of oxygen bubbles.
3.2.
In vitro biocompatibility, cell migration and macrophage polarization
Fibroblasts, macrophages, and human vascular endothelial cells (HUVECs) are closely associated with tissue repair and have been extensively used in biosafety experiments.27,28 Therefore, we carried out MTT viability assays using L929 fibroblasts (Fig. 3A), RAW 264.7 macrophages (Fig. 3B), and HUVECs (Fig. 3C) to evaluate the biocompatibility of the different particles. The results inhibited that over a particle concentration range of 12.5 to 200 μg mL−1, the viabilities of L929 fibroblasts, RAW 264.7 macrophages, and HUVECs were consistently higher than 80%, verifying that these particles were all biocompatible.
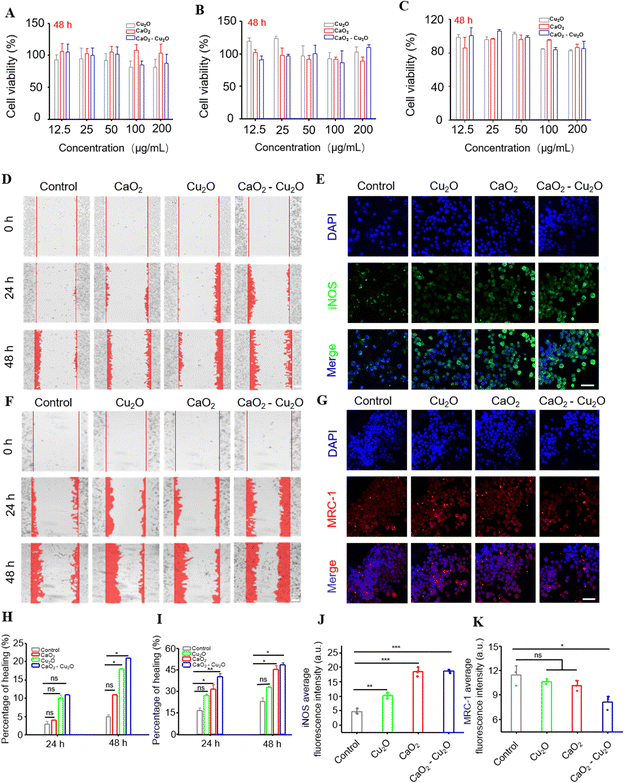 |
| Fig. 3 MTT assay of (A) L929 fibroblasts, (B) RAW 264.7 macrophages, and (C) HUVECs after incubation with different concentrations of Cu2O particles, CaO2 particles, and CaO2–Cu2O micromotors for 48 h. (D) Migration of L929 fibroblasts after incubation with Cu2O particles, CaO2 particles, or CaO2–Cu2O micromotors for 24 and 48 h; scale bar = 200 μm. (E) Immunostaining of iNOS (green) in RAW 264.7 macrophages after incubation with Cu2O particles, CaO2 particles, or CaO2–Cu2O micromotors for 24 and 48 h; scale bar = 100 μm. (F) Migration of HUVECs after incubation with Cu2O particles, CaO2 particles, or CaO2–Cu2O micromotors for 24 and 48 h; scale bar = 200 μm. (G) MRC-1 (red) in RAW 264.7 macrophages after incubation with Cu2O particles, CaO2 particles, and CaO2–Cu2O micromotors for 24 and 48 h; scale bar = 100 μm. (H) Quantitative analysis for migration of L929 fibroblasts. (I) Quantitative analysis for migration of HUVECs. (J and K) Corresponding quantitative analysis of mean fluorescence intensity (n = 3, ***p < 0.001, **p < 0.01, and *p < 0.05). | |
Multiple investigations have demonstrated that fibroblasts with faster migration rates are beneficial for wound healing.29,30In vitro cell monolayer scratch-test experiments were carried out to measure the migration of fibroblasts in the presence of different particles. The regrowth of ‘wounded’ areas was recorded over time to evaluate the migration of fibroblasts. Fig. 3D shows that after 24 h, the groups treated with Cu2O particles, CaO2 particles, and CaO2–Cu2O micromotors had more cells migrating into the scratched area than the control group. When the experimental time was prolonged to 48 h, a similar trend was observed. The corresponding semi-quantitative analysis (Fig. 3H) showed that the CaO2–Cu2O micromotors were most active in promoting fibroblast migration, followed by Cu2O particles and CaO2 particles, suggesting that Cu2O and CaO2 probably act synergistically. Angiogenesis is a key step in wound healing,31 and the efficient migration of HUVECs is necessary for angiogenesis in damaged tissue.32,33 Therefore, we also performed the cell-scratch experiment with HUVECs. The migration of HUVECs was significantly accelerated by all particle types tested at 24 or 48 h (Fig. 3F), but analysis (Fig. 3I) confirmed that the cells incubated with CaO2–Cu2O micromotors had the most cells in the scratched area.
