DOI:
10.1039/D4TB01433B
(Paper)
J. Mater. Chem. B, 2024,
12, 10309-10319
RNA-binding peptide and endosomal escape-assisting peptide (L2) improved siRNA delivery by the hexahistidine–metal assembly†
Received
30th June 2024
, Accepted 3rd September 2024
First published on 4th September 2024
Abstract
Small interfering RNAs (siRNAs), comprising 21–23 nucleotides, function by complementary binding to specific mRNA sequences, thereby suppressing target protein expression. Despite their vast potential in disease therapy, siRNAs face challenges due to their susceptibility to degradation and high electronegativity, rendering them unstable in the bloodstream and impeding their passage across endothelial barriers. Moreover, successful intracellular delivery necessitates overcoming endosomal entrapment, posing a significant hurdle for carrier material development. In this study, leveraging the strong affinity of histidine oligomers (His6) for metal ions, we engineered nanoparticles (HmA) by gentle assembly with divalent zinc ions under pH = 8 conditions. We designed the RNA-binding functional peptide L2-NTD to enhance siRNA stability and delivery efficiency when complexed with HmA. The resulting siRNA+L2-NTD@HmA nanoparticles were formed via in situ encapsulation, ensuring efficient siRNA delivery into cells with minimal cytotoxicity and degradation. This approach presents a novel strategy for the design and artificial fabrication of carriers for effective RNA delivery.
1. Introduction
The delivery of siRNAs for therapeutic purposes is hindered by various challenges primarily due to the unique pharmacological properties of siRNAs themselves. SiRNAs are relatively large molecules with high anionic charge densities, making it difficult for them to traverse cellular membranes. Moreover, their instability in the bloodstream and potential to trigger immune responses further complicate delivery.1 To be effective, intravenously administered siRNAs must overcome multiple barriers, including crossing vascular endothelial barriers, avoiding kidney filtration, and evading uptake by non-target cells. Once inside target cells, they must escape endosomal entrapment and reach the RNA interference machinery intact. These challenges necessitate siRNAs to be resistant to nuclease degradation while maintaining appropriate size and charge characteristics.2
Moreover, efficient siRNA delivery also requires carriers with a controlled size (between 20 and 200 nm) and positively charged surfaces to complex with the negatively charged siRNAs.3 However, overly positively charged carriers are prone to clearance by the bloodstream and the liver. Additionally, intracellular barriers such as endosomal entrapment and cytoplasmic diffusion further reduce delivery efficiency. While various siRNA delivery vectors have been investigated, they often suffer from shortcomings in terms of efficiency and cytotoxicity. Thus, there is a critical need for developing safer and more efficient delivery vectors to advance the practical application of siRNA therapeutics.4–7
Polyhistidine tags are frequently used in protein purification due to their high affinity for various metal ions, particularly in immobilized metal ion chromatography.8 The interaction between proteins and zinc ions has been extensively studied, leading to investigations into hierarchical assembly processes involving zinc and polyhistidine – modified materials. Recent research has focused on multicomponent coordination self-assembly strategies, resulting in the development of smart nanomedicines capable of tumor-specific delivery and therapeutic efficacy.9 Additionally, protein particles assembled from histidine-rich proteins and divalent metal ions have shown promise. Chen's group has introduced a method for fabricating His6-metal assembly (HmA) under mild conditions, offering advantages such as high drug loading capacity, compatibility with a wide range of drugs, low cytotoxicity, rapid internalization, lysosome evasion, and fast intracellular drug release.4 These characteristics make HmA appealing for various biomedical applications, for example, it has displayed a distinguished ability to encapsulate proteins, peptides, and small molecules.10 However, encapsulating siRNA within HmA has been challenging due to the difference in preparation conditions. The ultrasonic conditions required for HmA construction can lead to siRNA degradation. To address this issue, in the present study, an RNA-binding peptide (L2-NTD) in combination with an endosomal escape-assisting peptide (L2), L2-NTD, was designed to complex to siRNA initially, protecting it from degradation. This peptide–siRNA complex is then co-assembled with hexahistidine and metal ions to form the siRNA+L2-NTD@HmA assembly, thereby enhancing the stability and delivery efficiency of siRNA. This approach combines the advantages of HmA and peptide protection for siRNA, demonstrating potential for effective siRNA delivery into living cells.
Coronavirus, as an RNA virus, possesses a large genome of approximately 30 kilobases encoding several structural and non-structural proteins essential for its replication within host cells. Among these proteins, nucleocapsid phosphoproteins (N) play a crucial role in binding the viral genetic material RNA.11–14 The N proteins, which are homologous across different coronaviruses, consist of various structural domains, including the N-terminal structural domain (NTD), which is particularly important for RNA binding. Several studies have shown that NTD can specifically bind to a variety of RNAs,15,16 in particular, it is shorter than the traditional RNA binding peptides and easily available with high production.17,18 Considering its high production and binding efficiency to RNA, it was chosen as a binding peptide for siRNA in this study. On the other hand, human papillomavirus (HPV) is a non-enveloped double-stranded DNA virus characterized by a capsid containing major (L1) and minor (L2) capsid proteins.19 A segment of the L2 N-terminus (aa:396-439) aids HPV entry into cells and facilitates endosomal escape.20,21 To address the challenge of siRNA delivery, a multifunctional small peptide L2-NTD was constructed by linking the L2 peptide from HPV 16 to the NTD of coronavirus N protein (SARS-COV-2 GenBank: QHD43423.2). This fusion peptide aims to maintain siRNA stability, enhance its encapsulation rate in HmA, and improve delivery efficiency for both transmembrane and endosomal release. Upon validation, the siRNA+L2-NTD@HmA assembly demonstrated efficient siRNA delivery into cells with minimal cytotoxicity and degradation. This innovative approach offers a novel strategy for designing and fabricating carriers tailored for effective RNA delivery, potentially contributing to advancements in RNA therapeutics and viral infection treatments (Scheme 1).
