DOI:
10.1039/D3TB02861E
(Review Article)
J. Mater. Chem. B, 2024,
12, 4118-4137
Vaccine adjuvants: current status, research and development, licensing, and future opportunities
Received
4th December 2023
, Accepted 27th February 2024
First published on 9th April 2024
Abstract
Vaccines represent one of the most significant inventions in human history and have revolutionized global health. Generally, a vaccine functions by triggering the innate immune response and stimulating antigen-presenting cells, leading to a defensive adaptive immune response against a specific pathogen's antigen. As a key element, adjuvants are chemical materials often employed as additives to increase a vaccine's efficacy and immunogenicity. For over 90 years, adjuvants have been essential components in many human vaccines, improving their efficacy by enhancing, modulating, and prolonging the immune response. Here, we provide a timely and comprehensive review of the historical development and the current status of adjuvants, covering their classification, mechanisms of action, and roles in different vaccines. Additionally, we perform systematic analysis of the current licensing processes and highlights notable examples from clinical trials involving vaccine adjuvants. Looking ahead, we anticipate future trends in the field, including the development of new adjuvant formulations, the creation of innovative adjuvants, and their integration into the broader scope of systems vaccinology and vaccine delivery. The article posits that a deeper understanding of biochemistry, materials science, and vaccine immunology is crucial for advancing vaccine technology. Such advancements are expected to lead to the future development of more effective vaccines, capable of combating emerging infectious diseases and enhancing public health.
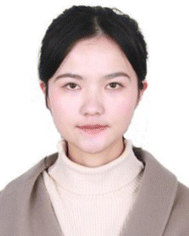
Ying Cui
| Ying Cui is a PhD student at the University of California, Los Angeles. She received her BS in Applied Chemistry from South China University of Technology, and her MS in Chemical Engineering from Zhejiang University. Her current research focuses on high-performance materials for biology and energy applications. |
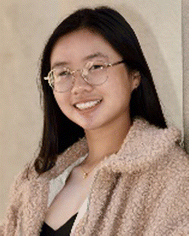
Megan Ho
| Megan Ho is a fourth-year undergraduate Bioengineering student at the University of California, Los Angeles. Her research interests include drug discovery and development. She is currently at Partillion Bioscience working with microfluidic devices to create nanovials for streamlined single cell analysis and testing. |
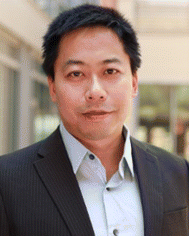
Yongjie Hu
| Yongjie Hu is a Principal Investigator at the Henry Samueli School of Engineering and Applied Science, University of California, Los Angeles. His current research utilizes interdisciplinary experimental and theoretical approaches to investigate biomedical and energy transport mechanisms and to develop advanced materials and characterization techniques. Prior to joining UCLA, he received his PhD degree from Harvard University and completed a postdoctoral fellowship at the Massachusetts Institute of Technology. |
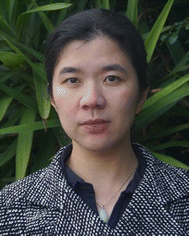
Yuan Shi
| Yuan Shi is a Principal Investigator at the David Geffen School of Medicine, University of California, Los Angeles (UCLA). Her current research focuses on using synthetic viral libraries in combination with deep sequencing platforms to study viral–host interactions and within-host viral evolutions, emphasizing exploitation of the information for next-generation vaccine and anti-viral treatment development. Prior to UCLA, she received her PhD degree in Biological and Biomedical Sciences from Harvard University and research training from Harvard Medical School. |
Introduction: the history of vaccine adjuvants
The field of vaccine adjuvant research has advanced significantly since its inception at the start of the 20th century (Fig. 1).1–14 As infectious diseases have become more prevalent, immunization has emerged as the most effective method for limiting their spread and reducing the associated harm. However, early vaccines often proved ineffective because purified antigens alone were unable to elicit a strong enough immune response. To overcome this challenge, the concept of adjuvants was introduced. Adjuvants are materials added to vaccines to boost their immunogenicity, thereby enhancing their effectiveness.
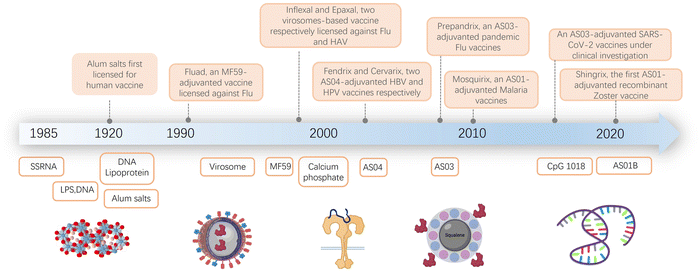 |
| Fig. 1 Timeline of major events in vaccine adjuvant development. This timeline illustrates the progressive advancement of vaccine adjuvants, evolving from natural components like RNA and DNA to synthetically engineered adjuvants. Schematics created with BioRender.com. | |
The development of modern adjuvants has been a slow and challenging process. Researchers aim to create vaccines that induce strong immune responses while maintaining safety. This effort involves selectively incorporating well-defined molecules and formulations, and exploring both natural and synthetic compounds. As summarized in Fig. 1, the composition of adjuvants has evolved over time. It has progressed from early natural ingredients to contemporary synthetic compounds.1,2,9,10,12,14–22
Despite the challenges in adjuvant development, over 30 licensed vaccines from various manufacturers currently contain adjuvants. Aluminum salts, the first discovered adjuvant, have been found to enhance the immune response to vaccines against diseases such as hepatitis B, tetanus, diphtheria, pertussis, and human papillomavirus. The effectiveness of aluminum salts is attributed to factors like their ability to form a depot at the injection site, induce inflammation, and activate dendritic cells and T cells.3,12,13,23–34 Although aluminum has been used in vaccines for over seven decades, its precise immune mechanism remains not fully understood.
Later adjuvant developments in the late 1990s included oil-in-water emulsions.35–42 These emulsions facilitate the slow release of antigens over an extended period, activate the innate immune system, and induce both humoral and cellular immune responses. One of the first uses of such emulsions was in Fluad, a licensed seasonal influenza vaccine for adults over 65 years old.43,44 Other FDA-licensed adjuvants include MF5913,36 (an oil-in-water emulsion adjuvant containing squalene, a biodegradable oil, and a surfactant, used in the influenza vaccine) and AS0345–47 (comprising α-tocopherol, squalene, and a surfactant, used in H1N1 influenza vaccine and some pandemic influenza vaccines). AS012,48 (a mix of liposomes and monophosphoryl lipid A) has been used in the RTS,S/AS01 malaria vaccine and the Shingrix vaccine for shingles.
While many other adjuvants have shown high potency in preclinical models, most have not yet been licensed for human use due to safety or tolerability concerns. Additionally, the molecular mechanisms of how existing adjuvants, including alum, MF59, and the Adjuvant Systems AS0 adjuvants, work in humans are still not well understood.
Benefits and effects from adjuvants
Overall, vaccine adjuvants play crucial roles in enhancing the effectiveness and accessibility of vaccines, and in reducing the burden of infectious diseases globally. Their uses and other practical applications are detailed below (Fig. 2):
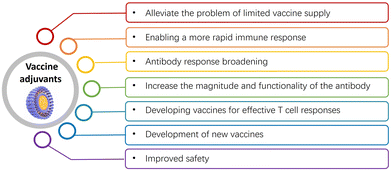 |
| Fig. 2 Potential benefits of adjuvants. | |
(1) Alleviating limited vaccine supply: adjuvants boost the immune response, allowing for fewer vaccine doses to achieve full protection. This eases healthcare system strain and increases accessibility for those unable to receive multiple doses.15,17,49 Adjuvants can also lower the necessary antigen doses in vaccines, thereby potentially reducing side effects and increasing availability. For instance, recombinant vaccines offer significant manufacturing advantages but are often weakly immunogenic on their own. Combining adjuvants with recombinant pandemic influenza protein can substantially reduce the amount of antigen needed to achieve target antibody levels, leading to a significant increase in manufacturing capacity. For example, AS03 is an oil-in-water emulsion adjuvant that has been used in vaccines such as the H1N1 influenza vaccine.2,45,46 It has been shown to improve immune responses and allow for antigen sparing, meaning that smaller amounts of the vaccine antigen can be used while still generating a strong immune response which can help stretch the available vaccine supply.
(2) Enabling rapid immune response: adjuvants stimulate a stronger, longer-lasting response to vaccine antigens,17,24,30,34,50–53 offering better protection. For instance, the addition of the AS04 adjuvant to hepatitis B antigen in GlaxoSmithKline's (GSK's) Fendrix enabled a reduction of a three-dose regimen to two. For example, Advax is a delta inulin-based adjuvant that has been shown to accelerate the immune response. It activates the innate immune system and promotes the production of cytokines and chemokines, which help enhance the adaptive immune response.
(3) Broadening antibody response: adjuvants expand the antibody response against pathogens with significant antigenic drift or strain variations,54,55 critical for diseases like influenza, human papillomavirus (HPV), and the malaria parasite. For example, MF59 is an oil-in-water emulsion adjuvant composed which has been shown to enhance both cellular and humoral immune responses and significantly increase antibody titers and improve the quality of the antibody response.36,56
(4) Enhancing antibody response magnitude and functionality: adjuvants not only increase the magnitude of antibody responses but also their functionality, affinity, or number of functional antibodies produced by the immune system.20,57,58 For example, AS04 is an adjuvant that combines aluminum salts with monophosphoryl lipid A (MPLA), a toll-like receptor 4 agonist. AS04 has been shown to enhance both the magnitude and functionality of antibody responses. It promotes the production of high-quality antibodies, including increased antibody titers and improved antibody affinity and avidity.59
(5) Enhancing T cell responses: new vaccine candidates are being developed to improve T cell responses, which are not effectively induced by existing adjuvants approved for human use.60–63 The goal is to elicit more effective engagement of T helper cells for optimizing the quality and durability of antibody responses, or to induce effector CD4+ or CD8+ T cells that can target and eliminate intracellular pathogens. Therefore, new vaccines may include agonists for toll-like receptors (TLRs) and other innate immune receptors to facilitate the generation of T helper cell responses. This approach aims to overcome the limitations of traditional adjuvants and create more effective vaccines that can provide long-lasting protection against a wider range of pathogens. By improving T cell responses, these vaccines may also provide benefits for individuals with weakened immune systems or those who may not respond well to traditional vaccines. For example, CpG ODNs are synthetic DNA molecules containing specific immunostimulatory sequences that act as toll-like receptor 9 (TLR9) agonists which have been demonstrated to enhance T cell responses by promoting the activation and differentiation of antigen-specific CD4+ and CD8+ T cells.12,64–66 They induce a Th1-type immune response, resulting in increased T cell proliferation, cytokine production, and cytotoxic T cell activity.
(6) Developing new vaccines: adjuvants are vital in developing vaccines for diseases where traditional vaccines are ineffective, enhancing immune responses and targeting multiple pathogen strains. They can enhance the immune response to a vaccine and help to target multiple strains of a pathogen.2,14,15,67 For example, in the case of the flu vaccine, adjuvants have been used to create vaccines that can provide better protection against different strains of the influenza virus.36,68 Additionally, adjuvants can be used to develop vaccines for diseases that are caused by pathogens for which there are no effective vaccines available yet, such as HIV or malaria. The development of adjuvants that can stimulate a strong and targeted immune response against these pathogens is an important area of research. For example, in virus-like particle (VLP) adjuvants, VLPs are self-assembled structures that mimic the overall structure of viruses but lack viral genetic material, making them non-infectious. VLPs can serve as both antigens and adjuvants.69,70 They have been used in the development of new vaccines to enhance immune responses, improve vaccine efficacy, and broaden the immune response to different viral strains or subtypes.
(7) Improving safety: by enhancing the immune response, adjuvants can reduce vaccine-associated adverse event risks. This is because the immune system is better able to target and eliminate the antigen, reducing the risk of an overactive immune response that can lead to side effects.37,45,71 Therefore, the use of adjuvants in vaccines has become an important strategy for improving the efficacy, safety, and accessibility of vaccines. For example, virosomes are liposome-like particles that incorporate viral proteins, such as influenza hemagglutinin, into their structure. They have been used as adjuvants in vaccines to improve safety. Virosomes provide the antigenic properties of viruses without the infectious genetic material, reducing the risk of vaccine-associated adverse events. Virosomal adjuvants have been utilized in vaccines for diseases like influenza and hepatitis A.
Categories of adjuvants and their acting mechanisms
In this section, we discuss typical vaccine adjuvants (Fig. 3), covering their components, functional mechanisms, applications in vaccines, and their advantages and disadvantages, as well as the challenges associated with them.