Macrophages are innate immune cells that are essential to host defense, wound healing and immune regulation.34 Macrophages can be categorized into pro-inflammatory (M1) and anti-inflammatory (M2) phenotypes.35 In the early stages of wound healing, such as the inflammatory phase, the M1 macrophages not only phagocytose pathogens and clear the damaged tissue in the wound,36–39 but also initiate tissue regeneration and promote angiogenesis.40 Therefore, we evaluated the effect of the different particles on the phenotypes of macrophages in polarization experiments. The strongest green fluorescence intensity for iNOS, a marker of M1-type macrophages, was detected in the group treated with CaO2–Cu2O micromotors, followed by groups treated separately with CaO2 particles and Cu2O particles (Fig. 3E and J). Incubation with CaO2 particles, Cu2O particles, or CaO2–Cu2O micromotors weakened the red fluorescent signal of MRC-1, the marker of M2 phenotype macrophages (Fig. 3G and K). These results indicate that CaO2–Cu2O micromotors can enhance pathogen killing and remove damaged tissue from the wound.41
3.3. Antimicrobial activity and hemostasis
The wound area is vulnerable to pathogens because of the lack of skin protection.42 Therefore, antimicrobial activity is an essential requirement for an effective wound dressing. The SEM images (Fig. 4A) showed that the surface of E. coli, S. aureus, and MRSA was distinct and smooth in the control groups. The bacteria treated with CaO2–Cu2O micromotors had a fused cytoderm, indicating that they were killed. To further observe the influences of the micromotors on the bacterium, TEM was used to observe the bacteria (Fig. 4B). The bacteria in the control group were intact and uniform. In contrast, all three types of bacteria in the micromotor-treated group exhibited apparent leakage of cytoplasm. Considering the proven antibacterial activity of Cu+, this effect may be due to cytomembrane damage in the presence of CaO2–Cu2O micromotors. We also used live/dead staining to evaluate the antibacterial effect of CaO2–Cu2O micromotors. The control group showed predominantly blue fluorescence (Fig. 4C), indicating that most of the bacteria were alive, while bacteria treated with CaO2–Cu2O micromotors exhibited extensive red fluorescence, regardless of the type of bacteria, indicating massive mortality. The corresponding analysis of the live/dead staining data displayed in Fig. 4D showed that the fatality rates of bacteria incubated with CaO2–Cu2O micromotors reached 35.1% for E. coli, 69.2% for S. aureus, and 78.3% for MRSA, which were significant differences compared with the control group. We also investigated the dose dependence of the antibacterial activity of the CaO2–Cu2O micromotors, and Fig. 4E and F show that the survival rates of bacteria decreased with the increase in the concentration of CaO2–Cu2O micromotors regardless of the incubation time (12 or 24 h). Most bacteria can be killed with 0.8 mg mL−1 CaO2–Cu2O micromotors in 24 h (except for MRSA). When the concentration of micromotors increased to 1.6 mg mL−1, E. coli, S. aureus, and MRSA were killed entirely. Through extensive research on the sterilization efficiency of different particles, it was determined that the CaO2–Cu2O combination has the most effective sterilization (Fig. S4, ESI†). Furthermore, CaO2 also exhibited antibacterial properties to some extent, probably due to its moderate oxidability. Unexpectedly, there was a significantly different survival rate between S. aureus and MRSA when the concentration of CaO2–Cu2O was lower than 0.4 mg mL−1 (Fig. 4E). It seemed that, compared to S. aureus, the MRSA was more resistant to composite particles at low concentrations. This result might be attributed to the heterogeneity of surface charge and permeability of MRSA. These promising experimental data demonstrate that CaO2–Cu2O micromotors possess efficient antimicrobial activity that can protect wound fibroblasts from pathogens.
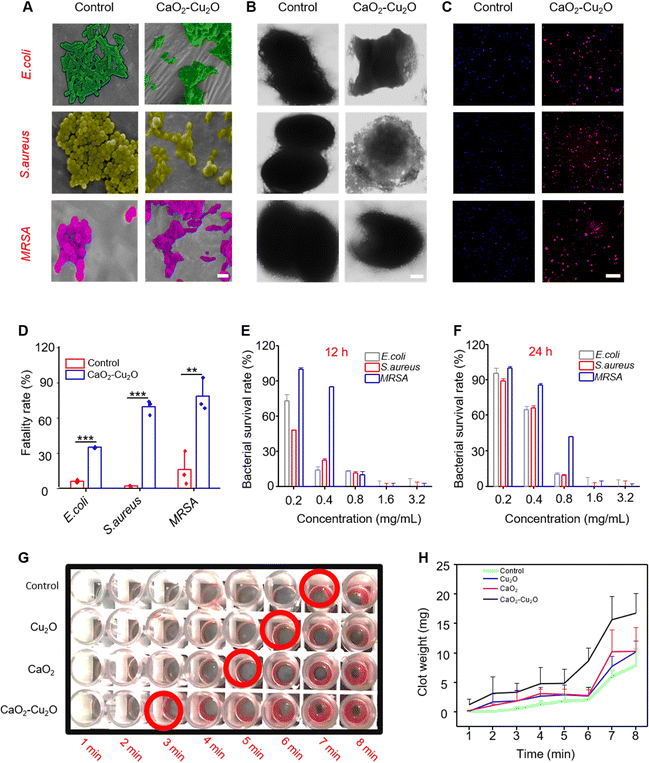 |
| Fig. 4 Antibacterial properties of CaO2–Cu2O micromotors. (A) SEM images of colonized E. coli (green), S. aureus (yellow), and MRSA (purple) with or without treatment with CaO2–Cu2O micromotors; scale bar = 2 μm. (B) TEM images of colonized E. coli, S. aureus, and MRSA with or without treatment with CaO2–Cu2O micromotors; scale bar = 400 nm. (C) Live (blue)/dead (red) staining of E. coli, S. aureus, and MRSA with or without treatment with CaO2–Cu2O micromotors; scale bar = 200 μm. (D) Quantitative analysis of bacterial live/dead stains. (E and F) CaO2–Cu2O micromotor dose dependence for antibacterial effect. (G) Images of clotting after the addition of microparticles. (H) Quantitative analysis of clotting weight (n = 3, ***p < 0.001, **p < 0.01, and *p < 0.05). | |
Hemostasis is the first stage of wound healing, and rapid hemostasis is essential for starting the following recovery phase. Many references reported that calcium ions activated platelets and were responsible for the activation of many blood coagulation factors.36,37,43,44 Thus, we evaluated the blood clotting effects of CaO2–Cu2O micromotors. The most rapid blood clotting was observed in the group treated with micromotors (3 min), followed by CaO2 particles (5 min) and Cu2O particles (6 min) (Fig. 4G). In contrast, the control group took the longest time (7 min) for the blood to clot. The weight of blood clots was determined as a function of time from 1 to 8 min (Fig. 4H), and the group treated with CaO2–Cu2O micromotors had the heaviest blood clots and, thus, the best capability for hemostasis.