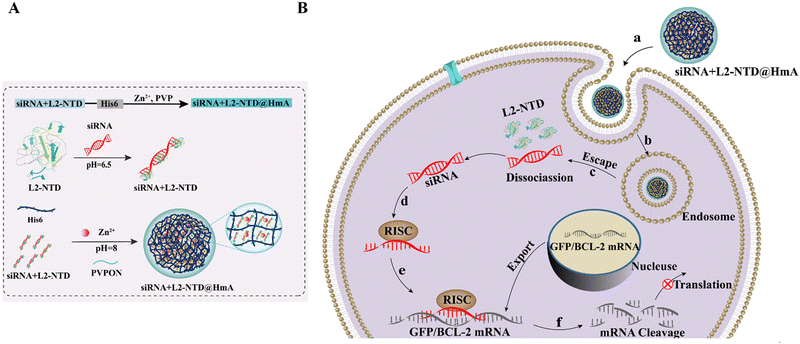 |
| Scheme 1 Schematic diagram of (A) construction of siRNA–L2-NTD@HmA assembly and (B) the targeted delivery of siRNA to tumor cells, with the aim of interfering with specific tumor-associated RNA molecules. | |
2. Materials and methods
2.1. Materials and equipment
Tryptone, yeast powder, and agar powder used in LB solid and liquid media were purchased from OXOID (UK). HEPES, NaCl, phosphate, and other solid drugs used in the experiment were purchased from Aladdin Chemical Co. Tris base was purchased from Sigma-Aldrich Company. Dithiothreitol (DTT), ethylenediaminetetraacetic acid (EDTA), kanamycin, isopropyl-β-D-thiogalactopyranoside (IPTG), sodium dodecylsulfonate (SDS), tetramethylethylenediamine (TEMED), bromophenol blue, komatsuglaze blue, and β-mercaptoethanol were all purchased from Genview. Receptor cells TransT1, BL21(DE3), and pLysS were purchased from Bio-Rad. Fluorescein isothiocyanate (FITC 96% 5- and 6-isomerization mixture) was purchased from Shanghai McLean Biochemical Technology Co. A plasmid extraction kit was purchased from Tengen Biochemical Technology (Beijing) Company Limited.
Serum used for cell culture was purchased from Sevier Biotechnology Co. The trypsin used to digest the cells was purchased from Ebixon (Shanghai) Biotechnology Co. TK-14 (TNTKRSAKRKRSLK) peptide was purchased from Shanghai Chupeptide Biotechnology Co. HmA vector particles were synthesized with reference to the methods reported in the literature.22 Besides, siRNA against BCL-2 (siBCL-2) was purchased from Shanghai Integrated Biotech Solutions Co. The sequences of siRNA are described as follows: for siRNA: sense strand = 5′-GCAUGCGACCUCUGUUUGATT-3′; antisense strand = 5′-UCAAACAGAGGUCGCAUGCTT-3′.23 While siRNA against GFP (siGFP) was also purchased from Shanghai Integrated Biotech Solutions Co., Ltd. The sequences of siGFP are described as follows: sense of 5′-CAAGCUGACCCUGAAGUUCTT-3′; and antisense of 3′-AACUUCAGGG-UCAGCUUGCC-3′.24
The protein purification system model is ÄKTA, from GE. The ultrasonic disruptor is VCX750 from UIBRA CELL. Besides, scanning electron microscopy (SEM) measurements and energy dispersive spectroscopy (EDS) were performed using a Hitachi Regulus 8100. The fluorescence inverted microscope uses the Olympus IX73PIF model. The gel imager is JY04S-3H from UVP, and the chemiluminescence imaging system is Tanon 5200 from PerkinElmer Inc. The flow cytometer is BD Accuri C6 from BD, and the carbon dioxide incubator model is MCO-15AC, from SANYO.
2.2. L2-NTD expression and purification
The plasmid of L2-NTD was synthesized by Genscript Biotechnology Co. and placed in 100 mL of LB medium for overnight incubation at 37 °C. It was then inoculated into 1000 mL of kanamycin-resistant LB liquid medium at a ratio of 1
:
200 and incubated at 37 °C until the OD600 value reached 0.6–0.8. After adding IPTG (0.2 mmol L−1), the expression was induced at 18 °C for 12–16 h. The bacteria were collected by centrifugation at 4000 rpm for 30 minutes. The supernatant was discarded, and the precipitate was resuspended in 1 × PBS (1 g of bacteria in 10 mL of PBS). The bacteria were then lysed by sonication on ice. The supernatant and precipitate (inclusion bodies) were separated and resuspended in equal volumes of PBS and analyzed by SDS-PAGE. The L2-NTD protein was purified using a 1 mL column volume of Ni-crystarose FF and an AKTA Purifier instrument. Gradient elution was performed with eluents at 10%, 20%, 40%, 60%, and 80%, and the fractions were collected and identified by SDS-PAGE and western blot.
2.3. Electrophoresis mobility shift (EMSA)
The siRNA–peptide complexes were identified using the horizontal EMSA analysis method.25 Briefly, a 5% non-denaturing polyacrylamide gel (acrylamide, 29
:
1) was prepared with electrophoresis buffer (30 mM MOPS). The mixture was poured into a UV-cleaned gel tray (100 mm × 70 mm × 20 mm, final thickness 10 mm) with a comb inserted into the center of the gel. After polymerization, the gel was transferred into a horizontal electrophoresis system, filled with electrophoresis buffer, and equilibrated at 4 °C for 1 hour (100 V). Peptide and siRNA were mixed in binding buffer (20 mM phosphate, 100 mM NaCl, 1 mM DTT, pH 6.5) and incubated on ice for 30 minutes. The samples were then mixed with 1/6 volume of 6× loading buffer (30% glycerol, 0.25% xylene cyanol, 0.25% bromophenol blue). The samples were loaded onto the gel and electrophoresed at 4 °C for 15–30 minutes (100 V). The gel was immersed in distilled water containing 1 μg mL−1 EtBr for 1 hour. The results were observed using a gel imaging system. Subsequently, the gels were immersed in 100 mL aqueous solution containing 10% acetic acid and 50% methanol for 1 hour. The gels were then stained with Coomassie Brilliant Blue overnight and decolorized with 10% acetic acid and 50% methanol until the gels were colourless. The results were observed.
2.4. FITC-labelling on peptides
As a stock solution, 1 mg of FITC was dissolved in 1 mL DMSO, while the peptide was dissolved in carbonate buffer (pH 9.0) to get a concentration of 1 mg mL−1. All small peptides to be labelled with FITC were conducted at 4 °C. For that, 50 μL FITC solution was added dropwise to 1 mL peptide solution under stirring. After an overnight reaction at 4 °C, the solution was transferred into a dialysis bag (1 kDa) and dialyzed in water for 2 days to remove free FITC. The solution was then freeze-dried to obtain powders of FITC-tagged peptides (FITC-L2NTD, FITC-TK-14, and FITC-BSA), and stored at −80 °C freezer prior to use.26
2.5. Construction of the FITC-L2-NTD@HmA assembly
The assembly of FITC-L2-NTD@HmA was carried out using the literature method.4 In brief, the concentration of L2-NTD was 1 mg mL−1, and 400 μL of a mixed solution of His6 (0.84 mg) and polyvinylpyrrolidone (PVPON, 58 kDa, 1 mg) was prepared and buffered with 50 mM HEPES at different pHs as indicated. The reaction system was placed on ice upon dropwise adding of 20 μL solution of Zn(NO3)2·6H2O (30 mg mL−1) under sonication (300 W, 80 kHz, 25 min). The solution changed from clear and transparent to light orange, which indicated the formation of FITC-L2-NTD@HmA particles. Then the produced pellet was collected by centrifugation (9.17g × 10 min), and the precipitate was washed three times with deionized H2O. Finally, the pellet was stored at 4 °C protected from light, and was sonicated again for 1 min before use.
