DOI:
10.1039/D3TB03046F
(Paper)
J. Mater. Chem. B, 2024,
12, 3240-3248
Radiation responsive PROTAC nanoparticles for tumor-specific proteolysis enhanced radiotherapy†
Received
26th December 2023
, Accepted 20th February 2024
First published on 21st February 2024
Abstract
Proteolysis targeting chimeras (PROTACs) is a promising strategy for cancer therapy. However, the always-on bioactivity of PROTACs may lead to non-target toxicity, which restricts their antitumor performance. Here, we developed an X-ray radiation responsive PROTAC nanomicelle (RCNprotac) by covalently conjugating a reported small molecule PROTAC (MZ1) to hydrophilic PEG via a diselenide bond-containing carbon chain, which then self-assembled into a 141.80 ± 5.66 nm nanomicelle. The RCNprotac displayed no bioactivity during circulation due to the occupation of the hydroxyl group on the E3 ubiquitin ligand component and could effectively accumulate at the tumor site owing to the enhanced permeability and retention effect. Upon exposure to X-ray radiation, the radiation-sensitive diselenide bonds were broken to specifically release MZ1 for tumor BRD4 protein degradation. Furthermore, the reduction in the BRD4 protein level could increase the tumor's sensitivity to radiation. RCNprotac showed a synergistic enhancement of antitumor effects both in vitro and in vivo. We believe that this X-ray-responsive PROTAC nanomicelle could provide a new strategy for the X-ray-activated spatiotemporally controlled protein degradation and for the BRD4 proteolysis enhanced tumor radiosensitivity.
1. Introduction
Proteolysis targeting chimeras (PROTACs), which could selectively degrade proteins, has emerged as a promising cancer therapeutic strategy.1 A traditional PROTAC molecule usually consists of a ligand to recruit the protein of interest (POI), a ligand to recruit E3 ubiquitin ligase, and a linker to tether these two ligands, which allows hijacking both the E3 ligase and POI and degrading the POI through the ubiquitin–proteasome system (UPS).2 Compared with traditional small-molecule inhibitors, PROTACs can target undruggable proteins and achieve a desired pharmacological effect at a much lower concentration due to their catalytic mode for proteolysis and reuse.3 Although some PROTACs have already entered clinical trials, their relatively high molecular weight (in the range of 800–1000 Da) and undesired solubility may reduce their cell permeability and tumor accumulation.1,4,5 The degradation of off-target proteins in normal tissues may cause systemic toxicity. Recently, PROTAC based prodrugs or smart nanoplatforms triggered by either the internal microenvironment conditions such as pH6 and enzymes7,8 or external stimuli such as light9,10 and X-ray11 have been developed to control the activation of PROTACs. Among them, X-ray radiation has attracted much attention with the advantages of excellent spatiotemporal precision and deep tissue penetration.
X-ray radiation therapy is a first-line clinical treatment for cancer.12 Besides directly killing cancer cells via DNA damage,13,14 X-ray radiation is also widely used to excite sensitizers to generate reactive oxygen species15,16 and to trigger the drug release from either the prodrug11,17,18 or the nanoplatform.19,20 Yang et al. developed the first X-ray triggered PROTAC prodrug by incorporating a radiation responsive phenyl azide cage into a PROTAC molecule (ARV-771).11 The resulting prodrug showed little activity on targeting proteins, but the protein degradation capability could be recovered once irradiated with X-ray both in vitro and in vivo. However, a PROTAC system with higher tumor accumulation and enhanced X-ray sensitivity is still highly desired.
Chemical conjugation of polyethylene glycol (PEG) is widely used to improve the solubility of small molecules.21 MZ1 is a well-studied bromodomain-containing protein 4 (BRD4)-targeting PROTAC and is considered as a well-developed platform for PROTAC mechanism studies and method development.22,23 Recent work revealed the important role of BRD4 in DNA damage repair and the BRD4 inhibitor JQ1 has been proved to be an effective radiosensitizer to prevent DNA damage repair, which is a major cause of cancer recurrence.24–26 Here, we proposed to construct an X-ray radiation responsive PROTAC nanomicelle (RCNprotac) for enhanced radiotherapy by conjugating PEG onto MZ1 via a diselenide linker (Scheme 1A). Diselenide bonds are widely used in radiation triggered drug delivery systems due to their sensitivity to low dose X-ray and the tumor microenvironment.27–30 PEGylated MZ1 became amphiphilic and could spontaneously form a 141.80 ± 5.66 nm monodisperse nanomicelle upon self-assembly. The resulting RCNprotac demonstrated excellent tumor accumulation due to the enhanced permeability and retention (EPR) effect. Upon exposure to X-ray radiation, MZ1 was released in a spatiotemporally controlled way to degrade BRD4 both in vitro and in vivo. In addition, the RCNprotac-mediated reduction in BRD4 levels has a radiation sensitization effect on tumors (Scheme 1B). This work provides a new X-ray responsive PROTAC release strategy for synergistically integrating radiotherapy and protein degradation to achieve effective anti-tumor therapy.
