DOI:
10.1039/D4TB01617C
(Paper)
J. Mater. Chem. B, 2024,
12, 11134-11141
Structural isomerism engineering regulates molecular AIE behavior and application in visualizing endogenous hydrogen sulfide†
Received
24th July 2024
, Accepted 18th September 2024
First published on 20th September 2024
Abstract
Hydrogen sulfide (H2S) is a critical bioregulator implicated in numerous physiological and pathological processes, including cancer and neurodegenerative diseases. Compared with traditional instrument analysis, fluorescence detection technology based on small molecules in real-time and in situ sensing H2S has attracted attention. In this investigation, we developed a system of coumarin-based fluorophores linked with aminopyridine via a dipolar imino-double bond. Their aggregation-induced emission (AIE) behaviors were further regulated via structural isomerism engineering. Owing to restricting intramolecular motions and high molecular dipole moment, 2-amino-pyridyl-substituted coumarin (CMR-o-Py) forms stable AIE nanoaggregates with brighter fluorescence than the others. The CMR-o-Py nanoaggregates serve as probes for sensing H2S with a detection limit of 18.1 μM in a hydrophilic environment via Michael addition between imino-bond and sulfide ions. The 1
:
1 stoichiometric binding energy constant between the probe and H2S is 5.68 × 108 M−1, and its half-time of the first-order binding reaction was estimated to be 4.85 min. Moreover, CMR-o-Py, with excellent biocompatibility, holds promise as an ideal sensor for endogenous H2S in living cells and onion tissues, further highlighting its potential application in biological fields.
Introduction
Hydrogen sulfide (H2S) has been well known as a hazardous gas with a dangerous air concentration of 100 ppm.1,2 Despite that, in biological systems, it plays a critical role in regulating various pathological processes3 and cancer proliferation, even involving some diseases including Alzheimer's disease, Parkinson's disease, and hypertension.4,5 In healthy animals and humans, the range of circulating physiological concentrations for H2S is from 10 to 100 μM.1 In view of this, H2S detection is essential for environmental safety and biomedicine. Looking back at traditional analytical methods such as H2S ion-selective electrodes and gas chromatography methods, the long equilibration time or equipment airtightness has limited their measurements even if they have a low limit of detection (LOD) in the nM range.6 Moreover, as H2S has high volatility and catabolic nature, it is challenging to sense in real time and in situ in biological systems using instruments. Hence, fluorescence technologies with the advantages of high sensitivity, selectivity, response capability, biocompatibility, low cost, and non-invasiveness have been noticed and developed,7 in which fluorescence probes, in particular small-molecule probes,6,8–10 play a crucial role in sensitive H2S detection in vitro and in vivo, accelerating the exploration of H2S-relative biology.
Generally, fluorescent probes based on small molecules for sensing H2S can be simply divided into two types. First, fluorophore scaffolds link H2S-responding groups such as 2,4-dinitrophenyl,11 2,4-dinitrobenzenesulfonyl,12 and 7-nitrobenzo-2-oxa-1,3-diazole,13,14 displaying a tiny or dim emission as a result of fluorescence resonance energy transfer (FRET) or photo-induced electron transfer effects. Upon targeting H2S species, the above-mentioned nitro-groups are cleaved from fluorophore frameworks via a nucleophilic aromatic substitution mechanism, followed by effective intramolecular charge transfer (ICT) property and obvious fluorescence. The fluorescent “off-to-on” or “turn-on” signals can avoid some interference from the background or light source and improve detection authenticity and reliability.15 Second, the free-double-bond site of fluorophore serves as an electrophilic center for Michael addition of nucleophilic sulfides (HS−/S2−),16 inducing the change in fluorescence. In general, the blue-shifting response emission comes from the decreasing conjugation length of fluorogen after the nucleophilic reaction with H2S. Compared to the signal before detection, the ratiometric sensing signals toward H2S reduce signal misjudgement and enhance detection dependability in some cases.17,18 This reveals that probes with turn-on or ratiometric fluorescence change upon sensing H2S effectively promote analytical performance.
In addition, to improve the probe's stability in a hydrophilic detection environment or solid test strips, fluorophores with aggregation-induced emission (AIE) characteristics,19–21 named AIEgen, have been introduced into H2S-targeting probes. As probes sense H2S, the forming AIE nanoaggregates will exhibit bright emissions due to the restriction of the intramolecular motions (RIM) mechanism.22–24 The nanoaggregates maintain the advantage of being resistant to photobleaching and background interference, advancing detection accuracy. Taking this into account, we introduced the dipolar imine double bond as an electrophilic position for H2S into the coumarin fluorophore (Scheme 1), constructing H2S-responding probes with AIE behavior (CMR-o-Py, CMR-m-Py, CMR-p-Py, and the standard CMR-Ph; Scheme S1, ESI†). For a molecular framework, the aminopyridine isomers serve as the electron-withdrawing rotors to polarize imine as well as release excited-state energy through configurational reorganization for their unimolecular state. Meanwhile, the forming nanoaggregates with declining reorganization energy in hydrophilic conditions express bright emissions due to the RIM effect. Among them, nanoaggregates based on CMR-o-Py having a stable AIE signal over 30 min sense H2S with an LOD value of 18.1 μM and a detectable pH range from 4.0 to 8.0. This suggests that they are ideal for acting as endogenous or exogenous H2S-triggered sensors in the biological environment and onion tissue. Imaging experiments within living cells confirm that CMR-o-Py, exhibiting favorable biocompatibility, can detect variations in both exogenous and endogenous H2S levels. Furthermore, CMR-o-Py also successfully visualizes the production of H2S-donor from onion tissues. We anticipate that this study will significantly contribute to developing innovative approaches for early detecting and diagnosing H2S in the biological environment.