3.4.
In vivo wound healing performance
The above-mentioned experiments confirmed that the biocompatible CaO2–Cu2O micromotors could permeate into a deep wound via oxygen propulsion, promote fibroblast and HUVEC migration, modulate macrophage polarization, and destroy pathogens. Therefore, CaO2–Cu2O micromotors theoretically could accelerate the recovery of a patient with a deep infected wound. To validate this, a rat model of the MRSA-infected full-thickness skin wound was used to evaluate the therapeutic effect. An injury with a diameter of 10 mm was produced on the dorsal skin, and the MRSA suspension was inoculated into the wound to establish an infection (Fig. 5A). Then, the wound area was treated with the different types of particles, and the healing rate was assessed during the following 14 days. The wound area was covered with an RSF film to protect it from external forces and air-borne pathogens. As reported, the RSF film had good biocompatibility and hydrophilicity, promoted adhesion of L929 fibroblasts, and was widely applied in tissue engineering and regenerative medicine.38,39 The uniformly prepared RSF film (Fig. S5, ESI†) possessed desirable transparency for the observation of wound recovery (Fig. S6, ESI†) and had good mechanical properties to protect the wound from an external force (Fig. S7, ESI†). Moreover, compared with 3M Transparent Dressing, the RSF film had better hydrophilicity (Fig. S8, ESI†) and promoted the adhesion of L929 fibroblasts (Fig. S9, ESI†). The RSF film was applied to the wound area of rats treated with CaO2–Cu2O micromotors, and these rats were termed the CaO2–Cu2O-RSF treated group. The wound areas were photographed on 0, 3, 7, and 14 days, and the healing rate was determined (Fig. 5B–D). At the early stage of wound healing (day 3), the healing rate of the control and the groups treated with Cu2O particles, CaO2 particles, CaO2–Cu2O micromotors, and CaO2–Cu2O-RSF was 31.2%, 35.5%, 48.2%, 56.3%, and 66.8%, subsequently increased to 72.4%, 73.6%, 80.5%, 81.8%, and 87.4% on day 7 (the proliferation stage), and finally reached 80.5%, 88.6%, 90.7%, 91.6%, and 96.8% on day 14 (the remodeling stage), respectively. This trend suggests that all the particles can promote healing of the infected wound but the CaO2–Cu2O micromotors, especially when combined with RSF wound dressing, had the most substantial effect because of their antibacterial activity, macrophage polarization regulation, and cell migration promotion. The best wound healing rate was achieved by applying CaO2–Cu2O-RSF, followed by treatment with CaO2–Cu2O micromotors without RSF coverage. Thus, we hypothesize that the synergy between CaO2 and Cu2O accelerated wound healing, and dressing the wound with the RSF film can further reinforce the therapeutic effect. We observed no significant difference in body weight (an important indicator of the potential toxicity of a treatment) among the four treatment groups, which proves the biosafety of the microparticles (Fig. 5E).