2.6. Construction of Cy5-siRNA+L2-NTD@HmA
First, L2-NTD and siRNA were mixed proportionally in a binding buffer (20 mM PBS, pH 6.5, 100 mM NaCl, 1 mM DTT), which was then incubated on ice for 30 min to prepare a mixture of peptide, His6 (0.84 mg) and PVPON (58 kDa, 1 mg) and buffered with 50 mM HEPES (pH 8.0) to get a total volume of 400 μL. The reaction system was placed on the ice again upon dropwise adding of 20 μL solution of Zn(NO3)2–6H2O (30 mg mL−1) under sonication (300 W, 80 kHz, 25 min). Once the solution changed from clear and transparent to light orange, it indicated the particle formation of siRNA–L2-NTD@HmA. The siRNA–L2-NTD@HmA pellet was collected by centrifugation (9.17g × 10 min), and the precipitate was washed three times with deionized H2O. Finally, the pellet was stored at 4 °C protected from light, and was sonicated again for 1 min before use. The construction of Cy5-siRNA + TK-14@HmA is the same as for siRNA–L2-NTD@HmA except using TK-14 instead of L2-NTD.
2.7. Ultraviolet-visible (UV-vis) absorption spectroscopy
UV-vis absorption was measured on a PerkinElmer Lambda 365 UV-vis spectrophotometer. The wavelength range was set to 400–900 nm, and 1 mL of the solution to be measured was added to a quartz cuvette (2 mL), while an equal amount of HEPES solution was used as a control.
2.8. Dynamic light scattering (DLS)
DLS measurements were performed using a Malvern Zetasizer Nano-ZS 90 (USA) instrument, which was switched on and warmed up for 30 min before use. 1 mL of the test solution was prepared by passing it through a 0.22 μm microporous filter membrane to exclude larger particles, and then placed in a 2 mL cuvette for DLS testing. The test was repeated three times for each sample to obtain reliable results.
2.9. Cell culture
All cell experiments were performed on a sterile ultra-clean bench. Cell culture medium with serum (complete medium) was configured: 10% FBS, 100 μg mL−1 penicillin, and 100 μg mL−1 streptomycin were added to DMEM. HeLa or 293T cells were added to the culture medium, and incubated in an incubator with humidity and 5% CO2 at 37 °C. When the cells grew to 60% to 80%, they were digested with 0.25% trypsin to be suspended. After being centrifuged at 1000 rpm for 5 min, the supernatant was discarded, and the cells were re-suspended by adding fresh medium for cell passaging or cell spreading.
2.10. Cytotoxicity test on cells
Experimental cells were inoculated at a density of 8000 cells in 96-well plates containing 100 μL complete medium per well. After 24 h incubation, the cells were washed with PBS buffer. Serum-free DMEM medium (empty medium) was re-suspended and re-sonicated to disperse the L2-NTD@HmA pellet in different concentrations, or other materials used as controls. Besides, the untreated cells were used as blank. After 4 h incubation, the medium was aspirated and replaced with complete medium for incubation overnight, and each well was washed three times with PBS buffer before assessing the cell viability using the Cell Counting Kit-8 (CCK-8) method. Three replicates were performed for each testing sample.
2.11. Fluorescence imaging of living cells
First, HeLa cells were inoculated with 5000 cells in 96-well plates, and 100 μL of complete medium was added to each well, and incubated for 24 h. The synthesized HmA vector particles were re-suspended in DMEM, and the cells were washed once with PBS and replaced with empty medium, which was added to the cell culture medium at a concentration of 30 μg mL−1 per well. After incubation for a certain period of time the cells were photographed for cell imaging using an inverted fluorescence microscope.
2.12. Endosomal escape assay
HeLa cells and 293T cells were seeded into 96-well plates at a density of 5 × 103 cells per well and allowed to reach sub-confluence. These cells were washed three times with serum-free DMEM and then incubated with 0.1 mg mL−1 calcein in the presence of 30 μg mL−1 L2-NTD, TK-14, L2-NTD@HmA, TK-14@HmA or 2.5 μL mL−1 Lipo2000 in serum-free DMEM for 4 h at 37 °C. Then cells were washed with PBS buffer three times and imaged with an OLYMPUS fluorescence microscope.
2.13. Flow-cytometry assay
HeLa cells were inoculated in 24-well plates at a density of 1 × 105 cells per well and cultured for 24 h to grow well. Blank cell control, Cy5-siGFP+L2-NTD@HmA, Cy5-siGFP+TK-14@HmA, Cy5 + siGFP@Lip2000, Cy5-siGFP, Cy5s-iGFP @HmA were set up as the test groups for transfection of HeLa cells, respectively. The gradient transfection time was set at 0, 1, 2, 4, and 6 h, respectively. The samples were collected, and subsequently the cells were digested using trypsin. After being centrifuged at 1000 rpm for 5 min to collect the cells in 1.5 mL EP tubes, the centrifuged EP tubes were inverted on cotton to aspirate the supernatant, the centrifugation was washed three times in PBS repeatedly, and the cells were re-suspended in PBS, and the fluorescence of the cells was measured by flow cytometry.
2.14. Western-blot assay for BCL-2 expression in cells
HeLa cells were inoculated respectively in a 6-well plate at a density of 3 × 105 cells per well, following being cultured for 24 h to grow well. After the addition of each sample, the cells were continued to be cultured for another 48 h. Standard western-blot experiments were then performed for each repeated well after cell lysis.
3. Results and discussion
3.1. Expression and purification of L2-NTD
The plasmid of L2-NTD was transformed and test-expressed in E. coli to confirm successful expression (Fig. 1A). The expressed protein was extracted from the supernatant after cell lysation and purified using a Ni-column via the His-tag fused at the C-terminal. SDS-PAGE analysis showed that the L2-NTD peptide was well expressed and could be eluted in large quantities at a concentration of 100 mM imidazole (no. 4–8, Fig. 1B). The absence of heterogeneous protein in the elution indicated high purity of the target peptide. After further confirmation by western blot using an anti-His-tag antibody (Fig. 1C), the peptide was concentrated using an ultrafiltration tube or diluted with buffer to the desired concentrations, and stored at −20 °C for further use.