 |
| Scheme 1 Design strategy for coumarin-based AIEgen through isomerization engineering. | |
Results and discussion
Characterization of coumarin-based materials
Coumarin-based materials were synthesized by a condensation reaction, forming a π-conjugated imine bond between 7-hydroxy-4-methyl-2-oxo-2H-chromene-8-carbaldehyde and hydrazine-pyridine isomers (Scheme S2, ESI†), to construct CMR-o-Py, CMR-m-Py, and CMR-p-Py, respectively, where the phenyl-substituted coumarin (CMR-Ph) serves as the standard material.25 Their identification data using NMR and HRMS are listed in the ESI.†
Photophysical properties of coumarin-based materials
The UV-vis absorption and PL spectra of coumarin-based materials were measured in a DMSO solution at a concentration of 1 μM (Fig. 1A and B). Compared to CMR-Ph, the pyridyl-substituted materials show blue-shifting absorption, attributed to the fact that the electron-withdrawing pyridyl ring stabilized the highest occupied molecular orbital (HOMO) and the lowest unoccupied molecular orbital (LUMO) (vide infra). Their bathochromic absorption abilities are in the order of CMR-Ph > CMR-m-Py > CMR-o-Py ≈ CMR-p-Py (Fig. 1A). Furthermore, they emit lowly because of intramolecular motions releasing excited-state energy, in which DMSO solvent's emission at 460 nm (Fig. S1, ESI†) can even be observed in the PL spectra (Fig. 1B). To gain insights into this phenomenon, the measured concentration was increased to ten times (10 μM). The result implies that DMSO's PL intensity disappears, but CMR-o-Py visibly grows 9-fold (Fig. S2, ESI†). Based on the DLS analysis of Fig. S3 (ESI†), CMR-o-Py forms nanoparticles with a distribution of 9.0 nm in DMSO at a concentration of 10 μM due to its low solubility. By contrast, the DLS signal is below 0.4 nm for 1 μM of concentration. This indicates that the apparent emission of CMR-o-Py in DMSO is attributed to its nanoaggregates with the AIE effect.
 |
| Fig. 1 Photophysical properties of coumarin-based molecules. (A) Normalized UV-vis absorption and (B) PL spectra of coumarin-based materials (1 μM in DMSO), (C) PL spectra of CMR-o-Py and (D) AIE curves of coumarin-based materials in different mixture solutions of DMSO/PBS (100 μM; pH = 7.4), and (E) DLS analysis and (F) the relative PL intensity of CMR-o-Py nanoaggregates during 30 min (I0: the starting intensity). Excitation wavelength: 405 nm; excitation slit: 1 nm; emission slit: 5 nm. | |
To explore the AIE behaviors of coumarin-based fluorogens, their UV-vis absorption and PL intensities in DMSO/PBS mixtures and DMSO were recorded (Fig. 1C and Fig. S4–S7, ESI†), and their PL intensities of maximum emitting wavelength versus PBS volume factions (vol%) were then plotted, as shown in Fig. 1D. The CMR-o-Py presents better AIE behavior in a higher PBS content (60 vol%) than that of the others, 20 vol% for CMR-m-Py, 10 vol% for CMR-p-Py, and 30 vol% for CMR-Ph, respectively. This is due to their poor solubility, causing prone accumulation and even sedimentation compared to CMR-o-Py. Meanwhile, the increasing particle sizes leading to light scattering will weaken the fluorescence signal. Even so, compared to DMR-Ph, the nanoaggregates based on pyridyl-coumarin isomers have higher emission intensities, especially CMR-o-Py. This may be attributed to its high dipole moment (5.92 D) (Fig. S7, ESI†). The favorable solubility avoids the product of large particle sizes.26 To further analyze with DLS, the particle distributions of CMR-o-Py nanoaggregates are 33.9 and 36.7 nm after 1 and 10 min (Fig. S8, ESI†), respectively, and then only grow to a diameter of ∼48 nm after 30 min (Fig. 1E), indicating good stability of particle size. Thus, the relative PL intensities are almost unchanged during 30 min (Fig. 1F). This reveals that their long stability in the 90-vol% PBS solution is favorable for detection application.