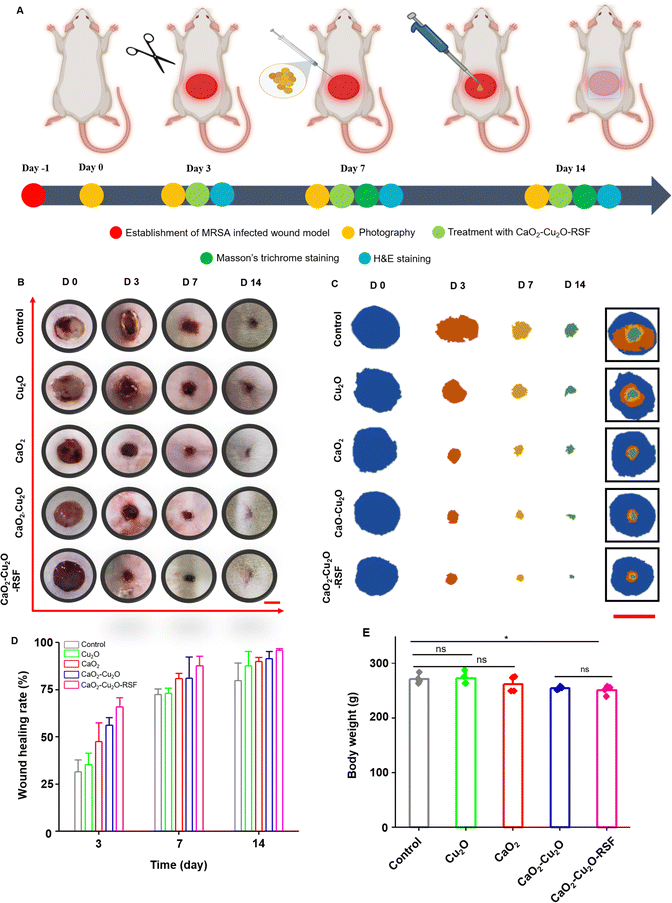 |
| Fig. 5
In vivo wound healing performance of different particles. (A) Protocol of the wound healing experiment on infected full-thickness skin model. (B) Representative images of skin wounds treated with Cu2O particles, CaO2 particles, CaO2–Cu2O micromotors, or CaO2–Cu2O-RSF on days 0, 3, 7, and 14; scale bar = 1 cm. (C) Traces of wound closure on days 0, 3, 7, and 14; scale bar = 1 cm. (D) Wound closure percentages. (E) Body weights. | |
The in vitro experiments demonstrated that CaO2–Cu2O micromotors could permeate into the deep area of simulated tissue fluid with the assistance of oxygen bubbles. However, whether the particles can enter the deep tissue in an actual wound is unknown. To test this hypothesis, the diffusion performance of CaO2–Cu2O micromotors in a wound area was investigated in histologic sections using PS particles as the control group. As shown in Fig. 6A, the PS particles tagged with red fluorescence were only seen aggregated on the surface of the wound, suggesting that PS particles cannot permeate into the deep tissue without some driving force. In contrast, CaO2–Cu2O micromotors with green fluorescence were observed in the deep tissue. These results confirmed that CaO2–Cu2O micromotors could penetrate fully into a deep-tissue wound with the assistance of oxygen bubbles.
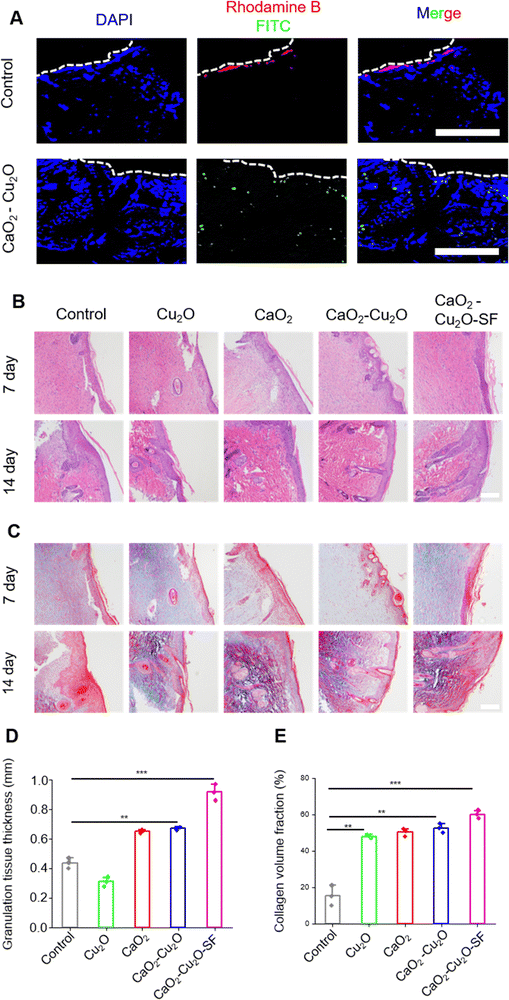 |
| Fig. 6 Histological evaluation of wound tissue. (A) Fluorescence staining images showing the distribution of CaO2–Cu2O micromotors (green) and PS particles (red) in the wound skin tissues; scale bar = 300 μm. (B) H&E-stained wound skin tissues on days 7 and 14; scale bar = 50 μm. (C) Masson's trichrome-stained wound skin tissues on days 7 and 14; scale bars = 50 μm. (D) Quantitative analysis of granulation tissue thickness. (E) Quantitative analysis of collagen volume fraction (n = 3, ***p < 0.001, **p < 0.01, and *p < 0.05). | |
To histologically assess the therapeutic effect and deduce the corresponding mechanism, H&E and Masson's trichrome staining (Fig. 6B and C) were performed on the regenerated skin tissue collected from the wound area. On the 7th day, the CaO2–Cu2O-SF group exhibited a more significant formation of a regular epidermal layer compared to the other groups. By the 14th day, the CaO2–Cu2O-SF group displayed a complete epidermal layer and distinct hair follicle structures. Quantitative analysis of the granulation tissue demonstrated that the CaO2–Cu2O-SF experimental group achieved optimal results (Fig. 6D). On the 14th day, Masson staining revealed collagen deposition in all experimental groups, with relatively less collagen deposition in the control group despite the complete healing of the epidermal layer. The CaO2–Cu2O-SF group exhibited the most pronounced collagen deposition. Quantitative analysis of collagen protein indicated that the levels were highest in the CaO2–Cu2O-SF group, suggesting its significant therapeutic efficacy (Fig. 6E).