 |
| Fig. 1 (A) Scheme to illustrate the construction and expression of L2-NTD in E coli. (B) SDS-PAGEs of L2-NTD (21 kDa) upon expression and purification through the Ni-column, which was extensively eluted at 100 mM imidazole with high purities (no. 4–8). (C) Western blots of L2-NTD under different conditions based on the antibody of His-tag. | |
3.2. Complexation of L2-NTD and siRNA
The binding of siRNA and peptides was confirmed by electrophoresis mobility shift assay (EMSA) (Fig. 2). The acidic protein BSA (pI = 4.7), used as a control, migrated towards the positive pole, while the basic peptides L2-NTD and TK-14 migrated towards the negative pole (Fig. 2A). However, after mixing each peptide with siBCL-2 (10 μM) at a molar ratio of 2
:
1, L2-NTD, TK-14, and BSA exhibited different migration patterns in the newly performed EMSA. Since the binding of RNA changes the surface charges of peptides, the migration of the complex would be opposite to that of the pristine peptide/protein. Consequently, the coomassie blue-stained protein (Fig. 2B, left) showed bands for all three proteins in positive directions, suggesting the binding of siRNA to L2-NTD or TK-14, but not to BSA due to electrostatic repulsion. Additionally, the corresponding nucleic acid images (Fig. 2B, right) showed only a band at the position of free RNA for the BSA + siBCL-2 complex, while both bands were present for L2-NTD and TK-14, indicating that binding to siBCL-2 occurred, resulting in a noticeable decrease in migration rate. Furthermore, gel electrophoresis was also performed with a 1% agarose gel under the same conditions as described above (Fig. 2C). It showed that L2-NTD interacts strongly with siRNA, resulting in a slower migration rate, whereas BSA does not bind to siRNA, and TK-14 has a relatively weaker binding to siRNA under the same conditions. Besides, nucleic acid electrophoresis experiments of siRNA before and after binding with L2-NTD were also conducted using RNAase digestion (Fig. S1, ESI†), which indicated the improved stability of siRNA after binding to the peptide, and hopefully will bring superior performance of siRNA encapsulation and delivery by HmA.
 |
| Fig. 2 Horizontal non-denaturing polyacrylamide gel (5%) results of TK-14, L2-NTD, and BSA at pH 6.5 (A) before and (B) after combination with siBCL-2 (left panel), and the corresponding RNA imaging (right panel). (C) RNA imaging of 1% agarose gel electrophoresis for TK-14, L2-NTD, and BSA after combination with siBCL-2. White box in solid line: free-RNA; white box in dotted line: peptide binding-RNA. | |
3.3. Construction of FITC-L2-NTD@HmA and its cell entry assay
Actually, at the beginning of this study, we also conducted the assembly of HmA at different pHs of 5.0, 7.0, 8.0, 9.0, and 10.0, respectively, and DLS was used to test the particle sizes of the assembly. It was found that large and stable particles of HmA can only be formed at pH 8.0 (Fig. S2, ESI†). As shown in Fig. 3A, the UV-vis absorption spectrum of FITC-labeled L2-NTD (FITC-L2-NTD) shows two bands located at 280 nm and 496 nm, corresponding to the aromatic residues such as tyrosine and/or tryptophan in the peptide, as well as FITC, respectively. Conformational changes in the peptide induced by ligand binding, pH changes, or temperature changes can cause a shift in absorbance around its original wavelength.27–29 Additionally, due to the light scattering effect of the formed nanoparticles, the baseline increases, potentially covering the weak absorption of the peptide (Fig. 3). It clearly showed that the absorption peak at 496 nm of the original FITC-L2-NTD undergoes a noticeable red-shift (Δλ = 9 nm), suggesting that the FITC-L2-NTD is possibly encapsulated inside the HmA particles. The inset in Fig. 3A shows that the solution changed from transparent to a milky orange after mixing FITC-L2-NTD, His6, and Zn2+, indicating the successful construction of FITC-L2-NTD@HmA.
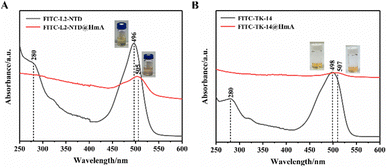 |
| Fig. 3 (A) UV-vis absorption spectra of FITC-L2-NTD@HmA and (B) FITC-TK-14@HmA. | |
Similarly, in Fig. 3B, the UV-vis absorption spectra of FITC-labeled TK-14 (FITC-TK-14) before and after co-assembly with His6 and Zn2+ show similar optical and color responses to FITC-L2-NTD, suggesting that FITC-TK-14 is also successfully encapsulated inside the HmA particles.
HmA exhibited a narrow size distribution with a mean diameter of 43.7 nm. When the small peptides were encapsulated in HmA, the hydrated particle sizes of L2-NTD@HmA and TK-14@HmA increased to 59.1 nm and 92.9 nm, respectively. Notably, the particle size of TK-14@HmA is much larger than that of L2-NTD@HmA, which might be attributed to the occurrence of stronger binding induced aggregates, as revealed by the corresponding SEM images (Fig. 4B). Additionally, the single distribution of particle sizes indicates relatively homogeneous assemblies were obtained for them. Furthermore, the SEM images confirmed their morphological characteristics and uniform size distribution (Fig. 4B), For example, more outstanding particles are shown for L2-NTD@HmA, in particular for TK-14@HmA in comparison to that of HmA, indicating successful assembly and structural integrity of peptide@HmA.
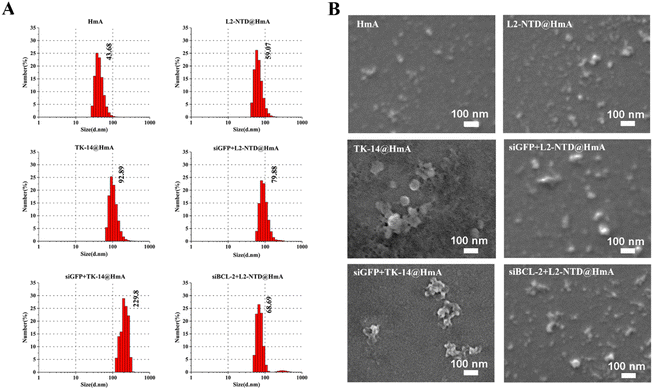 |
| Fig. 4 (A) DLS histograms of (B) HmA, L2-NTD@HmA, TK-14@HmA, siGFP+L2-NTD@HmA, siGFP+TK-14@HmA, siBCL-2+L2-NTD@HmA. (B) SEM of HmA, L2-NTD@HmA, TK-14@HmA, siGFP+L2-NTD@HmA, siGFP+TK-14@HmA, siBCL-2+L2-NTD@HmA. | |
To determine whether HmA could efficiently carry the small peptide into cells, HeLa cells were transfected with L2-NTD and FITC-L2-NTD@HmA, respectively. The corresponding fluorescence microscopy results after 2 h and 4 h of incubation are shown in Fig. 5. It was observed that the fluorescent peptide FITC-L2-NTD alone could not enter the cells regardless of the incubation time, while the HmA-coated assembly could successfully deliver FITC-L2-NTD into the cells. After 2 h of incubation, the green fluorescent FITC-L2-NTD@HmA had just entered the cells and mainly concentrated at the cell edges. However, after 4 h of incubation, the green fluorescent FITC-L2-NTD@HmA extended throughout the cell interior. Therefore, as a potential transmembrane carrier, HmA demonstrated a strong delivery ability, with 4 h being the optimal transfection time.
