DOI:
10.1039/D3TC04748B
(Paper)
J. Mater. Chem. C, 2024,
12, 2370-2378
Tunable mechanochromic luminescence of benzofuran-fused pyrazine: effects of alkyl chain length and branching pattern†
Received
23rd December 2023
, Accepted 26th January 2024
First published on 27th January 2024
Abstract
A series of bis(benzofuro)[2,3-b:2′,3′-e]pyrazines (BBFPz) with various alkyl chains of different length have been synthesized, and the effects of chain length on their mechanochromic luminescent properties were investigated. Some derivatives gave two polymorphs depending on the recrystallization conditions. Although the luminescent properties of the derivatives were almost the same in the solution state, distinct luminescence depending on their alkyl chains was observed in the crystalline state. The obtained crystals could be categorized into four packing motifs (Type A–D) with unique mechanochromic luminescent properties. Type D crystals with linear alkyl groups did not show mechanochromic luminescent characters. The luminescent color of Linear-Me slightly and reversibly changed upon grinding. Linear-Et (Form 1) and Linear-sBu, which belong to Type B and C, respectively, exhibited mechanochromic luminescent properties with rapid self-recovery character. The other crystals showed mechanochromic luminescent properties with relatively slow self-recovery rates. The present work demonstrates a quite limited example of covering both on/off mechanochromic luminescence (MCL) and self-recovery characters with a specific luminophore skeleton by changing the alkyl chains.
Introduction
Mechanochromic luminescent materials, which show a color change in response to external mechanical stimuli, have attracted great interest due to their impressive applications in security printing, memory devices, etc.1–3 The development of such smart stimuli-responsive materials is now one of the hot research topics in the field of material science.4–6 Mechanochromic luminescent active organic small molecules generally exhibit red-shifted emission upon grinding because of the rapid phase transition from the crystalline state to the amorphous state.7–12 Their original crystalline packing structures are usually recovered by heating or solvent vapor fuming. Some compounds are reported to undergo such recovery processes even by just standing under ambient conditions, which is called “self-recovery” character.13–17 Since a number of mechanochromic luminescent active compounds have been reported over the past two decades, considerable efforts have recently been devoted to elucidating the correlation between molecular/packing structures and their optical properties.18–20
The concept of side chain engineering has been recognized as a promising method for controlling physical and optical properties of functional materials.21–28 Alkyl side chains introduced to the periphery of the core luminophore scaffold significantly change their packing structures. Besides, alkyl groups may improve the solubility of the rigid molecules, which is quite beneficial to allow low-energy and high-volume production as well as wet processes for device fabrication.29–31 The first example of the alkyl length effect on the mechanochromic luminescent properties was reported by Weder and coworkers in 2008 for oligo(p-phenylene vinylene) derivatives.32 They found that the introduction of long alkoxy groups to the skeleton induced mechanochromic luminescence (MCL). In 2011, Fraser found that a series of difluoroboron diketonates with different alkoxy chain lengths could control the emission shifts, and the recovery time after grinding increased with the elongation of the chain length (Scheme 1).33 Thomas designed and synthesized dialkylamine-substituted phenylene-ethynylene conjugation systems, and found that alkyl-chain length affected on/off switching of MCL.34 Afterward, Zhang and Tang investigated the alkyl chain effect on the self-recovery behavior of Au(I) isocyanide complexes.35 In contrast to Fraser's report, longer alkyl chains could accelerate the self-recovery speed. There are additional examples of side chain engineering for controlling on/off switching of MCL or the self-recovery rate.36–50
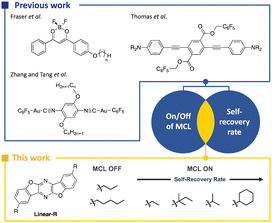 |
| Scheme 1 Organic mechanochromic luminescent materials with various alkyl chains. | |
Recently, we have synthesized several bis(benzofuro)[2,3-b:2′,3′-e]pyrazine (BBFPz) derivatives (Fig. 1, Linear-R).51Linear-H (R = H) did not have mechanochromic luminescent properties because of its high planarity and rigid structure. On the other hand, tert-butyl substituted Linear-tBu (R = tBu) formed two different crystal polymorphs and exhibited self-recovering mechanochromic luminescent properties. Moreover, their bent-type isomers, bis(benzofuro)[2,3-b:3′,2′-e]pyrazines (Bent-R), were also synthesized, and parent unsubstituted bis(benzofuro)[2,3-b:3′,2′-e]pyrazine (Bent-H: R = H) was found to be a good phosphorescent emitter in a dispersed state.52,53
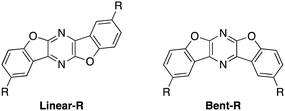 |
| Fig. 1 Structures of linear- and bent-type bisbenzofuropyrazines. | |
In this paper, we report detailed physical and optical properties of a series of newly synthesized alkyl-substituted bis(benzofuro)[2,3-b:2′,3′-e]pyrazines (Linear-R). Some compounds formed crystalline polymorphs under different recrystallization conditions. The obtained crystals could be categorized into four packing motifs with unique mechanochromic luminescent characters. Almost all reported examples of alkyl-chain-controlled mechanochromic luminescent active compounds have flexible and free-rotating single bonds within their core skeletons.54–56 In sharp contrast, Linear-R has a rigid and planar heterocycle luminophore, but the alkyl substituents control the stacking structure to make it sensitive to external stimuli. As a result, the present work demonstrates a quite limited example of covering both on/off switching of MCL and self-recovery characters with a specific luminophore skeleton.