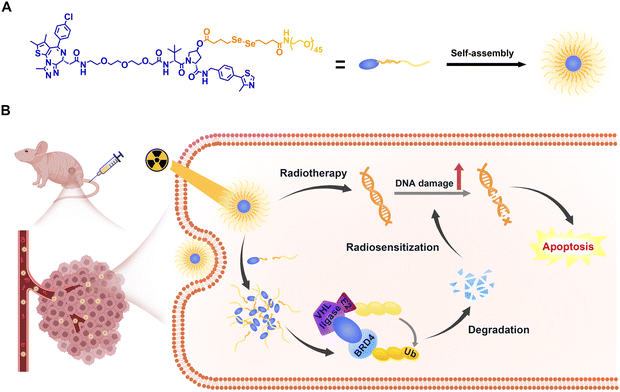 |
| Scheme 1 Illustration of (A) self-assembly of RCNprotac and (B) X-ray-triggered PROTAC release for in vivo BRD4 proteolysis and BRD4 degradation enhanced radiosensitization. | |
2. Results and discussion
2.1. Design, synthesis, and characterization of RCNprotac
To obtain X-ray responsive PROTAC, we esterified the hydroxyl group on the E3 ubiquitin ligand of MZ1 with a diselenide-bond-containing carbon chain and subsequently conjugated PEG2000 to introduce hydrophilicity, resulting in an amphiphilic RCNprotac precursor. In addition, to optimize the drug release capability, we tested the diselenide-bond-containing carbon chain with three different lengths (Fig. 1A). All compounds were synthesized (Schemes S1–S4, ESI†), and the resulting structures were confirmed by proton nuclear magnetic resonance (1H-NMR) and high-resolution mass spectrometry (HR-MS) (Fig. S1–S11, ESI†). All three different RCNprotac precursors were self-assembled in water to form nanomicelles on account of their amphiphilicity and were abbreviated as RCNprotac (α), RCNprotac (β), and RCNprotac (γ) based on the α-, β-, or γ-position of the selenium atom with respect to the ester bond, respectively. Transmission electron microscopy (TEM) (Fig. 1B) and dynamic light scattering (DLS) analyses (Fig. 1C) indicated the spherical morphology with uniform size distribution of RCNprotac (α), RCNprotac (β), and RCNprotac (γ) with similar particle sizes of 132.25 ± 3.33 nm, 137.58 ± 1.14 nm, and 141.80 ± 5.66 nm, respectively.
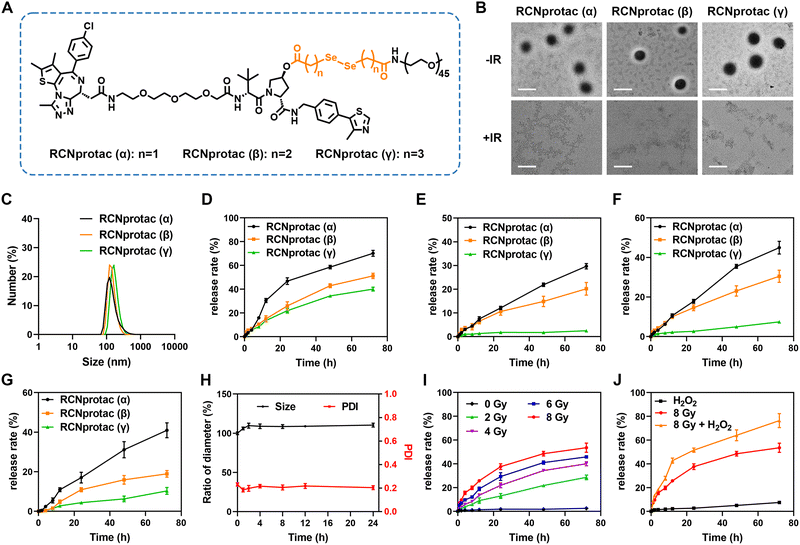 |
| Fig. 1 Characterization of RCNprotac. (A) Illustration of structures of three different RCNprotac precursors. (B) The representative TEM images of RCNprotac (α), RCNprotac (β), and RCNprotac (γ) incubated for 24 h without radiation or upon 8 Gy exposure (scale bar = 200 nm). (C) DLS profiles of RCNprotac (α), RCNprotac (β), and RCNprotac (γ). (D and E) MZ1 release profiles of three kinds of RCNprotac upon (D) 4 Gy radiation exposure or (E) without radiation. (F) and (G) MZ1 release profiles of three kinds of RCNprotac under (F)100 μM H2O2 or (G) 1 mM GSH. (H) Time-dependent changes of the size and PDI of RCNprotac (γ) after incubation in pH 7.4 DMEM supplemented with 10% FBS at 37 °C. (I) MZ1 release profiles of RCNprotac (γ) at different doses of radiation. (J) MZ1 release profiles of RCNprotac (γ) under 8 Gy, 100 μM H2O2, and 8 Gy + 100 μM H2O2 conditions. Data were represented as mean ± SD. | |
2.2. Radiation-responsive MZ1 release from RCNprotac
To verify the stability and radiation-responsive properties, the prepared nanomicelles were then exposed to X-ray radiation and the released MZ1 was detected by liquid chromatography-mass spectrometry (LC-MS). It is worth mentioning that the structure of MZ1 remained stable upon exposure to X-ray radiation up to 8 Gy (Fig. S12, ESI†). As shown in Fig. 1D, all three nanomicelles exhibited X-ray-triggered MZ1 release in an incubation time-dependent manner. Upon exposure to X-ray radiation at 4 Gy, RCNprotac (α) showed the most efficient release of MZ1 with 70.17% within 72 h, compared to 39.98% and 51.15% MZ1 released by RCNprotac (γ) and RCNprotac (β). Furthermore, the release behaviors of nanomicelles without X-ray radiation are shown in Fig. 1E, and the proportions of MZ1 leaked from RCNprotac (γ), RCNprotac (β), and RCNprotac (α) were 2.48%, 20.19%, and 29.68%, respectively. Since the diselenide bonds could specifically respond to hydrogen peroxide (H2O2) and glutathione (GSH),31–35 we also detected the release behaviors of three nanomicelles at 100 μM H2O2 or 1 mM GSH. As shown in Fig. 1F and G, RCNprotac (γ) showed much less release (7.42% and 10.30%) within 72 h under H2O2 and GSH conditions than RCNprotac (α) (44.96% and 40.95%) and RCNprotac (β) (30.50% and 18.96%), suggesting that RCNprotac (γ) was more stable and prevented excessive drug leakage. To ensure stability without radiation and responsiveness upon exposure to X-ray radiation, RCNprotac (γ) was chosen for further research. A negligible change in the hydrodynamic size was observed when incubating RCNprotac (γ) in Dulbecco's Modified Eagle Medium (DMEM) containing 10% fetal bovine serum (FBS) at 37 °C for 24 h, further demonstrating its excellent colloidal stability (Fig. 1H).
