DOI:
10.1039/D3TC04701F
(Paper)
J. Mater. Chem. C, 2024,
12, 3465-3473
Highly efficient and ultra-stable CsPbBr3 composites for LCD devices and X-ray imaging†
Received
20th December 2023
, Accepted 7th February 2024
First published on 19th February 2024
Abstract
Achieving excellent optical properties and stability is crucial for the practical utilization of perovskite emitters. Herein, ultra-stable CsPbBr3-DPSI/MS composites with a high photoluminescence quantum yield (PLQY) of 93.2% are obtained through passivation of the surface of CsPbBr3 quantum dots (QDs) by 3-(decyldimethylammonio)-propane-sulfonate inner salt (DPSI) and encapsulating these QDs using silica molecular sieve (MS) templates. After aging for 1000 hours under harsh synergistic conditions (i.e., temperature of 60 °C, relative humidity of 90%, and blue light irradiation with a power density of 3500 W m−2), these composites still retain 90% of their initial photoluminescence (PL) intensity. To the best of our knowledge, these CsPbBr3-DPSI/MS composites represent the most stable CsPbBr3 emitters in an accelerated aging test under these synergistic humidity–heat–light conditions. The liquid crystal display (LCD) backlight module utilizing these stable composites shows a wide color gamut of 111.7% NTSC. Furthermore, these CsPbBr3-DPSI/MS composites exhibit exceptional X-ray scintillator performance and impressive radiation hardness, delivering a high X-ray imaging spatial resolution of up to ≈16 lp mm−1 and a low detection limit of 339 nGyair s−1.
1. Introduction
All-inorganic CsPbX3 (X = Cl, Br, I) quantum dots (QDs) possess excellent optical and electrical properties,1 including a widely tunable bandgap,2 narrow emission width,3 and high photoluminescence quantum yield (PLQY).4 These properties make them promising candidates for various optoelectronic applications such as full-color liquid crystal displays (LCD),5,6 light-emitting diodes (LED),7,8 solar cells,9,10 and X-ray detectors.11,12 Despite these merits, the severe structural instability deriving from their vulnerable ionic bond remains a major obstacle to their industrialization.13–15 Exposure to moisture, oxygen, high-energy light, or heat can cause CsPbX3 QDs to undergo a phase transition or generate surface defects, resulting in an irreversible luminescence quenching.16 Fortunately, the stability of CsPbX3 QDs can be improved via shelling to reduce surface defects and insulate these QDs from the external environment.17 For instance, Bakr et al. demonstrated that zwitterionic lecithin ligands can stabilize the perovskite phase of CsPbI3 QDs for long periods in air.18 Kovalenko et al. reported that the surface passivation in CsPbBr3/KBr nanocomposites suppresses the PL thermal quenching of CsPbBr3 QDs.19 Although many efforts have been made to boost either the moisture-, thermal-, or photostability of CsPbX3 QDs, the developed CsPbX3 QDs still cannot tolerate the real application atmosphere of synergistic humidity, heat, and high-energy light irradiation.20
One of the promising strategies to improve the overall (thermal-, moisture-, and photo-) stability of CsPbX3 QDs is to encapsulate them in robust inorganic matrices, such as metal oxides (e.g., SiO2 and Al2O3),21–25 or glasses.26–30 These matrices isolate CsPbX3 QDs from moisture and O2, prevent ion migration, and inhibit inter-particle fusion at high temperature.22 Studies have demonstrated that CsPbX3 QDs embedded in inorganic matrix templates can survive in water, acidic solution, and heating for a long time.31 However, these inorganic matrices tend to be amorphous or possess a mismatched lattice constant with CsPbX3, which results in a lack of surface passivation effect for inside CsPbX3 QDs. Consequently, the enrichment of surface defects adversely impacts PLQY and photo-stability.32–34 For example, Xuan et al. reported CsPbBr3:Sr/PbBr(OH)/silicon oxide composites with high stability when exposed to water and heat. However, these composites showed unsatisfactory photo-stability, with only 54% of their initial PL intensity being retained after aging for 720 minutes under blue light with an intensity of 1500 W m−2.35 Zhang et al. reported that CsPbBr3 QDs embedded in silica can survive in water for 50 days without a visible drop in PL intensity, but exhibit a relatively low PLQY of 63%.22
Herein, we show CsPbBr3 QDs with both highly efficient and overall stability, which is achieved by in situ passivation and encapsulation of CsPbBr3 QDs in silica molecular sieve (MS) templates through a solid-state synthesis. Briefly, the obtained CsPbBr3 QDs are passivated by 3-(decyldimethylammonio)-propane-sulfonate inner salt (DPSI) and encapsulated by silica MS matrices. The resulting CsPbBr3-DPSI/MS composites exhibit a high PLQY of 93.2% and significantly improved stability against water, heat, and blue light irradiation. Even after aging for 1000 hours under harsh synergistic conditions (i.e., temperature of 60 °C, relative humidity of 90%, and blue light irradiation with a power density of 3500 W m−2), these CsPbBr3-DPSI/MS composites still retain 90% of their initial PL intensity. An LCD device presenting a wide color gamut of 111.7% National Television Standards Committee (NTSC) is obtained, when employing these stable composites as the green light source of the LCD backlight. Furthermore, the CsPbBr3-DPSI/MS composites exhibit a promising foreground in high-performance X-ray imaging detectors, achieving a high spatial resolution of 16 lp mm−1 and a detection limit of 339 nGyair s−1 that is much lower than the requirement of X-ray medical diagnostics of ≈5.5 μGyair s−1.