Previous in vitro polarization experiments revealed that CaO2–Cu2O micromotors could increase the expression of M1-type macrophages. Although M1 macrophages can phagocytose pathogens and initiate tissue regeneration, including angiogenesis, a persistent and intense inflammatory response can inhibit the proliferation and remodeling stage of wound healing. Therefore, immunostaining of iNOS was performed to assess the inflammatory response in different healing phases. In the inflammatory stage (day 3), it was noted that the fluorescence intensity for iNOS in the treated groups was much stronger than that in the control group (Fig. 7A and C). The result suggests that these particles can polarize macrophages into M1 phenotypes during the inflammatory stage. On day 7, the fluorescence intensity for iNOS showed no significant difference between the control group and the treated groups, indicating that the additional inflammation induced by particles did not prolong the proliferation stage or impede tissue regeneration. When the wound healing progressed into the remodeling phase (day 14), the control group had brighter green fluorescence than the treated groups, suggesting that the inflammation in the treated group was slighter. This variation might be due to the enhanced tissue regeneration in the treated groups. In this situation, to create a sterile environment for the wound area, we utilized CaO2–Cu2O, which interferes with the stages of infection and inflammation due to its antibactericidal properties. Unlike the M1 polarization induced by bacterial infection, the CaO2–Cu2O-induced polarization can be automatically relieved. This allows the wound area to transition gradually into a proliferative stage as the bacteria are eradicated. The results indicate that CaO2–Cu2O does not prolong the inflammation period by intervening in the infection and inflammation phase. Instead, it is conducive to better later recovery.
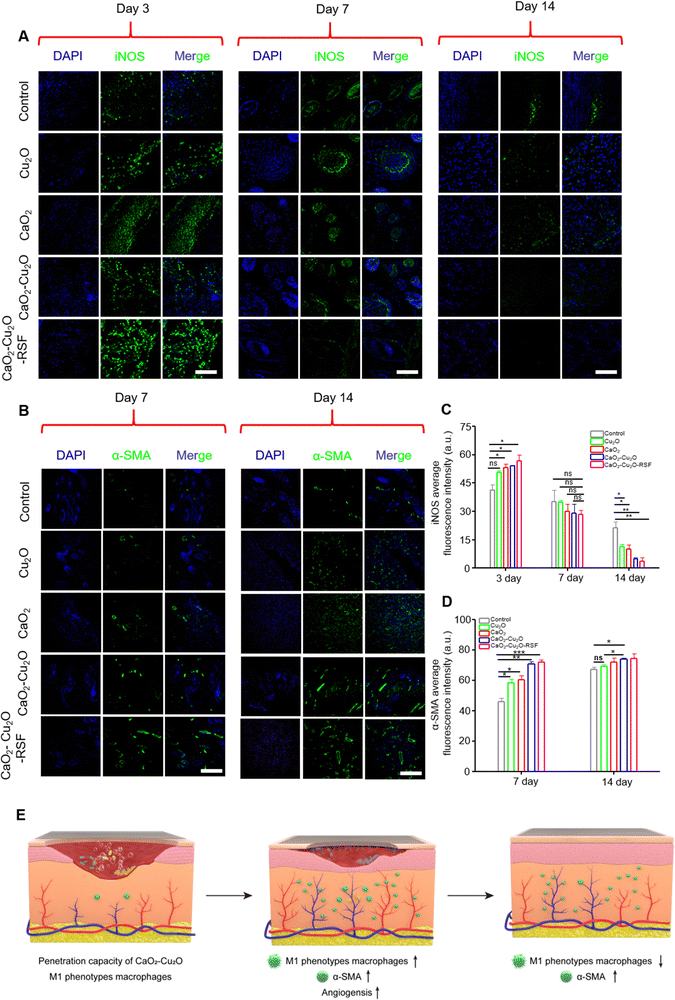 |
| Fig. 7 (A) Immunofluorescence images of iNOS (green) in skin tissue within the wound area on days 3, 7, and 14 reflect macrophage polarization in vivo; scale bar = 200 μm. (B) Immunofluorescence images of α-SMA (green) in skin tissue within the wound area on days 7 and 14 reflect angiogenesis in vivo; scale bars = 200 μm. (C) Quantitative analysis of iNOS density. (D) Quantitative analysis of α-SMA density. (E) Illustration of the permeation of CaO2–Cu2O micromotors in tissues to accelerate angiogenesis, bacterial killing, and collagen generation (n = 3, ***p < 0.001, **p < 0.01, and *p < 0.05). | |
Angiogenesis is crucial for wound healing because the newly formed blood vessels can transfer nutrients to the wound area to accelerate recovery.45 The cell-scratch assay showed that CaO2–Cu2O micromotors could promote the migration of HUVECs. The immunostaining of iNOS also suggested that CaO2–Cu2O micromotors could polarize macrophages into M1 phenotype in the inflammatory stage, thus improving angiogenesis. Therefore, the immunostaining of α-SMA was performed to verify the efficacy of CaO2–Cu2O micromotors on angiogenesis (Fig. 7B and D). In the proliferation stage (day 7), the green fluorescence of α-SMA was much stronger in the treated groups, indicating that the particles could indeed stimulate angiogenesis, with the CaO2–Cu2O micromotors and CaO2–Cu2O-RSF groups exhibiting the strongest fluorescence for α-SMA. When the time was prolonged to day 14, the fluorescence intensity for α-SMA increased in all groups, suggesting a higher blood vessel density in the wound area. The fluorescence intensity in the CaO2–Cu2O micromotors and CaO2–Cu2O-RSF groups was still significantly higher than in the control group. These promising results verified that the micromotors promoted angiogenesis in the wound area.