Results and discussion
Synthesis
The synthetic route to the bisbenzofuropyrazine derivatives is shown in Scheme 2. Two-fold nucleophilic substitution of 2,5-dibromopyrazine with o-bromophenols in basic DMSO solution gave the corresponding 2,5-diphenoxypyrazines, which were then subjected to intramolecular direct arylation conditions using a PdCl2(PPh3)2 catalyst to afford the target alkyl-substituted bisbenzofuropyrazines (Linear-R).
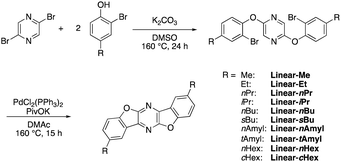 |
| Scheme 2 Synthetic routes to linear-type bisbenzofuropyrazines. | |
Optical properties (solution)
Photophysical properties of Linear-R were next examined by UV/Vis absorption and photoluminescence (PL) spectroscopy in diluted CHCl3 solutions. All compounds exhibited two characteristic absorption bands with absorption maxima around 260 nm and 380 nm, and emitted blue-violet fluorescence with relatively high luminescence quantum yields (ΦFL) of 0.55–0.93 (Table 1). As a representative example, the absorption and fluorescence spectra of Linear-Me are shown in Fig. 2. The photophysical properties of Linear-R in the dispersed solution state were less dependent on the alkyl groups.
Table 1 Summary of absorption and emission spectra
Compound (Linear-) |
λ
Abs (nm) |
ε
max (104 M−1 cm−1) |
λ
Fl (nm) |
Φ
Fl (−) |
Me
|
379 |
5.25 |
405 |
0.85 |
Et
|
379 |
4.76 |
406 |
0.77 |
nPr
|
379 |
3.97 |
406 |
0.72 |
iPr
|
379 |
4.44 |
407 |
0.87 |
nBu
|
379 |
3.99 |
406 |
0.81 |
sBu
|
379 |
5.92 |
407 |
0.85 |
nAmyl
|
380 |
4.56 |
407 |
0.82 |
tAmyl
|
380 |
5.86 |
408 |
0.89 |
nHex
|
380 |
3.49 |
406 |
0.76 |
cHex
|
380 |
4.35 |
408 |
0.55 |
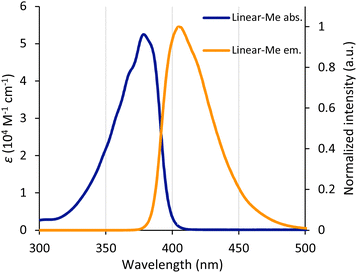 |
| Fig. 2 Absorption and emission spectra of Linear-Me in CHCl3 solution. The concentration was maintained at 1 × 10−5 M. | |
Crystallization
The synthesized Linear-R was reasonably soluble in organic solvents and readily recrystallized, whereas the previously reported Linear-H was almost insoluble.51Linear-Et and Linear-cHex crystallized as two polymorphs (Forms 1 and 2) depending on the recrystallization conditions, whereas the other eight compounds did not give polymorphs even after many trials with various solvent/temperature combinations. Linear-Et afforded colorless prismatic crystals by slow evaporation from a CHCl3 solution (Form 1) and colorless needle-like crystals by evaporation from a THF solution (Form 2). Variable temperature powder X-ray diffraction (VT-PXRD) measurements revealed that the Linear-Et (Form 2) underwent a phase transition to Form 1 around 140 °C, whereas Form 1 exhibited no transition below its melting point (Fig. S1, ESI†). This indicates that Form 1 is thermodynamically more stable than Form 2, but the difference in packing stability between Form 1 and Form 2 might be small because DSC measurements of both forms did not show clear peaks around the transition temperature (Fig. S2, ESI†). Linear-cHex crystallized as colorless needles by slow evaporation from the CH2Cl2 solution (Form 1) and as colorless blocks by slow evaporation from the EtOAc solution (Form 2). VT-PXRD measurements revealed that Form 2 underwent the first phase transition to Form 1 around 190 °C and the second transition occurred just below the melting point (Fig. S1, ESI†). However, similar to Linear-Et, DSC measurements of Linear-cHex exhibited no clear signal around the transition temperatures (Fig. S2, ESI†).
Crystal structures
The packing arrangements of the Linear-R compounds were evaluated by single crystal X-ray crystallographic analyses. All crystal structures showed a face-to-face slipped columnar structure and can be categorized into four packing motifs (Type A–D) according to the orientation of the alkyl substituents (Fig. 3 and 4). The classification was based on the mechanochromic luminescent properties, which were consistent with the orientation of the alkyl chains rather than the orientation of the aromatic scaffolds.