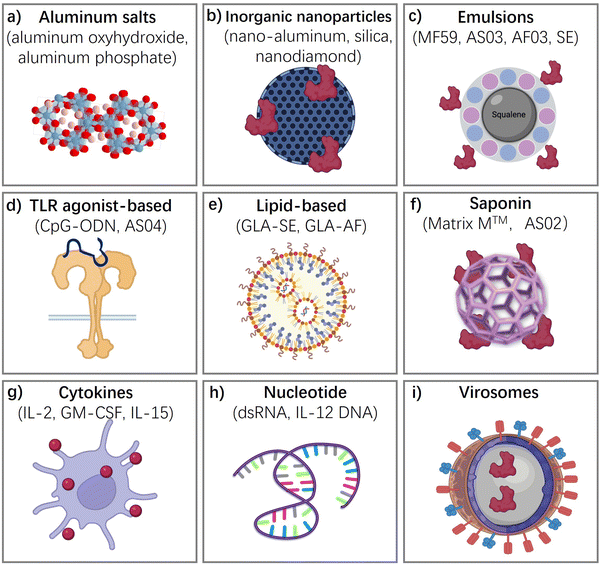 |
| Fig. 3 Types of vaccine adjuvants. Schematics created with BioRender.com. | |
Aluminum salts-based adjuvants
Aluminum salt-based adjuvants, commonly known as alum, were the first adjuvants used in licensed human vaccines and continue to be the most widely used adjuvants. They consist of aluminum hydroxide, aluminum phosphate, or a combination of both and are known to form a depot at the injection site, slowly releasing the vaccine antigen over time, which results in a stronger and prolonged immune response.3,12,13,23–34,40 They have been shown to enhance the immune response to a wide range of antigens, including those in vaccines for hepatitis B, human papillomavirus (HPV), and pneumococcal disease. Due to their proven safety record, wide-spectrum ability to strengthen immune responses, and ease of manufacture, they remain an attractive option for vaccine development. However, there have been some concerns about the potential for aluminum salts to cause adverse effects, such as local reactions at the injection site and rare cases of allergic reactions. The benefits of using aluminum salts as vaccine adjuvants generally outweigh the risks.
The exact mechanism by which aluminum salts-based adjuvants work is not fully understood, but there are several proposed theories (Fig. 4(a)). One of the key reasons for the popularity of alum as an adjuvant is their ability to form a depot at the site of injection. This depot slowly releases the vaccine antigen over time, providing a prolonged exposure of the immune system to the antigen. This prolonged exposure helps to enhance the immune response, leading to stronger and more durable immunity.
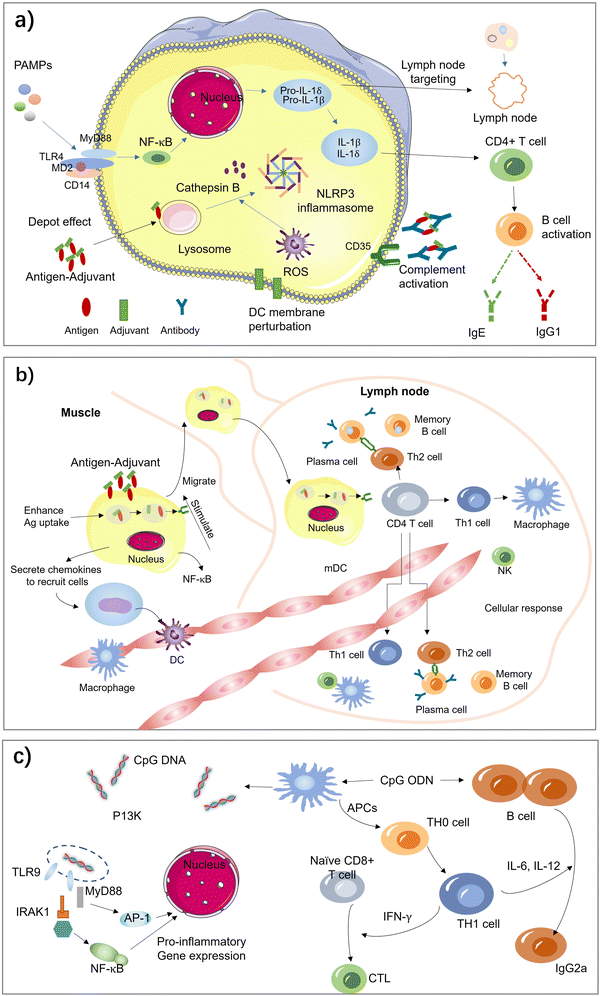 |
| Fig. 4 Acting mechanisms of vaccine adjuvants. (a) Aluminum salt-based adjuvants. (b) Emulsion adjuvants. (c) TLR agonist-based adjuvants. | |
In addition, alum is also believed to activate the innate immune system by inducing inflammation at the injection site. This inflammation is thought to be triggered by the activation of the inflammasome, a protein complex that plays a key role in the innate immune response. Activated inflammasomes release pro-inflammatory cytokines, which help to attract immune cells to the site of inflammation and activate them.24,25
Aluminum salts-based adjuvants are also believed to activate dendritic cells, which are specialized immune cells that present the antigen to T cells, responsible for recognizing and attacking foreign invaders. Aluminum salts-based adjuvants activate dendritic cells by inducing the release of pro-inflammatory cytokines, such as IL-1β and TNF-α, which attract dendritic cells to the injection site and activate them. In turn, dendritic cells promote the differentiation of effector T cells, which play a key role in the induction of immunity.29,30,34,72
Alum is also believed to stimulate the differentiation of CD4+ T cell and induce the production of antibodies by B cells, which are critical for the induction of immunity. This process is mediated by the production of IL-1β and IL-18 by dendritic cells, which promote the differentiation of effector T cells. The slow release of antigen from the depot also helps to prolong the exposure of B cells to the antigen, which can enhance the production of antibodies.
In summary, aluminum salts-based adjuvants work by forming a depot at the injection site, activating the inflammasome, inducing the release of pro-inflammatory cytokines, attracting dendritic cells to the site of injection, and enhancing the production of antibodies by B cells. These mechanisms work together to enhance the immune response to the antigen in the vaccine and improve protection against the target disease.
Inorganic nanoparticle-based adjuvants
Inorganic nanoparticle-based vaccine adjuvants,73–82 such as nano-aluminum,82 layered double hydroxides (LDH),81 and nano/mesoporous-silica,77,78 nanodiamond,80 quantum dots,75 have been investigated for their potential to enhance the immune response to vaccines. Inorganic nanoparticles present a versatile platform for surface modifications, allowing for precise control over size and shape parameters. This versatility facilitates the engineering of tailored architectures aimed at eliciting specific immune responses. Furthermore, these nanoparticles typically exhibit robust chemical and physicochemical stability, ensuring prolonged shelf life without the need for refrigeration during storage and transportation. Such attributes are crucial for practical vaccine deployment and distribution scenarios, particularly in resource-limited settings, and render them ideal candidates for stockpiling vaccines to address future emergency needs.
The mechanism of action of inorganic nanoparticle-based vaccine adjuvants involves their interaction with the immune system to enhance and modulate the immune response to antigens present in vaccines.73 Inorganic nanoparticles serve as carriers for antigens, ensuring their efficient delivery to antigen-presenting cells (APCs). Nanoparticles are designed to be efficiently taken up by APCs, such as dendritic cells and macrophages. This uptake is facilitated by the size, surface charge, and surface modifications of the nanoparticles. Some inorganic nanoparticle adjuvants, such as aluminum-based adjuvants, induce localized inflammation at the injection site. This attracts immune cells to the site and promotes the recruitment of APCs. Inorganic nanoparticle adjuvants contribute to the development of long-lasting immune memory. This ensures that the immune system “remembers” the encountered antigens, providing protection upon subsequent exposure to the pathogen.
Among them, γ-phase aluminum oxyhydroxide nanoparticles (γ-AlOOH) with nanofiber morphology was prepared and used as a delivery system for the hydrothermal extract of human tubercle bacillus (HTB).82 Due to its distinctive mesoporous fiber structure, the synthesized γ-AlOOH significantly enhanced cellular uptake by macrophage-like cells, showing an approximately two-fold increase compared to commercial alum.
Mesoporous silica possesses an ordered porous structure with uniform-sized pores in the mesoscale range (2 to 50 nm).77,78 This structure provides a large surface area and tunable properties, making it suitable for various applications, including drug delivery and vaccine adjuvants. The surface of mesoporous silica can be modified to tailor its properties for specific vaccine formulations and applications. Mesoporous silica can also be utilized to encapsulate immunomodulators, further enhancing the adjuvant effect. For example, amorphous silica can be used as a vaccine adjuvant to enhance immune response. Both nanoscale and microscale silica promoted NLRP3 inflammasome activation in myeloid cells, whereas only nanoscale silica activated the inflammasome in keratinocytes.
LDHs are layered materials with a positively charged brucite-like layer and interlayer anions, providing a unique structure for various applications, including drug delivery and immunomodulation.81 LDHs offer a high loading capacity for antigens due to their layered structure. LDHs can be designed to release antigens in a controlled manner, promoting a sustained and prolonged immune response. LDHs can be surface-modified to incorporate additional functionalities, such as targeting ligands or immunomodulatory agents, to further tailor their performance.
In summary, inorganic nanoparticle-based as vaccine adjuvants represents an active area of research, and their application in vaccine development holds promise for enhancing immune responses and improving the overall effectiveness of vaccines.
Emulsions adjuvants
Emulsions are formed when two liquids are brought together that are unable to form a homogenous mixture. Antigen is contained in these water droplets and the oil acts as a depot, slowly releasing antigen and enhances the immune response by decreasing clearance time and prolonging antigen exposure. Emulsions are another type of vaccine adjuvant that have been developed to enhance the immune response to vaccines. Emulsions consist of two immiscible liquids, typically an oil phase and a water phase, that are stabilized by a surfactant. Emulsions work by forming small droplets of the antigen in the oil phase, which are then dispersed throughout the water phase.2,35,36,83
One of the earliest oil-in-water adjuvants approved for human vaccines is MF59, which contains squalene, polysorbate 80, sorbitan trioleate, and trisodium citrate dehydrate. MF59 induces ATP release and upregulates cytokines and chemokines to enhance antigen-specific immune responses at the vaccination site. It has been shown to be safe and well-tolerated. Another oil-in-water emulsion-based adjuvant is AS03, which contains surfactant polysorbate 80 and two biodegradable oils, squalene, and DL-a-tocopherol. The European Commission granted marketing authorization for AS03-adjuvanted Pandemrix in 2009, and the AS03-adjuvanted influenza A (H5N1) monovalent vaccine was licensed by the FDA in 2013. AS03 has been increasingly used in influenza and malaria vaccines.84
Emulsions have been used in various vaccines, including influenza, hepatitis B, and human papillomavirus (HPV) vaccines. They improve vaccine efficacy, particularly in populations that may not respond well to traditional vaccines, such as the elderly or immunocompromised individuals. Emulsion adjuvants work by enhancing the immune response to vaccines through several mechanisms. There are several proposed mechanisms to understand how the emulsion-based adjuvants work in Fig. 4(b).41
One of the primary mechanisms is the slow release of antigen over an extended period of time. The small size of the emulsion droplets allows for an extended surface area to volume ratio, which enhances antigen presentation and uptake by antigen-presenting cells. This slow release of antigen can activate the immune system over a longer period of time, resulting in a more robust and prolonged immune response.
Emulsion adjuvants also activate the innate immune system. The emulsion droplets are recognized as foreign by the immune system, leading to the activation of antigen-presenting cells such as dendritic cells, macrophages, and monocytes. These cells then produce pro-inflammatory cytokines and a complex of chemokineses, such as TNF-α and IL-1β, which further activate the immune system and attract immune cells to the site of injection and form an immunocompetent environment for enhanced antigen transportation to the draining lymph nodes.
Another important mechanism of emulsion adjuvants is their ability to induce both humoral and cellular immune responses. The emulsion droplets can be taken up by antigen-presenting cells, which then present the antigen to T cells and B cells. T cells are responsible for directly attacks infected cells, while B cells produce antibodies that can neutralize pathogens. Emulsion adjuvants have been shown to activate both T cells and B cells, leading to a more comprehensive immune response.
In summary, emulsion adjuvants have become an important tool in the development of vaccines. They work by enhancing the immune response to vaccines through several mechanisms, including slow release of antigen, activation of the innate immune system and induce both humoral and cellular immune responses.
Toll-like receptor (TLR) agonists
Toll-like receptors (TLRs) are a type of pattern recognition receptors that play a crucial role in the innate immune response to invading pathogens.85–90 TLRs are transmembrane receptors that are primarily expressed by cells of the innate immune system. These receptors are classified into two groups: cell surface TLRs (TLR1, TLR2, TLR4, TLR5, and TLR6) and intracellular TLRs (TLR3, TLR7, TLR8, and TLR9), which are expressed on endosomal membranes. Each TLR contains a transmembrane domain, a leucine-rich repeat (LRR) segment that recognizes pathogen-associated molecular patterns (PAMPs) and damage-associated molecular patterns (DAMPs), and a toll/interleukin-1 receptor (TIR) domain that initiates downstream signal transduction and induces an inflammatory response. Thus, TLRs are attractive targets for adjuvants to stimulate an effective immune response that provides long-lasting protection against pathogens. Toll-like receptor (TLR) agonists are highly promising as adjuvants in vaccines against life-threatening and complex diseases such as cancer, AIDS, and malaria.