To systematically evaluate the preclinical safety of CaO2–Cu2O micromotors, organ indices, a hemogram, and the histopathology of the heart, liver, spleen, lung, and kidney were examined. There were almost no significant changes in organ indices (Fig. S10, ESI†). The routine blood analysis revealed no significant hematological differences among the groups, suggesting that these particles did not induce any abnormalities in the blood system (Fig. S11, ESI†). The histopathology sections revealed that the major organs of the treated groups had no obvious pathological features (Fig. S12, ESI†). The results demonstrate that these particles possessed excellent biosafety and had great potential in biomedical applications.
4. Conclusions
We have designed and constructed CaO2–Cu2O micromotors, which could penetrate deep wound tissues via gas propulsion. In vitro experiments demonstrated that these micromotors could kill bacteria, accelerate hemostasis, modulate the polarization of macrophages, and promote the migration of dermal fibroblasts and vascular endothelial cells. In vivo studies revealed that CaO2–Cu2O micromotors could promote the healing of infected wounds due to their bioactivities in accelerating angiogenesis, granulation tissue regeneration, and collagen deposition. Furthermore, the RSF film showed an apparent synergistic effect with CaO2–Cu2O micromotors for infected wound healing. These promising findings demonstrate that CaO2–Cu2O micromotors offer an advantageous therapeutic system for treating deep skin wounds with infections.
Data availability
Data will be made available on request.
Conflicts of interest
The authors declare that they have no known competing financial interests or personal relationships that could have appeared to influence the work reported in this paper.
Acknowledgements
This study was supported by the National Natural Science Foundation of China (82072060 and 22008201), the Fundamental Research Funds for the Central Universities (SWU-XDPY22006), the Venture & Innovation Support Program for Chongqing Overseas Returnees (2205012980212766), the Natural Science Foundation Project of Chongqing (cstc2020jcyj-msxmX0292), and the Natural Science Foundation Project of Chongqing for Distinguished Young Scholar (CSTB2022NSCQ-JQX0035).
References
- M. Gholipourmalekabadi, S. Sapru, A. Samadikuchaksaraei, R. L. Reis, D. L. Kaplan and S. C. Kundu, Silk fibroin for skin injury repair: where do things stand?, Adv. Drug Delivery Rev., 2020, 153, 28–53 CrossRef CAS PubMed.
- S. Alizadeh, P. Farshi, N. Farahmandian, Z. A. Ahovan, A. Hashemi and M. Majidi,
et al., Synergetic dual antibiotics-loaded chitosan/poly (vinyl alcohol) nanofibers with sustained antibacterial delivery for treatment of XDR bacteria-infected wounds, Int. J. Biol. Macromol., 2023, 229, 22–34 CrossRef CAS PubMed.
- Z. Wang, W. Hu, W. Wang, Y. Xiao, Y. Chen and X. Wang, Antibacterial electrospun nanofibrous materials for wound healing, Adv. Fiber Mater., 2023, 5(1), 107 CrossRef CAS.
- Z. A. Ahovan, Z. Esmaeili, B. S. Eftekhari, S. Khosravimelal, M. Alehosseini and G. Orive,
et al., Antibacterial smart hydrogels: New hope for infectious wound management, Mater. Today Bio., 2022, 100499 CrossRef PubMed.
- H. Choi, B. Kim, S. H. Jeong, T. Y. Kim, D. P. Kim and Y. K. Oh,
et al., Microalgae-Based Biohybrid Microrobot for Accelerated Diabetic Wound Healing, Small, 2023, 19(1), 2204617 CrossRef CAS PubMed.
- Q. Li, E. L. Hu, K. Yu, R. Q. Xie, F. Lu and B. T. Lu,
et al., Gemini Dressing with Both Super-hydrophilicity and-hydrophobicity Pursuing Isolation of Blood Cells for Hemostasis and Wound Healing, Adv. Fiber Mater., 2023, 1–20 Search PubMed.
- X. D. Zhao, D. N. Pei, Y. X. Yang, K. Xu, J. Yu and Y. C. Zhang,
et al., Green Tea Derivative Driven Smart Hydrogels with Desired Functions for Chronic Diabetic Wound Treatment, Adv. Funct. Mater., 2021, 31(18), 2009442 CrossRef CAS.
- Y. Zhang, Y. Wang, L. Chen, J. Zheng, X. Fan and X. Xu,
et al., An injectable antibacterial chitosan-based cryogel with high absorbency and rapid shape recovery for noncompressible hemorrhage and wound healing, Biomaterials, 2022, 285, 121546 CrossRef CAS PubMed.
- X. Zhao, Y. P. Liang, B. L. Guo, Z. H. Yin, D. Zhu and Y. Han, Injectable dry cryogels with excellent blood-sucking expansion and blood clotting to cease hemorrhage for lethal deep-wounds, coagulopathy and tissue regeneration, Chem. Eng. J., 2021, 403, 126329 CrossRef CAS.
- Y. Tian, L. Pang, R. Zhang, T. Xu, S. Wang and B. Yu,
et al., Poly-tetrahydropyrimidine Antibacterial Hydrogel with Injectability and Self-Healing Ability for Curing the Purulent Subcutaneous Infection, ACS Appl. Mater. Interfaces, 2020, 12(45), 50236 CrossRef CAS PubMed.