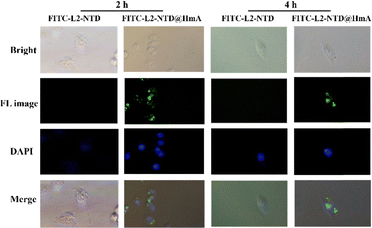 |
| Fig. 5 Fluorescence imaging of FITC-L2-NTD and FITC-L2-NTD@HmA (blue: nucleus; green: FITC) after transfection of HeLa cells for 2 h and 4 h. The concentration of the transfected particles was 30 μg mL−1. | |
Different amounts of the constructed FITC-L2-NTD@HmA were then added to the culture medium for a cell transmembrane assay. After 4 h of incubation, fluorescence images were observed for the test HeLa cells using a fluorescence microscope, proving that FITC-L2-NTD@HmA successfully entered the cells. Among the three concentrations of 15 μg mL−1, 30 μg mL−1, and 60 μg mL−1 of FITC-L2-NTD@HmA used for the assay, the fluorescence images in HeLa cells were the brightest and the cell morphology was mostly intact at a concentration of 30 μg mL−1 (Fig. 6). Therefore, 30 μg mL−1 will be used for the cell entry assay in the following experiments.
 |
| Fig. 6 Fluorescence imaging of HeLa cells after being transfected with FITC-L2-NTD@HmA constructed from different concentrations of FITC-L2-NTD@HmA for 4 h, the green is shown for FITC. | |
3.4. Cytotoxicity assay of L2-NTD@HmA
The co-assembly of the L2-NTD peptide with HmA involves the simultaneous incorporation of both components into a unified structure. In this process, the L2-NTD peptide, designed to bind to siRNA and enhance its stability, is first combined with HmA, which serves as the carrier for siRNA delivery. Therefore, the cytotoxicity of L2-NTD@HmA should be tested before using it to encapsulate siRNA. After transfecting the cells with L2-NTD@HmA at different concentration gradients, the cells were incubated overnight and the cytotoxicity of the transfected cells was detected by CCK-8 kit. As shown in Fig. 7A, L2-NTD@HmA is almost non-toxic to cells at concentrations up to 70 μg mL−1, so the subsequent vector concentration was controlled within this limit. The amount of Lipofectamine 2000 (Lip2000) required for wrapping the same concentration of siRNA (200 nM) was also tested as a control. The cytotoxicity of L2-NTD@Lip2000 was assayed for comparison. However, based on the standard protocol in the Lip2000 instructions, the operation to wrap L2-NTD failed. Therefore, only Lip2000 was used to perform the cell cytotoxicity assay using the CCK-8 kit after 4 h of treatment. The results (Fig. 7B) showed that the Lip2000 transfection reagent had a significant cytotoxic effect on cells, even at concentrations less than 6.4 μg mL−1. Such cytotoxicity of Lip2000 has been reported before, which has limited its application in in vivo drug delivery.30,31 Thus, the low toxicity of L2-NTD@HmA is evident in comparison to Lip2000.
 |
| Fig. 7 (A) Survival rate of HeLa and 293T cell after treatment with different concentrations of L2-NTD@HmA, and (B) those of HeLa and 293T cells after treatment with different Lip2000 for 4 h. | |
3.5. Construction and characterization of siRNA+L2-NTD@HmA
siGFP was first used as a model to construct an assembly with L2-NTD and HmA. The size and topology of the co-assembly measured by DLS and SEM images are shown in Fig. 4. DLS revealed that the average size of siGFP+L2-NTD@HmA is 79.9 nm (Fig. 4A), while that of siGFP+TK-14@HmA is much larger at 229.8 nm. This indicates again that the high ratio of basic residues in TK-14 (50%) results in larger nanoparticles when co-assembled with siRNA and HmA.
When using siRNA against BCL-2 (siBCL-2), the average size of siBCL-2+L2-NTD@HmA was measured to be 68.69 nm (Fig. 4A), which is close to that of siGFP+L2-NTD@HmA, suggesting that the incorporation of different siRNAs does not significantly affect the particle size of the constructed nanoparticles. These results imply the predominance of HmA itself in nanoparticle construction. However, after encapsulating the siBCL-2+TK-14 complex, the average size of siBCL-2+TK-14@HmA was evaluated to be 229.8 nm (Fig. 4A), confirming the possible aggregation of nanoparticles in the presence of TK-14, although the reason remains unclear.
As the size of nanoparticles formed by encapsulated siRNA–L2-NTD ranged between 40 nm and 80 nm, it indicated that siRNA–L2-NTD@HmA particles could effectively avoid blood clearance.32 Additionally, compared with TK-14, the particle size of siRNA+L2-NTD@HmA was more homogeneous, as revealed by the corresponding SEM images (Fig. 4B). Of note, as shown in Fig. 4B, in the presence of siRNA, both the shape and size of siRNA+peptide@HmA are obviously different from those of peptide@HmA, suggesting the incorporation of siRNA inside. To confirm it, the elemental mapping by SEM-EDS both for siGFP+L2-NTD@HmA (Fig. S3, ESI†) and siBCL-2+L2-NTD@HmA (Fig. S4, ESI†) are performed, where the element of P, being essentially originated from RNA are also presented, besides those of Zn, C, N and O mainly for peptide and HmA. Moreover, the elemental mapping by SEM-EDS (Fig. S3 and S4, ESI†) indicated that the elements of Zn, C, N, O, P and are all homogeneously located inside the particles of assemblies. Such results confirm the in situ encapsulation of siRNA inside HmA, which not only changes the size but also the morphologies of the assemblies (Fig. 4B). In particular, the particles were quite stable after being stored for one week at 4 °C, which was validated by DLS assessment.
Due to the high instability of siRNA, to verify whether the zinc ion coordination process affects siRNA, the siBCL-2+L2-NTD@HmA was put in the acidic environment with different pH values to disassemble HmA and release the encapsulated siRNA, and then nucleic acid electrophoresis was performed to verify the integrity of siRNA. The result in Fig. S5 (ESI†) indicates that siBCL-2 was detectable after being placed in acidic buffer with different pHs for 6 h. In particular, at pH 4.5, the siBCL-2 band appears brighter, indicating that HmA almost completely dissociates at pH 4.5. It not only demonstrated that Zn2+ coordination does not affect the structure of siRNA, but also provided evidence for the release of siRNA from HmA in endosome escape (pH 4.5).