Theoretical calculations of coumarin-based molecules
The time-dependent density functional theory (TD-DFT) is a well-known and proven method for analyzing electron cloud distributions and energy levels of molecules in the ground (S0) and excited (S1) states, which relate to molecular photophysical properties and applicability. Molecular simulations were thus performed for coumarin-based AIEgens with TD-DFT using the B3LYP functional at a 6-31G(d) basis set, as shown in Fig. 2 and Fig. S9–S13, Tables S1–S3 (ESI†). The electron densities of HOMO are distributed from pyridyl and phenyl-rings to imino-coumarin, whereas those of LUMO are mainly delocalized on imino-coumarin and its carboxyl group. The energy levels of HOMO and LUMO are down-shifted in the order of CMR-Ph, CMR-o-Py, CMR-m-Py, and CMR-p-Py, respectively. This is attributed to the electron-withdrawing abilities of pyridine isomers, which are higher than that of CMR-Ph, corresponding to the experimental results of UV-vis absorption (Fig. 1A). For the pyridyl isomer-based molecules, CMR-o-Py with lower excited and ground reorganization energies (
and Er) suggests that its changes in molecular configuration between the S0 and S1 states27 are smaller than those of the others (CMR-m-Py and CMR-p-Py). This means the latter molecules in the excited state mostly release energy via a nonradiative pathway,28 compared to the former. This may be the reason for the high fluorescence of CMR-o-Py in DMSO (Fig. 1B). Additionally, the order of the change in dipole moments between S0 and S1 states is CMR-o-Py (5.92 D) > CMR-p-Py (2.48 D) > CMR-m-Py (2.45 D), where CMR-Ph (0 D) is the standard (Fig. S9, ESI†). The higher dipole moment for CMR-o-Py is helpful for dissolution and/or nanoaggregate formation in polar solvents.26 Furthermore, it avoids aggregation with large-sized particles, leading to instability and decline of the fluorescence signal. Consistent with the above-mentioned photophysical analysis, CMR-o-Py and its nanoaggregates served as the fluorescent probe for detection, which is feasible to a certain extent as expected.
 |
| Fig. 2 Theoretical calculations of coumarin-based molecules. (A) The electron cloud distributions of HOMO and LUMO and their energy levels. (B) Schematic diagram of energy levels and their relative energy. Eab, Em, Er, , and Ead denote the energy difference of absorption from S0 to S1 states, that of emission from S1 to S0 states, that of relaxation in the S0 state, that of relaxation in the S1 state, and that between the minimum S1 and S0 states, respectively. kr is the radiative decay rate and estimated using the formula, kr = f × (Em)2/1.5, where f is the emission's oscillation factor. | |
Detection of H2S with CMR-o-Py nanoaggregates
Based on the stability of CMR-o-Py nanoaggregates in a DMSO/PBS (v/v, 1/9; pH = 7.4; 100 μM) buffer solution for 30 min (Fig. 1F), thirty substances with ten equivalents (eq.) versus probe were selected for fluorescence detection upon excitation at a wavelength of 405 nm (Fig. 3A, B and Fig. S14, ESI†). In relation to the nanoaggregates' signal, the absorption at 364 nm (Fig. S15, ESI†) and fluorescence at 450 nm (Fig. 3C) were remarkably enhanced about 1.76 and 2.61 times, respectively, upon the addition of H2S. By contrast, the nanoaggregates formed in a 60 vol% PBS solution only show a 1.54 times enhanced PL signal (Fig. S16, ESI†), although their AIE behavior is excellent. Moreover, the typical sulfide-based analytes (HS− and Cys) only slightly augment the emission intensity, whereas glutathione (GSH) weakens it. Under the same conditions, adding other anions and various biologically related analytes does not influence probe sensing H2S even if some anions (HSO4−, HSO3−, SO42−, and H2PO4−) also decline the probe's fluorescence. In comparison, most metal ions do not affect the fluorescence of nanoaggregates, except Ni+ ions with a slight signal decrease (Fig. S16D, ESI†). In different buffer solutions, the nanoaggregates have obvious response signals in the pH range from 6 to 8 (Fig. 3D and Fig. S17, ESI†), which makes it suitable for application in the biological environment.
 |
| Fig. 3 Fluorescence detection of CMR-o-Py nanoaggregates in buffer solutions. (A) and (B) Relative PL intensities (I/I0 − 1) of nanoaggregates (100 μM in 90 vol% DMSO/PBS, pH = 7.4) and their additional different substances (1 mM; 0. none, 1. F−, 2. Cl−, 3. Br−, 4. I−, 5. SCN−, 6. CH3CO2−, 7. NO2−, 8. HSO4−, 9. HSO3−, 10. S2O42−, 11. HS−, 12. H2S, 13. H2Sn, 14. SO42−, 15. S2O32−, 16. H2PO4−, 17. ClO4−, 18. β-Ala, 19. Gly, 20. L-His, 21. L-Pro, 22. DL-Met, 23. L-Ser, 24. L-Leu, 25. L-Val, 26. L-Thr, 27. GSH, 28. Cys, 29. Na+, 30. K+), (C) PL spectra of nanoaggregates (100 μM) with and without the different sulfide substances, (D) relative PL intensities (I/I0) of nanoaggregates with H2S in different pH buffer solutions, (E) PL spectra of nanoaggregates (100 μM) with different concentrations of H2S (0–1000 μM), and (F) plot of PL intensities of (E) versus H2S concentrations (0–8 eq. relative for nanoaggregates). The inset of (F) is the linear relationship (y = 54 712x + 735 732, R2 = 0.998) between the PL intensities and H2S concentrations (0–0.8 eq.). | |
Upon further incubation of the nanoaggregates (100 μM) with H2S different concentrations (10–1000 μM; 0.1–10 eq.) for 15 min, the changes in PL intensities were recorded to determine the LOD value. Upon excitation at 405 nm, the fluorescence signal at 450 nm increases along with the increase in H2S concentration (Fig. 3E). It reaches a plateau after adding H2S over a concentration of 500 μM (5 eq.), and the linear range is 0–80 μM (0–0.8 eq. versus probe) (Fig. 3F). The LOD was calculated to be 0.181 eq. (18.1 μM for 100 μM of nanoaggregates) according to the formula, LOD = 3σ/k,20 where 3 is from the ASTM standard, σ is the standard deviation of 10 blank experiments (3306.7), and k is the sensitivity estimated from the slope value of the linearity plot (the inset of Fig. 3F). Meanwhile, with the gradually decreasing H2S concentration, the limit of quantity (LOQ) was determined to be 60.4 μM (∼2.05 ppm), where the standard deviation times used is 10. The LOD and LOQ values for H2S are between the monitoring standard (10–100 μM) for physiological circulating concentrations and below the dangerous gas concentration (100 ppm) in the air.1 As shown in Fig. S18 (ESI†), in an environment where H2S exists, the nanoaggregate-adsorbed filter paper emits an enhanced blue fluorescence signal with increasing time.29 For point-of-care testing, the filter papers adsorbed with CMR-o-Py nanoaggregates were respectively dropped into H2S of different concentrations. Under 365 nm irradiation, gradually growing and blue-shifted fluorescence can be observed with the increase in H2S concentrations (Fig. S19, ESI†).