- Y. G. Cao, S. S. Liu, Y. Ma, L. L. Ma, M. H. Zu and J. F. Sun,
et al., Oral Nanomotor-Enabled Mucus Traverse and Tumor Penetration for Targeted Chemo-Sono-Immunotherapy against Colon Cancer, Small, 2022, 18(42), 2203466 CrossRef CAS PubMed.
- X. Zhou, H. Wang, J. Zhang, X. Li, Y. Wu and Y. Wei,
et al., Functional poly (ε-caprolactone)/chitosan dressings with nitric oxide-releasing property improve wound healing, Acta Biomater., 2017, 54, 128 CrossRef CAS PubMed.
- H. Weng, W. Jia, M. Li and Z. Chen, New injectable chitosan-hyaluronic acid based hydrogels for hemostasis and wound healing, Carbohydr. Polym., 2022, 294, 119767 CrossRef CAS PubMed.
- Q. Yu, B. H. Su, W. F. Zhao and C. S. Zhao, Janus Self-Propelled Chitosan-Based Hydrogel Spheres for Rapid Bleeding Control, Adv. Sci., 2022, 2205989 Search PubMed.
- W. Wang, H. M. Feng, J. G. Liu, M. T. Zhang, S. Liu and C. Feng,
et al., A photo catalyst of cuprous oxide anchored MXene nanosheet for dramatic enhancement of synergistic antibacterial ability, Chem. Eng. J., 2020, 386, 124116 CrossRef CAS.
- S. T. Wang, W. Wang, L. F. Yue, S. G. Cui, H. Y. Wang and C. Y. Wang,
et al., Hierarchical Cu2O nanowires covered by silver nanoparticles-doped carbon layer supported on Cu foam for rapid and efficient water disinfection with lower voltage, Chem. Eng. J., 2020, 382, 122855 CrossRef CAS.
- W. He, X. Wang, T. Hang, J. Chen, Z. Wang and D. A. Mosselhy,
et al., Fabrication of Cu(2 +)-loaded phase-transited lysozyme nanofilm on bacterial cellulose: Antibacterial, anti-inflammatory, and pro-angiogenesis for bacteria-infected wound healing, Carbohydr. Polym., 2023, 309, 120681 CrossRef CAS PubMed.
- Y. Feng, Z. Cheng, A. K. Larsen, H. Shi, T. Sun and P. Zhang,
et al., Amyloid-like nanofibrous network confined and aligned ultrafine bimetallic nanozymes for smart antibacterial therapy, Mater. Today Bio, 2023, 22, 100730 CrossRef CAS PubMed.
- Y. Feng, F. Chen, J. M. Rosenholm, L. Liu and H. Zhang, Efficient nanozyme engineering for antibacterial therapy, Mater. Futures, 2022, 1(2), 023502 CrossRef.
- J. Bai, Y. Feng, W. Li, Z. Cheng, J. M. Rosenholm and H. Yang,
et al., Alternative Copper-Based Single-Atom Nanozyme with Superior Multienzyme Activities and NIR-II Responsiveness to Fight against Deep Tissue Infections, Research, 2023, 6, 0031 CrossRef CAS PubMed.
- H. L. Xu, P. P. Chen, L. F. Wang, M. Q. Tong, Z. H. Cu and Y. Z. Zhao,
et al., Skin-permeable liposome improved stability and permeability of bFGF against skin of mice with deep second degree scald to promote hair follicle neogenesis through inhibition of scar formation, Colloids Surf., B, 2018, 172, 573 CrossRef CAS PubMed.
- S. Shen, M. Mamat, S. C. Zhang, J. Cao, Z. D. Hood and L. Figueroa-Cosme,
et al., Synthesis of CaO2 Nanocrystals and Their Spherical Aggregates with Uniform Sizes for Use as a Biodegradable Bacteriostatic Agent, Small, 2019, 15(36), 1902118 CrossRef PubMed.
- H. Guan, P. Cai, X. Zhang, Y. Zhang, G. Chen and C. Dong, Cu2O templating strategy for the synthesis of octahedral Cu2O@ Mn (OH)2 core–shell hierarchical structures with a superior performance supercapacitor, J. Mater. Chem. A, 2018, 6(28), 13668 RSC.
- G. M. Sun, Y. I. Shen and J. W. Harmon, Engineering Pro-Regenerative Hydrogels for Scarless Wound Healing, Adv. Healthcare Mater., 2018, 7(14), 1800016 CrossRef PubMed.
- W. Huang, Y. Wang, Z. Huang, X. Wang, L. Chen and Y. Zhang,
et al., On-Demand Dissolvable Self-Healing Hydrogel Based on Carboxymethyl Chitosan and Cellulose Nanocrystal for Deep Partial Thickness Burn Wound Healing, ACS Appl. Mater. Interfaces, 2018, 10(48), 41076 CrossRef CAS PubMed.
- R. T. Li, K. Liu, X. Huang, D. Li, J. X. Ding and B. Liu,
et al., Bioactive Materials Promote Wound Healing through Modulation of Cell Behaviors, Adv. Sci., 2022, 9(10), 2105152 CrossRef CAS PubMed.
- Y. F. Lu, H. S. Li, J. Wang, M. Y. Yao, Y. Peng and T. F. Liu,
et al., Engineering Bacteria-Activated Multifunctionalized Hydrogel for Promoting Diabetic Wound Healing, Adv. Funct. Mater., 2021, 31(48), 2105749 CrossRef CAS.