3.6. Transmembrane assays of siRNA+L2-NTD@HmA co-assembly
To investigate if the encapsulation of siRNA+L2-NTD in HmA assembly can promote the transmembrane delivery of nanoparticles to cells, we analyzed the intracellular distribution of Cy5-labeled siGFP (Cy5-siGFP) after co-assembly with L2-NTD and HmA in living cells using both confocal fluorescence imaging and flow cytometry (Fig. 8). HeLa cells were first imaged directly after incubation with Cy5-siGFP+L2-NTD@HmA or Cy5-siGFP+TK-14@HmA. After 4 h of incubation, red fluorescence was observed in the cytoplasm of HeLa cells under a microscope (Fig. 8A), proving that Cy5-siGFP was successfully brought into the cells upon binding with the peptide and encapsulation in HmA. Notably, the fluorescence images for Cy5-siGFP+L2-NTD@HmA were significantly brighter than those for Cy5-siGFP+TK-14@HmA, indicating the superiority of L2-NTD over TK-14 in complexing with siRNA and further encapsulation in HmA.
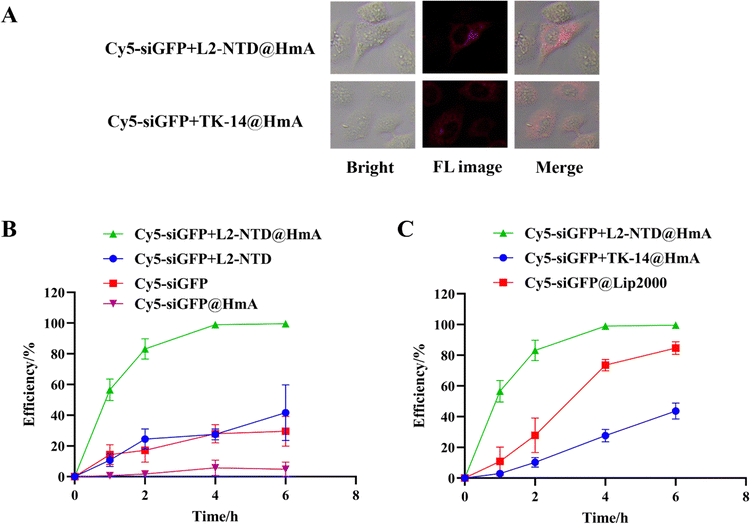 |
| Fig. 8 (A) Fluorescence imaging of HeLa cells 4 h after transfection with 30 μg mL−1 Cy5-siGFP+L2-NTD@HmA and Cy5-siGFP+TK-14@HmA, respectively (red is for Cy5). (B) and (C) Outline of flow-cytometry results for HeLa cells after being treated with the constructed materials at different time gradients. | |
Flow cytometry correlates the processed cell population by scanning individual cells for properties such as fluorescence and sample characteristics, offering the advantage of rapid detection and high sensitivity.27,33 The flow cytometry results showed low detectable signals for cells treated with Cy5-siGFP or Cy5-siGFP+L2-NTD, compared to those treated with Cy5-siGFP+L2-NTD@HmA (Fig. 8B), illustrating that without HmA encapsulation, siRNA or Cy5-siGFP+L2-NTD does not enter the cells effectively. Additionally, the minimal difference between Cy5-siGFP and Cy5-siGFP+L2-NTD suggests that L2-NTD conjugation alone does not enhance or inhibit the delivery efficiency of single siGFP into cells. The strong signal for Cy5-siGFP+L2-NTD@HmA indicates that HmA encapsulation significantly improved the transfection efficiency of the co-assembly. Interestingly, the inferior performance of siRNA@HmA compared to Cy5-siGFP alone could be attributed to siRNA degradation during HmA encapsulation with sonification. This confirms that without the protection of L2-NTD, siRNA cannot be effectively encapsulated in HmA under sonification conditions.
Moreover, as shown in Fig. 8C, when using Lipofectamine 2000 (Lip2000) as a positive control to carry Cy5-siGFP into HeLa cells, detectable signals were observed for the treated cells, although the efficiency was not as high as that of Cy5-siGFP+L2-NTD@HmA. This difference demonstrates the superiority of HmA over the commercially available carrier Lip2000 for gene delivery. Finally, the transmembrane assay was also performed on Cy5-siGFP+TK-14@HmA for comparison (Fig. 8C). Much lower transmembrane efficiency was obtained for Cy5-siGFP+TK-14@HmA compared to Cy5-siGFP+L2-NTD@HmA (Fig. 8C), demonstrating again the superiority of the specifically designed L2-NTD over TK-14 under identical conditions. Therefore, the above results reveal two key facts: first, L2-NTD is necessary for protecting and encapsulating siRNA using HmA, as complexing with L2-NTD protects siRNA from degradation during HmA construction; second, the encapsulation of the siRNA–L2-NTD complex by HmA significantly improves its transmembrane efficiency in cells.
3.7. Endosomal escape assay of L2-NTD@HmA
The endosomal escape capability of L2-NTD@HmA was investigated by tracking the intracellular distribution of co-incubated calcein.34 Calcein is a membrane-impermeable fluorescent dye that is passively macropinocytosed by the cell and trafficked into endosomes and lysosomes. It self-quenches at high concentrations and at low pH (such as in endosomes/lysosomes), resulting in low-intensity, punctate fluorescence when trapped in endocytic vesicles. However, once the membrane is compromised, calcein escapes from endosomes/lysosomes and diffuses into the cytosol, where the fluorescence increases.35 To determine whether the cytosolic entry of L2-NTD@HmA and TK-14@HmA was accompanied by enhanced endosomal leakage, we co-incubated each with calcein. Additionally, cells incubated with L2-NTD, TK-14, and Lip2000 served as positive controls, while cells incubated with calcein alone served as negative controls. After 4 h of co-incubation with these materials in HeLa (Fig. 9A) and 293T cells (Fig. 9B), the distribution of calcein in the cytoplasm was observed for each material.