2. Experiment section
2.1 Materials
Cesium bromide (CsBr, 99.9%), lead bromide (PbBr2, 99.9%), and 3-(decyldimethylammonio)-propane-sulfonate inner salt (DPSI, 98%) were purchased from Macklin. The MCM-41 MSs were purchased from XFNANO. Cyclohexane was purchased from Sinopharm Chemical Reagent Co., China. All the chemicals were used as-received without further purification.
2.2 Solid-state syntheses of CsPbBr3-DPSI/MS composites
0.0425 g CsBr, 0.0733 g PbBr2, and 0.0307 g DPSI were dispersed in 0.1505 g MCM-41 MS powder and the mixture was stirred for 20 min. The obtained powder was calcined at 560 °C for 30 min with a heating rate of 20 °C min−1 in a tube furnace with 1 in diameter quartz tube under an air atmosphere of 0.05 MPa. After cooling to room temperature at a cooling rate of 4.7 °C min−1, the CsPbBr3-DPSI/MS composites were acquired.
2.3 Preparation of scintillator films
The CsPbBr3-DPSI/MS composite solution (≈0.1 g mL−1 in cyclohexane) was spin-coated on a glass substrate at 500 rpm for 30 s and then dried on the hot plate at 60 °C for 5 min. These procedures were repeated 5 times until the desired film thickness was achieved.
2.4 Fabrication of diffusion plates
Polystyrene plastic particles were mixed with CsPbBr3-DPSI/MS composites in a twin-screw extruder with the 180 °C heating temperature. After the melt extrusion process, the CsPbBr3-DPSI/MS composites were embedded in plastic. A plate molding press machine was used to turn these mixed particles into a diffusion plate.
2.5 Composition and structural characterization
Fourier-transform infrared spectroscopy data were measured using a Thermo Scientific Nicolet iS5 system. X-ray photoelectron spectroscopy data were measured using a Thermo Fisher, ESCALAB Xi+. Crystal structures were obtained using a Rigaku D/MAX 2500 with Cu-Kα sources (λ = 0.1542
nm) and a transmission electron microscope (TEM) (FEI Tecnai G2 F20). Elemental compositions of the samples were characterized via a TEM (FEI Tecnai G2 F20) combined with EDS.
2.6 Optical characterization
Steady-state and time-resolved PL spectra were measured using a Zolix Instruments OmniFluo900 spectrometer equipped with a picosecond pulsed laser diode (405 nm, 58.6 ps). Time- and wavelength-dependent PL mapping was performed with a streak camera (Zolix, ST10) under the illumination of a light conversion Pharos Yb:KGW laser (PHAROS PH2, 405 nm, 200 fs, 1 kHz). Spectral thermal stability was examined using a 405 nm continuous wave laser as the excitation source and an FX 2000 spectrometer to obtain the PL signals. Spectral photostability was examined using a 455 nm LED as the excitation source and an FX 2000 spectrometer to obtain the PL signals. PLQYs were evaluated by using a fluorescence spectrometer (Ocean Insight, QEPro) with an integrated sphere excited at a wavelength of 410 nm using an LED chip source.
2.7 Radioluminescence and X-ray imaging
Radioluminescence (RL) emission spectra and RL stability tests were performed using an X-ray source (Magpro, TUB00146-W06, 12 W) and a spectrometer (Edinburgh, FLS 980). X-ray images of samples were taken using the above-mentioned X-ray source and a QHY9701 camera without any filter. The radiation stability was tested under ambient air conditions with relative humidity of about 40–60% and temperature of about 15–25 °C.
3. Results and discussion
CsPbBr3-DPSI/MS composites were synthesized through a solid-state synthesis method (Fig. 1), in which CsBr, PbBr2, and DPSI powders were mixed with silica MS templates, and heated at 560 °C for 30 min. During the heating process, CsBr reacted with PbBr2 to form CsPbBr3 QDs in the mesopores of silica MS templates, and DPSI tightly bound to the surface of the CsPbBr3 QDs due to the interaction between the negatively charged SO3− group in DPSI and the uncoordinated Pb2+ in CsPbBr3 QDs.36 With the heating going on, the porous structure of the silica MS templates gradually collapsed, encapsulating the internal QDs and ultimately obtaining the CsPbBr3-DPSI/MS composites. Brunauer–Emmett–Teller (BET) and transmission electron microscopy (TEM) measurements were conducted to characterize the collapse of MS templates (Fig. S1, ESI†). The BET result shows that the surface area of MS templates decreases from 998 to 15 m2 g−1 after the solid-state syntheses, indicating that the porous structure of the MS templates gradually collapses during the heating process. Besides, the TEM image of original MS templates shows a wide distribution of mesoporous in the MS. After the solid-state synthesis, it can be observed that CsPbBr3 nanocrystals were encapsulated in the MS, and the mesoporous structures of MS were collapsed.