- H. Zeng, Z. R. Ying, X. Luo, S. Tan, X. H. Liu and X. Y. Zhao,
et al., Gallic acid-modified bioglass with combined photothermal and antibacterial effects for the regeneration of infected diabetic wound, Composites, Part B, 2023, 110668 CrossRef CAS.
- Z. Wang, W. You, W. Wang, W. Tian, F. Chen and Y. Xiao,
et al., Dihydromyricetin-incorporated multilayer nanofibers accelerate chronic wound healing by remodeling the harsh wound microenvironment, Adv. Fiber Mater., 2022, 1–16 Search PubMed.
- E. Rognoni, A. O. Pisco, T. Hiratsuka, K. H. Sipila, J. M. Belmonte and S. A. Mobasseri,
et al., Fibroblast state switching orchestrates dermal maturation and wound healing, Mol. Syst. Biol., 2018, 14(8), e8174 CrossRef PubMed.
- M. Kang, H. Kim, J. You, Y. Choi, B. Kwon and C. Park,
et al., Hydrogel cross-linking–programmed release of nitric oxide regulates source-dependent angiogenic behaviors of human mesenchymal stem cell, Sci. Adv., 2020, 6(9), eaay5413 CrossRef CAS PubMed.
- Z. Lin, R. Li, Y. Liu, Y. Zhao, N. Ao and J. Wang,
et al., Histatin1-modified thiolated chitosan hydrogels enhance wound healing by accelerating cell adhesion, migration and angiogenesis, Carbohydr. Polym., 2020, 230, 115710 CrossRef CAS PubMed.
- Y. Lu, H. Li, J. Wang, M. Yao, Y. Peng and T. Liu,
et al., Engineering Bacteria-Activated Multifunctionalized Hydrogel for Promoting Diabetic Wound Healing, Adv. Funct. Mater., 2021, 31(48), 2105749 CrossRef CAS.
- E. L. Mills, B. Kelly, A. Logan, A. S. H. Costa, M. Varma and C. E. Bryant,
et al., Succinate Dehydrogenase Supports Metabolic Repurposing of Mitochondria to Drive Inflammatory Macrophages, Cell, 2016, 167(2), 457 CrossRef CAS PubMed.
- M. Sharifiaghdam, E. Shaabani, R. Faridi-Majidi, S. C. De Smedt, K. Braeckmans and J. C. Fraire, Macrophages as a therapeutic target to promote diabetic wound healing, Mol. Ther., 2022, 30(9), 2891 CrossRef CAS PubMed.
- D. Cabrera, K. Walker, S. Moise, N. D. Telling and A. G. S. Harper, Controlling human platelet activation with calcium-binding nanoparticles, Nano Res., 2020, 13(10), 2697 CrossRef CAS PubMed.
- Y. Yang, Y. Du, J. Zhang, H. Zhang and B. Guo, Structural and functional design of electrospun nanofibers for hemostasis and wound healing, Adv. Fiber Mater., 2022, 4(5), 1027 CrossRef CAS.
- S. Sapru, S. Das, M. Mandal, A. K. Ghosh and S. C. Kundu, Prospects of nonmulberry silk protein sericin-based nanofibrous matrices for wound healing–in vitro and in vivo investigations, Acta Biomater., 2018, 78, 137 CrossRef CAS PubMed.
- S. Kar, S. Talukdar, S. Pal, S. Nayak, P. Paranjape and S. C. Kundu, Silk Gland Fibroin from Indian Muga Silkworm Antheraea assama as Potential Biomaterial, Tissue Eng. Regener. Med., 2013, 10(4), 200 CrossRef CAS.
- A. Shapouri-Moghaddam, S. Mohammadian, H. Vazini, T. Mahdi, E. Seyed-Alireza and M. Fatemeh,
et al., Macrophage plasticity, polarization, and function in health and disease, J. Cell. Physiol., 2018, 233(9), 6425 CrossRef CAS PubMed.
- J. Mao, L. Chen, Z. Cai, S. Qian, Z. Liu and B. Zhao,
et al., Advanced biomaterials for regulating polarization of macrophages in wound healing, Adv. Funct. Mater., 2022, 32(12), 2111003 CrossRef CAS.
- J. Huang, J. Wu, J. Wang, M. Xu, J. Jiao and Y. Qiang,
et al., Rock Climbing-Inspired Electrohydrodynamic Cryoprinting of Micropatterned Porous Fiber Scaffolds with Improved MSC Therapy for Wound Healing, Adv. Fiber Mater., 2023, 5(1), 312 CrossRef CAS.
- F. I. Ataullakhanov, A. V. Pohilko, E. I. Sinauridze and R. I. Volkova, Calcium threshold in human plasma clotting kinetics, Thromb. Res., 1994, 75(4), 383 CrossRef CAS PubMed.
- L. Jing, S. Rota, F. Olivier, D. Momier, J. M. Guigonis and S. Schaub,
et al., Proteomic analysis identified LBP and CD14 as key proteins in blood/biphasic calcium phosphate microparticle interactions, Acta Biomater., 2021, 127, 298–312 CrossRef CAS PubMed.
- Q. Y. Zhang, J. Tan, R. Nie, Y. T. Song, X. L. Zhou and Z. Y. Feng,
et al., Acceleration of wound healing by composite small intestinal submucosa hydrogels through immunomodulation, Composites, Part B, 2023, 110550 CrossRef CAS.
|
This journal is © The Royal Society of Chemistry 2024 |
Click here to see how this site uses Cookies. View our privacy policy here.