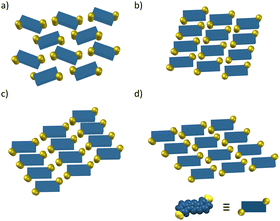 |
| Fig. 3 Stylized models of molecular packing along the columnar structure in the crystalline state: (a) Type A, (b) Type B, (c) Type C, and (d) Type D. | |
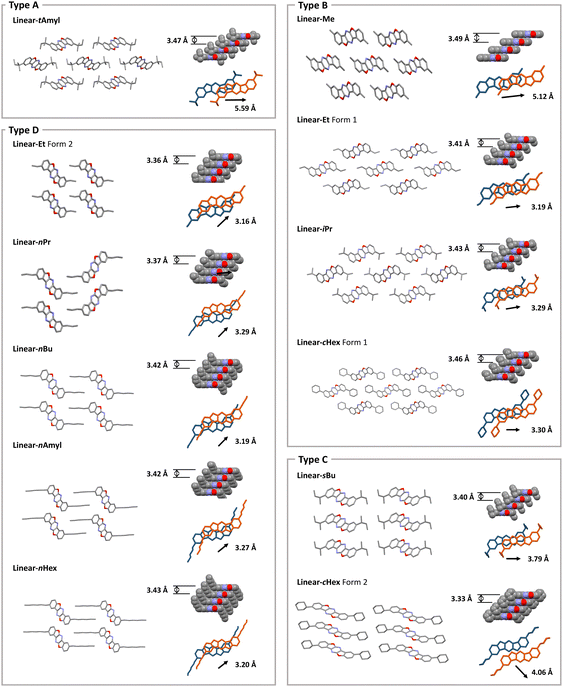 |
| Fig. 4 Categorized crystal structures. Hydrogen atoms are omitted for clarity. The double-headed arrows denote the interplanar distance between the mother skeletons in the columnar structure. The single-headed arrows denote the slippage distance of two adjacent mother skeletons in the columnar structure. Grey: carbon; red: oxygen; purple: nitrogen. | |
Linear-tAmyl belongs to the Type A group (Fig. 3a and 4). The longest slippage distance of 5.59 Å was observed probably because the sterically demanding tert-amyl substituents interrupted face-to-face stacking of the aromatic moieties. Linear-Me, Linear-Et (Form 1), Linear-iPr, and Linear-cHex (Form 1) belong to the Type B group (Fig. 3b and 4). Here, BBFPz skeletons form intercolumnar contact at the short molecular axis, and the alkyl groups occupy spaces between the π scaffolds along the long axis. The slippage distances were relatively short ranging from 3.19 to 3.30 Å, except for Linear-Me crystals (5.12 Å). Because the Linear-Me crystal has compact methyl groups compared to the other crystals, relatively weak dispersion energy gave large slippage distance. Linear-sBu and Linear-cHex (Form 2) belong to the Type C group (Fig. 3c and 4), where BBFPz skeletons form intercolumnar contact at the long molecular axis. The alkyl groups were in contact at the short molecular axis. These crystals gave short π–π stacking distances (3.40 Å and 3.33 Å), whereas the bulky alkyl groups prevented further approach towards the mother skeleton to give a slightly larger slippage distance (3.79 Å and 4.06 Å). Linear-Et (Form 2), Linear-nPr, Linear-nBu, Linear-nAmyl, and Linear-nHex belong to the Type D packing motif (Fig. 3d and 4). BBFPz skeletons form intercolumnar contact at the long molecular axis, and the alkyl substituents form zipper-like layer structures. The short stacking distance (3.36–3.43 Å) and relatively small slippage distances (3.16–3.29 Å) would reflect the strong π–π interaction within these crystals.
Optical properties (crystal)
The photophysical properties of Linear-R in the crystalline state were found to be different from those in solution (Fig. 5 and Table 2). Linear-tAmyl (Type A) exhibited strong blue emission with the emission maximum wavelength (λem_max) at 438 nm (Fig. 5i). The insertion of bulky tert-amyl substituents among the columnar structures prevented the mother skeletons from strong contact, which gave monomeric blue emission. The fluorescence lifetime of 2.86 ns was also indicative of monomeric emission. The quantum efficiency of the crystal was 0.60. The photophysical properties of Type B crystals depend on their alkyl substituents. The luminescence spectra of Linear-Me and Linear-cHex (Form 1) crystals were slightly red-shifted to 448 and 443 nm, respectively, compared with the crystal of Linear-tAmyl (Fig. 5a and k). The fluorescence lifetimes of the Linear-Me and Linear-cHex (Form 1) crystals were 2.68 and 10.4 ns, respectively. Additionally, the excitation spectra of the crystals were similar to that of Linear-tAmyl, indicating that luminescence from Linear-Me is monomeric emission and that from Linear-cHex (Form 1) is excimer emission.57–61Linear-Et (Form 1) and Linear-iPr showed more red-shifted light-blue emission with the emission maximum wavelength (λem_max) at 467 and 468 nm with a lifetime (τem) of 8.51 and 12.6 ns, respectively (Fig. 5b and e). Additionally, the excitation spectra of these crystals were red-shifted compared to that of monomerically emissive Linear-tAmyl. The observed red-shifted emission and excitation spectra may be caused by strong ground-state interaction due to the close packing structure. The quantum efficiencies of Linear-Me, Linear-Et (Form 1), Linear-iPr, and Linear-cHex (Form 1) were 0.28, 0.41, 0.21, and 0.52, respectively. These results indicate that π–π interaction among the luminophores decreased the luminescence.62 Introducing alkyl groups changed the mode of π–π stacking and tuned the luminescent properties. The photophysical properties of Type C crystals were highly dependent on the alkyl side chain. The fluorescence spectrum of the Linear-cHex (Form 2) crystals was sharp and slightly red-shifted (λem_max = 442 nm, τem = 12.0 ns; Fig. 5l). Its excitation spectra were almost the same as that of Linear-tAmyl, indicating that excimer emission occurs. The Linear-cHex (Form 2) crystals had a close stacking distance, but the mother skeletons were sufficiently separated from each other by bulky cyclohexyl substituents. Thus, its emission was originated not from a ground-state dimer but from an excimer. On the other hand, the crystals of Linear-sBu showed more red-shifted and broadened light-blue emission (λem_max = 462 nm, τem = 26.2 ns) (Fig. 5g). The quantum efficiencies of Linear-sBu and Linear-cHex (Form 2) were 0.47 and 0.31, respectively. Crystals of Type D (Linear-Et Form 2, Linear-nPr, Linear-nBu, Linear-nAmyl, Linear-nHex) showed similar emission spectra, which were broadened and red-shifted (λem_max: 469–480 nm, τem = 12.1–23.0 ns) (Fig. 5c, d, f, h and j). The quantum efficiencies of these crystals ranged from 0.29 to 0.67. In the Type B and Type D crystals, the fluorescence quantum yields were relatively high as the free volumes in their unit cell decreased (Fig. S3 and Table S1, ESI†). This is probably because the small free volumes minimize molecular vibration to suppress nonradiative decay.63,64 Apparently, the introduction of various kinds of alkyl substituents to the benzofuropyrazine mother skeleton is a quite important method to control polymorph and luminescence properties.