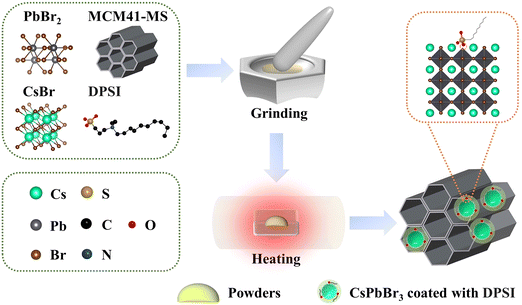 |
| Fig. 1 Schematic of the syntheses of the CsPbBr3-DPSI/MS composites and the defect passivation mechanism of DPSI. | |
The coordination of DPSI on the surface of CsPbBr3 QDs was investigated by Fourier-transform infrared (FT-IR) spectroscopy and X-ray photoelectron spectroscopy (XPS). As shown in Fig. 2a, the S
O stretching vibration peak of DPSI at 1044 cm−1 shifts to a lower wavenumber of 1021 cm−1 in CsPbBr3-DPSI/MS composites, indicating the lone electron pair donation from O of DPSI to the Pb2+ empty orbitals of CsPbBr3.37 The presence of DPSI in the obtained samples was further confirmed by the observation of S 2p and N 1s peaks in the XPS spectra (Fig. 2b and Fig. S2, ESI†). Meanwhile, the Pb 4f and Br 3d peaks of CsPbBr3-DPSI/MS composites shift towards lower binding energy compared to those of the reference sample (Fig. 2c and d), indicating chemical interactions between the SO3− group in DPSI and the Pb2+ in CsPbBr3 QDs.38 TEM together with energy-dispersive X-ray spectroscopy (EDS) was carried out. Fig. 2e shows that the EDS mapping images of Cs, Pb, Br, S, N, and C elements all overlap with the TEM image of CsPbBr3-DPSI/MS composites, which indicates that CsPbBr3 QDs with DPSI are uniformly distributed inside in the silica MS template. Fig. 2f gives the high-resolution TEM image of the CsPbBr3-DPSI/MS composite and the corresponding diffraction pattern, in which two lattice spacings of 0.33 nm and 0.29 nm are observed, corresponding to the (111) plane and (200) plane of cubic CsPbBr3. The X-ray diffraction (XRD) result (Fig. 2g) also confirms the cubic CsPbBr3 phase in the obtained CsPbBr3-DPSI/MS composites.
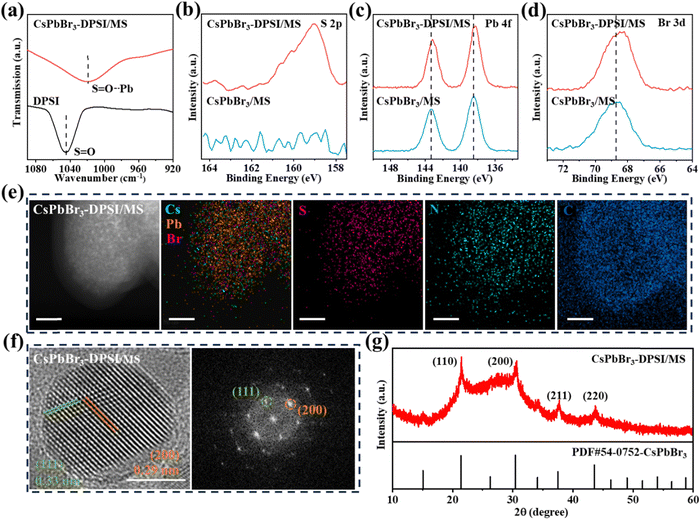 |
| Fig. 2 Evidence of DPSI coordinating on the surface of CsPbBr3 QDs and structural characterization of the CsPbBr3 QDs. (a) FT-IR spectra of the DPSI and CsPbBr3-DPSI/MS. XPS spectra for CsPbBr3/MS and CsPbBr3-DPSI/MS: (b) S 2p, (c) Pb 4f, (d) Br 3d. (e) TEM image and the corresponding elemental mapping images of the CsPbBr3-DPSI/MS. Scale bar: 50 nm. (f) HRTEM image and the corresponding diffraction pattern obtained by FFT. Scale bar: 5 nm. (g) PXRD pattern of the CsPbBr3-DPSI/MS. | |
Optical measurements were used to investigate the emission and the related electronic properties of the obtained CsPbBr3-DPSI/MS composites. Compared with the reference sample (CsPbBr3/MS composites), the PLQY of CsPbBr3-DPSI/MS composites is significantly enhanced from 27.4% to 93.2% (Fig. 3a and b). This enhancement suggests a substantial reduction in non-radiative losses, which can be attributed to effective defect passivation. The PLQY of the obtained samples as a function of the DPSI additive amount in precursors is shown in Fig. S3 (ESI†). When increasing the DPSI additive amount from 15 to 44 mg, the PLQY of CsPbBr3 nanocrystals gradually increases and reaches the highest PLQY at an DPSI additive amount of 37 mg. To get further insight into the recombination mechanism of the photogenerated carriers, we employed transient spectroscopies to investigate the PL decay process of the CsPbBr3-DPSI/MS composites and the reference sample (CsPbBr3/MS composites). As shown in Fig. 3c, the time-resolved photoluminescence (TRPL) decay curves are fitted using the biexponential equation
|  | (1) |
where
a1 and
a2 are amplitudes;
τ1 and
τ2 are lifetimes for fast and long PL decay processes (details shown in Table S1, ESI
†).
39 For the CsPbBr
3-DPSI/MS composites, the fast and the long components are respectively 8.32 ns (78.91%) and 43.59 ns (21.09%), while those for the reference sample are respectively 11.97 ns (54.65%) and 96.88 ns (45.35%). The lifetime of the fast components agrees well with that of the exciton radiation recombination in CsPbBr
3.
40 According to the previous reports, apart from the nonradiative recombination, doped electrons can detrap from shallow defect states to conduction band minimum for radiative recombination with a longer lifetime than exciton radiation (see
Fig. 3d).
39 Therefore, we attribute the long components to the interaction between excitons and defects. For CsPbBr
3-DPSI/MS composites, the significant percentage decrease for the long component is indicative of the defect passivation, and the percentage increase for the fast component indicates the improvement of the exciton radiation recombination. Furthermore, a streak camera equipped with a femtosecond laser of 405 nm (1 kHz, 200 fs) was used to get insights into the PL decay with the time scale of hundred picoseconds that is related to the surface state recombination (see
Fig. 3e and f).