CpG oligodeoxynucleotides (ODNs) are synthetic DNA molecules with a phosphorothioate backbone that contains unmethylated CpG motifs.64–66Fig. 4(c) describe how the TRL-based adjuvants acting: CpG ODN is a potent adjuvant that induces type 1 helper T (Th1) responses and promotes the generation of cytotoxic T lymphocytes (CTLs) and secretion of interferon-gamma (IFN-γ). Activation of TLR9 by CpG ODN enhances specific humoral and cellular immune responses to antigens. Due to its unique characteristic, CpG ODN can be used as an adjuvant for vaccination via intramuscular, subcutaneous, oral, and intranasal routes. As effective vaccine adjuvants, CpG ODN can promote the immunostimulatory effect of antigens, activate antigen-presenting cells (APCs), and accelerate immune responses. CpG ODNs facilitate the expression of major histocompatibility complex (MHC), CD40, and CD86 on plasmacytoid dendritic cells (pDCs) and enhance antigen processing, leading to a robust and sustained immune response.
Liposomes
Liposomes, a type of vaccine adjuvant, are composed of phospholipids and cholesterol and are small, spherical structures that can encapsulate antigens and other immunostimulatory molecules.91–93 They are biodegradable, biocompatible, and allow for versatile structure modifications that enable adjustable characteristics and toxicity. Liposomes can be used to deliver antigens to antigen-presenting cells, such as dendritic cells, which can activate the immune system. Additionally, liposomes are able to enhance both humoral and cellular immune responses, inducing a Th1-biased response that is important for clearing intracellular pathogens and enhancing antibody responses, making them an attractive adjuvant for vaccine development. One of the unique characteristics of liposomes is their ability to be modified to target specific cell types. They can be modified with antibodies or peptides that target specific receptors on dendritic cells, increasing the uptake of the liposome-encapsulated antigen. Liposomes can also be used to co-deliver multiple antigens or immunostimulatory molecules, leading to a more robust immune response, particularly for complex pathogens that may require multiple antigens to induce a protective immune response.
AS01, an adjuvant system based on liposomes, contains two immunostimulatory agents, 3-O-desacyl-4′-monophosphoryl lipid A (MPL) and QS-21.2,48 Vaccines that use AS01 adjuvant include the newly developed malaria vaccine RTS, S (Mosquirix), as well as vaccine candidates against herpes zoster (HZ/su), HIV, and tuberculosis. AS01 can promote the production of both antigen-specific antibodies and antigen-specific CD4+ T cells, which distinguishes it from other adjuvants.
Other adjuvants
Cytokines are a diverse group of signaling molecules that play important roles in the immune response.94,95 They are secreted by various cells of the immune system and act on specific receptors to mediate a range of biological functions, such as inflammation, cell proliferation, and differentiation. Cytokines can be divided into different categories based on their structure and function, including interleukins, interferons, tumor necrosis factors, and chemokines.
Polymers, on the other hand, are compounds that consist of repeating units joined together by covalent bonds. They can be either natural or synthetic and have a wide range of applications in materials science, medicine, and biotechnology.96,97 In the context of vaccines, polymers can be used as carriers to create slow-release depots that entrain antigens and other immunomodulatory agents in their large, cross-linked structure. This results in sustained antigen presentation and activation of the immune system, which can lead to stronger and longer-lasting immune responses.
Saponins are naturally occurring compounds that have amphipathic properties, meaning they have both hydrophilic and hydrophobic regions.52,98,99 They have been used in traditional medicine for centuries and have many potential pharmaceutical applications, including as adjuvants in vaccines. Saponins can enhance the immune response by stimulating antigen-presenting cells such as dendritic cells and by promoting the production of cytokines and chemokines. One example of a saponin-based adjuvant is QS-21, which is used in combination with MPL (monophosphoryl lipid A) in the AS01 adjuvant system. AS01 is an effective adjuvant that has been used in the development of vaccines against malaria, tuberculosis, and herpes zoster, among other diseases.
Virosomes are composed of phospholipids and viral envelope proteins, which are typically derived from viruses that do not cause disease in humans.100,101 The viral envelope proteins provide an immunogenic surface that can activate the immune system, while the phospholipids help to stabilize the virosome structure and increase its uptake by antigen-presenting cells.
Various vaccines and their adjuvants roles
In this section, we discuss the roles of adjuvants in various vaccines. Fig. 5 summarizes the major confirmed vaccines and approaches currently under development. These include whole-virus vaccines (such as live-attenuated, inactivated, and viral-vector vaccines), subunit vaccines, and nucleic acid vaccines (including pDNA and mRNA).
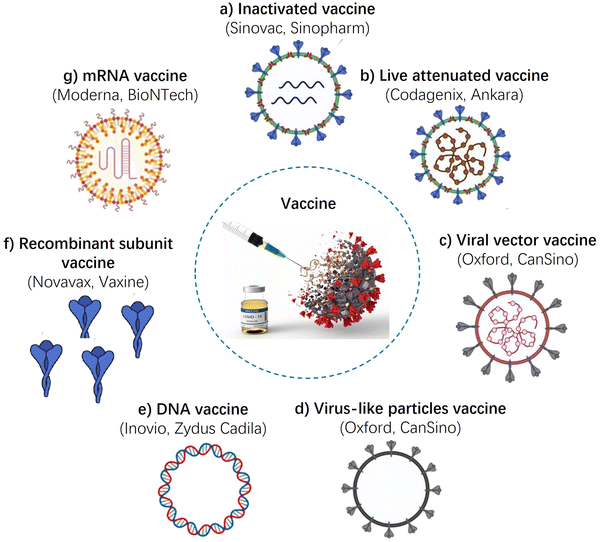 |
| Fig. 5 Summar of various vaccines with their adjuvants. | |
Inactivated vaccines
Inactivated vaccines are usually produced from virus-infected cells and are inactivated, which ensures that the viruses are no longer infectious but retain their antigenic properties, making them useful for generating an immune response.102,103 Adjuvants can be added to inactivated vaccines to enhance the immune response. Aluminum salts, such as aluminum hydroxide and aluminum phosphate, are the most commonly used adjuvants in inactivated vaccines. They enhance the immune response by adsorbing the antigen onto their surface, which allows for slow release of the antigen and stimulation of the immune system over a longer period of time. Other adjuvants used in inactivated vaccines include MF59, AS03, and AS04. MF59 is an oil-in-water emulsion that enhances the immune response by creating an antigen depot at the injection site, and also by stimulating the innate immune system. These adjuvants have been used in vaccines such as the H1N1 influenza vaccine and the human papillomavirus (HPV) vaccine. In one study, the CoronaVac inactivated vaccine with alum adjuvant demonstrated highly effective neutralizing effects against SARS-CoV-2.104
Live-attenuated-virus vaccine
Live-attenuated virus vaccines have been a successful immunization strategy due to their ability to rapidly expand in the host system after injection, resulting in effective immunization.49,105 Live-attenuated virus vaccines are weakened forms of the virus that are able to replicate within the body but do not cause disease. As such, they can elicit a strong and long-lasting immune response. Adjuvants are not typically used with live-attenuated virus vaccines because the vaccine itself is already a strong stimulant of the immune system. However, some studies have explored the use of adjuvants with live-attenuated virus vaccines to potentially enhance their effectiveness. One example is the use of the oil-in-water emulsion MF59 as an adjuvant with a live-attenuated influenza vaccine, which has been shown to improve the immune response in elderly individuals.36 However, more research is needed to determine the safety and effectiveness of adjuvants with live-attenuated virus vaccines.
Viral vector vaccine
Replication competent and incompetent adenoviral vectors have been commonly used to express the antigens of other viral proteins for immunization.49,106 Adjuvants can be used in conjunction with viral vector vaccines to enhance the immune response generated by the vaccine. For example, the Oxford-AstraZeneca COVID-19 vaccine is a viral vector vaccine that uses a weakened version of a chimpanzee adenovirus to deliver the genetic material for the SARS-CoV-2 spike protein.107 This vaccine contains an adjuvant called AS03, which is a combination of squalene (a natural oil), polysorbate 80 (a detergent), and vitamin E.
Virus-like particles vaccine
In this strategy, structural viral proteins are co-expressed to form non-infectious particles as the vaccine immunogen. They resemble real virions but they lack the virus genome. Production of VLPs in the cells with further reconstruction into the stable and immunogenic forms is a multi-stage process. Adjuvants can be used in virus-like particles vaccines to enhance the immune response and improve vaccine efficacy.32,69,70 Commonly used adjuvants for virus-like particles vaccines include aluminum salts, MF59 (an oil-in-water emulsion), and CpG oligonucleotides (TLR-9 agonists). These adjuvants stimulate the immune system to produce a stronger and more long-lasting response to the VLP vaccine. In some cases, the VLP itself can act as an adjuvant, as it has been shown to stimulate the immune system through multiple pathways. Overall, the use of adjuvants in VLP vaccines can help to increase their potency, reduce the required dose, and improve vaccine coverage.
DNA vaccines
DNA-based vaccines utilize DNA plasmids as a vector to transfer genes encoding an antigen into host cells, specifically antigen-presenting cells.106,108,109 The underlying mechanism of these vaccines relies on the delivery of genetic material to the cell nucleus, activating the mammalian promoter in the vector and initiating transcription of the target genes through the host cellular mechanism. However, DNA vaccines often have limited immunogenicity compared to other vaccine types, which can limit their effectiveness. Adjuvants can help to overcome this limitation by enhancing the immune response to the encoded antigen. Various adjuvants have been tested in DNA vaccines, including alum, CpG oligodeoxynucleotides (CpG ODNs), and liposomes. Alum has been shown to enhance the immune response to DNA vaccines in mice, but has had limited success in human trials. CpG ODNs are synthetic molecules that mimic bacterial DNA, and can activate the immune system by interacting with toll-like receptors (TLRs) on immune cells. Liposomes are small lipid vesicles that can encapsulate the DNA and adjuvant, and deliver them directly to antigen-presenting cells. Liposome-based adjuvants have shown promise in preclinical studies of DNA vaccines.
mRNA vaccines
mRNA vaccines involve direct injection of the mRNA molecule into the host cell, which is then translated into the target protein in the cytoplasm.110–112 These vaccines typically contain an open reading frame (ORF) with a 3′ poly-adenylated tail that can stimulate both cellular and humoral immune responses. Compared to conventional vaccines, mRNA vaccines offer numerous advantages, such as safety, flexibility, scalability, and cost-effectiveness. mRNA vaccines do not typically require adjuvants as the mRNA molecules themselves stimulate the immune system. The mRNA vaccines against COVID-19, for example, use a lipid nanoparticle delivery system to protect the mRNA and facilitate its entry into cells, but this delivery system is not considered an adjuvant in the traditional sense. However, some research is being conducted to explore the use of adjuvants in mRNA vaccines to enhance their effectiveness, especially for certain populations that may have weaker immune responses to the vaccines, such as the elderly.
Protein subunit-based vaccine
Protein subunit-based vaccines contain only a specific protein or proteins from a pathogen, rather than the entire pathogen.2,113,114 Adjuvants are often used with protein subunit vaccines to enhance their effectiveness by increasing the immune response to the protein antigen. Aluminum salts (such as aluminum hydroxide) are the most commonly used adjuvants for protein subunit-based vaccines, as they have been shown to enhance the antibody response to the antigen. Other adjuvants, such as MF59 (oil-in-water emulsion), AS03 (squalene oil-in-water emulsion), and AS04 (aluminum hydroxide and MPL), have also been used in protein subunit-based vaccines to improve their immunogenicity. Additionally, novel adjuvants, such as toll-like receptor agonists, are being studied for use with protein subunit-based vaccines to further enhance their immune response.
Clinical trials and licensing of vaccine adjuvants
Developing a vaccine with an adjuvant is a difficult task that can take years as it is important to ensure that each component of the vaccine is compatible both alone and in combination. The Center for Biologics Evaluation and Research (CBER), a division of the US Food and Drug Administration, has released a guide to facilitate the development of new vaccine formulations in response to the urgent need to develop vaccines against infectious diseases (Fig. 6).10,71,115 The guide recommends starting with preclinical tests using an appropriate animal model to evaluate the safety and immunogenicity of the formulation, including the adjuvant effect on the immune response. It is essential to include control groups consisting of adjuvant and antigen alone to provide evidence for the adjuvant effect. Immunogenicity evaluation may include humoral and cellular responses, and initial protective efficacy information can be obtained if an animal model for the disease is available. After preclinical testing and good manufacturing practice (GMP) production of the vaccine formulation, human clinical trials begin.