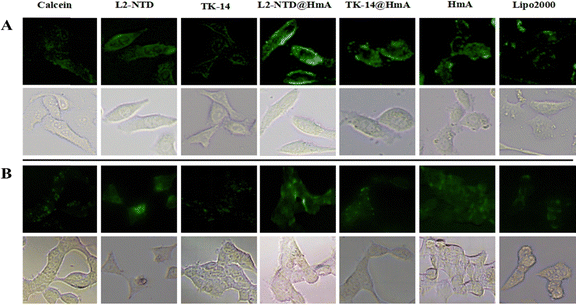 |
| Fig. 9 The cellular distribution of the cell-impermeable fluorescent dye calcein in (A) HeLa and (B) 293T cells upon co-incubation for 4 h with (from left to right) calcein (0.1 mg mL−1), L2-NTD (30 μg mL−1), TK-14 (30 μg mL−1), L2-NTD@HmA (30 μg mL−1), TK-14@HmA (30 μg mL−1), HmA (30 μg mL−1), and Lip2000 (2.5 μg mL−1). | |
First, the incubation of calcein and its co-incubation with TK-14 and L2-NTD resulted in improved green puncta for the latter, confirming that L2-NTD and TK-14 slightly promoted endosome-to-cytosol release by inducing endosomal leakage. In particular, the green fluorescence induced by L2-NTD was noticeably brighter than that of TK-14, indicating its superior ability in promoting endosomal release. The comparison of HmA and Lip2000 also illustrated a brighter green fluorescence for HmA over Lip2000, demonstrating HmA's advantage over Lip2000 for endosomal release. Finally, L2-NTD@HmA showed the strongest fluorescence in the cytoplasm, both in HeLa (Fig. 9A) and 293T cells (Fig. 9B), which can be attributed to the synergy of L2-NTD and HmA for both transmembrane delivery and endosomal release.20,21,34
The nitrogen atom on the imidazole ring with two unpaired electrons can accept hydrogens and buffer acidic endosomal environments between pH 5 and 7. The buffering of acidic endosomes by histidine-containing carriers is an important factor for enhancing nucleic acid delivery. It has been suggested that histidine-containing carriers neutralize the pH, resulting in the active transport of protons with an influx of chloride ions to preserve neutrality in endosomes. This influx leads to the passive transport of water into endosomes, causing osmotic swelling and lysis,36 enabling the escape of nucleic acids into the cytosol. An alternative mechanism for endosomal lysis is the interaction of the positively charged carriers with the endosomal membrane.37 Upon acidification of the endosomes, the histidine-containing carriers become protonated. This protonation enables charge–charge repulsion of the polymers, resulting in the breakup of the complex.38
3.8. Gene silencing of BCL-2 by siBCL-2+L2-NTD@HmA
BCL-2 is associated with the apoptosis of tumor cells and is highly expressed in many tumor cells but less so in normal cells.39 Therefore, both HeLa and A549 cells were employed to assay the expression level of BCL-2 in the presence of siBCL-2. Living cells were seeded in a 6-well plate at 3 × 105 cells per well and cultured for 24 h to allow for growth. After this period, the constructed nanomaterials of siBCL-2+L2-NTD@HmA, siBCL-2+TK-14@HmA, and siBCL-2@Lip2000 were transfected into each cell line. The cells were then cultured for another 48 h post-transfection. Finally, cellular proteins were extracted from cell lysates and analyzed using the standard western-blot assay (Fig. 10A).
 |
| Fig. 10 Transfection of HeLa cells using naked siBCL-2 or that being encapsulated in different carriers: (A) western-blots of BCL-2 expressed in the cells 48 h post-transfection and (B) histograms of semi-quantitative analysis of the grey value ratio of BCL-2 to β-tubulin using Image J, and the comparison of BCL-2 protein expression levels in the test group relative to the control. | |
Naked siBCL-2 served as a negative control, with results also shown in Fig. 10A for comparison. In parallel, β-tubulin was used as an intracellular reference. Lighter BCL-2 bands relative to the β-tubulin band indicate lower expression of BCL-2 in the same cells. The grey values of individual bands for BCL-2 and β-tubulin were calculated using ImageJ software, and the ratio of BCL-2 to β-tubulin was used to evaluate the expression level of BCL-2 in each experimental group, with the control set as 100% to create a histogram of protein content. As shown in Fig. 10B, siBCL-2+L2-NTD@HmA and siBCL-2@Lip2000 exhibited a significant silencing effect on the expression of BCL-2 protein in HeLa cells. The siBCL-2+TK-14@HmA group also showed a reduction in BCL-2 protein expression, but not as strongly as the other two groups. Notably, the significant gap between siBCL-2+L2-NTD@HmA and siBCL-2+TK-14@HmA revealed the unique effectiveness of L2-NTD and its substantial contribution to transmembrane transport, endosomal escape, and ultimately, gene silencing of BCL-2 in HeLa cells.
4. Conclusions
This study demonstrates a multi-functional assembly for siRNA delivery constructed from the His6-metal assembly (HmA) and an RNA-binding peptide L2-NTD. The conjunction of siRNA with L2-NTD not only enabled its encapsulation within HmA for efficient delivery but also improved the transmembrane transport and endosomal release of the co-assembly. The protein expression assay of siBCL-2 showed that the combined use of HmA and L2-NTD resulted in significant inhibition of BCL-2 expression, surpassing the performance of siRNA + TK-14@HmA and siRNA@Lip2000. This enhanced efficacy is attributed to the synergy between HmA and L2-NTD in siRNA delivery and endosomal escape. The introduction of the functional peptide L2-NTD greatly contributed to the delivery and endosomal escape of siRNA. This concept can be extended to the design of other siRNA drugs or vaccines for various purposes. Furthermore, this vector is not limited to siRNA; it also has the potential to harbor and deliver other types of RNA, providing a new approach for developing safer and more efficient nucleic acid therapeutic drugs in the future.
Author contributions
Yan Zhang: designed the methodology and conducted most of the experiments, wrote the original draft; Li-Miao Qin: designed and experimentally validated some of the experiments, data curation; Meng-fan Feng: provided experimental methods and technical support; Xianghui Yu: contributed resources and provided supervision; Yuqing Wu: acquired funding, provided supervision, and contributed to writing – review & editing. All authors discussed, commented, and have given approval to the final version of the manuscript.
Data availability
The data supporting this article have been included as part of the ESI.†
Conflicts of interest
There are no conflicts between authors to declare.
Acknowledgements
This work was supported by the Jilin Province Science and Technology Development Plan Project (No. 20230204040YY), the financial support of NSFC (No. 21875085), and the Innovation & Opening Program of the State Key Laboratory of Supramolecular Structure and Materials, Jilin University.
Notes and references
- J. Zhang, B. Chen, C. Gan, H. Sun, J. Zhang and L. Feng, Int. J. Nanomed., 2023, 18, 7605–7635 CrossRef CAS.
- A. Rajeev, A. Siby, M. J. Koottungal, J. George and F. John, ChemistrySelect, 2021, 6, 13350–13362 CrossRef CAS.
- A. Paul, A. Muralidharan, A. Biswas, B. V. Kamath, A. Joseph and A. T. Alex, OpenNano, 2022, 7, 100063 CrossRef.
- C. Yang, Z. Lin, X. Zhang, Z. Xu, G. Xu, Y. Wang, T. Tsai, P. Cheng, W. Law, K. Yong and C. Chen, Macromol. Biosci., 2024, 24, 2300362 CrossRef CAS.
- K. Lee, D. Min, Y. Choi, J. Kim, S. Yoon, J. Jang, S. Park, M. Tanaka, Y. W. Cho, H. Koo, H. Jeon and J. Choi, Biotechnol. J., 2020, 15, 2000079 CrossRef CAS PubMed.