Response mechanism of H2S
To explore the mechanism of CMR-o-Py nanoaggregates as probes toward H2S sensing, the plot of the relative PL intensity with different H2S concentrations (Fig. 3F) was reorganized according to the B–H method. As shown in Fig. 4A, the B–H plot suggests that the binding energy constant (Kb) between the probe and H2S is 5.68 × 108 M−1 with 1
:
1 stoichiometry. This result is also observed from Job's plot analysis (Fig. 4B), a method for determining the stoichiometry of a binding event; the maximum PL intensity appears as the molar fraction of H2S is 0.5. Subsequently, for the response time of the probe to H2S, the change in PL intensity at 450 nm was gradually recorded over time (Fig. 4C), in which ten equivalents of H2S were used. The relative PL intensity (I/I0) approaches the maximum after 30 min. To investigate the kinetics of the bonding reaction, we assumed that the concentration and PL intensity are relevant to some extent, indicating that I0 can be expressed as the probe's concentration ([P0]) before sensing H2S. The intensity (I) at 30 min can be considered the primary signal of the probe/H2S complex, i.e., the probe completely reacts with H2S. Thus, the relative probe's concentration, [P]/[P0], and its natural logarithm values can be plotted over time (Fig. 4D and E). On the basis of the linearity relationship within 20 min of Fig. 4E, the responding mechanism belongs to a first-order reaction. The rate constant of reaction (kR) between the probe and H2S is 0.143 min−1, in which its responding half-life (t1/2 = ln
2/kR) can be estimated to be 4.85 min. Furthermore, the kR and t1/2 values were 1.05 min−1 and 39.5 s, respectively, as 50-fold H2S was added to the probe.
 |
| Fig. 4 H2S sensing mechanism of CMR-o-Py nanoaggregates as probes. (A) Benesi–Hildebrand plot and (B) Job's plot analysis for determining the stoichiometry ratio of the probe with H2S (×10 eq.), (C) relative PL intensity (I/I0), (D) relative probe's concentration ([P]/[P0]), and (E) ln([P]/[P0]) versus response time for different equivalents of H2S, (F) HRMS spectrum of CMR-o-Py linking HS−/S2− ions, and (G) speculated mechanism of CMR-o-Py sensing HS−/S2− ions. I0 and I are the PL intensities of the probe and their sensing H2S, respectively. [P0] and [P] are the estimated initial and time-dependent concentrations of the probe, respectively. | |
Further verifying the binding reaction with HRMS, as shown in Fig. 4F, two new peaks for probe/H2S complexes appear at m/z = 329.2423 and 330.2468, respectively, while the m/z value of CMR-o-Py is 296.1021 (predicted value 296.2980) without H2S existence. According to the Michael addition mechanism of imine group and nucleophilic sulfides, the complexes are surmised as CMR-o-Py linking S2− and HS− ions, respectively (Fig. 4G). The theoretical m/z values of complexes (329.0834 and 330.0912) correspond with HRMS peaks (Fig. 4F). In addition, the HRMS signal of the former is higher than that of the latter, explaining the specific detection of Na2S, as a source of H2S, by CMR-o-Py nanoaggregates. Based on the above-mentioned reliable sensing mechanism, the CMR-o-Py nanoaggregates inspire us to investigate their imaging ability towards H2S in the biological environment and plant tissue.
In vitro biocompatibility of CMR-o-Py
Owing to the high sensitivity of H2S, the CMR-o-Py nanoaggregates show potential for monitoring the change in biological H2S level. Before the biological application, the cytotoxicity of CMR-o-Py and its nanoaggregates on HeLa cells was investigated using a CCK-8 assay. After 24 h of incubation with CMR-o-Py (0–50 μM), the HeLa cells maintain good viability (Fig. S20, ESI†). At a subsequent experimental concentration of 20 μM, the cell viability is over 90%. The result demonstrates that CMR-o-Py and its nanoaggregates have good biocompatibility for cellular imaging.