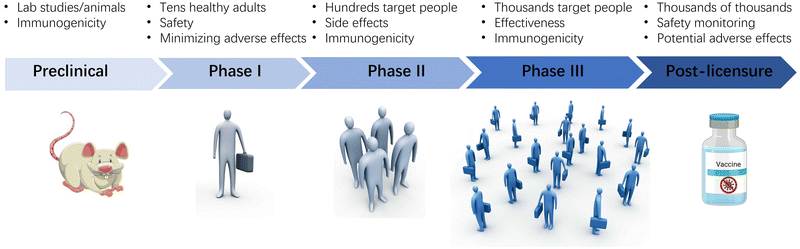 |
| Fig. 6 The evolving stages of vaccine development. | |
Phase I studies evaluate safety and dosage in healthy individuals, with initial immunogenicity information obtained. Phase IIa trials involve hundreds of volunteers to evaluate immunogenicity, tolerability, and safety. When tests reach phases IIb/III, the focus is on ascertaining immunogenicity and efficacy in the vaccine target population, with the study duration increasing as the number of volunteers involved increases.
After confirming the safety and efficacy of the vaccine, it can be licensed and marketed. However, the formulation undergoes postmarket safety monitoring in phase IV to evaluate additional rare adverse reactions. These activities aim to ensure that appropriate actions are taken based on the results of safety monitoring. Regulatory authorities rigorously apply pharmacovigilance processes to evaluate potential safety signals that arise after licensure. However, evaluating studies attempting to establish a causal association with vaccination can be complex and inconclusive, despite the wealth of safety information that comes after licensure.
There have been instances in which vaccines have been withdrawn after licensure due to demonstrated causal associations between the vaccine and adverse events, or due to poor efficacy that changes the benefit-risk ratio. While there have been examples where alleged causal relationships between vaccination and adverse events have been shown to be unfounded. Vaccine manufacturers and regulatory agencies take prompt actions when evidence arises from safety signal monitoring or new important adverse events are reported. They communicate the evidence rapidly to healthcare professionals and the public to allow informed decision-making about the most current benefit-risk profile.
Clinical trials examples of vaccine adjuvants
Emulsions.
MF59 is an example for the development of a vaccine eliciting HIV-specific humoral and cellular immune responses, which is desirable for the prevention of HIV infection.36 A combination vaccine regimen consisting of priming with DNA followed by boosting with a recombinant envelope glycoprotein adjuvanted in MF59 has been investigated in the phase I clinical trial to study the safety of and immune response to a combination HIV vaccine regimen in HIV uninfected adults.116 Besides, inactivated influenza A/H9N2 vaccine with and without MF59 adjuvant in ambulatory adults are in the in the phase 2 clinical trial to assess the safety of 4 different dosages of purified monovalent surface antigen (SA) influenza A/H9N2 virus vaccine with or without MF59 adjuvant and administered intramuscularly to ambulatory adults.117 Montanide ISA 51 and Montanide ISA 720 are two examples of water-in-oil emulsion adjuvants that have been investigated in clinical studies. They have different oil to water ratios.118 Montanide ISA 51 has been assessed in malaria vaccines in phase 1 and influenza vaccines in phase 2 studies, and it has been licensed in a therapeutic lung cancer vaccine in Cuba. Montanide ISA 720 has been tested in a phase 2 clinical trial for a malaria vaccine. Both adjuvants have been shown to promote effective immune responses, particularly enhanced specific cytotoxic T-lymphocyte (CTL) responses. However, transient side effects have been observed, such as fever, headache, or flu-like symptoms, although both appear to be well-tolerated. AS03 adjuvant in sanofi H1N1 influenza vaccine administered at different dose levels in healthy adult and elderly populations to see how the body reacts to different strengths of the H1N1 flu shot when it is given with or without an “adjuvant.” This study will also compare how age affects the body's response to the H1N1 flu shot.119
Aluminum-based adjuvant.
In a phase 1 clinical trial, the safety, tolerability, and immunogenicity of a single dose of Merck 0657nI staphylococcus aureus vaccine with or without merck aluminum adjuvant has been evaluated. Besides, to extend the evaluation of safety and immunogenicity of MN recombinant soluble gp120/HIV-1 (MN rsgp120/HIV-1) in combination with QS21 with or without alum has been evaluated in a phase 1 trial.120
Lipid-based adjuvants.
Lipid-based material has been used as a vaccine adjuvant since its unique vesicle structure was found to be extremely suitable for encapsulating various biologically active materials, such as DNA and fatty acids.90,121 At the early stage, scientists focused on Lipid A, the hydrophobic anchor of LPS, which was believed to be a potent adjuvant that could significantly increase both the humoral and cellular immune response. However, Lipid-A based adjuvant was not considered suitable for human vaccines due to its high toxicity. Instead, GLA-SE and GLA-AF, which are both Lipid A analogues, have been found to be particularly effective in enhancing protection against H5N1 with low toxicity and limited side effects. GLA-AF has also been tested as an alternative to emulsions in many vaccines, most notably in pandemic influenza.122
TLR agonist-based.
TLR3 agonist poly-ICLC has been studied for safety study of adjuvant vaccine to treat Melanoma patients. This is a Phase I open label dose escalation study of the TLR3 agonist poly-ICLC as an adjuvant for NY-ESO-1 protein vaccination in patients with high risk melanoma. The goal of adjuvant vaccine therapy is to train the endogenous immune system to recognize and target minimal residual disease.123
Saponin.
Saponin-based OPT-821 has been used in trivalent ganglioside in aetastatic aarcoma patients who are rendered disease free in clinical phase 3 trial. If the trivalent vaccine can stimulate the patient's immune system to develop antibodies which recognize and target the GM2, GD2 and GM3 sugars, then the patient's antibodies could attack and kill any remaining sarcoma cells potentially preventing the recurrence of sarcoma.124,125 MatrixMTM has also been tested in phase 1 and phase 2 clinical trials for pandemic influenza and RSV vaccines, and has shown to be safe and well tolerated, with enhanced humoral and cellular immune responses. Other saponin-based adjuvants, such as QS-21, have been evaluated in clinical trials for cancer vaccines and infectious disease vaccines, and have shown to induce strong immune responses and improve vaccine efficacy.126 However, their complex structure and variable composition may affect their consistency and reproducibility, and their potential toxicity and hemolytic activity need to be carefully evaluated in preclinical and clinical studies in dLNs. AS02, composing of MPL and QS-21, is undergoing phase 2 trial in a malaria vaccine.
Nucleotide.
Adjuvant components such as CpG ODN, dsRNA, and IL-12 DNA are examples of nucleotide-based adjuvants that activate PRRs to stimulate the immune response.65,66 For instance, dsRNA can secrete cytokines like IFN-a and IFN-c, signal through TLR3, MDA-5, and NLRP3 inflammasome, and is currently undergoing clinical trials in influenza and rabies vaccines.127 DNA vaccines adjuvanted with IL-12 DNA are introduced to provide efficient protection against a wide range of HIV variants, and recent trials have shown that they are well tolerated and can enhance the immune response.128 Pika adjuvant is another nucleotide-based adjuvant that is a synthetic analogue of dsRNA stabilized with kanamycin and calcium, which promotes the production of interferon, IL-2, and IL-12. This adjuvant can transform preventive vaccines that mainly produce humoral immunity into therapeutic vaccines with strong cellular immunity.129
Cytokines.
In recent research, molecular adjuvants have been shown to effectively stimulate immune response, particularly in subunit vaccines. Cytokines such as IL-2 and GM-CSF have been studied in vaccines targeting foot and mouth disease, and GM-CSF is currently being evaluated for use in Hepatitis B and HIV vaccines. Its adjuvant effect is attributed to its capacity to promote macrophage differentiation and proliferation, and activate antigen presenting cells.130 Similarly, IL-12 and IL-15 have been employed as adjuvants in an HIV vaccine undergoing phase 1 clinical trial. These cytokines have demonstrated the ability to stimulate both NK cells and T-cell proliferation.
Virosomes, which are enveloped virus-like particles, have been licensed in Europe for use in vaccines against Hepatitis A and influenza and have been used in clinical trials for Falciparum Malaria and Chronic Hepatitis C vaccine.131
Peptide, MUC1 – poly-ICLC vaccine is being tested in a phase II trial designed to assess antibody and T cell responses to MUC1 vaccine among subjects at increased risk for colorectal cancer by virtue of a history of advanced adenoma. The MUC1 – poly-ICLC vaccine is being developed to prevent polyps from advancing into colon cancer and to prevent polyps from recurring, which help the immune system in the body identify the changes in MUC1 that accompany the progression to cancer and eliminate the abnormal cells that make abnormal MUC1.132
Other.
Other forms of adjuvants are also being investigated in clinical trials. A phase I clinical study for an investigational PIKA (polyinosinic–polycytidylic acid based adjuvant) rabies vaccine comprising inactivated and purified rabies virus and the PIKA adjuvant was to assess the clinical safety of the vaccine composition in healthy adult volunteers and to evaluate the vaccine's efficacy based on an accelerated vaccine regimen.133 Mayo Clinic in conjunction with Agri-King Corporation has developed a novel synbiotic called AKSB (Agri-King Synbiotic). A phase I placebo-controlled trial of AKSB in normal human volunteers, over 65 years of age, is designed to study the safety of this probiotic when patients are also receiving an influenza vaccine.134 Calcium phosphate, in the form of hydroxyapatite, has been licensed for use in vaccines for diphtheria, tetanus, pertussis, and poliomyelitis in France. Calcium phosphate is believed to be a natural compound in the human body and well-tolerated, making it a potential substitute for aluminum adjuvants which can have undesirable side effects.135 Bacterial flagellin, a ligand for TLR5, has also shown adjuvant effects that lead to mixed Th1 and Th2 responses. In a clinical trial, a bivalent P. aeruginosa flagella adjuvanted vaccine helped patients with cystic fibrosis lower their risk for infection with P. aeruginosa.136 Polysaccharides are another potential adjuvant that can enhance the immune response of vaccines and promote cellular immunity, humoral immunity, and mucosal immunity. There are also novel adjuvants that combine several types of adjuvants listed above, with the effective components synergizing to create optimal immune responses.64
Vaccine adjuvants against COVID-19
The COVID-19 pandemic has highlighted the need for a safe and effective vaccine to control the spread of the SARS-CoV-2 virus. The development of an ideal vaccine requires extensive research and resources to ensure that it meets the demands of the current situation. Vaccines aim to induce a protective immune response that can prevent or reduce the severity of a viral infection.137–141
Benefits of adjuvants in COVID-19 vaccines
There are several reasons/motivations using adjuvants in COVID-19 vaccines: (1) one of the main requirements of an effective COVID-19 vaccine is its ability to stimulate a robust immune response that can produce high levels of neutralizing antibodies. To achieve this, a vaccine may require multiple doses or adjuvants. Adjuvants can help to reduce the amount of antigen needed in the vaccine and, therefore, reduce its cost. (2) Incorporating a suitable adjuvant in a SARS-CoV-2 vaccine can address these requirements by reducing the amount of antigen needed to produce a protective immune response. The vaccine's production and distribution should be cost-effective and scalable to meet the overwhelming demand. With the pandemic affecting most countries worldwide, there is an immense need for a vaccine that can be produced and distributed quickly and efficiently. (3) Furthermore, adjuvants can also enhance the vaccine's efficacy by providing a prolonged immune response and broadening its range of protection against different strains of the virus. Therefore, research and development of adjuvanted vaccines against COVID-19 are crucial to develop a safe, effective, and cost-effective vaccine to control the pandemic.103,106,137–140,142–147
Licensed adjuvants and candidates for COVID-19
NVX-CoV2373 is a promising vaccine candidate developed by Novavax for the prevention of COVID-19. It is a protein-based vaccine that is created using the genetic sequence of the first strain of SARS-CoV-2, the virus that causes COVID-19.147 The S protein is a key target for COVID-19 vaccines because it is the primary means by which the virus attaches to and infects human cells. To enhance the immune response and stimulate high levels of neutralizing antibodies, NVX-CoV2373 is formulated with Novavax's patented saponin-based Matrix-M™ adjuvant.126 This adjuvant has been extensively tested and has demonstrated a potent and well-tolerated effect by stimulating the entry of antigen-presenting cells into the injection site and enhancing antigen presentation in local lymph nodes, thereby boosting the immune response. This protein-based vaccine candidate is designed to teach the immune system how to recognize and fight the SARS-CoV-2 virus, providing protection against COVID-19. Clinical trials have shown that NVX-CoV2373 is highly effective in preventing COVID-19, with an overall efficacy rate of over 90%. After being approved, NVX-CoV2373 could play a critical role in the fight against COVID-19, particularly in areas where other vaccines may not be as readily available or effective. Several other COVID-19 vaccines have been authorized for emergency use around the world, and some of them utilize adjuvants. For examples, AstraZeneca COVID-19 vaccine uses an adjuvant MF59, which is an oil-in-water emulsion of squalene oil to enhance the immune response to the vaccine. Sinovac COVID-19 vaccine uses an adjuvant alum, which is an aluminum salt that helps to enhance the immune response.107
Emerging developments of new vaccine adjuvants
Design principles of novel adjuvants
In order to develop effective adjuvant vaccines, it is important to understand how adjuvants work and follow some design principles. The problem-oriented principle involves considering four main issues: (1) the required immune response to prevent disease from a specific pathogen, (2) the relevant innate immune cells that can induce the required immune response, (3) the localization of these innate cell subsets within the body, and (4) the expression of pattern recognition receptors (PRRs) on these cells. Based on these issues, four steps need to be considered when designing a vaccine adjuvant.6,9,13,67,68,106 Firstly, the type of immune effector elements needed for vaccine efficacy in the host should be identified. This should take into account the antigen type, target cell subsets and phenotype, and immunization routes to guide the choice of delivery system and immune stimulators. As PRRs are key targets for producing effective vaccine generation, it is important to evaluate the localization of relevant innate immune cells within the body and PRR expression on target cells in designing vaccine adjuvants. Different PRR signaling pathways may be used to develop customized responses to different aggressions. Secondly, the identification of a suitable vaccine antigen should be based on an understanding of the molecular mechanisms of immune recognition and protection. The suitable antigen should also enable targeting of the vaccine-induced response within the intended target population. In addition, the vaccine must present sufficient amounts of the right antigen in the right conformation to the appropriate cell populations to induce the immune response protection, while considering the tolerability and safety ramifications of the induced inflammatory response. Thirdly, adjuvants need a delivery system, whether synthetic or natural. This requires the design of an appropriate delivery mode of the antigen of interest, in combination with relevant immune stimulators. Fourthly, the preparation process of both vaccines and adjuvants should be simple. For example, if no special emulsifier is required, the production process is simpler. If the ratio of adjuvant to antigen is low, it may increase the scope for vaccine improvement.