- K. Ji, Y. Xiao and W. Zhang, J. Pept. Sci., 2020, 26, e3230 CrossRef CAS PubMed.
- G. Kandasamy and D. Maity, Biomed. Mater., 2024, 19, 022001 CrossRef.
- R. Tang, Q. Bai, S. Ma and J. Ou, TrAC, Trends Anal. Chem., 2023, 158, 116862 CrossRef CAS.
- S. Li, Q. Zou, Y. Li, C. Yuan, R. Xing and X. Yan, J. Am. Chem. Soc., 2018, 140, 10794–10802 CrossRef CAS.
- W. Huang, P. Hao, J. Qin, S. Luo, T. Zhang, B. Peng, H. Chen and X. Zan, Acta Biomater., 2019, 90, 441–452 CrossRef CAS.
- D. A. Brian and R. S. Baric, Curr. Top. Microbiol. Immunol., 2005, 287, 1–30 CAS.
- C. Chang, M.-H. Hou, C.-F. Chang, C.-D. Hsiao and T. Huang, Antiviral Res., 2014, 103, 39–50 CrossRef CAS PubMed.
- A. Wu, Y. Peng, B. Huang, X. Ding, X. Wang, P. Niu, J. Meng, Z. Zhu, Z. Zhang, J. Wang, J. Sheng, L. Quan, Z. Xia, W. Tan, G. Cheng and T. Jiang, Cell Host Microbe, 2020, 27, 325–328 CrossRef CAS.
- E. J. Snijder, E. Decroly and J. Ziebuhr, Adv. Virus Res., 2016, 96, 59–126 CAS.
- P. Sarma, N. Shekhar, M. Prajapat, P. Avti, H. Kaur, S. Kumar, S. Singh, H. Kumar, A. Prakash, D. P. Dhibar and B. Medhi, J. Biomol. Struct. Dyn., 2021, 39, 2724–2732 CrossRef CAS.
- P. Dhankhar, V. Dalal, V. Singh, S. Tomar and P. Kumar, J. Biomol. Struct. Dyn., 2020, 40, 4084–4099 CrossRef.
- S. Bednarek, V. Madan, P. J. Sikorski, R. Bartenschlager, J. Kowalska and J. Jemielity, Philos. Trans. R. Soc., B, 2018, 373, 20180167 CrossRef PubMed.
- C. Ciani, A. Pérez-Ràfols, I. Bonomo, M. Micaelli, A. Esposito, C. Zucal, R. Belli, V. G. D'Agostino, I. Bianconi, V. Calderone, L. Cerofolini, O. Massidda, M. B. Whalen, M. Fragai and A. Provenzani, Biomolecules, 2022, 12, 922 CrossRef CAS PubMed.
- R. Yadav, L. Zhai and E. Tumban, Viruses, 2019, 12, 18 CrossRef PubMed.
- S. Tamburini, Y. Zhang, A. Gagliardi, G. D. Lascio, E. Caproni, M. Benedet, M. Tomasi, R. Corbellari, I. Zanella, L. Croia, G. Grandi, M. Müller and A. Grandi, Vaccines, 2023, 11, 1582 CrossRef CAS.
- H. Yan, S. S. Foo, W. Chen, J.-S. Yoo, W.-J. Shin, C. Wu and J. U. Jung, mBio, 2019, 10, e01834-19 CrossRef PubMed.
- W. Huang, S. Zhou, B. Tang, H. Xu, X. Wu, N. Li, X. Zan and W. Geng, Acta Biomater., 2021, 129, 199–208 CrossRef CAS.
- H. Li, X. Yang, F. Gao, C. Qian, C. Li, D. Oupicky and M. Sun, J. Controlled Release, 2018, 292, 78–90 CrossRef CAS.
- W. Liu, C. Ma, J. Cao, H. Zhou and T. Guo, J. Controlled Release, 2024, 367, 316–326 CrossRef CAS.
- W. Perea and N. L. Greenbaum, Anal. Biochem., 2020, 599, 113736 CrossRef CAS PubMed.
- P. Shi, S. Luo, B. Voit, D. Appelhans and X. Zan, J. Mater. Chem. B, 2018, 6, 4205–4215 RSC.
- A. Lehmann, J. H. F. Wixted, M. V. Shapovalov, H. Roder, R. L. Dunbrack and M. K. Robinson, mAbs, 2015, 7, 1058–1071 CrossRef CAS.
- C. Berndt, J. D. Schwenn and C. H. Lillig, Chem. Sci., 2015, 6, 7049–7058 RSC.
- M. Esteki, E. Dashtaki, Y. V. Heyden and J. Simal-Gandara, Antibiotics, 2020, 9, 383 CrossRef CAS PubMed.
- Z. Huang, J. Liu, C. Zhang and X. Yang, Heliyon, 2022, 8, e12118 CrossRef CAS PubMed.
- Z. Li, C. Zhang, Z. Wang, J. Shen, P. Xiang, X. Chen, J. Nan and Y. Lin, J. Cell. Physiol., 2019, 234, 21166–21181 CrossRef CAS.
- C. Guo, H. Yuan, Y. Yu, Z. Gao, Y. Zhang, T. Yin, H. He, J. Gou and X. Tang, J. Controlled Release, 2023, 360, 734–746 CrossRef CAS PubMed.
- K. C. Sanjaya, A. Ranzoni, J. Hung, M. A. T. Blaskovich, D. Watterson, P. R. Young and M. A. Cooper, Anal. Chim. Acta, 2020, 1107, 85–91 CrossRef CAS PubMed.
- Y. Hu, T. Litwin, A. R. Nagaraja, B. Kwong, J. Katz, N. Watson and D. J. Irvine, Nano Lett., 2007, 7, 3056–3064 CrossRef CAS PubMed.
- S. A. Smith, L. I. Selby, A. P. R. Johnston and G. K. Such, Bioconjugate Chem., 2019, 30, 263–272 CrossRef CAS PubMed.
- J. P. Behr, B. Demeneix, J. P. Loeffler and J. Perez-Mutul, Proc. Natl. Acad. Sci. U. S. A., 1989, 86, 6982–6986 CrossRef CAS.
- Q.-R. Chen, Nucleic Acids Res., 2002, 30, 1338–1345 CrossRef CAS.
- D. Putnam, A. N. Zelikin, V. A. Izumrudov and R. Langer, Biomaterials, 2003, 24, 4425–4433 CrossRef CAS PubMed.
- Iksen, W. Witayateeraporn, B. Hardianti and V. Pongrakhananon, Phytother. Res., 2024, 38, 2249–2275 CrossRef CAS.
Footnotes |
† Electronic supplementary information (ESI) available. See DOI: https://doi.org/10.1039/d4tb01433b |
‡ The first two authors contributed equally to the present study. |
|
This journal is © The Royal Society of Chemistry 2024 |
Click here to see how this site uses Cookies. View our privacy policy here.