We next explored the release characteristics of RCNprotac (γ) at different radiation doses. As shown in Fig. 1I, RCNprotac (γ) exhibited a radiation dose-dependent release with 28.66% MZ1 release upon exposure to 2 Gy radiation, 39.97% MZ1 release upon exposure to 4 Gy radiation and 53.51% MZ1 release upon exposure to 8 Gy radiation within 72 h. Subsequently, the X-ray triggered release behavior was further explored in the presence of 100 μM H2O2 to mimic the tumor microenvironment (Fig. 1J). Impressively, 76.36% release was detected under H2O2 and 8 Gy X-ray radiation together, which was much higher than that under H2O2 alone (7.42%) and X-ray alone (53.51%), suggesting that the tumor microenvironment could promote the X-ray triggered breakage of diselenide bonds. In addition to MZ1, a small peak of seleninic acid ([M + H]+) with molecular weight 1184 was observed by LC-MS for the RCNprotac-released substance (Fig. S13, ESI†). This is consistent with a previous report revealing that the diselenide bonds were cleaved and oxidized to seleninic acid upon exposure to X-ray radiation.27 It is assumed that seleninic acid promoted the easy hydrolysis of the adjacent ester bond and ultimately led to the release of MZ1 (Fig. S14, ESI†). Overall, we believe that RCNprotac (γ) demonstrated excellent biostability and X-ray triggered MZ1 release.
2.3.
In vitro cytotoxicity and protein degradation studies of RCNprotac
Subsequently, RCNprotac (γ) was referred to as RCNprotac for convenience. MDA-MB-231, a breast cancer cell line with excessive BRD4 expression, was chosen as the cell model.36 Compared with MZ1, RCNprotac showed significantly lower cell cytotoxicity with a half-maximal inhibitory concentration (IC50) of around 238.60 μM in the absence of radiation (Fig. 2A). X-ray radiation alone also showed negligible cytotoxicity to MDA-MB-231 cells (Fig. S15, ESI†). However, after 4 Gy radiation, RCNprotac showed significantly decreased cell viability of MDA-MB-231 with an IC50 of around 7.88 μM, similar to that of MZ1.
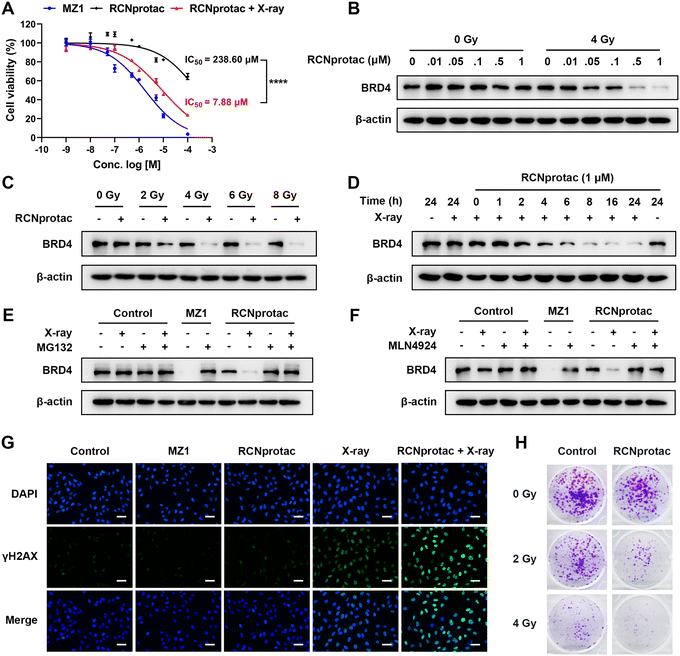 |
| Fig. 2 Activation of RCNprotac upon exposure to X-ray radiation for protein degradation in vitro. (A) Viability of MDA-MB-231 breast cancer cells after treatment with MZ1 or RCNprotac for 24 h with or without 4 Gy X-ray radiation. (B) Western blotting analysis of BRD4 levels in MDA-MB-231 cells treated with the indicated doses of RCNprotac with or without 4 Gy X-ray radiation for 24 h. (C) Western blot analysis of BRD4 levels at various radiation doses after incubation with 1 μM RCNprotac. (D) Time course of BRD4 degradation at 1 μM RCNprotac with 4 Gy X-ray radiation. (E) and (F) Western blot analysis of BRD4 protein after pretreatment with (E) 10 μM MG132 or (F) 3 μM MLN4924 for 2 h, followed by 1 μM RCNprotac treatment with or without 4 Gy X-ray radiation. (G) The representative images of γH2AX fluorescence staining of MDA-MB-231 cells treated with RCNprotac with or without 4 Gy X-ray radiation (scale bar = 50 μm). (H) Colony formation assay of MDA-MB-231 cells subjected to 0 Gy, 2 Gy, or 4 Gy radiation combined with 100 nM RCNprotac. | |
BRD4 is a transcriptional and epigenetic regulator and the silencing of BRD4 could result in the apoptosis of cancer cells.33 We next assessed the X-ray controlled BRD4 protein degradation of RCNprotac by western blot analysis. As illustrated in Fig. 2B, RCNprotac displayed no appreciable proteolytic activity without radiation while the treatment of RCNprotac + X-ray radiation significantly reduced the BRD4 levels in a dose-dependent manner. The BRD4 degradation efficiency of 1 μM RCNprotac + X-ray was similar to that of 0.5 μM MZ1, probably due to the incomplete release of MZ1 from RCNprotac (Fig. S16 and S17, ESI†). Then the radiation dose dependent effect was investigated. As shown in Fig. 2C, 1 μM RCNprotac significantly decreased the BRD4 protein level to 44.60%, 22.76% and 19.73% at radiation doses of 2 Gy, 4 Gy, and 6 Gy, respectively, while no significant BRD4 expression change was observed under radiation alone with the dosage of up to 8 Gy. The kinetics study demonstrated that RCNprotac effectively reduced BRD4 levels in a time-dependent manner after X-ray radiation (Fig. 2D). Considering that MZ1 degrades proteins through E3 ligase mediated ubiquitination and proteasome, the effects of proteasome and neddylation inhibitor were also examined. The results of western blot further revealed that the degradation activity of RCNprotac + X-ray was blocked in the presence of proteasome inhibitor MG132 (Fig. 2E) or neddylation inhibitor MLN4924 (Fig. 2F), confirming that the radiation-initiated proteolytic process by RCNprotac was in the same way as MZ1. Collectively, RCNprotac could be activated by X-ray radiation to release MZ1 for BRD4 proteolysis.