Adjuvant formulations for new vaccines
Several formulation approaches have already been established for adjuvants included in licensed products.12,69,148 These include insoluble aluminum salts used as an adsorbent, low oil content that is easy to inject, oil-in-water emulsions, and liposomal delivery systems. While compositionally different, these systems share some significant similarities, as they are all fabricated as nanoparticles. Additionally, these systems can function as effective “delivery systems” for both antigens and immune potentiators, including TLR agonists. The proper selection of both the immunostimulant and formulation components of an adjuvant is crucial for inducing an appropriate immune response tailored to control the target pathogen.149 Different formulations of the same immunomodulatory molecules may induce substantially different immune responses, as demonstrated in the malaria vaccine program. For example, the RTS,S vaccine candidate formulated with AS02 protected six out of seven vaccine recipients from infection, whereas the same antigen with AS03 or AS04 protected only two out of seven or one out of eight recipients, respectively.150 To build improved adjuvants, it is essential to ensure that each component is necessary and adds clear value, while not introducing significant unjustified liabilities. AS01 is currently the most successful adjuvant included in a licensed product, with efficacy >97% against varicella zoster.151 The development of the AS01 adjuvant was based on the insight that the two key components, MPL and QS-21, resulted in a synergy of innate activation when used together. This synergy could not be achieved with either molecule alone, and a liposomal delivery system was developed to co-deliver the two immune potentiators to the same immune cell populations. Additionally, the liposomal formulation was effective in reducing the potential of QS-21 to induce significant local and systemic reactogenicity. However, novel system vaccinology approaches could be used to identify novel adjuvant pathways and formulations that could be harnessed to design the next generation of adjuvants.
Advancing systems vaccinology
Bali et al.9 proposed an approach for accelerating adjuvant discovery and development in the clinic is an interdisciplinary approach based on systems vaccinology, which involves the early use of human studies to generate ‘omics’ data that can be used to formulate novel hypotheses about the mechanisms by which candidate adjuvants stimulate robust and durable antigen-specific T and B cell responses.9 These hypotheses can then be retested in animal models, and the mechanistic insights that ensue can be used to design novel adjuvant concepts. The proposed framework for adjuvant development places greater emphasis on testing many potential adjuvant concepts in small clinical trials (phase 0/I) early in their developmental pipeline, which can rapidly test novel adjuvants and obtain mechanistic insights using systems vaccinology approaches. Additionally, systems vaccinology approaches can be used to define not only the mechanisms of action of adjuvants but also the underlying mechanisms by which formulations work, the underlying mechanisms of adverse reactions that occur soon after vaccination, and the rational design of optimal formulations for vaccine delivery. Overall, this interdisciplinary approach based on systems vaccinology has the potential to transform the science of adjuvants and revitalize the development of novel adjuvants for use in vaccines.
Non-invasive vaccine delivery
Research on non-invasive vaccine delivery is a key focus that could have a significant impact on global mass vaccination campaigns.145,152–154 Developing safe, effective, and low-cost vaccine formulations with adjuvants that result in desired immune responses and long-term immunity is a priority. Extensive research has already led to the development of several novel adjuvants that are currently being evaluated in clinical trials or licensed. One area of focus has been on developing lipid-containing adjuvants that are safe and improve vaccine efficacy for non-invasive delivery. However, more information is needed regarding the interactions between cell surface receptors after stimulation by adjuvant molecules and the compatibility of these adjuvants with existing or novel formulations. As our understanding of the molecular mechanisms involved in immune protection improves and new methods in synthetic chemistry are developed, breakthroughs in vaccine development are anticipated. Novel technologies, such as new glycol-conjugation methods, reverse vaccinology, and next-generation sequencing techniques, should lead to novel vaccine strategies for diseases such as HBV, pertussis toxin, Lyme borreliosis, and HPV, with or without adjuvants. The research community aims to ensure high levels of broad protection by introducing new immunostimulants and novel delivery systems that combine efficacy with long-lasting memory immune responsiveness and safety. This new approach will no longer rely solely on structural vaccinology due to the variability of target epitopes and the constant emergence of new pathogens and tumor antigens.
Conclusion and perspectives
Adjuvants have played a crucial role in vaccine development for nearly a century, enhancing immunogenicity. Initially, adjuvant selection was largely empirical, but recent advancements have led to a more targeted and rational approach. A deeper understanding of the immune system has enabled the development of more effective vaccine formulations. This review provided an overview of adjuvants used in vaccines, ranging from traditional to COVID-19 vaccines, focusing on their types, mechanisms of action on immune responses, and their role in vaccine formulations. These insights lay the groundwork for selecting suitable adjuvants for both traditional and coronavirus vaccines.
Understanding the impact of various adjuvants on immune responses, their synergies with different antigen types and vaccine platforms, and comprehensive analyses of these adjuvants will aid in choosing those that offer the necessary immunological protection. Adjuvants like aluminum-based salts, TLR agonists, emulsions, and other novel options have unique physicochemical properties that significantly influence the strength, duration, and type of immune responses. Such novel adjuvants have already enhanced influenza vaccines and those for hepatitis B and HPV.
With the ongoing challenge of emerging diseases such as COVID-19 and the search for more definitive cures, the development of safe and effective vaccines is imperative. A more informed selection of adjuvants and antigens could not only enhance protection for populations that respond poorly to traditional vaccines but also open new avenues beyond preventive applications. This approach could be pivotal in addressing current and future global health challenges.
Conflicts of interest
The authors declare no competing financial interests.
Acknowledgements
Y. S. acknowledges support from the National Institutes of Health under grant no. R01AI143287. Y. H. acknowledges support from the National Institute of General Medical Sciences under grant no. R35GM147391.
References
- A. Facciolà, G. Visalli, A. Laganà and A. Di Pietro, An Overview of Vaccine Adjuvants: Current Evidence and Future Perspectives, Vaccines, 2022, 10, 819 CrossRef PubMed
.
-
F. Z. Firdaus, M. Skwarczynski and I. Toth, Developments in Vaccine AdjuvantsAdjuvants, in Vaccine Design: Methods and Protocols, Volume 3. Resources for Vaccine Development, ed. S. Thomas, Springer, US, 2022, pp. 145–178 Search PubMed
.
-
R. N. Lodaya, S. Gregory, M. M. Amiji and D. T. O'Hagan, 2 – Overview of vaccine adjuvants, in Practical Aspects of Vaccine Development, ed. P. Kolhe and S. Ohtake, Academic Press, 2022, pp. 9–25 Search PubMed
.
-
D. L. Novicki, Introduction to Vaccines and Adjuvants, in Nonclinical Development of Novel Biologics, Biosimilars, Vaccines and Specialty Biologics, ed. L. M. Plitnick and D. J. Herzyk, Academic Press, 2013, ch. 8, pp. 213–224 Search PubMed
.
- D. T. O'Hagan and N. M. Valiante, Recent advances in the discovery and delivery of vaccine adjuvants, Nat. Rev. Drug Discovery, 2003, 2(9), 727–735, DOI:10.1038/nrd1176
.
- D. T. O’Hagan, R. N. Lodaya and G. Lofano, The continued advance of vaccine adjuvants – ‘we can work it out’, Semin. Immunol., 2020, 50, 101426, DOI:10.1016/j.smim.2020.101426
.
- A. D. Pasquale, S. Preiss, F. T. D. Silva and N. Garçon, Vaccine Adjuvants: from 1920 to 2015 and Beyond, Vaccines, 2015, 3(2), 320–343 CrossRef PubMed
.
- C. Pifferi, R. Fuentes and A. Fernández-Tejada, Natural and synthetic carbohydrate-based vaccine adjuvants and their mechanisms of action, Nat. Rev. Chem., 2021, 5(3), 197–216, DOI:10.1038/s41570-020-00244-3
.
- B. Pulendran, P. S. Arunachalam and D. T. O’Hagan, Emerging concepts in the science of vaccine adjuvants, Nat. Rev. Drug Discovery, 2021, 20(6), 454–475, DOI:10.1038/s41573-021-00163-y
.
- S. G. Reed, M. T. Orr and C. B. Fox, Key roles of adjuvants in modern vaccines, Nat. Med., 2013, 19(12), 1597–1608, DOI:10.1038/nm.3409
.
-
R. R. Shah, K. J. Hassett and L. A. Brito, Overview of Vaccine Adjuvants: Introduction, History, and Current Status. in Vaccine Adjuvants: Methods and Protocols, ed. C. B. Fox, Springer, New York, 2017, pp. 1–13 Search PubMed
.
- S. Shi, H. Zhu, X. Xia, Z. Liang, X. Ma and B. Sun, Vaccine adjuvants: Understanding the structure and mechanism of adjuvanticity, Vaccine, 2019, 37(24), 3167–3178, DOI:10.1016/j.vaccine.2019.04.055
.
- M. Singh and D. O'Hagan, Advances in vaccine adjuvants, Nat. Biotechnol., 1999, 17(11), 1075–1081, DOI:10.1038/15058
.
- S. K. Verma, P. Mahajan, N. K. Singh, A. Gupta, R. Aggarwal, R. Rappuoli and A. K. Johri, New-age vaccine adjuvants, their development, and future perspective, Front. Immunol., 2023, 14, 1043109, DOI:10.3389/fimmu.2023.1043109
.
- J. C. Aguilar and E. G. Rodríguez, Vaccine adjuvants revisited, Vaccine, 2007, 25(19), 3752–3762, DOI:10.1016/j.vaccine.2007.01.111
.
- L. A. Brito and D. T. O'Hagan, Designing and building the next generation of improved vaccine adjuvants, J. Controlled Release, 2014, 190, 563–579, DOI:10.1016/j.jconrel.2014.06.027
.
- R. L. Coffman, A. Sher and R. A. Seder, Vaccine Adjuvants: Putting Innate Immunity to Work, Immunity, 2010, 33(4), 492–503, DOI:10.1016/j.immuni.2010.10.002
.
- S. Lee and M. T. Nguyen, Recent Advances of Vaccine Adjuvants for Infectious Diseases, Immune Netw., 2015, 15(2), 51–57, DOI:10.4110/in.2015.15.2.51
.
- E. De Gregorio, E. Caproni and J. Ulmer, Vaccine Adjuvants: Mode of Action, Front. Immunol., 2013, 4, 214, DOI:10.3389/fimmu.2013.00214
.
- M. Singh and D. T. O'Hagan, Recent Advances in Vaccine Adjuvants, Pharm. Res., 2002, 19(6), 715–728, DOI:10.1023/A:1016104910582
.
- A. S. McKee, M. W. Munks and P. Marrack, How Do Adjuvants Work? Important Considerations for New Generation Adjuvants, Immunity, 2007, 27(5), 687–690, DOI:10.1016/j.immuni.2007.11.003
.
- D. T. O’Hagan and C. B. Fox, New generation adjuvants – From empiricism to rational design, Vaccine, 2015, 33, B14–B20, DOI:10.1016/j.vaccine.2015.01.088
.
- N. W. Baylor, W. Egan and P. Richman, Aluminum salts in vaccines—US perspective, Vaccine, 2002, 20, S18–S23, DOI:10.1016/S0264-410X(02)00166-4
.