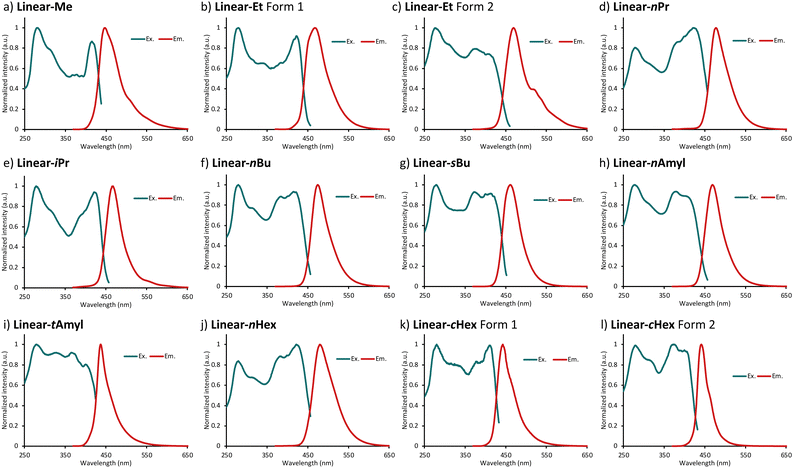 |
| Fig. 5 Excitation and emission spectra of formed crystals. (a) Linear-Me, (b) Linear-Et Form 1, (c) Linear-Et Form 2, (d) Linear-nPr, (e) Linear-iPr, (f) Linear-nBu, (g) Linear-sBu, (h) Linear-nAmyl, (i) Linear-tAmyl, (j) Linear-nHex, (k) Linear-cHex Form 1, (l) Linear-cHex Form 2. | |
Table 2 Summary of photophysical data of Linear-R
Compound (Linear-) |
λ
Fl (nm) |
Φ
Fl (−) |
τ
Fl
(ns) |
λ
Fl (ground) (nm) |
λ
Fl (ground-heat) (nm) |
λ
Fl (ground-fuming) (nm) |
Average emission lifetime.
|
Me
|
448 |
0.28 |
3.07 |
468 |
469 |
467 |
Et Form 1
|
467 |
0.41 |
8.51 |
445 |
— |
— |
Et Form 2
|
470 |
0.67 |
20.1 |
471 |
— |
— |
nPr
|
478 |
0.35 |
14.5 |
475 |
— |
— |
iPr
|
468 |
0.21 |
12.6 |
518 |
469 |
467 |
nBu
|
474 |
0.49 |
23.0 |
471 |
— |
— |
sBu
|
462 |
0.47 |
26.2 |
448 |
— |
— |
nAmyl
|
469 |
0.44 |
17.9 |
472 |
— |
— |
tAmyl
|
438 |
0.60 |
2.86 |
509 |
421 |
417 |
nHex
|
480 |
0.29 |
12.1 |
475 |
— |
— |
cHex Form 1
|
443 |
0.52 |
10.4 |
516 |
447 |
435 |
cHex Form 2
|
442 |
0.31 |
12.0 |
516 |
434 |
433 |
Mechanochromic properties
The crystals of Linear-R showed diverse MCL depending on the alkyl substituents and packing structures (Fig. 6 and Table 2). Crystals categorized into the Type D packing structure (Linear-Et (Form 2), Linear-nPr, Linear-nBu, Linear-nAmyl, and Linear-nHex) did not show considerable spectral changes upon grinding (Fig. S4, ESI†). On the other hand, for the Linear-tAmyl crystals, a relatively long-lived green-emissive state was observed after grinding (Fig. 6c). A new fluorescence band emerged around 509 nm, whereas the original peak at 438 nm decreased and slightly blue-shifted (427 nm). We also investigated the IR spectra of Linear-iPr as a representative example before and after grinding, but did not identify any clear difference (Fig. S5, ESI†). Thus, the excitation spectra were measured to clarify the changes of interactions. Excitation spectra measured at 509 and 427 nm were identical to each other, indicating that these two emissions were derived from the same excited species. We conducted powder X-ray diffraction (PXRD) analyses for Linear-tAmyl before/after grinding (Fig. S7, ESI†). Since the characteristic signals almost disappeared after grinding, it is conclusive that the green-emissive state was almost an amorphous structure (Fig. 7a and b). A similar trend was observed in Linear-cHex (Forms 1 and 2) although their spectral changes were somewhat smaller compared to those of Linear-tAmyl (Fig. 6d and e). Linear-Me and Linear-iPr exhibited much smaller spectral changes (Fig. 6a and b). According to PXRD measurements, Linear-cHex (Forms 1 and 2), Linear-Me, and Linear-iPr were also in amorphous systems after grinding (Fig. S7, ESI†). The fluorescence peak of the Linear-Et (Form 1) crystals appeared to be blue shifted upon grinding. However, the self-recovery process (vide infra) was too fast to capture red-shifted peaks during grinding (Fig. S4a, S6 and Video 1, ESI†). Linear-sBu crystals exhibited a similar behavior to Linear-Et (Form 1; Fig. S4 and Video 2, ESI†).