Several studies demonstrated that the BRD4 inhibitor JQ1 sensitizes different types of cancer cells to radiation.34–36 We speculated that RCNprotac can serve as a BRD4 inhibitor to radiosensitize breast cancer cells. Radiation causes double-stranded DNA breaks and phosphorylation of the histone protein H2AX (γH2AX) is thought to be a signal for DNA breaks.37 Immunofluorescence staining revealed that MZ1 or RCNprotac treatment alone had no influence on γH2AX expression (Fig. 2G). In contrast, more γH2AX-positive foci were formed under RCNprotac + X-ray radiation conditions, compared with radiation alone. Western blot analysis further validated the result with a significant higher expression of γH2AX after RCNprotac + X-ray treatment (Fig. S18, ESI†). Subsequently, we used colony formation assay to further determine the radiosensitivity of MDA-MB-231 (Fig. 2H). The RCNprotac + X-ray treatment remarkably decreased colony formation compared to radiation or RCNprotac only. These findings indicated that X-ray activation of RCNprotac improved the radiosensitivity of breast cancer cells. Altogether, we believed that X-ray could trigger the release of MZ1 from RCNprotac to knock down BRD4 for BRD4 deficient apoptosis and enhanced radiosensitization.
2.4.
In vivo antitumor therapy using RCNprotac
The therapeutic effect of X-ray radiation activated RCNprotac was further studied in nude mice bearing subcutaneous MDA-MB-231 tumor. The biodistribution of RCNprotac was first studied by near infrared fluorescence imaging. When the tumor volume reached 200 mm3, mice were intravenously (i.v.) injected with Cy5-labeled MZ1 or RCNprotac (ex. 670 nm, em. 649 nm). As shown in Fig. 3A, MZ1 was rapidly cleared from the body within 4 h with no obvious fluorescence signals at the tumor site. In comparison, the tumor site was gradually lit up within 2 h after the injection of RCNprotac, peaking at 12 h and lasting up to 24 h. The tumor tissue and major organs including heart, liver, spleen, lungs, and kidneys were harvested at 24 h post-injection. The ex vivo fluorescence imaging further confirmed that the RCNprotac signal mainly accumulated in the tumor, liver, and kidneys. These results validated that RCNprotac can passively target tumors due to the EPR effect.
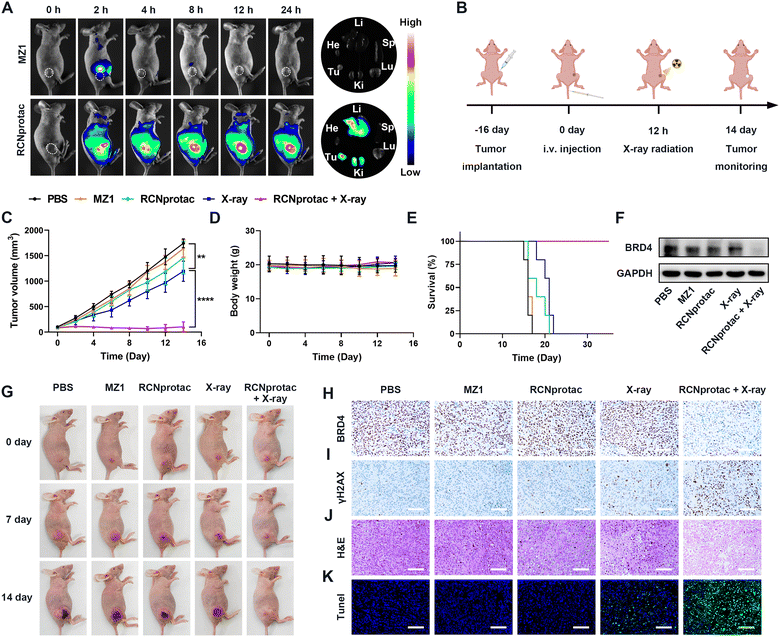 |
| Fig. 3
In vivo biodistribution and antitumor evaluation of RCNprotac. (A) Fluorescence images of MDA-MB-231 tumor-bearing nude mice at different timepoints and isolated organs (heart, liver, spleen, lungs, and kidneys) and tumors at 24 h post injection after intravenous administration of Cy5 labeled MZ1 or RCNprotac. (B) Schematic illustration of the MDA-MB-231 subcutaneous tumor model and the schedule for administration and X-ray radiation. (C) The tumor growth curves of different treatment groups in 14 days (n = 5). (D) The body weight curves of different treatment groups (n = 5). (E) Survival time of MDA-MB-231 tumor-bearing nude mice with different treatments in 35 days (n = 5). (F) Western blot analysis of BRD4 expression in tumor tissues at the end of the treatment. (G) Representative pictures of tumor-bearing nude mice on days 0, 7 and 14 after different treatments. (H)–(I) Representative immunohistochemical staining analysis of (H) BRD4 and (I) γH2AX in tumor sections after various treatments (scale bar = 100 μm). (J) and (K) (J) H&E and (K) TUNEL staining of the tumor tissue sections (scale bar = 100 μm). | |
To study the antitumor performance of RCNprotac, MDA-MB-231 tumor-bearing mice were randomly assigned to five different groups when the tumor volumes reached 100 mm3 and treated with PBS, MZ1, RCNprotac, X-ray (1 Gy), and RCNprotac + X-ray (1 Gy), respectively. RCNprotac or MZ1 was injected intravenously at an MZ1 dose of 6 mg/kg, and radiotherapy was performed at 12 h post-injection as illustrated in Fig. 3B. The MZ1 group barely inhibited tumor growth, probably due to the poor tumor accumulation (Fig. 3C and G). Since E3 ubiquitin ligase is covered in RCNprotac, it barely showed therapeutic effects even when effectively reaching tumor sites. The X-ray group exhibited a slight tumor suppression with 24.28% reduction in tumor volume during the study period. By contrast, the RCNprotac + X-ray group significantly inhibited tumor growth with a 92.61% reduction in tumor volume. Furthermore, the tumor weights (Fig. S19, ESI†) and survival curves (Fig. 3E) of the mice demonstrated that X-ray-activated RCNprotac synergistically with radiotherapy had a pronounced tumor suppressive effect and significantly prolonged the survival time of the mice.