Exogenous and endogenous H2S imaging in living cells
Exogenous and endogenous H2S imaging in HeLa cells was investigated to evaluate the biological applications of CMR-o-Py by confocal imaging. After incubation with 20 μM of CMR-o-Py for 2 hours, a very faint blue fluorescence appears in the normal group cells without any treatment, which may be caused by the small amount of endogenous H2S within cells reacting with CMR-o-Py (Fig. 5A and B). To verify whether H2S is the cause of weak intracellular blue fluorescence, the probe's ability to provide intracellular feedback was explored by varying the intracellular H2S levels. For the imaging of exogenous H2S, the normal HeLa cells were first incubated with 200 μM of Na2S and then further incubated with CMR-o-Py (20 μM). Compared with the normal group, the fluorescence of HeLa cells pre-treated with H2S was significantly enhanced (Fig. 5C and D). Moreover, to verify that the endogenous H2S level in cells can be up-regulated under Cys stimulation, the normal HeLa cells were pre-incubated with 400 μM of Cys and then 20 μM of CMR-o-Py. The experimental results indicate that the blue fluorescence in cells pre-incubated with Cys is also significantly enhanced (Fig. 5E and F), which is consistent with the obtained exogenous results (Fig. 5D). Based on the ability of N-ethylmaleimide (NEM) to remove the endogenous H2S within cells,30 a negative control experiment for a comparative study was further conducted. After incubation of 10 μM NEM and then 20 μM CMR-o-Py, the normal HeLa cells show no fluorescence to be observed (Fig. 5G and H). The significant reduction of fluorescence intensity in HeLa cells indicates that CMR-o-Py detects no H2S in the presence of NEM. The above-mentioned experimental results demonstrate the ability of CMR-o-Py to track both exogenous and endogenous H2S and provide feedback on content changes in H2S levels in the cells.
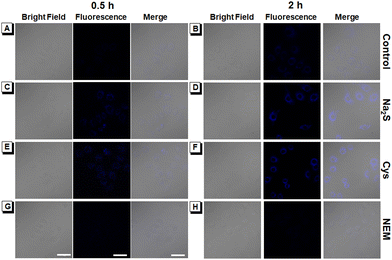 |
| Fig. 5 Confocal fluorescence imaging of exogenous and endogenous H2S in HeLa cells. HeLa cells were incubated with 20 μM of CMR-o-Py (A) and (B), Na2S (200 μM, 0.5 h) and then CMR-o-Py (20 μM) (C) and (D), Cys (400 μM, 0.5 h) and then CMR-o-Py (20 μM) (E) and (F) and NEM (10 μM, 0.5 h) and then CMR-o-Py (20 μM) (G) and (H) excitation wavelength: 405 nm; filter: 430–470 nm. Scale bar: 50 μm. | |
Endogenous H2S imaging in onion tissues
H2S is thought to play an essential regulatory role in plant tissues, such as Alliaceae (garlic and onion) and Brassicaceae (cabbage and wasabi), regulating and influencing many signaling events and cellular physiological processes.31 Recent evidence has shown that they also serve as natural H2S-donors for preventing and treating metabolic disorders.32,33 Directly detecting endogenous H2S and its potential release in higher plants is still a challenge. In this work, onion tissues were thus selected as the experimental object to inspect their endogenous H2S production by fluorescence imaging. As presented in Fig. 6B, bright blue fluorescence is observed in onion tissues after incubation with CMR-o-Py compared to the control group without the probe (Fig. 6A). To confirm the signal derived from endogenous H2S, the onion tissue was pre-treated with AOA, an inhibitor of cystathionine-β-synthase/cystathionine-γ-lyase suppressing H2S generation.34 As shown in the negative control experiment of Fig. 6C, only a very faint blue fluorescence can be observed. Based on these results, CMR-o-Py successfully monitors endogenous H2S produced from onion tissue. This suggests that visualizing endogenous H2S of plant tissue with AIE-active nanoaggregates will provide an advantageous analysis in some horticultural plants.
 |
| Fig. 6 Fluorescence imaging of endogenous H2S in onion tissues. (A) Onion tissue only. (B) Onion tissue was incubated with 20 μM of CMR-o-Py for 2 h. (C) After treatment with AOA (50 mM, 1 h), the onion tissue was incubated with 20 μM of CMR-o-Py for 2 h. Excitation wavelength: 405 nm; scale bar: 200 μm. | |
Conclusions
In this study, coumarin-based AIEgens have been successfully synthesized and their photophysical properties and AIE behaviors were regulated by introducing structurally isomerized aminopyridyl rotors. The theoretical calculation of TD-DFT implies that their electron distributions, energy levels, and dipole moments are attributed to different electron-withdrawing abilities of pyridyl isomers. Notably, CMR-o-Py, with a high dipole moment and low reorganization energy, shows a propensity for forming stable nanoaggregates, a characteristic beneficial for its use as a fluorescent probe in polar solvents and biological environments. CMR-o-Py nanoaggregates demonstrate high selectivity and sensitivity to H2S with a LOD of 18.1 μM (∼0.62 ppm) and respond well in the pH range of 6 to 8. By the B–H method and Job's plot analysis, the sensing H2S mechanism of CMR-o-Py nanoaggregates suggests a 1
:
1 binding stoichiometry and first-order reaction kinetics. Furthermore, CMR-o-Py nanoaggregates with good biocompatibility can monitor changes in both exogenous and endogenous H2S within cells. In onion tissues, CMR-o-Py nanoaggregates also successfully detect the production of endogenous H2S levels, demonstrating its potential for evaluating natural H2S-donor from plant tissues. This investigation provides not only a valuable tool for the real-time and in situ detection of H2S but also significant and innovative approaches for the early detection and diagnosis of conditions associated with sulfide ions.