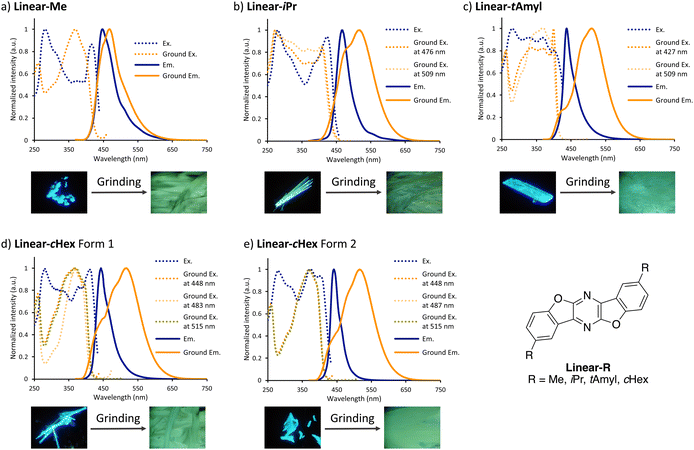 |
| Fig. 6 Excitation spectra (Ex.) and emission spectra (Em.) of the formed crystals and ground powders of (a) Linear-Me, (b) Linear-iPr, (c) Linear-tAmyl, (d) Linear-cHex Form 1, and (e) Linear-cHex Form 2. | |
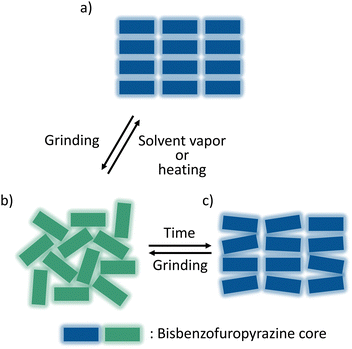 |
| Fig. 7 Illustration of molecular arrangement models after various stimuli. (a) Pristine powder, (b) powder after grinding, low-ordered amorphous, and (c) ground powder after self-recovery. | |
Relationship between MCL and crystal structure
The BBFPz scaffold within Linear-tAmyl crystals (Type A) was surrounded by flexible tert-amyl groups with considerably large free volume (Fig. 3a and 4). External mechanical stimuli would effectively disturb the packing structure to form suitable sites for excimer emission. As a result, distinct red-shifted emission was observed after grinding (Fig. 6c). As for Linear-cHex crystals (Forms 1 and 2), the BBFPz skeleton shows considerable short intermolecular contact (Fig. 3b, c and 4). This interaction would make these crystals less responsive to external mechanical stimuli, thereby showing smaller changes in their spectral shapes upon grinding (Fig. 6d and e). This mechanism was also supported by calculation of intermolecular potentials in the crystalline state (Fig. S8, ESI†).65,66Linear-Me and Linear-iPr also exhibited MCL but with smaller color changes. Since Linear-Me and Linear-iPr have sterically much less demanding alkyl groups, their fluorescence spectra did not significantly change after grinding (Fig. 6a and b). A similar trend was observed in our previous work.51 In contrast, the packing system of Type D crystals, Linear-Et (Form 2), Linear-nPr, Linear-nBu, Linear-nAmyl, and Linear-nHex, would be rather rigid because their columnar structures are anchored by the interaction between relatively long linear alkyl substituents, thereby not showing characteristic mechanochromism (Fig. 3d and 4). Theoretical calculations show that the intermolecular interaction energies between stacked molecules increase on increasing the number of carbons in the alkyl groups, which is also known as the “fastener effect” (Table S2, ESI†).67
Self-recovery of fluorescence
We then investigated the self-recovery behavior of the aforementioned five crystals showing MCL: Linear-Me, Linear-iPr, Linear-tAmyl, and Linear-cHex (Forms 1 and 2). Linear-Et (Form 1) and Linear-sBu were excluded herein because their recovery processes were too rapid to trace. The rapid self-recovery character might be derived from their rigid packing systems and relatively large free volume around the columnar structures. MCL of Linear-Me can be regarded as irreversible since its fluorescence spectrum remained unchanged after 24 h (Fig. S9, ESI†). The other four compounds recovered their original blue color luminescence by just standing under ambient conditions. Linear-tAmyl showed the most remarkable recovery profile: the monomeric emission at around 410 nm increased, whereas the excimer emission at around 510 nm almost disappeared even over 24 h (Fig. 8b). Interestingly, the PXRD patterns of the ground Linear-tAmyl did not change after 24 h (Fig. S10, ESI†). We assume that the molecular arrangement of Linear-tAmyl becomes low-ordered amorphous upon grinding, in which some luminophores are oriented closely to form excimers. It then converts to high-ordered amorphous, where luminophores are isolated from each other by the alkyl spacer (Fig. 7c). Linear-iPr and Linear-cHex (Forms 1 and 2) also exhibited self-recovery character at room temperature but much slower than Linear-tAmyl (Fig. 8a, c and d). This is probably due to the smaller size of free volume around the aromatic scaffold within their packing structures to recover the original structure, thus requiring prolonged periods for the completion of recovery (Fig. S3, ESI†). Considering the absence of self-recovery characters in Linear-R with relatively compact substituents such as H51 or Me, and linear alkyl groups, it is suggested that the presence of relatively bulky substituents with branched alkyl chains plays a crucial role in exhibiting self-recovering characters. The overall decrease of emission intensity during the experiments can be attributed to the degradation of these compounds by the excitation light. The recovery process was significantly accelerated by heating or exposure to CHCl3 vapor, and their original blue-emissive states were restored within 1 minute (Fig. S11, ESI†). After heating or fuming the powders with CHCl3 vapor, the PXRD peaks of the sample reappear, which suggests the recovery of the ordered structure (Fig. S12 and S13, ESI†). The PXRD pattern of the recovered states matched well with that of the initial powder, indicating that heating or vapor fuming accelerates the recovery process to the original crystalline state. As mentioned above, this process would be dependent on the nature of the side chains rather than the stress size and direction of mechanical forces. In addition, the luminescence color change of Linear-iPr, Linear-tAmyl, and Linear-cHex (Forms 1 and 2) by grinding and CHCl3 fuming could be repeated at least five times (Fig. S14, ESI†).