Mice were sacrificed after 14 days of treatment and the dissected tumor tissues were analyzed. Both western blot and BRD4 immunohistochemical examination demonstrated a remarkable decrease of BRD4 expression in the RCNprotac + X-ray group, while other groups failed to affect BRD4 levels, confirming radiation-activated protein degradation by RCNprotac in vivo (Fig. 3F, H and Fig. S20, ESI†). Additionally, RCNprotac + X-ray greatly upregulated tumor γH2AX expression compared with the X-ray group, indicating that the decreased expression level of BRD4 could significantly radiosensitize MDA-MB-231 breast tumor (Fig. 3I). Notably, hematoxylin and eosin (H&E, Fig. 3J) staining and terminal deoxynucleotidyl transferase dUTP nick end labeling (TUNEL, Fig. 3K) indicated more obvious cell apoptosis and necrosis in the RCNprotac + X-ray group. All these results revealed that X-ray radiation activated RCNprotac specifically degraded the tumor BRD4 protein and the reduction of BRD4 protein radiosensitized tumor cells for more apoptosis to effectively inhibit tumor growth.
Moreover, mice treated with RCNprotac + X-ray did not suffer from obvious systemic side effects or significant body weight loss during the treatment period (Fig. 3D). To further assess the biosafety of RCNprotac + X-ray, the major organs (heart, liver, spleen, lungs, and kidneys) were extracted for H&E staining. No visible histological damage was observed on the sections (Fig. S21, ESI†). Besides, the serum biochemical indicators of liver, kidney, and myocardial functions were all within the standard range (Fig. S22, ESI†). Taken together, RCNprotac combined with X-ray radiation could provide an effective and safe strategy for anti-tumor therapy.
3. Conclusions
In summary, we developed a novel X-ray-activatable RCNprotac to synergistically integrate radiotherapy with protein degradation-mediated radiosensitization to optimize antitumor therapy. RCNprotac was readily prepared by self-assembly of an amphiphilic conjugate obtained by connecting MZ1 and hydrophilic PEG via a diselenide bond containing carbon chain. By covering E3 ubiquitin ligase, RCNprotac remained stable under normal physiological conditions, reducing its potential systemic toxicity. Meanwhile, the PEG modification of RCNprotac improved the biodistribution and circulation for enhanced tumor accumulation. In the presence of X-ray radiation, selenide bonds in RCNprotac were broken and effectively released MZ1 for degradation of BRD4 protein, which radiosensitizes tumor cells, exerting synergistic suppression of tumor growth with radiotherapy. Overall, our study provides a new approach for the precise X-ray regulation of PROTAC for enhanced cancer radiotherapy and biosafety.
4. Experimental section
4.1. Materials
All chemical reagents were of reagent grade and were used as received. 4-Dimethylaminopyridine (DMAP), trifluoroacetic acid (TFA), and N-(3-dimethylaminopropyl)-N′-ethyl carbodi-imide hydrochloride (EDCI, 98%) were purchased from Sigma-Aldrich. mPEG2000-NH2 was purchased from ToYongBio. tert-Butyloxycarbonyl-11-amino-3,6,9-trioxaundecanoic acid (Boc-NH-PEG3-CH2-COOH), tert-butyl 2-bromoacetate, t-butyl 4-bromobutanoate, tert-butyl 3-bromopropionate and 3-methyl-L-valyl-(4R)-4-hydroxy-N-[4-(4-methyl-1,3-thiazol-5-yl)benzyl]-L-prolinamide ((S,R,S)-AHPC) were purchased from Macklin. O-(7-azabenzotriazol-1-yl)-N,N,N′,N′-tetramethyluronium hexafluorophosphate (HATU) and sodium borohydride (NaBH4) were purchased from Innochem. N,N-Diisopropylethylamine (DIPEA) was purchased from Aladdin. [(6S)-4-(4-chlorophenyl)-2,3,9-trimethyl-6H-thieno[3,2-f][1,2,4] triazolo[4,3-a][1,4]diazepin-6-yl]acetic acid (JQ1-COOH) was purchased from Bidepharm. The 3-(4,5-dimethylthiazol-2-yl)-2,5-diphenyltetrazolium bromide (MTT) kit was obtained from KeyGen Biotech. Co. Ltd. The Gamma H2A.X Staining Kit (Ab242296) was obtained from Abcam. Crystal violet was obtained from J&K Scientific.
4.2. Preparation and characterization of RCNprotac (α), (β), and (γ)
2 mg of RCNprotac (α), (β), and (γ) precursors were respectively dissolved in 100 μL of DMSO and the mixture was then respectively dropwise added into 1 mL of deionized (DI) water under vigorous stirring (1000 rpm). The precursors were allowed to self-assemble for 6 h at room temperature and the solution was dialyzed against pure water (MWCO: 2000 Da) for 24 h to remove organic solvent to obtain RCNprotac (α), RCNprotac (β), and RCNprotac (γ). The hydrodynamic diameter and polydispersity index (PDI) of nanomicelles were measured using a Zetasizer instrument (Nano ZS90, Malvern, UK), and the morphology was observed using transmission electron microscopy (TEM, JEOL 1200EX, Japan).
4.3. The MZ1 release profiles of three nanomicelles
The release profiles of MZ1 from RCNprotac (α), RCNprotac (β) and RCNprotac (γ) were evaluated. The pH 7.4 PBS solution containing 30% (v/v) ethanol was used as the release medium. After receiving the indicated dose of radiation, the suspensions of three nanomicelles (150 nmol, MZ1 equivalent) were sealed in a dialysis tube (MWCO 2000 Da) and gently shaken at 37 °C in 15 mL of the medium, with or without 100 μM H2O2 or 1 mM GSH. At the predetermined time points, the amount of the released MZ1 was examined by HPLC measurement (mobile phase: A: ACN, B: H2O with 0.1% TFA; flow rate: 0.5 mL min−1; column temperature: 25 °C; UV: 254 nm; elute gradient: 0–20 min, from 5% A to 100% A; injection volume: 4 μL). The molecular structure of the released MZ1 was confirmed by LC-MS (Agilent 1260 Infinity II, Agilent, USA).