41 The average PL lifetime is extended from 323 ps for CsPbBr
3/MS composites to 454 ps for CsPbBr
3-DPSI/MS composites, which confirms the effective passivation on the surface of CsPbBr
3 QDs by DPSI. Besides, we have further compared the full width at half maxima (FWHM) of CsPbBr
3-DPSI/MS composites with several reported phosphors (Table S2, ESI
†). FWHM of CsPbBr
3-DPSI/MS can reach to 25 nm, which is comparable to that of previously reported CsPbBr
3-Beta composites and CsPbBr
3@glass nanocomposites.
 |
| Fig. 3 Optical properties of CsPbBr3 QDs. (a) PL spectra of the CsPbBr3/MS and CsPbBr3-DPSI/MS. (b) Photographs of the CsPbBr3/MS and CsPbBr3-DPSI/MS (immersed in water) under UV excitation at 365 nm. (c) TRPL decay curves of the CsPbBr3/MS and CsPbBr3-DPSI/MS. (d) Schematic representation of the PL processes related to the exciton and exciton–shallow defects interactions. Time- and wavelength-dependent PL mapping were measured with a streak camera under the illumination of a femtosecond laser of 405 nm (1 kHz, 200 fs) for (e) CsPbBr3/MS and (f) CsPbBr3-DPSI/MS. | |
Next, we investigated the stability of the CsPbBr3-DPSI/MS composites against water, heat, and photoirradiation. The CsPbBr3-DPSI/MS composites were stable in water for 250 hours with almost no drop in the PL intensity (Fig. S4, ESI†), indicating that the MS matrix provided excellent water resistance for inside CsPbBr3 nanocrystals by isolating them from the external environment. As shown in Fig. 4a, when the heating temperature is increased from 300 K to 333 K and 373 K, the PL intensity of CsPbBr3-DPSI/MS retains 84% and 41% of their initial PL intensity, respectively. In contrast, the PL intensity of CsPbBr3/MS decreases to 65% and 25% of their initial intensity at 333 K and 373 K, respectively. These results indicate that the DPSI can enhance the heat stability of CsPbBr3 QDs. In addition, DPSI can greatly improve the photo-stability of CsPbBr3 QDs. Under blue light irradiation with a power density of 3500 W m−2, the PL intensity of the CsPbBr3-DPSI/MS composites is still maintained above 100% under irradiation for 48 h (Fig. 4b and d). In contrast, the PL intensity of the CsPbBr3/MS composites drops to 41% of their initial values under the same irradiation for 38 h (Fig. 4c and d). These results suggest that the improved photo and thermal stability of the CsPbBr3-DPSI/MS composites originated from the effective passivation by DPSI, preventing the formation of photo- or thermal-induced defects in CsPbBr3 QDs. Furthermore, to verify the overall stability of CsPbBr3-DPSI/MS composites under the real application conditions of synergistic humidity, heat, and high-energy light irradiation, we conducted accelerated operational stability tests on these composites under high temperature (HT 60 °C), high humidity (RH 90%), and 3500 W m−2 blue light irradiation. After aging for 1000 h, the CsPbBr3-DPSI/MS composites still retain 90% of their initial PL intensity (Fig. 4e). To the best of our knowledge, these CsPbBr3-DPSI/MS composites represent the most stable CsPbBr3 emitters in an accelerated aging test under synergistic humidity–heat–light conditions compared with the previously reported CsPbBr3 emitters (Table S3, ESI†). Besides, these CsPbBr3-DPSI/MS composites exhibit great chemical stability. We directly mixed these composites with 1 mol L−1 KI, 1 mol L−1 KCl, and 1 mol L−1 HCl aqueous solutions, respectively. The CsPbBr3 nanocrystals maintain their own PL characteristics without obvious peak shifts or fluorescence quenching even after 72 hours (Fig. S5, ESI†).
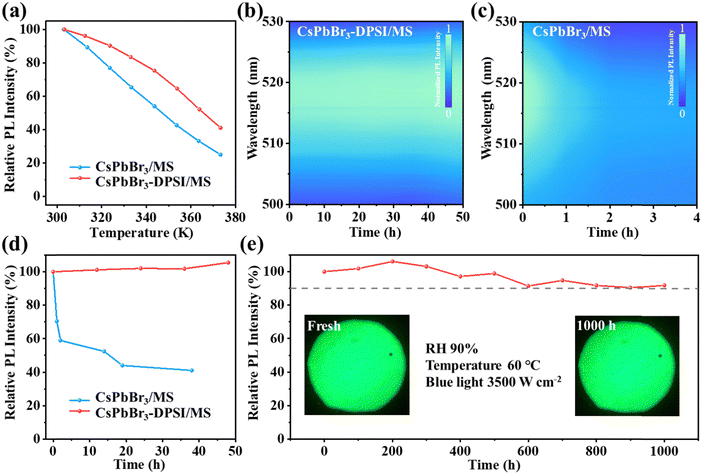 |
| Fig. 4 Thermal and photo stability of the CsPbBr3 QDs. (a) Temperature-dependent relative integrated PL intensity of the CsPbBr3/MS and CsPbBr3-DPSI/MS. Pseudo-color maps of the time-dependent PL spectra of the (b) CsPbBr3-DPSI/MS and (c) CsPbBr3/MS subjected to blue light irradiation. (d) Time-dependent relative integrated PL intensity of the CsPbBr3/MS and CsPbBr3-DPSI/MS subjected to blue light irradiation. (e) Time-dependent relative integrated PL intensity of the CsPbBr3-DPSI/MS composites-based film subjected to the harsh aging conditions (temperature: 60 °C, relative humidity: 90%, blue light irradiation with a high power density of 3500 W m−2), inset figures are photographs of the CsPbBr3-DPSI/MS composite based film under 365 nm UV light irradiation. | |