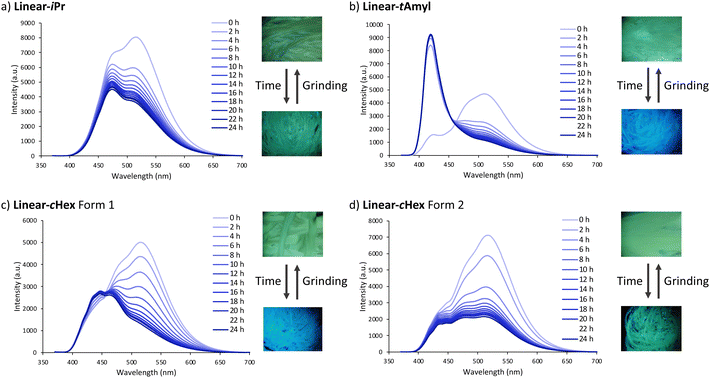 |
| Fig. 8 Change over time of luminescence after mechanical stimuli. (a) Linear-iPr, (b) Linear-tAmyl, (c) Form 1 of Linear-cHex, and (d) Form 2 of Linear-cHex. | |
Conclusions
A series of bis(benzofuro)[2,3-b:2′,3′-e]pyrazines modified with different alkyl chains (Linear-R) were synthesized in this study. The obtained compounds exhibited similar luminescence properties in solution, regardless of the alkyl substituents. However, they showed distinct luminescent properties in the crystalline state. Linear-Et and Linear-cHex formed two crystalline polymorphs (Forms 1 and 2) under different recrystallization conditions, while other compounds gave a single crystal under any tested conditions. Their mechanochromic luminescent properties are highly dependent on the alkyl side chains. Derivatives with long linear alkyl chains did not show MCL. On the other hand, other derivatives bearing smaller or branched alkyl groups exhibited MCL. The peaks emerging at longer wavelength in the ground powders stem from excimer emissions. Mechanical force causes a crystalline-to-amorphous transition as shown by PXRD measurement. The ground powder with large free volume around the mother skeleton in the columnar structures showed relatively short recovery times, and the ground powder with small free volume gave relatively long recovery times. Overall, this work demonstrates how the alkyl chains with different length and size affect the mechanochromic luminescent properties in the crystalline state. Additionally, this is a limited example of controlling the on/off switching of MCL and self-recovery rate with the same mother skeleton by changing the alkyl chains. These unique systems could be promising candidates for application as light-emitting devices, and the findings obtained in the present study will guide the rational design of additional mechanochromic luminescent materials.
Author contributions
S. N. performed all experiments. K. O. supported VT-PXRD measurement. K. H., N. T., and M. M supervised the project. Y. N. supported X-ray analysis. S. N., Y. N., K. H., and N. T. prepared the manuscript. All the authors discussed the results and commented on the manuscript.
Conflicts of interest
There are no conflicts to declare.
Acknowledgements
This work was supported by JSPS KAKENHI Grant No. JP 22H02077 (Grant-in-Aid for Scientific Research (B), to K. H.) and JP 17H06092 (Grant-in-Aid for Specially Promoted Research, to M. M.), and by JST FOREST Program, Grant Number JPMJFR211X to K. H. as well as by JST SPRING, Grant Number JPMJSP2138 to S. N.
Notes and references
- X. Liu, M. Li, M. Liu, Q. Yang and Y. Chen, Chem. – Eur. J., 2018, 24, 13197–13204 CrossRef CAS PubMed.
- Y. Wang, X. Tan, Y.-M. Zhang, S. Zhu, I. Zhang, B. Yu, K. Wang, B. Yang, M. Li, B. Zou and S. X.-A. Zhang, J. Am. Chem. Soc., 2015, 137, 931–939 CrossRef CAS PubMed.
- A. Pucci and G. Ruggeri, J. Mater. Chem., 2011, 21, 8282–8291 RSC.
- F. Nie and D. Yan, Angew. Chem., Int. Ed., 2023, 62, e202302751 CrossRef CAS PubMed.
- B. Zhou and D. Yan, Adv. Funct. Mater., 2023, 33, 2300735 CrossRef CAS.
- Y. Yang, X. Yang, X. Fang, K.-Z. Wang and D. Yan, Adv. Sci., 2018, 5, 1801187 CrossRef PubMed.
- Z. Chi, X. Zhang, B. Xu, C. Ma, Y. Zhang, S. Liu and J. Xu, Chem. Soc. Rev., 2012, 41, 3878–3896 RSC.
- Z. Ma, Z. Wang, M. Teng, Z. Xu and X. Jia, ChemPhysChem, 2015, 16, 1811–1828 CrossRef CAS PubMed.
- Y. Sagara, S. Yamane, M. Mitani, C. Weder and T. Kato, Adv. Mater., 2016, 28, 1073–1095 CrossRef CAS PubMed.
- C. Wang and Z. Li, Mater. Chem. Front., 2017, 1, 2174–2194 RSC.
- Z. Yang, Z. Chi, Z. Mao, Y. Zhang, S. Liu, J. Zhao, M. P. Aldred and Z. Chi, Mater. Chem. Front., 2018, 2, 861–890 RSC.
- S. Ito, Chem. Lett., 2021, 50, 649–660 CrossRef CAS.
- L. Bu, M. Sun, D. Zhang, W. Liu, Y. Wang, M. Zheng, S. Xue and W. Yang, J. Mater. Chem. C, 2013, 1, 2028–2035 RSC.