4.4. Nanomicelle stability
To investigate the stability of the prepared RCNprotac, 100 μL of nanomicelles (200 μmol) were respectively diluted in 1.5 mL of DMEM containing 10% of FBS. The particle sizes, after incubating at 37 °C, were measured using a Zetasizer at various time points (0, 1, 2, 4, 8, 12, and 24 h).
4.5. Cell culture
MDA-MB-231 cells were maintained in DMEM (Gibco) supplemented with 10% (v/v) FBS, 100 units/mL penicillin and 100 μg mL−1 streptomycin. All cells were cultured under a humidified atmosphere of 5% CO2 at 37 °C.
4.6. Cytotoxicity assay
The cytotoxicity of RCNprotac with or without X ray radiation against MDA-MB-231 cells was evaluated by MTT assay. MDA-MB-231 cells were seeded into a 96-well plate and incubated overnight. Then, the medium was substituted with fresh medium including different concentrations of free MZ1 or RCNprotac. For the RCNprotac + X-ray group, cells were pretreated with RCNprotac for 2 h and then subjected to irradiation using a biological irradiator (CellRad, Precision X-ray, USA) at a dose of 4 Gy X-ray radiation. After 24 h, 100 μL of MTT solution (1 mg mL−1) was added into each well and kept for another 4 h. Then, the obtained formazan was dissolved in 200 μL of dimethyl sulfoxide (DMSO) and the absorbance at 490 nm was detected using a microplate reader (Multiskan Sky, Thermo Fisher Scientific, USA). Each measurement was repeated three times.
4.7. Colony formation assay
MDA-MB-231 cells were seeded in a 6-well plate at a density of 1200 cells per well and incubated for 24 h for cell attachment. Then, the cells were divided into six groups (control, 2 Gy, 4 Gy, 100 nM RCNprotac, 100 nM RCNprotac + 2 Gy, and 100 nM RCNprotac + 4 Gy) and treated accordingly. After treating for 24 h, the cells were further cultured in normal medium for an additional 10 days to allow colony formation, during which the medium was replaced with fresh medium every 3 days. Then, the colonies were fixed with 1 mL 4% paraformaldehyde for 1 h at 4 °C and stained with fresh 1% crystal violet for 5 min at room temperature. The plates were washed with PBS, air dried, and scanned for photographs.
4.8. Western blot
MBA-MB-231 cells were seeded into a 6-well plate and incubated overnight. Different concentrations of RCNprotac were added to the cells, and then they were irradiated with or without X-ray after 2 h of incubation. After incubating for the indicated times, the cells were lysed with RIPA lysis buffer (KGP702-100, Keygen Biotech Co., Ltd) containing 1 mM phenylmethanesulfonylfluoride (PMSF), and the protein lysate was obtained by centrifugation at 12
000 g for 15 min at 4 °C. Subsequently, the protein was determined by BCA protein assay (E112-01, Vazyme Biotech Co., Ltd), denatured at 100 °C, separated by SDS-polyacrylamide gel electrophoresis (SDS-PAGE), and transferred onto a polyvinylidene difluoride (PVDF) membrane. The membranes were then blocked with 5% (w/v) non-fat dry milk for 2 h, followed by overnight incubation with primary antibodies at 4 °C and for 1 h with the corresponding secondary antibodies at 37 °C. The target protein bands were visualized using a TanonTM ECL Chemiluminescent Substrate (Tanon, Shanghai) and imaged with a Tanon 4600 SF (Tanon, Shanghai) using automated exposure settings. For BRD4 protein detection in tumor tissues, harvested tumor tissues were frozen and lysed with lysis solution by milling and centrifugation to obtain the tumor lysate. The subsequent steps were the same as the cell lysate. Anti-beta actin (1
:
5000, 66
009-1-Ig, Proteintech), anti-BRD4 (1
:
1000, ab128874, Abcam), anti-γH2AX (1
:
2000, 05-636, Sigma-Aldrich/Merck), anti-GAPDH (1
:
50
000, 60
004-1-Ig, Proteintech), anti-rabbit IgG (1
:
2000, 7074, Cell Signaling), and anti-mouse IgG (1
:
2000, 7076, Cell Signaling) were used. All antibodies were prepared in TBST containing 5% non-fat dry milk.
4.9. Immunofluorescence
Cells plated in a 96-well plate were treated with 1 μM MZ1 or RCNprotac and then irradiated with 4 Gy. After incubation for 4 h, the cells were washed with PBS and fixed with 4% paraformaldehyde for 15 min at room temperature before being subjected to permeabilization with 100% methanol at 4 °C for 10 min. Then, the cells were blocked with 1% BSA/PBS buffer at 37 °C for 30 min and incubated with the anti-phospho-histone H2A.X antibody at 37 °C (1
:
100 in 1% BSA/PBS) for 1 h. After washing with PBST (0.05% Tween-20/PBS), the cells were incubated with the secondary antibody (1
:
100 FITC conjugate solution) diluted with 1% BSA/PBS at 37 °C for 1 h. Washing with PBST washing buffer was followed by 10 min of counterstaining with DAPI, and the immunofluorescence staining was visualized and photographed using a Nikon ECLIPSE Ts2 (Nikon, Japan).
4.10. Tumor model
Female BALB/c nude mice (5 weeks) were purchased from Huachuang Sino Co. Ltd (Jiangsu, China) and housed in a temperature controlled (25 °C) environment with a 12 h light–dark cycle and free access to standard food and water. To build mice tumor models, 100 μL of MDA-MB-231 cell suspension (8 × 106 cells/mouse) were injected subcutaneously into the right fat pad of the nude mice. The mice were used to evaluate in vivo biodistribution and therapeutic effects 16 days after tumor cell inoculation. All animal procedures, which were approved by China Pharmaceutical University, were carried out in accordance with the Guide for Care and Use of Laboratory Animals.
4.11.