Experimental section
Chemicals and instrumentation
All chemicals are of analytical grade. L-Cysteine (Cys), sodium hydrosulfide (NaHS), sodium sulfide (Na2S) as a source of hydrogen sulfide (H2S), N-ethylmalemide (NEM), and carboxymethoxylamine hemihydrochloride (AOA) were purchased from Sigma-Aldrich and Energy Chemical Co., Ltd and used directly without further purification. Dulbecco's modified Eagle's medium (DMEM), 10% fetal bovine serum (FBS), and 1% penicillin–streptomycin were obtained from TransGen Biotech Co., Ltd, and cell counting Kit-8 (CCK-8) was obtained from Beyotime Biotech Co. Ltd. The nuclear magnetic resonance (NMR) data were recorded using JEOL JNM-ECS400, and high-resolution mass spectra (HRMS) were recorded using a Bruker compact ESI-QTOF-MS. UV-vis absorption and photoluminescence (PL) spectra were recorded using an Avantes SensLine spectrometer (ProTrusTech, Taiwan) and a Horiba FluoroMax Plus fluorescence spectrometer, respectively. Dynamic light scattering (DLS) was analyzed using Litesizer DLS500. CCK-8 assay was recorded using SpectraMax i3 (Molecular Devices, Shanghai). Fluorescence imaging and confocal microscopic images were individually recorded using Leica DM4000M and Olympus FV3000.
Synthesis of coumarin-based materials
Same equivalents of 7-hydroxy-4-methyl-2-oxo-2H-chromene-8-carbaldehyde25 and hydrazine-ring were dissolved in ethanol. After refluxing for 8 h, the solution returned to room temperature, and the precipitates were filtered, washed with ethanol, and dried in vacuo.
CMR-Ph25.
Yellow solid, 98.0% of yield. 1H NMR (400 MHz, DMSO-d6) δ (ppm): 12.10 (br, NH), 10.81 (s, OH), 8.53 (s, 1H), 7.62 (d, J = 7.6 Hz, 1H), 7.29 (t, J = 7.3 Hz, 2H), 6.94–6.85 (m, 4H), 6.21 (s, 1H), 2.38 (s, 3H). 13C NMR (100 MHz, DMSO-d6) δ (ppm): 159.49, 159.43, 154.00, 151.21, 143.67, 133.61, 129.61, 125.97, 119.99, 113.00, 112.14, 111.78, 110.75, 106.73, 18.41. HRMS (ESI m/z): calculated for C17H14N2O3, [M + H]+ = 295.3100, found: 295.1065.
CMR-o-Py.
Yellow solid, 93.0% of yield. 1H NMR (400 MHz, DMSO-d6) δ (ppm): 12.36 (br, NH), 11.32 (s, OH), 8.69 (s, 1H), 8.20 (d, J = 5.6 Hz, 1H), 7.70 (t, J = 7.6 Hz, 1H), 7.64 (d, J = 8.8 Hz, 1H), 6.95 (d, J = 6.4 Hz, 1H), 6.87–6.82 (m, 2H), 6.23 (s, 1H), 2.38 (s, 3H). 13C NMR (100 MHz, DMSO-d6) δ (ppm): 159.93, 159.50, 154.90, 153.97, 151.57, 148.27, 138.37, 135.43, 126.42, 115.98, 113.20, 112.01, 110.70, 106.58, 106.45, 18.58. HRMS (ESI m/z): calculated for C16H13N3O3, [M + H]+ = 296.2980, found: 296.1021.
CMR-m-Py.
Yellow solid, 90.0% of yield. 1H NMR (400 MHz, DMSO-d6) δ (ppm): 11.38 (s, OH), 8.58 (s, 1H), 8.32 (s, 1H), 8.16 (d, J = 5.2 Hz, 1H), 7.78–7.68 (m, 2H), 7.67 (d, J = 8.8 Hz, 1H), 6.97 (d, J = 8.8 Hz, 1H), 6.22 (s, 1H), 2.37 (s, 3H). 13C NMR (100 MHz, DMSO-d6) δ (ppm): 159.51, 153.98, 151.86, 142.27, 137.35, 128.24, 127.11, 126.60, 124.19, 113.07, 112.32, 110.83, 106.71, 18.45. HRMS (ESI m/z): calculated for C16H13N3O3, [M + H]+ = 296.2980, found: 296.1021.
CMR-p-Py.
Yellow solid, 91.0% of yield. 1H NMR (400 MHz, DMSO-d6) δ (ppm): 8.69 (s, 1H), 8.31 (d, J = 6.8 Hz, 2H), 7.20 (d, J = 8.8 Hz, 1H), 7.21 (m, 2H), 6.97 (d, J = 8.8 Hz, 1H), 6.24 (s, 1H), 2.38 (s 3H). 13C NMR (100 MHz, DMSO-d6) δ (ppm): 160.17, 159.46, 154.17, 153.89, 152.56, 142.84, 141.01, 128.43, 113.10, 112.37, 110.87, 106.49, 18.45. HRMS (ESI m/z): calculated for C16H13N3O3, [M + H]+ = 296.2980, found: 296.1017.
