DOI:
10.1039/D3VA00176H
(Communication)
Environ. Sci.: Adv., 2024,
3, 355-365
A preliminary investigation of microbial communities on the Athabasca Glacier within deposited organic matter†
Received
28th June 2023
, Accepted 19th January 2024
First published on 31st January 2024
Abstract
Glacier ecosystems are shrinking at an accelerating rate due to changes in climate and also increased darkening from allochthonous and autochthonous carbon leading to subsequent changes in the absorption of light, associated heat, and microbial communities. In this study, in combination with measurement of nutrients and polycyclic aromatic hydrocarbons (PAHs), compositions of microbial communities on surfaces of the Athabasca Glacier (Canadian Rockies, Alberta, Canada) were measured and characterized by use of metabarcoding and scanning electron microscopy. Three matrices, glacier ice, cryoconite hole, and supraglacial surface sediment, were analyzed to gain a first insight into microbial communities on the Athabasca Glacier. Both, eukaryotic and prokaryotic microbial biodiversity was positively correlated with PAH concentrations of Benzo[a]pyrene, Indeno[123-cd]pyrene, Chrysene, Benzo[ghi]perylene, and Dibenz[ah]anthracene. Furthermore, the combustion of petroleum was identified as a major source of PAHs found on the Athabasca Glacier. The high levels of deposition and nutrients observed in this study may lead to an increase in microbial activity and growth that could accelerate glacier melting by further reducing surface albedo. More research is needed to understand the impacts of microbial activity and biodiversity on surface albedo and its effects on glacier meltwater, in the context of global climate change.
Environmental significance
Glacier ecosystems are shrinking at an accelerating rate due to changes in climate, and additionally from increased deposition of soot, which has resulted in darkening of glacier surfaces and subsequent changes in absorption of light, associated heat, and altered microbial communities. In this study, in combination with measurement of nutrients and polycyclic aromatic hydrocarbons (PAHs), compositions of microbial communities on surfaces of the Athabasca Glacier (Alberta, Canada) were measured by use of metabarcoding and scanning electron microscopy. Increasing deposition and accumulation of organic and inorganic compounds on surfaces of the Athabasca Glacier could affect quality of meltwater and might also accelerate melting of the glacier via positive feedback loops from increased microbial biomass reducing surface albedo. The observed processes could foreshadow that the flow of rivers fed by this glacier, and upon which millions of people in Canada and several threatened ecosystems rely, could fall dry in the summer much quicker than currently predicted by climate models. Thus, it is of critical importance to the effective management of this water resource, as well as to the development of strategies to mitigate their impacts, to understand the influence of microbial activity on surface albedo and its effects on meltwater used by humans, in the context of global climate change.
|
1. Introduction
Glaciers, ice fields, and ice sheets cover approximately 10% of the Earth's land surface and represent the largest constituent of the cryosphere.1 Accelerating rates of retreat of glaciers worldwide2 are of global concern, threatening resources of water, biodiversity, associated ecosystem services, and the sustainability of human societies.3–6 This is occurring in the Canadian Rockies at particularly high rates and is expected to accelerate in the future due to climate change.7–9 Solar radiation constitutes the dominant energy for surface melting on almost all snow and ice systems,10 including mountain glaciers.11 Absorbed solar radiation, which is controlled by albedo of the surface, determines rates at which glaciers melt. Albedo of glaciers and perennial snowfields can be decreased through atmospheric deposition of light-absorbing particles.12–16 Light-absorbing particles, including carbonaceous (such as black carbon, brown carbon, algae, and microorganisms) and inorganic (such as mineral dust and volcanic ash) materials, can originate from various sources.17 Black carbon in snow and ice is the result of atmospheric transport and deposition and its main sources are diesel engines, industry, residential solid fuel, and open burning, such as wildfires.18 The amount of light-absorbing particles is also affected by climate variability. Drier conditions, for instance, can lead to more frequent dust transport, while warmer temperatures can increase surface melting and create conditions that favor the growth of algae and microorganisms.17
Glaciers are known to be unique habitats that host a variety of microorganisms as diverse as those living in freshwater habitats19–21 Psychrotroph and psychrophilic microorganisms dominate these supraglacial environments.21 Results of previous studies have shown that these microbial communities play an active role in biogeochemical transformation22 at local and global scales. However, due to their slow growth rates and difficulty of culturing, only little is known about microbial communities in glacial snow and ice.23 Their presence further increases the absorption of light and decreases surface albedo through a mechanism known as bioalbedo.14 Microbes can secrete extracellular polymeric substances (EPS) that lead to the formation of aggregates of minerals and anthropogenic particles. These aggregates can persist on surfaces, extending the persistence of surface-darkening particles and substantially sustaining their effects on albedo and rates of melting.11,24–26 This aggregation with inorganic material leads to the formation of cryoconite, a dark-colored material covering the ice. Over time, cryoconite holes, consisting of small depressions filled with meltwater, can develop when solar-heated inorganic and organic debris melt into the ice. Due to its contribution to melting and biogeochemical cycling, microbial and chemical compositions of cryoconites have been studied.27 The accumulation of specific substances, such as atmospherically deposited anthropogenic contaminants, within these cryoconite holes has been the subject of recent studies.28–33 Pollutants can be transported long distances in the atmosphere and deposited on glacier surfaces, where they undergo post-depositional processes of distributing among different environmental matrices and chemical alterations.34 Consequently, these substances can either enter the food chain or be released into the environment by meltwater impacting downstream ecosystems. This is shown in a previous study29 demonstrating that a glacier-fed tributary was the major source of persistent organic pollutants in a subalpine lake in the Canadian Rockies. The transformation of meltwater chemistry by cryophilic biota and nival ecosystem processes can have a profound impact on nutrient and contaminant mass budgets.35 A better understanding of the relationships between deposited organic matter, microbial community composition and albedo is required to improve understanding of glacier melt rates and biogeochemical cycling on glacier surfaces, as well as the environmental fate of deposited pollutants.