The high luminescence efficiency and overall stability ensure that the CsPbBr3-DPSI/MS composites are potentially applicable to commercial display and imaging applications, such as full-color LCD and X-ray scintillator materials.42–44 As a demo-of-concept, a diffusion plate based on the CsPbBr3-DPSI/MS composites (CPB-plate) was fabricated by a hot-melt extrusion method, which was employed as down-converters for an LCD backlight (Fig. 5a). The PL spectrum of the CPB-plate exhibits a narrow green emission with the center peak at 520 nm (inset in Fig. 5a). Fig. 5b shows the construction of the LCD device equipped with a CPB-plate (CPB-LCD), where purple LED chips (composed of blue LED and K2SiF6:Mn4+ powders, Fig. S6, ESI†) were used to excite the emission of the CPB-plate and provide the red and blue light. Fig. S7 (ESI†) shows the transmittance spectrum of three primary colors in the LCD module. Fig. 5c gives the white-light spectra of the CPB-LCD and an ordinary commercial LCD (OC-LCD), respectively. In comparison, the green part of the spectrum in CPB-LCD is narrower than that in OC-LCD. Fig. 5d and e exhibit the spectra of three primary colors in CPB-LCD and OC-LCD, respectively. The three primary color coordinates of CPB-LCD and OC-LCD are shown in Table S4 (ESI†). Both the blue light and red light in CPB-LCD are similar to that in OC-LCD, while the FWHM of green light in CPB-LCD (19.3 nm) is smaller than that in OC-LCD (40.7 nm), which results in the color gamut area of CPB-LCD (111.7% NTSC) being wider than that of OC-LCD (95.2% NTSC) (Fig. 5f). The picture of a green gecko on the CPB-LCD presents a more vivid green color in comparison with the OC-LCD (Fig. 5g). These results prove that the CsPbBr3-DPSI/MS composites are a promising candidate for the fabrication of LCD with a wide color gamut.
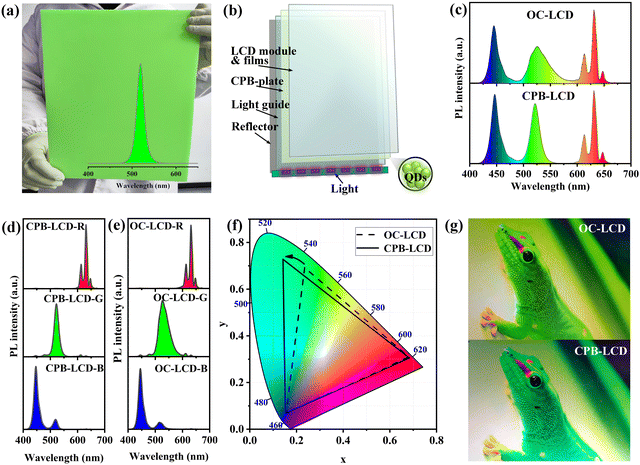 |
| Fig. 5 Optical characterization and performance of LCD application based on CsPbBr3-DPSI/MS composites. (a) Optical image and PL spectrum of the CPB-plate. (b) Schematic of the CPB-LCD construction. (c) White-light spectra of OC-LCD and CPB-LCD. (d) and (e) Spectra of three primary colors in CPB-LCD and OC-LCD, respectively. (f) Color gamut coverage of CPB-LCD and OC-LCD. (g) Pictures displayed on CPB-LCD and OC-LCD. | |
Furthermore, the feasibility of the CsPbBr3-DPSI/MS composites in an X-ray imaging detector was evaluated by a home-made X-ray imaging projection system, where an X-ray source, a test object, a CsPbBr3-DPSI/MS composite film and a charge-coupled device (CCD) camera were placed in sequence as exhibited in Fig. 6a. Fig. S8 (ESI†) shows the calculated attenuation with coherent scattering of CsPbBr3 at a different X-ray photon energy, indicating that the X-ray absorption efficiency of CsPbBr3 is comparable to that of other commercial scintillators (Bi4Ge3O12, CsI). Thanks to their strong X-ray absorption and high luminescence efficiency, the radioluminescence (RL) intensity of CsPbBr3-DPSI/MS composites is stronger than that of commercial Bi4Ge3O12 scintillators (Fig. 6b). The X-ray imaging system based on the CsPbBr3-DPSI/MS composites film exhibits a low detection limit of 339 nGyair s−1 (Fig. 6c), which is about 16 times lower than that required for commercial computed tomography (5500 nGyair s−1).45 Besides, the RL intensity of CsPbBr3-DPSI/MS composites has a wide linear response range from 390 nGyair s−1 to 23.1 mGyair s−1, which is beneficial for obtaining high-contrast images (Fig. S9, ESI†).46 Impressively, the CsPbBr3-DPSI/MS composites exhibit excellent radiation stability. As shown in Fig. 6d, under cyclical X-ray irradiation with a stepwise increase in the dose rate, the CsPbBr3-DPSI/MS composites are extremely robust without obvious degradation in the RL intensity. Then a fatigue test was performed on the CsPbBr3-DPSI/MS composites with an accumulated irradiation dose of ≈262 Gy at room temperature (50 kV, dose rate ≈23.1 mGyair s−1). As shown in Fig. 6e, even after exposure to such a high fluence and large dose of X-ray irradiation, the CsPbBr3-DPSI/MS composites still retain 90% of their initial RL intensity, which is comparable to other perovskites or perovskite-like metal halide scintillators with outstanding stability, such as CsPbBr3@PbBrOH (keep stable under the X-ray irradiation with a total dose of 0.338 Gy) and Cs3Cu2I5 (following exposure to X-ray irradiation at a rate of 17.4 mGyair s−1 and a cumulative dose of 43 Gy, the RL intensity retains 78% of its initial value).47,48 The X-ray imaging system based on the CsPbBr3-DPSI/MS composite film achieves a spatial resolution of ≈16 lp mm−1, as obtained from an X-ray image of a standard X-ray test pattern plate (Fig. 6f), which is even superior to most perovskite-based X-ray imaging systems (Table S5, ESI†). To assess the feasibility of the X-ray imaging system based on the CsPbBr3-DPSI/MS composites, we presented several examples of non-destructive testing for objects with complex internal structures that are opaque to visible light. Fig. 6g and h show images of a chip and a capsule containing an internal spring under both white light and X-rays, respectively, where the internal structures of both samples can be clearly exhibited under X-ray irradiation. These experimental results highlight the great potential of the CsPbBr3-DPSI/MS composites in high-resolution X-ray imaging.