In vivo biodistribution
Mice were injected intravenously with Cy5-labelled MZ1 or RCNprotac when their tumor volumes reached 200 mm3, and fluorescence images were captured at the predetermined time points. Eventually, the mice were sacrificed and major organs (heart, liver, spleen, lungs, and kidneys) and tumor tissues were extracted at 24 h post-injection for ex vivo fluorescence imaging.
4.12.
In vivo antitumor efficiency
When the tumor volume reached 100 mm3, the mice were assigned into five groups randomly (n = 5 for each group) and then intravenously injected with PBS, MZ1, and RCNprotac at the identical MZ1 dose of 6.0 mg kg−1. Twelve hours post injection, mice of the X-ray group and the RCNprotac + X-ray group received 1 Gy of X-ray radiation to the tumor, while the rest of the body was shielded with lead. Tumor volumes and body weights were monitored every 2 days, and the volumes were calculated using the following formula: Tumor volume (mm3) = length × width2/2. The mice were sacrificed after 14 days of treatment, and the tumor tissues were collected, weighed, and sectioned for pathological analysis (H&E, TUNEL, BRD4, and γH2AX staining). Additionally, the tumor lysate was also analyzed for BRD4 content by western blotting.
4.13. Biosafety studies
To investigate the biosafety of RCNprotac, blood and main organs of mice (including the heart, lungs, liver, kidneys, and spleen) were collected after 14 days of different treatments. The blood was used for the potential hepatic, renal and heart toxicity assessment, and the organs were fixed and stained with H&E.
4.14. Statistical analysis
Data were processed with GraphPad Prism 9.0 and represented as mean ± SD. The experimental data were statistically calculated using one-way analysis of variance (ANOVA) with a Tukey post-hoc test. p-values less than 0.05 were considered to be statistically significant; *p < 0.05, **p < 0.01, ***p < 0.001, ****p < 0.0001; ns, no significance (p > 0.05).
Author contributions
X. S. conceived the concept. X. S. and W. X. supervised the study. M. X. and X. S. designed the research. M. X. carried out the synthesis of compounds and performed in vitro experiments. M. X. and Y. Y. carried out cell experiments. M. X. carried out animal experiments and Y. Y. helped with tumor model establishment. C. L. helped with synthesis of compounds. M. X. and X. S. prepared the manuscript. Y. R. contributed to the improvement of the manuscript. O.M. revised the manuscript. All authors have given approval to the final version of the manuscript.
Conflicts of interest
There are no conflicts to declare.
Acknowledgements
This work was supported by the National Key R&D Program of China (2022YFE0105400), the National Natural Science Foundation of China (82272138, 81971738), the Jiangsu Province Outstanding Youth Fund (BK20220086), and the Double First-Class Fund, China Pharmaceutical University (CPUQNJC22_03).
Notes and references
- Y. Chen, I. Tandon, W. Heelan, Y. X. Wang, W. P. Tang and Q. Y. Hu, Chem. Soc. Rev., 2022, 51, 5330–5350 RSC.
- X. Y. Li, W. C. Pu, Q. Q. Zheng, M. Ai, S. Chen and Y. Peng, Mol. Cancer, 2022, 21, 99 CrossRef CAS.
- D. A. Nalawansha and C. M. Crews, Cell Chem. Biol., 2020, 27, 998–1014 CrossRef CAS.
- D. Chirnomas, K. R. Hornberger and C. M. Crews, Nat. Rev. Clin. Oncol., 2023, 20, 265–278 CrossRef CAS.
- S. X. Zeng, Y. Q. Ye, H. Y. Xia, J. L. Min, J. M. Xu, Z. Y. Wang, Y. L. Pan, X. L. Zhou and W. H. Huang, Eur. J. Med. Chem., 2023, 261, 115793 CrossRef CAS.
- J. Gao, B. Hou, Q. W. Zhu, L. Yang, X. Y. Jiang, Z. F. Zou, X. T. Li, T. F. Xu, M. Y. Zheng, Y. H. Chen, Z. A. Xu, H. X. Xu and H. J. Yu, Nat. Commun., 2022, 13, 4318 CrossRef CAS.
- S. Shi, Y. Du, Y. Zou, J. Niu, Z. Y. Cai, X. N. Wang, F. H. Qiu, Y. Ding, G. C. Yang, Y. Z. Wu, Y. G. Xu and Q. H. Zhu, J. Med. Chem., 2022, 65, 5057–5071 CrossRef CAS.
- J. Liu, H. Chen, Y. Liu, Y. D. Shen, F. Y. Meng, H. Ü. Kaniskan, J. Jin and W. Y. Wei, J. Am. Chem. Soc., 2021, 143, 7380–7387 CrossRef CAS.
- J. Liu, H. Chen, L. N. Ma, Z. X. He, D. Wang, Y. Liu, Q. Lin, T. H. Zhang, N. Gray, H. Ü. Kaniskan, J. Jin and W. Y. Wei, Sci. Adv., 2020, 6, eaay5154 CrossRef CAS.
- G. Xue, K. Wang, D. L. Zhou, H. B. Zhong and Z. Y. Pan, J. Am. Chem. Soc., 2019, 141, 18370–18374 CrossRef CAS.
- C. Yang, Y. Yang, Y. Li, Q. Ni and J. Li, J. Am. Chem. Soc., 2022, 145, 385–391 CrossRef.
- D. Schaue and W. H. McBride, Nat. Rev. Clin. Oncol., 2015, 12, 527–540 CrossRef.
- T. Nakano, K. Akamatsu, M. Tsuda, A. Tujimoto, R. Hirayama, T. Hiromoto, T. Tamada, H. Ide and N. Shikazono, Proc. Natl. Acad. Sci. U. S. A., 2022, 119, e2119132119 CrossRef CAS.
- Y. Wu, Y. Q. Song, R. Z. Wang and T. L. Wang, Mol. Cancer, 2023, 22, 96 CrossRef CAS.
- X. Y. Zhong, X. W. Wang, G. T. Zhan, Y. A. Tang, Y. Z. Yao, Z. L. Dong, L. Q. Hou, H. Zhao, S. J. Zeng, J. Hu, L. Cheng and X. L. Yang, Nano Lett., 2019, 19, 8234–8244 CrossRef CAS.