- T. Clapp, P. Siebert, D. Chen and L. Jones Braun, Vaccines with Aluminum-containing Adjuvants: Optimizing Vaccine Efficacy and Thermal Stability, J. Pharm. Sci., 2011, 100(2), 388–401, DOI:10.1002/jps.22284
.
-
R. K. Gupta, B. E. Rost, E. Relyveld and G. R. Siber, Adjuvant Properties of Aluminum and Calcium Compounds. in Vaccine Design: The Subunit and Adjuvant Approach, ed. M. F. Powell and M. J. Newman, Springer, US, 1995, pp. 229–248 Search PubMed
.
- P. He, Y. Zou and Z. Hu, Advances in aluminum hydroxide-based adjuvant research and its mechanism, Hum. Vaccines Immunother., 2015, 11(2), 477–488, DOI:10.1080/21645515.2014.1004026
.
- S. L. Hem, Elimination of aluminum adjuvants, Vaccine, 2002, 20, S40–S43, DOI:10.1016/S0264-410X(02)00170-6
.
- S. L. Hem and H. HogenEsch, Relationship between physical and chemical properties of aluminum-containing adjuvants and immunopotentiation, Expert Rev. Vaccines, 2007, 6(5), 685–698, DOI:10.1586/14760584.6.5.685
.
- H. HogenEsch, Mechanism of Immunopotentiation and Safety of Aluminum Adjuvants, Front. Immunol., 2013, 3, 406, DOI:10.3389/fimmu.2012.00406
.
- H. HogenEsch, Mechanisms of stimulation of the immune response by aluminum adjuvants, Vaccine, 2002, 20, S34–S39, DOI:10.1016/S0264-410X(02)00169-X
.
- H. HogenEsch, D. T. O’Hagan and C. B. Fox, Optimizing the utilization of aluminum adjuvants in vaccines: you might just get what you want, npj Vaccines, 2018, 3(1), 51, DOI:10.1038/s41541-018-0089-x
.
- Z. Liang, Y. Yang, G. Yu, H. Zhu, X. Xia, C. Chen, D. Fu, M. Li, G. Cheng and C. Xue,
et al., Engineering aluminum hydroxyphosphate nanoparticles with well-controlled surface property to enhance humoral immune responses as vaccine adjuvants, Biomaterials, 2021, 275, 120960, DOI:10.1016/j.biomaterials.2021.120960
.
-
E. B. Lindblad and N. E. Schønberg, Aluminum Adjuvants: Preparation, Application, Dosage, and Formulation with Antigen, in Vaccine Adjuvants: Methods and Protocols, ed. G. Davies, Humana Press, 2010, pp. 41–58 Search PubMed
.
- F. Lu and H. HogenEsch, Kinetics of the inflammatory response following intramuscular injection of aluminum adjuvant, Vaccine, 2013, 31(37), 3979–3986, DOI:10.1016/j.vaccine.2013.05.107
.
- A. C. Allison, Squalene and Squalane Emulsions as Adjuvants, Methods, 1999, 19(1), 87–93, DOI:10.1006/meth.1999.0832
.
- S. Calabro, E. Tritto, A. Pezzotti, M. Taccone, A. Muzzi, S. Bertholet, E. De Gregorio, D. T. O’Hagan, B. Baudner and A. Seubert, The adjuvant effect of MF59 is due to the oil-in-water emulsion formulation, none of the individual components induce a comparable adjuvant effect, Vaccine, 2013, 31(33), 3363–3369, DOI:10.1016/j.vaccine.2013.05.007
.
- C. B. Fox and J. Haensler, An update on safety and immunogenicity of vaccines containing emulsion-based adjuvants, Expert Rev. Vaccines, 2013, 12(7), 747–758, DOI:10.1586/14760584.2013.811188
.
- R. K. Gupta, E. H. Relyveld, E. B. Lindblad, B. Bizzini, S. Ben-Efraim and C. K. Gupta, Adjuvants
– a balance between toxicity and adjuvanticity, Vaccine, 1993, 11(3), 293–306, DOI:10.1016/0264-410X(93)90190-9
.
- Y.-J. Lin, C.-N. Wen, Y.-Y. Lin, W.-C. Hsieh, C.-C. Chang, Y.-H. Chen, C.-H. Hsu, Y.-J. Shih, C.-H. Chen and C.-T. Fang, Oil-in-water emulsion adjuvants for pediatric influenza vaccines: a systematic review and meta-analysis, Nat. Commun., 2020, 11(1), 315, DOI:10.1038/s41467-019-14230-x
.
- S. Martiñón, A. Cisneros, S. Villicaña, R. Hernández-Miramontes, E. Mixcoha and P. Calderón-Vargas, Chemical and Immunological Characteristics of Aluminum-Based, Oil–Water Emulsion, and Bacterial-Origin Adjuvants, J. Immunol. Res., 2019, 2019, 3974127, DOI:10.1155/2019/3974127
.
- D. T. O'Hagan, T. F. Tsai and L. A. Brito, Emulsion based vaccine adjuvants, Hum. Vaccines Immunother., 2013, 9(8), 1698–1700, DOI:10.4161/hv.24829
.
- D. T. O’Hagan, R. van der Most, R. N. Lodaya, M. Coccia and G. Lofano, “World in motion” – emulsion adjuvants rising to meet the pandemic challenges, npj Vaccines, 2021, 6(1), 158, DOI:10.1038/s41541-021-00418-0
.
- C. Robson, S. R. Baskar, R. Booy, P. E. Ferguson, N. Gilroy, J. Kok, I. Sandaradura and D. Dwyer, Influenza: overview on prevention and therapy, Aust. Prescr., 2019, 42(2), 51–55, DOI:10.18773/austprescr.2019.013
.
- T. F. Tsai, Fluad®-MF59®-Adjuvanted Influenza Vaccine in Older Adults, Infect. Chemother., 2013, 45(2), 159–174, DOI:10.3947/ic.2013.45.2.159
.
- C. Cohet, R. van der Most, V. Bauchau, R. Bekkat-Berkani, T. M. Doherty, A. Schuind, F. Tavares Da Silva, R. Rappuoli, N. Garçon and B. L. Innis, Safety of AS03-adjuvanted influenza vaccines: A review of the evidence, Vaccine, 2019, 37(23), 3006–3021, DOI:10.1016/j.vaccine.2019.04.048
.
- N. Garçon, D. W. Vaughn and A. M. Didierlaurent, Development and evaluation of AS03, an Adjuvant System containing α-tocopherol and squalene in an oil-in-water emulsion, Expert Rev. Vaccines, 2012, 11(3), 349–366, DOI:10.1586/erv.11.192
.
- S. Morel, A. Didierlaurent, P. Bourguignon, S. Delhaye, B. Baras, V. Jacob, C. Planty, A. Elouahabi, P. Harvengt and H. Carlsen,
et al., Adjuvant System AS03 containing α-tocopherol modulates innate immune response and leads to improved adaptive immunity, Vaccine, 2011, 29(13), 2461–2473, DOI:10.1016/j.vaccine.2011.01.011
.
- A. M. Didierlaurent, B. Laupèze, A. Di Pasquale, N. Hergli, C. Collignon and N. Garçon, Adjuvant system AS01: helping to overcome the challenges of modern vaccines, Expert Rev. Vaccines, 2017, 16(1), 55–63, DOI:10.1080/14760584.2016.1213632
.
- J. O. Josefsberg and B. Buckland, Vaccine process technology, Biotechnol. Bioeng., 2012, 109(6), 1443–1460, DOI:10.1002/bit.24493
.
- L. Chedid, F. Audibert, P. Lefrancier, J. Choay and E. Lederer, Modulation of the immune response by a synthetic adjuvant and analogs, Proc. Natl. Acad. Sci. U. S. A., 1976, 73(7), 2472–2475, DOI:10.1073/pnas.73.7.2472
.
- G. Leroux-Roels, Unmet needs in modern vaccinology: Adjuvants to improve the immune response, Vaccine, 2010, 28, C25–C36, DOI:10.1016/j.vaccine.2010.07.021
.
- Z. I. Rajput, S.-h Hu, C.-w Xiao and A. G. Arijo, Adjuvant effects of saponins on animal immune responses, J. Zhejiang Univ., Sci., B, 2007, 8(3), 153–161, DOI:10.1631/jzus.2007.B0153
.
- A. Pashine, N. M. Valiante and J. B. Ulmer, Targeting the innate immune response with improved vaccine adjuvants, Nat. Med., 2005, 11(4), S63–S68, DOI:10.1038/nm1210
.
- P. Marrack, A. S. McKee and M. W. Munks, Towards an understanding of the adjuvant action of aluminium, Nat. Rev. Immunol., 2009, 9(4), 287–293, DOI:10.1038/nri2510
.
- V. E. J. C. Schijns, Immunological concepts of vaccine adjuvant activity: Commentary, Curr. Opin. Immunol., 2000, 12(4), 456–463, DOI:10.1016/S0952-7915(00)00120-5
.
- D. T. O’Hagan, G. S. Ott, G. V. Nest, R. Rappuoli and G. D. Giudice, The history of MF59® adjuvant: a phoenix that arose from the ashes, Expert Rev. Vaccines, 2013, 12(1), 13–30, DOI:10.1586/erv.12.140
.
- Y. T. Lim, Vaccine adjuvant materials for cancer immunotherapy and control of infectious disease, Clin. Exp. Vaccine Res., 2015, 4(1), 54–58, DOI:10.7774/cevr.2015.4.1.54
.
- K. Vedhara, K. Ayling, K. Sunger, D. M. Caldwell, V. Halliday, L. Fairclough, A. Avery, L. Robles, J. Garibaldi and N. J. Welton,
et al., Psychological interventions as vaccine adjuvants: A systematic review, Vaccine, 2019, 37(25), 3255–3266, DOI:10.1016/j.vaccine.2019.04.091
.
- A. M. Didierlaurent, S. Morel, L. Lockman, S. L. Giannini, M. Bisteau, H. Carlsen, A. Kielland, O. Vosters, N. Vanderheyde and F. Schiavetti,
et al., AS04, an Aluminum Salt- and TLR4 Agonist-Based Adjuvant System, Induces a Transient Localized Innate Immune Response Leading to Enhanced Adaptive Immunity1, J. Immunol., 2009, 183(10), 6186–6197, DOI:10.4049/jimmunol.0901474
.
- C. K. Baumgartner and L. P. Malherbe, Regulation of CD4 T-cell receptor diversity by vaccine adjuvants, Immunology, 2010, 130(1), 16–22, DOI:10.1111/j.1365-2567.2010.03265.x
.
- J. W. Hadden, T-cell adjuvants, Int. J. Immunopharmacol., 1994, 16(9), 703–710, DOI:10.1016/0192-0561(94)90090-6
.
- A. O. Kamphorst, K. Araki and R. Ahmed, Beyond adjuvants: Immunomodulation strategies to enhance T cell immunity, Vaccine, 2015, 33, B21–B28, DOI:10.1016/j.vaccine.2014.12.082
.
- R. R. Rapaka, A. S. Cross and M. A. McArthur, Using Adjuvants to Drive T Cell Responses for Next-Generation Infectious Disease Vaccines, Vaccines, 2021, 9(8), 820 CrossRef CAS PubMed
.
- C. Bode, G. Zhao, F. Steinhagen, T. Kinjo and D. M. Klinman, CpG DNA as a vaccine adjuvant, Expert Rev. Vaccines, 2011, 10(4), 499–511, DOI:10.1586/erv.10.174
.
- D. M. Klinman, Adjuvant Activity of CpG Oligodeoxynucleotides, Int. Rev. Immunol., 2006, 25(3–4), 135–154, DOI:10.1080/08830180600743057
.
- J. Scheiermann and D. M. Klinman, Clinical evaluation of CpG oligonucleotides as adjuvants for vaccines targeting infectious diseases and cancer, Vaccine, 2014, 32(48), 6377–6389, DOI:10.1016/j.vaccine.2014.06.065
.
- O. Pérez, B. Romeu, O. Cabrera, E. Gonzalez, A. Batista-Duharte, A. Labrada, R. Pérez, L. Reyes, W. Ramírez and S. Sifontes,
et al., Adjuvants are Key Factors for the Development of Future Vaccines: Lessons from the Finlay Adjuvant Platform, Front. Immunol., 2013, 4, 407, DOI:10.3389/fimmu.2013.00407
.
- D. T. O’Hagan and E. De Gregorio, The path to a successful vaccine adjuvant – ‘The long and winding road’, Drug Discovery Today, 2009, 14(11), 541–551, DOI:10.1016/j.drudis.2009.02.009
.
- V. Cimica and J. M. Galarza, Adjuvant formulations for virus-like particle (VLP) based vaccines, Clin. Immunol., 2017, 183, 99–108, DOI:10.1016/j.clim.2017.08.004
.