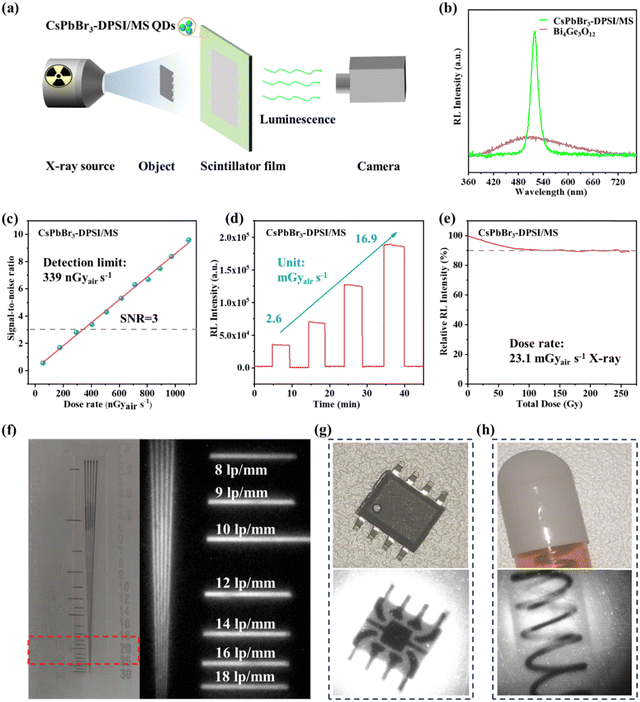 |
| Fig. 6 Radioluminescence characterization of CsPbBr3-DPSI/MS based scintillator film and X-ray imaging demonstrations. (a) Schematic of X-ray imaging system. (b) RL spectra of CsPbBr3-DPSI/MS and Bi4Ge3O12. (c) The signal-to-noise of X-ray response for the CsPbBr3-DPSI/MS-based scintillator film as a function of X-ray dose rate. (d) RL intensity of the CsPbBr3-DPSI/MS-based scintillator film under cyclical X-ray irradiation with a stepwise increased dose rate from 2.6 to 16.9 mGyair s−1. (e) Relative RL intensity of the CsPbBr3-DPSI/MS-based scintillator film as a function of total X-ray dose under continuous irradiation of 23.1 mGyair s−1 X-ray. (f) Bright-field (left) and X-ray (right) images of standard X-ray test pattern plate. (g) Bright-field (top) and X-ray (bottom) images of a chip. (h) Bright-field (top) and X-ray (bottom) images of a capsule containing an internal spring. | |
4. Conclusions
In summary, CsPbBr3-DPSI/MS composites with excellent PL properties and overall stability (against heat, moisture, and photo-irradiation) were synthesized through a solid-state synthesis method. In these composites, CsPbBr3 QDs were passivated by DPSI and followed by further encapsulation by silica MS matrices, leading to an enhanced PLQY of 93.2% and remarkable stability under harsh synergistic humidity–heat–light aging conditions. The CsPbBr3-DPSI/MS composites retained more than 90% of their initial PL intensity after aging for 1000 hours under the following synergistic conditions: temperature of 60 °C, relative humidity of 90%, and blue light irradiation with a power density of 3500 W m−2. The LCD backlight module utilizing these stable composites shows a wide color gamut of 111.7% NTSC. Besides, these CsPbBr3-DPSI/MS composites were applied in X-ray imaging with excellent radiation stability, a low detection limit of 339 nGyair s−1, and a spatial resolution of 16 lp mm−1. Our work clarifies the crucial roles of defect passivation and encapsulation in achieving highly efficient and stable CsPbBr3 QDs, and promising practical application of perovskite emitters.
Author contributions
Peng Wang (data curation: equal; formal analysis: equal; writing – original draft: equal), Zhaoyu Wang (formal analysis: equal; methodology: equal; writing – original draft: equal; writing – review & editing: equal), Meiyi Zhu (data curation: equal; methodology: equal; writing – review & editing: equal), QiuTing Cai (methodology: supporting), Hanyan Huang (conceptualization: supporting), Chengyuan Tang (formal analysis: supporting), Haoran Zhang (methodology: supporting), Chao Fan (conceptualization: lead; formal analysis: lead; methodology: lead; writing – original draft: lead), Xingliang Dai (conceptualization: equal; data curation: equal), Haiping He (conceptualization: equal; validation: lead; writing – review & editing: equal), Zhizhen Ye (funding acquisition: lead; project administration: equal; writing – review & editing: lead).
Conflicts of interest
There are no conflicts to declare.
Acknowledgements
This work was supported by the National Natural Science Foundation of China (no. 52072337 and 52102188), the Key Research and Development Program of Zhejiang Province (no. 2021C01030), the Young Elite Scientists Sponsorship Program by CAST (YESS20210444), the Natural Science Foundation of Zhejiang Province (LQ21F040005), the Shanxi-Zheda Institute of Advanced Materials and Chemical Engineering (grant no. 2022SZ-TD004), Leading Talent Entrepreneurship Project of Ouhai District (Wenzhou City), and the Project funded by China Postdoctoral Science Foundation (no. 2022TQ0267), the Project funded by China Postdoctoral Science Foundation (512200-X92203) and the Project funding for Postdoctors of Zhejiang Province (no. 2022133).