- G. R. Krishna, M. S. Kiran, C. L. Fraser, U. Ramamurty and C. M. Reddy, Adv. Funct. Mater., 2013, 23, 1422–1430 CrossRef CAS.
- P. S. Hariharan, N. S. Venkataramanan, D. Moon and S. P. Anthony, J. Phys. Chem. C, 2015, 119, 9460–9469 CrossRef CAS.
- S. Ito, T. Yamada, T. Seki, Y. Yamaguchi and M. Asami, Chem. – Asian J., 2016, 11, 1963–1970 CrossRef CAS PubMed.
- T. Wang, N. Zhang, K. Zhang, J. Dai, W. Bai and R. Bai, Chem. Commun., 2016, 52, 9679–9682 RSC.
- Y. Yang, X. Fang, S.-S. Zhao, F. Bai, Z. Zhao, K.-Z. Wang and D. Yan, Chem. Commun., 2020, 56, 5267–5270 RSC.
- B. Lu, S. Liu and D. Yan, Chin. Chem. Lett., 2019, 30, 1908–1922 CrossRef CAS.
- D. Yan and D. G. Evans, Mater. Horiz., 2014, 1, 46–57 RSC.
- K. O’Hara, C. J. Takacs, S. Liu, F. Cruciani, P. Beaujuge, C. J. Hawker and M. L. Chabinyc, Macromolecules, 2019, 52, 2853–2862 CrossRef.
- C. J. Zeman and K.
S. Schanze, J. Phys. Chem. A, 2019, 123, 3293–3299 CrossRef CAS PubMed.
- P. Schmode, A. Savva, R. Kahl, D. Ohayon, F. Meichsner, O. Dolynchuk, T. Thurn-Albrecht, S. Inai and M. Thelakkat, ACS Appl. Mater. Interfaces, 2020, 12, 13029–13039 CrossRef CAS PubMed.
- Y.-C. Lin, C.-K. Chen, Y.-C. Chiang, C.-C. Hung, M.-C. Fu, S. Inagaki, C.-C. Chueh, T. Higashihara and W.-C. Chen, ACS Appl. Mater. Interfaces, 2020, 12, 33014–33027 CrossRef CAS PubMed.
- Y. Ding, F. Zhao, S. Kin, X. Wang, H. Lu, G. Zhang, K. Cho and L. Qiu, ACS Appl. Mater. Interfaces, 2020, 12, 41832–41841 CrossRef CAS PubMed.
- S.-H. Kang, D. Lee, H. Kim, W. Choi, J. Oh, J. H. Oh and C. Yang, ACS Appl. Mater. Interfaces, 2021, 13, 52840–52849 CrossRef CAS PubMed.
- X. Wang, S. Liu, C. Ren, L. Cao, W. Zhang and T. Wu, Macromolecules, 2022, 55, 6415–6425 CrossRef CAS.
- K. Perera, W. Wu, L. You, J. F. Elman, Z. Wang, X. Wang, M. Ahmed, Z. Ke and J. Mei, Macromolecules, 2023, 56, 480–489 CrossRef CAS.
- J.-H. Jou, S. Kumar, D. Tavgeniene, C.-C. An, P.-H. Fang, E. Zaleckas, J. V. Grazulevicius and S. Grigalevicius, J. Mater. Chem. C, 2014, 2, 8707–8714 RSC.
- J. Chen, C. Shi, Q. Fu, F. Zhao, Y. Hu, Y. Feng and D. Ma, J. Mater. Chem., 2012, 22, 5164–5170 RSC.
- J.-H. Jou, C.-J. Li, S.-M. Shen, S.-J. Peng, Y.-L. Chen, Y.-C. Jou, J. H. Hong, C.-L. Chin, J.-J. Shyue, S.-P. Chen, J.-Y. Li, P.-H. Wang and C.-C. Chen, J. Mater. Chem. C, 2013, 1, 4201–4208 RSC.
- J. Kunzelman, M. Kinami, B. R. Crenshaw, J. D. Protasiewicz and C. Weder, Adv. Mater., 2008, 20, 119–122 CrossRef CAS.
- N. D. Nguyen, G. Zhang, J. Lu, A. E. Sherman and C. L. Fraser, J. Mater. Chem., 2011, 21, 8409–8415 RSC.
- S. A. Sharber, K.-C. Shih, A. Mann, F. Frausto, T. E. Haas, M.-P. Nieh and S. W. Thomas, Chem. Sci., 2018, 9, 5415–5426 RSC.
- Y. Dong, J. Zhang, A. Li, J. Gong, B. He, S. Xu, J. Yin, S. H. Liu and B. Z. Tang, J. Mater. Chem. C, 2020, 8, 894–899 RSC.
- Y. Wang, W. Liu, L. Bu, J. Li, M. Zheng, D. Zhang, M. Sun, Y. Tao, S. Xue and W. Yang, J. Mater. Chem. C, 2013, 1, 856–862 RSC.
- L. Bu, Y. Li, J. Wang, M. Sun, M. Zheng, W. Liu, S. Xue and W. Yang, Dyes Pigm., 2013, 99, 833–838 CrossRef CAS.
- M. Zheng, D. T. Zhang, M. X. Sun, Y. P. Li, T. L. Liu, S. F. Xue and W. J. Yang, J. Mater. Chem. C, 2014, 2, 1913–1920 RSC.
- P. Xue, B. Yao, X. Liu, J. Sun, P. Gong, Z. Zhang, C. Qian, Y. Zheng and R. Lu, J. Mater. Chem. C, 2015, 3, 1018–1025 RSC.
- Y. Liu, Y. Lei, M. Liu, F. Li, H. Xiao, J. Chen, X. Huang, W. Gao, H. Wu and Y. Cheng, J. Mater. Chem. C, 2016, 4, 5970–5980 RSC.