- F. M. Buonaguro, M. L. Tornesello and L. Buonaguro, Virus-like particle vaccines and adjuvants: the HPV paradigm, Expert Rev. Vaccines, 2009, 8(10), 1379–1398, DOI:10.1586/erv.09.81
.
- N. Garçon, L. Segal, F. Tavares and M. Van Mechelen, The safety evaluation of adjuvants during vaccine development: The AS04 experience, Vaccine, 2011, 29(27), 4453–4459, DOI:10.1016/j.vaccine.2011.04.046
.
- B. N. Lambrecht, M. Kool, M. A. M. Willart and H. Hammad, Mechanism of action of clinically approved adjuvants, Curr. Opin. Immunol., 2009, 21(1), 23–29, DOI:10.1016/j.coi.2009.01.004
.
- X. Li, X. Wang and A. Ito, Tailoring inorganic nanoadjuvants towards next-generation vaccines, Chem. Soc. Rev., 2018, 47(13), 4954–4980, 10.1039/c8cs00028j
.
- X. Wang, X. Li, K. Onuma, Y. Sogo, T. Ohno and A. Ito, Zn- and Mg- containing tricalcium phosphates-based adjuvants for cancer immunotherapy, Sci. Rep., 2013, 3, 2203, DOI:10.1038/srep02203
.
- D. Sen, T. J. Deerinck, M. H. Ellisman, I. Parker and M. D. Cahalan, Quantum Dots for Tracking Dendritic Cells and Priming an Immune Response In Vitro and In Vivo, PLoS One, 2008, 3(9), e3290, DOI:10.1371/journal.pone.0003290
.
- X. Wang, S. Ihara, X. Li, A. Ito, Y. Sogo, Y. Watanabe, A. Yamazaki, N. M. Tsuji and T. Ohno, Rod-Scale Design Strategies for Immune-Targeted Delivery System toward Cancer Immunotherapy, ACS Nano, 2019, 13(7), 7705–7715, DOI:10.1021/acsnano.9b01271
.
- X. Wang, X. Li, A. Ito, Y. Watanabe, Y. Sogo, N. M. Tsuji and T. Ohno, Stimulation of In Vivo Antitumor Immunity with Hollow Mesoporous Silica Nanospheres, Angew. Chem., Int. Ed., 2016, 55(5), 1899–1903, DOI:10.1002/anie.201506179
.
- X. Wang, X. Li, K. Yoshiyuki, Y. Watanabe, Y. Sogo, T. Ohno, N. M. Tsuji and A. Ito, Comprehensive Mechanism Analysis of Mesoporous-Silica-Nanoparticle-Induced Cancer Immunotherapy, Adv. Healthcare Mater., 2016, 5(10), 1169–1176, DOI:10.1002/adhm.201501013
.
- A. S. Yazdi, G. Guarda, N. Riteau, S. K. Drexler, A. Tardivel, I. Couillin and J. Tschopp, Nanoparticles activate the NLR pyrin domain containing 3 (Nlrp3) inflammasome and cause pulmonary inflammation through release of IL-1α and IL-1β, Proc. Natl. Acad. Sci. U. S. A., 2010, 107(45), 19449–19454, DOI:10.1073/pnas.1008155107
.
- Y. Zhang, Z. Cui, H. Kong, K. Xia, L. Pan, J. Li, Y. Sun, J. Shi, L. Wang and Y. Zhu,
et al., One-Shot Immunomodulatory Nanodiamond Agents for Cancer Immunotherapy, Adv. Mater., 2016, 28(14), 2699–2708, DOI:10.1002/adma.201506232
.
- A. Li, L. Qin, D. Zhu, R. Zhu, J. Sun and S. Wang, Signalling pathways involved in the activation of dendritic cells by layered double hydroxide nanoparticles, Biomaterials, 2010, 31(4), 748–756, DOI:10.1016/j.biomaterials.2009.09.095
.
- X. Wang, X. Li, Y. Sogo and A. Ito, Simple synthesis route of mesoporous AlOOH nanofibers to enhance immune responses, RSC Adv., 2013, 3, 8164–8167 RSC
.
- D. T. O’Hagan, G. S. Ott, E. De Gregorio and A. Seubert, The mechanism of action of MF59 – An innately attractive adjuvant formulation, Vaccine, 2012, 30(29), 4341–4348, DOI:10.1016/j.vaccine.2011.09.061
.
- S. Lartey, R. D. Pathirana, F. Zhou, Å. Jul-Larsen, E. Montomoli, J. Wood and R. J. Cox, Single dose vaccination of the ASO3-adjuvanted A(H1N1)pdm09 monovalent vaccine in health care workers elicits homologous and cross-reactive cellular and humoral responses to H1N1 strains, Hum. Vaccines Immunother., 2015, 11(7), 1654–1662, DOI:10.1080/21645515.2015.1048939
.
- S. Adams, Toll-like receptor agonists in cancer therapy, Immunotherapy, 2009, 1(6), 949–964, DOI:10.2217/imt.09.70
.
- C. N. Baxevanis, I. F. Voutsas and O. E. Tsitsilonis, Toll-like receptor agonists: current status and future perspective on their utility as adjuvants in improving antic ancer vaccination strategies, Immunotherapy, 2013, 5(5), 497–511, DOI:10.2217/imt.13.24
.
- S. Gnjatic, N. B. Sawhney and N. Bhardwaj, Toll-Like Receptor Agonists: Are They Good Adjuvants?, Cancer J., 2010, 16(4), 382–391 CrossRef CAS PubMed
.
- A. Kaur, J. Baldwin, D. Brar, D. B. Salunke and N. Petrovsky, Toll-like receptor (TLR) agonists as a driving force behind next-generation vaccine adjuvants and cancer therapeutics, Curr. Opin. Chem. Biol., 2022, 70, 102172, DOI:10.1016/j.cbpa.2022.102172
.
- D. N. Toussi and P. Massari, Immune Adjuvant Effect of Molecularly-defined Toll-Like Receptor Ligands, Vaccines, 2014, 2, 323–353 CrossRef CAS PubMed
.
- J.-X. Yang, J.-C. Tseng, G.-Y. Yu, Y. Luo, C.-Y. F. Huang, Y.-R. Hong and T.-H. Chuang, Recent Advances in the Development of Toll-like Receptor Agonist-Based Vaccine Adjuvants for Infectious Diseases, Pharmaceutics, 2022, 14(2), 423 CrossRef CAS PubMed
.
- N. Wang, M. Chen and T. Wang, Liposomes used as a vaccine adjuvant-delivery system: From basics to clinical immunization, J. Controlled Release, 2019, 303, 130–150, DOI:10.1016/j.jconrel.2019.04.025
.
- G. Gregoriadis, Immunological adjuvants: a role for liposomes, Immunol. Today, 1990, 11, 89–97, DOI:10.1016/0167-5699(90)90034-7
.
- C. R. Alving, Z. Beck, G. R. Matyas and M. Rao, Liposomal adjuvants for human vaccines, Expert Opin. Drug Delivery, 2016, 13(6), 807–816, DOI:10.1517/17425247.2016.1151871
.
- A. W. Heath and J. H. L. Playfair, Cytokines as immunological adjuvants, Vaccine, 1992, 10(7), 427–434, DOI:10.1016/0264-410X(92)90389-2
.
-
M. G. Tovey and C. Lallemand, Adjuvant Activity of Cytokines, in Vaccine Adjuvants: Methods and Protocols, ed. G. Davies, Humana Press, 2010, pp. 287–309 Search PubMed
.
- A. K. Shakya and K. S. Nandakumar, Applications of polymeric adjuvants in studying autoimmune responses and vaccination against infectious diseases, J. R. Soc., Interface, 2013, 10(79), 20120536, DOI:10.1098/rsif.2012.0536
.
- A. M. Weiss, S. Hossainy, S. J. Rowan, J. A. Hubbell and A. P. Esser-Kahn, Immunostimulatory Polymers as Adjuvants, Immunotherapies, and Delivery Systems, Macromolecules, 2022, 55(16), 6913–6937, DOI:10.1021/acs.macromol.2c00854
.
- H.-X. Sun, Y. Xie and Y.-P. Ye, Advances in saponin-based adjuvants, Vaccine, 2009, 27(12), 1787–1796, DOI:10.1016/j.vaccine.2009.01.091
.
- P. Wang, Natural and Synthetic Saponins as Vaccine Adjuvants, Vaccines, 2021, 9(3), 222 CrossRef CAS PubMed
.
- C. Moser, M. Müller, M. D. Kaeser, U. Weydemann and M. Amacker, Influenza virosomes as vaccine adjuvant and carrier system, Expert Rev. Vaccines, 2013, 12(7), 779–791, DOI:10.1586/14760584.2013.811195
.
- C. Moser, I. C. Metcalfe and J.-F. Viret, Virosomal adjuvanted antigen delivery systems, Expert Rev. Vaccines, 2003, 2(2), 189–196, DOI:10.1586/14760584.2.2.189
.
-
G. Del Giudice and R. Rappuoli, Inactivated and Adjuvanted Influenza Vaccines. in Influenza Pathogenesis and Control, ed. M. B. A. Oldstone and R. W. Compans, Springer International Publishing, 2015, pp. 151–180 Search PubMed
.
- Z. Liang, H. Zhu, X. Wang, B. Jing, Z. Li, X. Xia, H. Sun, Y. Yang, W. Zhang and L. Shi,
et al., Adjuvants for Coronavirus Vaccines, Front. Immunol., 2020, 11, 589833, DOI:10.3389/fimmu.2020.589833
.
- Y. Liu, Z. Wang, X. Zhuang, S. Zhang, Z. Chen, Y. Zou, J. Sheng, T. Li, W. Tai and J. Yu,
et al., Inactivated vaccine-elicited potent antibodies can broadly neutralize SARS-CoV-2 circulating variants, Nat. Commun., 2023, 14(1), 2179, DOI:10.1038/s41467-023-37926-7
.
- A. S. Lauring, J. O. Jones and R. Andino, Rationalizing the development of live attenuated virus vaccines, Nat. Biotechnol., 2010, 28(6), 573–579, DOI:10.1038/nbt.1635
.
- A. Bouazzaoui, A. A. H. Abdellatif, F. A. Al-Allaf, N. M. Bogari, S. Al-Dehlawi and S. H. Qari, Strategies for Vaccination: Conventional Vaccine Approaches Versus New-Generation Strategies in Combination with Adjuvants, Pharmaceutics, 2021, 13(2), 140 CrossRef CAS PubMed
.
- H. J. Whitaker, R. S. M. Tsang, R. Byford, N. J. Andrews, J. Sherlock, P. Sebastian Pillai, J. Williams, E. Button, H. Campbell and M. Sinnathamby,
et al., Pfizer-BioNTech and Oxford AstraZeneca COVID-19 vaccine effectiveness and immune response amongst individuals in clinical risk groups, J. Infect., 2022, 84(5), 675–683, DOI:10.1016/j.jinf.2021.12.044
.
- B. Ferraro, M. P. Morrow, N. A. Hutnick, T. H. Shin, C. E. Lucke and D. B. Weiner, Clinical Applications of DNA Vaccines: Current Progress, Clin. Infect. Dis., 2011, 53(3), 296–302, DOI:10.1093/cid/cir334
.
- J. R. Greenland and N. L. Letvin, Chemical adjuvants for plasmid DNA vaccines, Vaccine, 2007, 25(19), 3731–3741, DOI:10.1016/j.vaccine.2007.01.120
.
- M. D. Buschmann, M. J. Carrasco, S. Alishetty, M. Paige, M. G. Alameh and D. Weissman, Nanomaterial Delivery Systems for mRNA Vaccines, Vaccines, 2021, 9(1), 65 CrossRef CAS PubMed
.
- M. Karam and G. Daoud, mRNA vaccines: Past, present, future, Asian J. Pharm. Sci., 2022, 17(4), 491–522, DOI:10.1016/j.ajps.2022.05.003
.
- N. Pardi, M. J. Hogan, F. W. Porter and D. Weissman, mRNA vaccines – a new era in vaccinology, Nat. Rev. Drug Discovery, 2018, 17(4), 261–279, DOI:10.1038/nrd.2017.243
.
- C. Foged, Subunit vaccines of the future: the need for safe, customized and optimized particulate delivery systems, Ther. Delivery, 2011, 2(8), 1057–1077, DOI:10.4155/tde.11.68
.
- B. Y. Chua, T. Sekiya and D. C. Jackson, Opinion: Making Inactivated and Subunit-Based Vaccines Work, Viral Immunol., 2018, 31(2), 150–158, DOI:10.1089/vim.2017.0146
.
- J. d S. Apostólico, V. A. S. Lunardelli, F. C. Coirada, S. B. Boscardin and D. S. Rosa, Adjuvants: Classification, Modus Operandi, and Licensing, J. Immunol. Res., 2016, 2016, 1459394, DOI:10.1155/2016/1459394
.