- L. Song, P. P. Li, W. Yang, X. H. Lin, H. Liang, X. F. Chen, G. Liu, J. Li and H. H. Yang, Adv. Funct. Mater., 2018, 28, 1707496 CrossRef.
- J. Geng, Y. C. A. Zhang, Q. Gao, K. Neumann, H. Dong, H. Porter, M. Potter, H. Ren, D. Argyle and M. Bradley, Nat. Chem., 2021, 13, 805–810 CrossRef CAS PubMed.
- Q. F. Fu, H. Y. Li, D. B. Duan, C. L. Wang, S. Y. Shen, H. M. Ma and Z. B. Liu, Angew. Chem., Int. Ed., 2020, 59, 21546–21552 CrossRef CAS.
- H. Z. Deng, L. S. Lin, S. Wang, G. C. Yu, Z. J. Zhou, Y. J. Liu, G. Niu, J. B. Song and X. Y. Chen, Adv. Mater., 2019, 31, e1903443 CrossRef PubMed.
- X. F. Chen, J. B. Song, X. Y. Chen and H. H. Yang, Chem. Soc. Rev., 2019, 48, 3073–3101 RSC.
- J. S. Kang, P. P. DeLuca and K. C. Lee, Expert Opin. Emerging Drugs, 2009, 14, 363–380 CrossRef CAS.
- M. S. Gadd, A. Testa, X. Lucas, K. H. Chan, W. Z. Chen, D. J. Lamont, M. Zengerle and A. Ciulli, Nat. Chem. Biol., 2017, 13, 514–521 CrossRef CAS.
- M. Zengerle, K. H. Chan and A. Ciulli, ACS Chem. Biol., 2015, 10, 1770–1777 CrossRef CAS.
- M. D. Ni, J. J. Li, H. Y. Zhao, F. Xu, J. Y. Cheng, M. Yu, G. H. Ke and X. H. Wu, Oncogene, 2021, 40, 2711–2724 CrossRef CAS.
- L. Yang, Y. Y. Zhang, W. W. Shan, Z. Y. Hu, J. Yuan, J. J. Pi, Y. Y. Wang, L. L. Fan, Z. Q. Tang, C. S. Li, X. W. Hu, J. L. Tanyi, Y. Fan, Q. H. Huang, K. Montone, C. V. Dang and L. Zhang, Sci. Transl. Med., 2017, 9, eaal1645 CrossRef.
- J. Wang, Y. Wang, H. Mei, Z. Y. Yin, Y. Y. Geng, T. Zhang, G. Wu and Z. Y. Lin, Cancer Lett., 2017, 391, 141–151 CrossRef CAS.
- D. Shao, F. Zhang, F. M. Chen, X. Zheng, H. Z. Hu, C. Yang, Z. X. Tu, Z. Wang, Z. M. Chang, J. N. Lu, T. Y. Li, Y. Zhang, L. Chen, K. W. Leong and W. F. Dong, Adv. Mater., 2020, 32, e2004385 CrossRef.
- L. X. Zhang, S. T. Zhang, J. Y. Xu, Y. Y. Li, J. L. He, Y. Yang, T. Huynh, P. H. Ni, G. X. Duan, Z. X. Yang and R. H. Zhou, Acs Appl. Mater. Interfaces, 2020, 12, 43398–43407 CrossRef CAS.
- B. J. Sun, C. Luo, X. B. Zhang, M. R. Guo, M. C. Sun, H. Yu, Q. Chen, W. Q. Yang, M. L. Wang, S. Y. Zuo, P. Y. Chen, Q. M. Kan, H. T. Zhang, Y. J. Wang, Z. G. He and J. Sun, Nat. Commun., 2019, 10, 3211 CrossRef.
- S. Y. Zuo, B. J. Sun, Y. X. Yang, S. Zhou, Y. Zhang, M. R. Guo, M. C. Sun, C. Luo, Z. G. He and J. Sun, Small, 2020, 16, e2005039 CrossRef.
- M. Binnewies, E. W. Roberts, K. Kersten, V. Chan, D. F. Fearon, M. Merad, L. M. Coussens, D. I. Gabrilovich, S. Ostrand-Rosenberg, C. C. Hedrick, R. H. Vonderheide, M. J. Pittet, R. K. Jain, W. P. Zou, T. K. Howcroft, E. C. Woodhouse, R. A. Weinberg and M. F. Krummel, Nat. Med., 2018, 24, 541–550 CrossRef CAS.
- J. R. Wang, X. R. Sun, W. W. Mao, W. L. Sun, J. B. Tang, M. H. Sui, Y. Q. Shen and Z. W. Gu, Adv. Mater., 2013, 25, 3670–3676 CrossRef CAS.
- J. Li, Y. Gu, W. Zhang, C. Y. Bao, C. R. Li, J. Y. Zhang, T. Liu, S. Li, J. X. Huang, Z. G. Xie, S. C. Hua and Y. Wan, Int. J. Biol. Sci., 2019, 15, 1755–1770 CrossRef CAS.
- G. Q. Ma, J. Liu, J. L. He, M. Z. Zhang and P. H. Ni, ACS Biomater. Sci. Eng., 2018, 4, 2443–2452 CrossRef CAS.
- N. Ma, Y. Li, H. P. Xu, Z. Q. Wang and X. Zhang, J. Am. Chem. Soc., 2010, 132, 442–443 CrossRef CAS PubMed.
- J. F. Hu, D. Pan, G. Li, K. Q. Chen and X. M. Hu, Cell Death Dis., 2022, 13, 1059 CrossRef CAS PubMed.
- A. Ivashkevich, C. E. Redon, A. J. Nakamura, R. F. Martin and O. A. Martin, Cancer Lett., 2012, 327, 123–133 CrossRef CAS.
Footnotes |
† Electronic supplementary information (ESI) available: 1H-NMR and MS spectra and other relevant figures. See DOI: https://doi.org/10.1039/d3tb03046f |
‡ These authors contributed equally. |
|
This journal is © The Royal Society of Chemistry 2024 |
Click here to see how this site uses Cookies. View our privacy policy here.