Detection procedure of H2S
First, 10 μL of CMR-o-Py (1 mM in dimethyl sulfoxide; DMSO) was added to a volume of phosphate buffer solution (PBS, 4 mM, pH = 7.40) to prepare CMR-o-Py nanoaggregates as probes. The analytes were individually mixed with the 100 μM probe-containing buffer system (90 vol% PBS/DMSO), and the total solution volume was 1.0 mL. The buffer solutions were measured using a PL spectrometer at an excitation wavelength of 405 nm, in which excitation and emission slits were 1 and 5 nm, respectively. The experiment was conducted three times in parallel. Moreover, the limit of detection (LOD) for H2S was determined from the changes in PL intensities upon addition of different concentrations of Na2S (0–800 μM or 0–8 eq.). Based on the fluorescence titration data, the binding constant (Ka) was determined using the Benesi–Hildebrand (B–H) equation:35
where I0 is the intensity of the probe without H2S, and I and Imax are the intensities of the probe with different H2S concentrations (20–800 μM) and mixed with 800 μM H2S, respectively.
Cytotoxicity of living cells in vitro
The metabolic viability of HeLa cells was evaluated by a CCK-8 assay. DMEM supplemented with 10% FBS and 1% penicillin–streptomycin was used as the culture medium. HeLa cells were seeded in 96-well plates at a density of 5 × 103 cells per well.36 After culture in the incubator (5% CO2, 37 °C) for 24 h, the old medium was replaced using CMR-o-Py at 0, 6.25, 12.5, 25, 50, and 100 μM concentrations (1‰ DMSO in PBS solution). Upon incubation for another 24 h, 10 μL of CCK-8 solution was added and incubated at 37 °C for 4 h, and then the absorbance at 450 nm from each well was recorded using a microplate reader. The cell viability was expressed by the absorbance ratio from the cells treated with probes to that of the cells incubated with a culture medium only.
H2S imaging of living cells
For the control group, HeLa cells were incubated with CMR-o-Py (20 μM in 1‰ DMSO of 1× PBS solution) in the darkness. For the exogenous H2S imaging, the cells were pre-incubated with Na2S (200 μM in PBS) and then 20 μM CMR-o-Py. For the endogenous H2S imaging, the cells were pre-incubated with 400 μM Cys (the main precursor for H2S production by enzymatic catalysis) and then 20 μM CMR-o-Py. Finally, the cells were imaged by confocal microscopy (excitation wavelength: 405 nm; filter: 430–470 nm).
H2S imaging of onion tissues
Endogenous H2S imaging of onion tissues was conducted.37 Onion inner epidermal tissues were incubated with CMR-o-Py (20 μM in 1‰ DMSO of 1× PBS solution) for 2 h in the darkness. For negative control, the onion tissues were pre-incubated with 50 mM AOA (as H2S inhibitor) for 1 h, and then with 20 μM CMR-o-Py for 2 h. Onion tissues were washed three times with 1× PBS before fluorescence imaging.
Data availability
The data for this article, including molecular design, photophysical properties, theoretical calculation, and biological images, are original and available in this manuscript and ESI.†
Conflicts of interest
There are no conflicts to declare.
Acknowledgements
Y.-X. Wang, C.-C. Ni, and J.-S. Ni are grateful for financial support from the National Science and Technology Council (NSTC 111-2113-M-992-001-MY2 and NSTC 113-2113-M-992-001, Taiwan) and theoretical calculation support from the National Center for High-Performance Computing (NCHC, Taiwan). Y. Li, D. Liu, and J. Ni thank Wuxi “Taihu Light” Science and Technology Research Project (Y20232029, China) and Youth Projects of Wuxi Health Commission (Q202324, China).
Notes and references
- R. Wang, Physiol. Rev., 2012, 92, 791–896 CrossRef CAS PubMed.
- R. El-Shaheny, F. Belal, Y. El-Shabrawy and M. El-Maghrabey, Trends Environ. Anal. Chem., 2021, 31, e00133 CrossRef CAS.
- R. Liu, S. Zhang, H. Zeng, N. Gao, Y. Yin, M. Zhang and L. Mao, Angew. Chem., Int. Ed., 2024, 63, e202318973 CrossRef CAS PubMed.
- F. Rong, T. Wang, Q. Zhou, H. Peng, J. Yang, Q. Fan and P. Li, Bioact. Mater., 2023, 19, 198–216 CAS.
- K. M. Dillon, R. J. Carrazzone, J. B. Matson and K. Kashfi, Biochem. Pharmacol., 2020, 176, 113931 CrossRef CAS PubMed.
- H. Li, Y. Fang, J. Yan, X. Ren, C. Zheng, B. Wu, S. Wang, Z. Li, H. Hua, P. Wang and D. Li, TrAC, Trends Anal. Chem., 2021, 134, 116117 CrossRef CAS.
- W. C. Yang, S. Y. Li, S. Ni and G. Liu, Aggregate, 2024, 5, e460 CrossRef CAS.
- X. Zeng, W. Chen, C. Liu, J. Yin and G. F. Yang, J. Agric. Food Chem., 2021, 69, 13700–13712 CrossRef CAS PubMed.
- C. Ding and T. Ren, Coord. Chem. Rev., 2023, 482, 215080 CrossRef CAS.
- K. G. Fosnacht and M. D. Pluth, Chem. Rev., 2024, 124, 4124–4257 CrossRef CAS PubMed.
- W. Cai, T. Xin, L. Sun, C. Fan, G. Liao, Y. Tu, G. Liu and S. Pu, Spectrochim. Acta, Part A, 2024, 316, 124341 CrossRef CAS PubMed.