References
- Y. Li, X. Zhang, H. Huang, S. V. Kershaw and A. L. Rogach, Mater. Today, 2020, 32, 204–221 CrossRef CAS
.
- S. Liang, M. Zhang, G. M. Biesold, W. Choi, Y. He, Z. Li, D. Shen and Z. Lin, Adv. Mater., 2021, 33, 2005888 CrossRef CAS
.
- Y. Wei, Z. Cheng and J. Lin, Chem. Soc. Rev., 2019, 48, 310–350 RSC
.
- Q. Zhong, M. Cao, Y. Xu, P. Li, Y. Zhang, H. Hu, D. Yang, Y. Xu, L. Wang, Y. Li, X. Zhang and Q. Zhang, Nano Lett., 2019, 19, 4151–4157 CrossRef CAS
.
- M. Zhao, Q. Zhang and Z. Xia, Mater. Today, 2020, 40, 246–265 CrossRef CAS
.
- H. Li, Y. Gao, X. Ying, Y. Feng, M. Zhu, D. Zhang, G. Lu, R. Tao, Q. Cai, H. He, X. Dai, Z. Ye and J. Huang, Chem. Eng. J., 2023, 454, 140038 CrossRef CAS
.
- X. K. Liu, W. Xu, S. Bai, Y. Jin, J. Wang, R. H. Friend and F. Gao, Nat. Mater., 2021, 20, 10–21 CrossRef CAS PubMed
.
- Y. F. Lan, J. S. Yao, J. N. Yang, Y. H. Song, X. C. Ru, Q. Zhang, L. Z. Feng, T. Chen, K. H. Song and H. B. Yao, Nano Lett., 2021, 21, 8756–8763 CrossRef CAS PubMed
.
- J. Yuan, A. Hazarika, Q. Zhao, X. Ling, T. Moot, W. Ma and J. M. Luther, Joule, 2020, 4, 1160–1185 CrossRef CAS
.
- X. Zhang, H. Huang, X. Ling, J. Sun, X. Jiang, Y. Wang, D. Xue, L. Huang, L. Chi, J. Yuan and W. Ma, Adv. Mater., 2022, 34, 2105977 CrossRef CAS
.
- J. H. Heo, D. H. Shin, J. K. Park, D. H. Kim, S. J. Lee and S. H. Im, Adv. Mater., 2018, 30, 1801743 CrossRef PubMed
.
- S. Cho, S. Kim, J. Kim, Y. Jo, I. Ryu, S. Hong, J. J. Lee, S. Cha, E. B. Nam, S. U. Lee, S. K. Noh, H. Kim, J. Kwak and H. Im, Light: Sci. Appl., 2020, 9, 156 CrossRef
.
- M. N. An, S. Park, R. Brescia, M. Lutfullin, L. Sinatra, O. M. Bakr, L. De Trizio and L. Manna, ACS Energy Lett., 2021, 6, 900–907 CrossRef CAS
.
- L. Chen, M. He, W. Gong, P. Hu, S. Yuan, A. Chen, L. Wei, Q. Zhang and Q. Li, Nano Energy, 2023, 108, 108235 CrossRef CAS
.
- S. Zhang, Z. Liu, W. Zhang, Z. Jiang, W. Chen, R. Chen, Y. Huang, Z. Yang, Y. Zhang, L. Han and W. Chen, Adv. Energy Mater., 2020, 10, 2001610 CrossRef CAS
.
- J. I. Kim, Q. Zeng, S. Park, H. Lee, J. Park, T. Kim and T. W. Lee, Adv. Mater., 2023, 2209784 CrossRef CAS
.
- X. Wang, Z. Bao, Y.-C. Chang and R.-S. Liu, ACS Energy Lett., 2020, 5, 3374–3396 CrossRef CAS
.
- W. J. Mir, A. Alamoudi, J. Yin, K. E. Yorov, P. Maity, R. Naphade, B. Shao, J. Wang, M. N. Lintangpradipto, S. Nematulloev, A. H. Emwas, A. Genovese, O. F. Mohammed and O. M. Bakr, J. Am. Chem. Soc., 2022, 144, 13302–13310 CrossRef CAS
.
- D. N. Dirin, B. M. Benin, S. Yakunin, F. Krumeich, G. Raino, R. Frison and M. V. Kovalenko, ACS Nano, 2019, 13, 11642–11652 CrossRef CAS PubMed
.
- K. Xing, S. Cao, X. Yuan, R. Zeng, H. Li, B. Zou and J. Zhao, Phys. Chem. Chem. Phys., 2021, 23, 17113–17128 RSC
.
- A. Loiudice, S. Saris, E. Oveisi, D. T. L. Alexander and R. Buonsanti, Angew. Chem., Int. Ed., 2017, 56, 10696–10701 CrossRef CAS PubMed
.
- Q. Zhang, B. Wang, W. Zheng, L. Kong, Q. Wan, C. Zhang, Z. Li, X. Cao, M. Liu and L. Li, Nat. Commun., 2020, 11, 31 CrossRef CAS
.
- H. Dong, S. Tian, X. Sun, I. S. Mukhin, R. M. Islamova, G. Li and J. Tian, Adv. Opt. Mater., 2023, 11, 2300309 CrossRef CAS
.
- S. Tian, X. Zhou, C. Bi, X. Sun, M. Zhang, S. Yang and J. Tian, Adv. Opt. Mater., 2022, 10, 2200751 CrossRef CAS
.