- P. Spearman, M. A. Lally, M. Elizaga, D. Montefiori, G. D. Tomaras, M. J. McElrath, J. Hural, S. C. De Rosa, A. Sato and Y. Huang,
et al., A trimeric, V2-deleted HIV-1 envelope glycoprotein vaccine elicits potent neutralizing antibodies but limited breadth of neutralization in human volunteers, J. Infect. Dis., 2011, 203(8), 1165–1173, DOI:10.1093/infdis/jiq175
.
- R. L. Atmar, W. A. Keitel, S. M. Patel, J. M. Katz, D. She, H. El Sahly, J. Pompey, T. R. Cate and R. B. Couch, Safety and immunogenicity of nonadjuvanted and MF59-adjuvanted influenza A/H9N2 vaccine preparations, Clin. Infect. Dis., 2006, 43(9), 1135–1142, DOI:10.1086/508174
.
- J. Aucouturier, L. Dupuis, S. Deville, S. Ascarateil and V. Ganne, Montanide ISA 720 and 51: a new generation of water in oil emulsions as adjuvants for human vaccines, Expert Rev. Vaccines, 2002, 1(1), 111–118, DOI:10.1586/14760584.1.1.111
.
- L. A. Jackson, W. H. Chen, J. T. Stapleton, C. L. Dekker, A. Wald, R. C. Brady, S. Edupuganti, P. Winokur, M. J. Mulligan and H. L. Keyserling,
et al., Immunogenicity and safety of varying dosages of a monovalent 2009 H1N1 influenza vaccine given with and without AS03 adjuvant system in healthy adults and older persons, J. Infect. Dis., 2012, 206(6), 811–820, DOI:10.1093/infdis/jis427
.
- C. B. Creech, R. W. Frenck, E. A. Sheldon, D. J. Seiden, M. K. Kankam, E. T. Zito, D. Girgenti, J. M. Severs, F. W. Immermann and L. K. McNeil,
et al., Safety, tolerability, and immunogenicity of a single dose 4-antigen or 3-antigen Staphylococcus aureus vaccine in healthy older adults: Results of a randomised trial, Vaccine, 2017, 35(2), 385–394, DOI:10.1016/j.vaccine.2016.11.032
.
-
J. T. Ulrich and K. R. Myers, Monophosphoryl Lipid A as an Adjuvant. in Vaccine Design: The Subunit and Adjuvant Approach, ed. M. F. Powell and M. J. Newman, Springer, US, 1995, pp. 495–524 Search PubMed
.
- K. Okemoto, K. Kawasaki, K. Hanada, M. Miura and M. Nishijima, A potent adjuvant monophosphoryl lipid A triggers various immune responses, but not secretion of IL-1beta or activation of caspase-1, J. Immunol., 2006, 176(2), 1203–1208, DOI:10.4049/jimmunol.176.2.1203
.
- A. Pavlick, A. B. Blazquez, M. Meseck, M. Lattanzi, P. A. Ott, T. U. Marron, R. M. Holman, J. Mandeli, A. M. Salazar and C. B. McClain,
et al., Combined Vaccination with NY-ESO-1 Protein, Poly-ICLC, and Montanide Improves Humoral and Cellular Immune Responses in Patients with High-Risk Melanoma, Cancer Immunol. Res., 2020, 8(1), 70–80, DOI:10.1158/2326-6066.Cir-19-0545
.
- E. Rosenbaum, R. Chugh, C. W. Ryan, M. Agulnik, M. M. Milhem, S. George, R. L. Jones, B. Chmielowski, B. A. Van Tine and H. Tawbi,
et al., A randomised phase II trial of a trivalent ganglioside vaccine targeting GM2, GD2 and GD3 combined with immunological adjuvant OPT-821 versus OPT-821 alone in metastatic sarcoma patients rendered disease-free by surgery, Eur. J. Cancer, 2022, 176, 155–163, DOI:10.1016/j.ejca.2022.09.003
.
- I. Y. Cheung, N. V. Cheung, S. Modak, A. Mauguen, Y. Feng, E. Basu, S. S. Roberts, G. Ragupathi and B. H. Kushner, Survival Impact of Anti-GD2 Antibody Response in a Phase II Ganglioside Vaccine Trial Among Patients With High-Risk Neuroblastoma With Prior Disease Progression, J. Clin. Oncol., 2021, 39(3), 215–226, DOI:10.1200/jco.20.01892
.
- L. Stertman, A. E. Palm, B. Zarnegar, B. Carow, C. Lunderius Andersson, S. E. Magnusson, C. Carnrot, V. Shinde, G. Smith and G. Glenn,
et al., The Matrix-M™ adjuvant: A critical component of vaccines for the 21(st) century, Hum. Vaccines Immunother., 2023, 19(1), 2189885, DOI:10.1080/21645515.2023.2189885
.
- Y. Wang, M. Swiecki, S. A. McCartney and M. Colonna, dsRNA sensors and plasmacytoid dendritic cells in host defense and autoimmunity, Immunol. Rev., 2011, 243(1), 74–90, DOI:10.1111/j.1600-065X.2011.01049.x
.
- R. Jalah, V. Patel, V. Kulkarni, M. Rosati, C. Alicea, B. Ganneru, A. von Gegerfelt, W. Huang, Y. Guan and K. E. Broderick,
et al., IL-12 DNA as molecular vaccine adjuvant increases the cytotoxic T cell responses and breadth of humoral immune responses in SIV DNA vaccinated macaques, Hum. Vaccines Immunother., 2012, 8(11), 1620–1629, DOI:10.4161/hv.21407
.
- E. Shen, L. Li, L. Li, L. Feng, L. Lu, Z. Yao, H. Lin and C. Wu, PIKA as an adjuvant enhances specific humoral and cellular immune responses following the vaccination of mice with HBsAg plus PIKA, Cell. Mol. Immunol., 2007, 4(2), 113–120 CAS
.
- C. Zhang, B. Wang and M. Wang, GM-CSF and IL-2 as adjuvant enhance the immune effect of protein vaccine against foot-and-mouth disease, Virol. J., 2011, 8, 7, DOI:10.1186/1743-422x-8-7
.
- M. Kheirvari, H. Liu and E. Tumban, Virus-like Particle Vaccines and Platforms for Vaccine Development, Viruses, 2023, 15(5), 1109, DOI:10.3390/v15051109
.
- T. Kimura, J. R. McKolanis, L. A. Dzubinski, K. Islam, D. M. Potter, A. M. Salazar, R. E. Schoen and O. J. Finn, MUC1 vaccine for individuals with advanced adenoma of the colon: a cancer immunoprevention feasibility study, Cancer Prev. Res., 2013, 6(1), 18–26, DOI:10.1158/1940-6207.Capr-12-0275
.
- S. Kalimuddin, L. Wijaya, Y. F. Z. Chan, A. W. L. Wong, H. M. L. Oh, L. F. Wang, J. A. Kassim, J. Zhao, Z. Shi and J. G. Low, A phase II randomized study to determine the safety and immunogenicity of the novel PIKA rabies vaccine containing the PIKA adjuvant using an accelerated regimen, Vaccine, 2017, 35(51), 7127–7132, DOI:10.1016/j.vaccine.2017.10.097
.
- I. Bjarnason, G. Sission and B. Hayee, A randomised, double-blind, placebo-controlled trial of a multi-strain probiotic in patients with asymptomatic ulcerative colitis and Crohn's disease, Inflammopharmacology, 2019, 27(3), 465–473, DOI:10.1007/s10787-019-00595-4
.
- J. D. Masson, M. Thibaudon, L. Bélec and G. Crépeaux, Calcium phosphate: a substitute for aluminum adjuvants, Expert Rev. Vaccines, 2017, 16(3), 289–299, DOI:10.1080/14760584.2017.1244484
.
- S. K. Gupta, P. Bajwa, R. Deb, M. M. Chellappa and S. Dey, Flagellin a toll-like receptor 5 agonist as an adjuvant in chicken vaccines, Clin. Vaccine Immunol., 2014, 21(3), 261–270, DOI:10.1128/cvi.00669-13
.
- L. Corey, J. R. Mascola, A. S. Fauci and F. S. Collins, A strategic approach to COVID-19 vaccine R&D, Science, 2020, 368(6494), 948–950, DOI:10.1126/science.abc5312
.
- M. Jeyanathan, S. Afkhami, F. Smaill, M. S. Miller, B. D. Lichty and Z. Xing, Immunological considerations for COVID-19 vaccine strategies, Nat. Rev. Immunol., 2020, 20(10), 615–632, DOI:10.1038/s41577-020-00434-6
.
- J. H. Kim, F. Marks and J. D. Clemens, Looking beyond COVID-19 vaccine phase 3 trials, Nat. Med., 2021, 27(2), 205–211, DOI:10.1038/s41591-021-01230-y
.
- S. Machingaidze and C. S. Wiysonge, Understanding COVID-19 vaccine hesitancy, Nat. Med., 2021, 27(8), 1338–1339, DOI:10.1038/s41591-021-01459-7
.
- E. Mathieu, H. Ritchie, E. Ortiz-Ospina, M. Roser, J. Hasell, C. Appel, C. Giattino and L. Rodés-Guirao, A global database of COVID-19 vaccinations, Nat. Hum. Behav., 2021, 5(7), 947–953, DOI:10.1038/s41562-021-01122-8
.
- P. S. Arunachalam, A. C. Walls, N. Golden, C. Atyeo, S. Fischinger, C. Li, P. Aye, M. J. Navarro, L. Lai and V. V. Edara,
et al., Adjuvanting a subunit COVID-19 vaccine to induce protective immunity, Nature, 2021, 594(7862), 253–258, DOI:10.1038/s41586-021-03530-2
.
- K. S. Park, X. Sun, M. E. Aikins and J. J. Moon, Non-viral COVID-19 vaccine delivery systems, Adv. Drug Delivery Rev., 2021, 169, 137–151, DOI:10.1016/j.addr.2020.12.008
.
- A. Pormohammad, M. Zarei, S. Ghorbani, M. Mohammadi, M. H. Razizadeh, D. L. Turner and R. J. Turner, Efficacy and Safety of COVID-19 Vaccines: A Systematic Review and Meta-Analysis of Randomized Clinical Trials, Vaccines, 2021, 9(5), 467 CrossRef CAS PubMed
.
- J. Wang, Y. Peng, H. Xu, Z. Cui and R. O. Williams, The COVID-19 Vaccine Race: Challenges and Opportunities in Vaccine Formulation, AAPS PharmSciTech, 2020, 21(6), 225, DOI:10.1208/s12249-020-01744-7
.
- N. Zhang, K. Li, Z. Liu, K. S. Nandakumar and S. Jiang, A Perspective on the Roles of Adjuvants in Developing Highly Potent COVID-19 Vaccines, Viruses, 2022, 14(2), 387 CrossRef CAS PubMed
.
- P. T. Heath, E. P. Galiza, D. N. Baxter, M. Boffito, D. Browne, F. Burns, D. R. Chadwick, R. Clark, C. Cosgrove and J. Galloway,
et al., Safety and Efficacy of NVX-CoV2373 Covid-19 Vaccine, N. Engl. J. Med., 2021, 385(13), 1172–1183, DOI:10.1056/NEJMoa2107659
.
- A. García and J. B. De Sanctis, An overview of adjuvant formulations and delivery systems, APMIS, 2014, 122(4), 257–267, DOI:10.1111/apm.12143
.
- I. Sarkar, R. Garg and S. van Drunen Littel-van den Hurk, Selection of adjuvants for vaccines targeting specific pathogens, Expert Rev Vaccines, 2019, 18(5), 505–521, DOI:10.1080/14760584.2019.1604231
.
- M. B. Laurens, RTS,S/AS01 vaccine (Mosquirix™): an overview, Hum. Vaccines Immunother., 2020, 16(3), 480–489, DOI:10.1080/21645515.2019.1669415
.
- H. Lal, A. L. Cunningham, O. Godeaux, R. Chlibek, J. Diez-Domingo, S.-J. Hwang, M. J. Levin, J. E. McElhaney, A. Poder and J. Puig-Barberà,
et al., Efficacy of an Adjuvanted Herpes Zoster Subunit Vaccine in Older Adults, N. Engl. J. Med., 2015, 372(22), 2087–2096, DOI:10.1056/NEJMoa1501184
.
- A. C. Anselmo, Y. Gokarn and S. Mitragotri, Non-invasive delivery strategies for biologics, Nat. Rev. Drug Discovery, 2019, 18(1), 19–40, DOI:10.1038/nrd.2018.183
.
- M. A. Kalam, A. A. Khan and A. Alshamsan, Non-invasive administration of biodegradable nano-carrier vaccines, Am. J. Transl. Res., 2017, 9(1), 15–35 CAS
.
- M. Skwarczynski and I. Toth, Non-invasive mucosal vaccine delivery: advantages, challenges and the future, Expert Opin. Drug Delivery, 2020, 17(4), 435–437, DOI:10.1080/17425247.2020.1731468
.
|
This journal is © The Royal Society of Chemistry 2024 |
Click here to see how this site uses Cookies. View our privacy policy here.