- T. Hong, S. Cheng, X. Zhong, Y. Zuo, Y. Dong, Z. Shi and Z. Zhao, J. Chin. Chem. Soc., 2023, 70, 136–144 CrossRef CAS.
- J. Ren, W. Zhang, X. Gao, B. Song and J. Yuan, Chem. Eng. J., 2024, 486, 150349 CrossRef CAS.
- X. Cai, J. Zhang, H. Ye, K. Cui, T. Hao, L. Yi and X. Yang, Chem. – Eur. J., 2023, 29, e202301105 CrossRef CAS PubMed.
- H. Jiang, Q. Zhang, N. Li, Z. Li, L. Chen, F. Yang, S. Zhao and X. Liu, J. Hazard. Mater., 2024, 467, 133735 CrossRef CAS PubMed.
- Y. Zhang, Y. Chen, X. Shi, Y. Bai, W. He and Z. Guo, New J. Chem., 2022, 46, 21464–21469 RSC.
- K. Xu, L. Gao, Y. Liang, Z. Meng, S. Gong, Z. Wang and S. Wang, J. Food Compos. Anal., 2024, 127, 106005 CrossRef CAS.
- T. He, W. J. Guo, Y. Z. Chen, X. F. Yang, C. H. Tung and L. Z. Wu, Aggregate, 2023, 4, e250 CrossRef CAS.
- Y. Duo, Z. Xiang, G. Gao, G. Luo and B. Z. Tang, TrAC, Trends Anal. Chem., 2023, 167, 117252 CrossRef CAS.
- J.-S. Ni and G.-H. Lu, Spectrochim. Acta, Part A, 2023, 300, 122908 CrossRef CAS PubMed.
- L. Huang, Y. Zhou, D. Jiao, J. Ren, Y. Qi, H. Wang, Y. Shi, D. Ding and X. Xue, Aggregate, 2023, 5, e403 CrossRef.
- J.-S. Ni, X. Zhang, G. Yang, T. Kang, X. Lin, M. Zha, Y. Li, L. Wang and K. Li, Angew. Chem., Int. Ed., 2020, 59, 11298–11302 CrossRef CAS PubMed.
- P.-Y. Ho, T. Y. Chou, C. Kam, W. Huang, Z. He, A. H. W. Ngan and S. Chen, Sci. Adv., 2023, 9, eabn5390 CrossRef PubMed.
- F. Wang, P. Y. Ho, C. Kam, Q. Yang, J. Liu, W. Wang, E. Zhao and S. Chen, Aggregate, 2023, 4, e312 CrossRef CAS.
- R. G. Kalkhambkar, G. M. Kulkarni and M. V. Kulkarni, J. Heterocycl. Chem., 2013, 50, 1014–1020 CrossRef CAS.
- A. F. M. Barton, Chem. Rev., 1975, 75, 731–753 CrossRef CAS.
- S. Lin, Q. Ou, Q. Peng and Z. Shuai, J. Chin. Chem. Soc., 2023, 70, 287–296 CrossRef CAS.
- Y.-C. Yang, J. S. Lin and J.-S. Ni, J. Mater. Chem. A, 2023, 11, 26164–26172 RSC.
- R. Huang, M. Li, D. Lin, Y. Shao, C. Shang, Q. Liu, G. Liu, N. Li, R. Miao, H. Peng, Y. Tang and Y. Fang, Aggregate, 2023, 4, e203 CrossRef CAS.
- E. R. Mojica, S. Kim and D. S. Aga, Appl. Environ. Microbiol., 2008, 74, 323–326 CrossRef CAS PubMed.
- M. A. Munoz-Vargas, S. Gonzalez-Gordo, J. M. Palma and F. J. Corpas, Int. J. Mol. Sci., 2022, 23, 5648 CrossRef CAS PubMed.
- J. Spezzini, E. Piragine, L. Flori, V. Calderone and A. Martelli, Pharmacol. Res., 2024, 38, 2388–2405 CAS.
- T. Nohara, Y. Fujiwara, M. El-Aasr, T. Ikeda, M. Ono, D. Nakano and J. Kinjo, J. Nat. Med., 2021, 75, 741–751 CrossRef CAS PubMed.
- A. Ahmad and C. Szabo, Pharmacol. Res., 2016, 113, 348–355 CrossRef CAS PubMed.
- Z. Bai, H. Zhang, R. Xue, X. Lu, X. Li, W. MacSwain, W. Zheng, J. Xu, Y. Yu and Y. L. Bai, Aggregate, 2024 DOI:10.1002/agt2.583.
- G. Q. Zhang, W. Feng, Z. Gao, G. L. Zhang, X. Wu, Y. Xiao, X. Li, L. Zheng, D. Ding, J. Guo and B. Situ, Aggregate, 2023, 4, e286 CrossRef CAS.
- N. Wei, Z. Y. Liang, W. L. Fang, X. F. Guo, H. Wang and H. X. Zhang, J. Colloid Interface Sci., 2023, 642, 145–153 CrossRef CAS PubMed.
Footnotes |
† Electronic supplementary information (ESI) available: The detailed synthesis route, 1H and 13C NMR, HRMS, calculation data, PL spectra in different DMSO/PBS mixture solutions, and cell viability. See DOI: https://doi.org/10.1039/d4tb01617c |
‡ The first two authors have contributed equally to this work. |
|
This journal is © The Royal Society of Chemistry 2024 |
Click here to see how this site uses Cookies. View our privacy policy here.