- B. Li, Y. Zhang, Y. Xu and Z. Xia, J. Mater. Chem. C, 2021, 9, 12118–12123 RSC
.
- S. X. Li, Y. Pan, W. M. Wang and Y. Li, Chem. Eng. J., 2022, 434, 134593 CrossRef CAS
.
- S. Liao, Z. Yang, J. Lin, S. Wang, J. Zhu, S. Chen, F. Huang, Y. Zheng and D. Chen, Adv. Funct. Mater., 2023, 33, 2210558 CrossRef CAS
.
- S. Chen, J. Lin, J. Huang, T. Pang, Q. Ye, Y. Zheng, X. Li, Y. Yu, B. Zhuang and D. Chen, Adv. Funct. Mater., 2024, 34, 2309293 CrossRef CAS
.
- S. Chen, J. Lin, S. Zheng, Y. Zheng and D. Chen, Adv. Funct. Mater., 2023, 33, 2213442 CrossRef CAS
.
- J. Lin, Y. Lu, X. Li, F. Huang, C. Yang, M. Liu, N. Jiang and D. Chen, ACS Energy Lett., 2021, 6, 519–528 CrossRef CAS
.
- Y. Zhang, L. Han, B. Li and Y. Xu, Chem. Eng. J., 2022, 437, 135290 CrossRef CAS
.
- C. Fan, H. Liu, J. Zhou, X. Dai, H. He and Z. Ye, InfoMat, 2023, 5, 12417 CrossRef
.
- M. R. Kar, S. Ray, B. K. Patra and S. Bhaumik, Mater. Today Chem., 2021, 20, 100424 CrossRef CAS
.
- J. Li, D. Zhou, Y. Liu, Y. Chen, J. Chen, Y. Yang, Y. Gao and J. Qiu, ACS Appl. Mater. Interfaces, 2023, 15, 22219–22230 CrossRef CAS
.
- T. Xuan, S. Guo, W. Bai, T. Zhou, L. Wang and R.-J. Xie, Nano Energy, 2022, 95, 107003 CrossRef CAS
.
- S. Liu, Z. Guo, X. Wu, X. Liu, Z. Huang, L. Li, J. Zhang, H. Zhou, L. D. Sun and C. H. Yan, Adv. Mater., 2023, 35, 2208078 CrossRef CAS
.
- B. Guo, R. Lai, S. Jiang, L. Zhou, Z. Ren, Y. Lian, P. Li, X. Cao, S. Xing, Y. Wang, W. Li, C. Zou, M. Chen, Z. Hong, C. Li, B. Zhao and D. Di, Nat. Photonics, 2022, 16, 637–643 CrossRef CAS
.
- J. Zhang, X. Xing, D. Qian, A. Wang, L. Gu, Z. Kuang, J. Wang, H. Zhang, K. Wen, W. Xu, M. Niu, X. Du, L. Yuan, C. Cao, Y. Cao, L. Zhu, N. Wang, C. Yi, W. Huang and J. Wang, Adv. Funct. Mater., 2022, 32, 2111578 CrossRef CAS
.
- Y. Huang, F. Li, L. Qiu, F. Lin, Z. Lai, S. Wang, L. Lin, Y. Zhu, Y. Wang, Y. Jiang and X. Chen, ACS Appl. Mater. Interfaces, 2019, 11, 26384–26391 CrossRef CAS
.
- G. H. Ahmed, J. Yin, O. M. Bakr and O. F. Mohammed, ACS Energy Lett., 2021, 6, 1340–1357 CrossRef CAS
.
- S. W. Eaton, M. Lai, N. A. Gibson, A. B. Wong, L. Dou, J. Ma, L. W. Wang, S. R. Leone and P. Yang, Proc. Natl. Acad. Sci. U. S. A., 2016, 113, 1993–1998 CrossRef CAS PubMed
.
- F. Cao, D. Yu, W. Ma, X. Xu, B. Cai, Y. M. Yang, S. Liu, L. He, Y. Ke, S. Lan, K. L. Choy and H. Zeng, ACS Nano, 2020, 14, 5183–5193 CrossRef CAS PubMed
.
- A. Jana, S. Cho, S. A. Patil, A. Meena, Y. Jo, V. G. Sree, Y. Park, H. Kim, H. Im and R. A. Taylor, Mater. Today, 2022, 55, 110–136 CrossRef CAS
.
- F. Zhou, Z. Li, W. Lan, Q. Wang, L. Ding and Z. Jin, Small Methods, 2020, 4, 2000506 CrossRef CAS
.
- H. Wei, Y. Fang, P. Mulligan, W. Chuirazzi, H.-H. Fang, C. Wang, B. R. Ecker, Y. Gao, M. A. Loi, L. Cao and J. Huang, Nat. Photonics, 2016, 10, 333–339 CrossRef CAS
.
- W. Ma, T. Jiang, Z. Yang, H. Zhang, Y. Su, Z. Chen, X. Chen, Y. Ma, W. Zhu, X. Yu, H. Zhu, J. Qiu, X. Liu, X. Xu and Y. M. Yang, Adv. Sci., 2021, 8, 2003728 CrossRef CAS PubMed
.
- H. Guo, Y. Zhu, Q. Zhao, Q. Jiang, Y. Ma, J. Chen, L. Song, Z. Shi and C. Xu, Sci. China Mater., 2023, 66, 2004–2012 CrossRef CAS
.
- Z. Wang, Y. Wei, C. Liu, Y. Liu and M. Hong, Laser Photonics Rev., 2023, 17, 2200851 CrossRef CAS
.
|
This journal is © The Royal Society of Chemistry 2024 |
Click here to see how this site uses Cookies. View our privacy policy here.