- T. Han, X. Gu, J. W. Y. Lam, A. C. S. Leung, R. T. K. Kwok, T. Han, B. Tong, J. Shi, Y. Dong and B. Z. Tang, J. Mater. Chem. C, 2016, 4, 10430–10434 RSC.
- Z. Chen, Y. Nie and S. H. Liu, RSC Adv., 2016, 6, 73933–73938 RSC.
- Y. Lei, Y. Zhou, L. Qian, Y. Wang, M. Liu, X. Huang, G. Wu, H. Wu, J. Ding and Y. Cheng, J. Mater. Chem. C, 2017, 5, 5183–5192 RSC.
- L. Qian, Y. Zhou, M. Liu, X. Huang, G. Wu, W. Gao, J. Ding and H. Wu, RSC Adv., 2017, 7, 42180–42191 RSC.
- S. A. Sharber, K.-C. Shih, A. Mann, F. Frausto, T. E. Haas, M.-P. Nieh and S. W. Thomas, Chem. Sci., 2018, 9, 5415–5426 RSC.
- M. Ikeya, G. Katada and S. Ito, Chem. Commun., 2019, 55, 12296–12299 RSC.
- H. Jiang, X.-J. Liu, R.-R. Jia, T.-H. Xu and M. Xia, RSC Adv., 2019, 9, 30381–30388 RSC.
- H. Gao, P. Xue, J. Peng, L. Zhai, M. Sun, J. Sun and R. Lu, New J. Chem., 2019, 43, 77–84 RSC.
- Z. Qiu, Z. Yang, W.-C. Chen, L. Xing, S. Hu, S. Ji, Q. Yang, N. Cai, X. Ouyang and Y. Huo, J. Mater. Chem. C, 2020, 8, 4139–4147 RSC.
- H. Hu, Z. Chen and S. Pu, Tetrahedron Lett., 2021, 67, 152846 CrossRef CAS.
- S. Nakamura, N. Tohnai, Y. Nishii, T. Hinoue and M. Miura, ChemPhotoChem, 2019, 3, 46–53 CrossRef CAS.
- S. Nakamura, M. Tsuboi, T. Taniguchi, Y. Nishii, N. Tohnai and M. Miura, Chem. Lett., 2020, 49, 921–924 CrossRef CAS.
- M. Tsuboi, S. Nakamura, Y. Nishii, N. Tohnai and M. Miura, Chem. Lett., 2022, 51, 819–822 CrossRef CAS.
- Rare examples of MCL with the rigid skeletons: G. Li, Y. Xu, W. Zhuang and Y. Wang, RSC Adv., 2016, 6, 84787–84793 RSC.
- Rare examples of MCL with the rigid skeletons: T. Kusukawa, S. Shibata, F. Kannen and K. Yoza, Tetrahedron, 2022, 111, 132735 CrossRef CAS.
- Rare examples of MCL with the rigid skeletons: S. Banerjee, A. Akhuli and M. Sarkar, Chem. Phys., 2023, 565, 111762 CrossRef CAS.
- Y. Mizobe, M. Miyata, I. Hisaki, Y. Hasegawa and N. Tohnai, Org. Lett., 2006, 8, 4295–4298 CrossRef CAS PubMed.
- T. Hinoue, Y. Shigenoi, M. Sugino, Y. Mizobe, I. Hisaki, M. Miyata and N. Tohnai, Chem. – Eur. J., 2012, 18, 4634–4643 CrossRef CAS PubMed.
- N. Tohnai, M. Sugino, Y. Araki, K. Hatanaka, I. Hisaki and M. Miyata, Dalton Trans., 2013, 42, 15922–15926 RSC.
- M. Sugino, Y. Araki, K. Hatanaka, I. Hisaki, M. Miyata and N. Tohnai, Cryst. Growth Des., 2013, 13, 4986–4992 CrossRef CAS.
- M. Sugino, K. Hatanaka, T. Miyano, I. Hisaki, M. Miyata, A. Sakon, H. Uekusa and N. Tohnai, Tetrahedron Lett., 2014, 55, 732–736 CrossRef CAS.
- H. Langhals, T. Potrawa, H. Nöth and G. Linti, Angew. Chem., Int. Ed. Engl., 1989, 28, 478–480 CrossRef.
- Y. Mizobe, H. Ito, I. Hisaki, M. Miyata, Y. Hasegawa and N. Tohnai, Chem. Commun., 2006, 2126–2128 RSC.
- M. Sugino, K. HAtanaka, Y. Araki, I. Hisaki, M. Miyata and N. Tohnai, Chem. – Eur. J., 2014, 20, 3069–3076 CrossRef CAS PubMed.
- A. Gavezzotti, Acc. Chem. Res., 1994, 27, 309–314 CrossRef CAS.
- A. Gavezzotti and G. Filippini, J. Phys. Chem., 1994, 98, 4831–4837 CrossRef CAS.
- H. Inokuchi, G. Saito, P. Wu, K. Seki, T. B. Tang, T. Mori, K. Imaeda, T. Enoki, Y. Higuchi, K. Inaka and N. Yasuoka, Chem. Lett., 1986, 1263–1266 CrossRef CAS.
Footnote |
† Electronic supplementary information (ESI) available: Experimental methods, synthetic methods, NMR spectra, HRMS, photophysical data, DSC data, PXRD data, and crystallographic tables. CCDC 2305891–2305902. For ESI and crystallographic data in CIF or other electronic format see DOI: https://doi.org/10.1039/d3tc04748b |
|
This journal is © The Royal Society of Chemistry 2024 |
Click here to see how this site uses Cookies. View our privacy policy here.