The deposition of organic matter on the Athabasca Glacier has the potential to affect the biodiversity of its prokaryotic and eukaryotic microbial communities. To investigate this hypothesis, the present study employed 16S rDNA (prokaryotic) and 18S rDNA (eukaryotic) metabarcoding and scanning electron microscopy, to gain a first insight into the biodiversity of microbial communities on surfaces of the Athabasca Glacier in Alberta, Canada. Inorganic nutrients and polycyclic aromatic hydrocarbons (PAHs) amounts were assessed along with PAHs congener ratios as markers of soot deposition sources. To our knowledge, this represents the first study of the compositions of microbial communities on the Athabasca Glacier.
2. Materials and methods
2.1 Location and collection of samples
Samples were collected on July 23rd, 2018, from the Athabasca Glacier (52.19182, −117.25165, at 2177 m.a.s.l.) in the Canadian Rocky Mountains (Fig. 1). Samples were opportunistically collected from three matrices, ice (n = 3), glacier surface sediment (n = 1), and cryoconite holes (n = 3), for each sample, parts of the frozen material were extracted and placed in resealable plastic bags. The Athabasca Glacier is an outlet glacier of the Columbia Icefield, which is the hydrological apex of North America and contributes runoff to the largest rivers crossing the continent.8 Smoke from wildfires occurring in heavily forested British Columbia, west of the Columbia Icefield, tends to flow eastward to the Canadian Rockies.9 In the last decade, fire activity upwind of the Columbia Icefield has been highly variable and includes the two worst fire seasons ever recorded, 2017 and 2018.36 In 2018 alone, over 1.35 million hectares were burned.37 The samples were collected following a season of intense wildfires in 2017, which was then followed by an active melt season. In addition to the wildfires, there are other potential sources of PAH contamination on the Athabasca Glacier, such as the tourist activities that have been taking place there since 1969. These activities involve visitors taking tours on the glacier ice using an all-terrain vehicle known as the “Ice Explorer”, which may contribute to increased levels of PAH concentrations. In addition, PAHs are known to be transported over long ranges within the atmosphere. Therefore, PAHs on the Athabasca Glacier could be attributed to long-distance atmospheric transport as well as local sources.
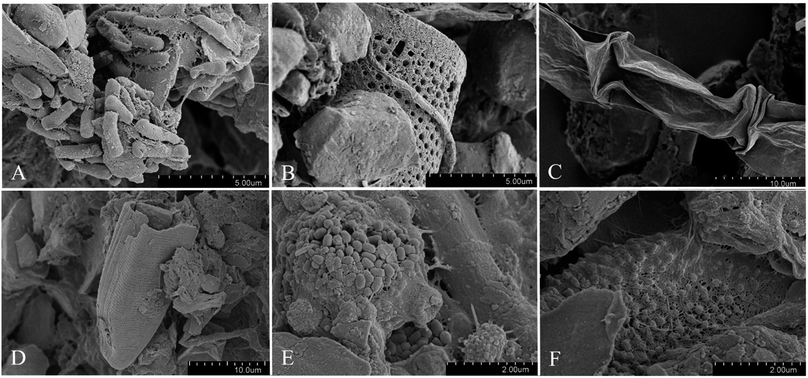 |
| Fig. 1 Scanning electron microscopy of samples from the Athabasca Glacier (Alberta, Canada): of cryoconite holes (A and B) and ice (C–F). (A) Cluster of aggregated bacterial rod-shaped cell association attached to inorganic material. (B and D) Diatoms. (C) Collapsed cell of glacier algae Ancylonema. (E) Clusters of ultramicrobacteria embedded in EPS. (F) EPS-embedded bacteria with regular separation on a surface. Additional SEM images are included in the ESI.† | |
2.2 Scanning electron microscopy
Imaging and description of present microbial communities was conducted by use of scanning electron microscopy (SEM) imaging. Samples were processed and imaged according to a previously outlined protocol with slight modifications.38 Briefly, after samples were dehydrated, they were treated with hexamethyldisilazane (HMDS) twice for 15 min each (instead of 10 min as described by Hynninen et al., 2018), before being mounted on a SEM stub and sputter-coated with a thin layer of gold (Edwards S150B). Samples were then imaged using a SU8010 electron microscope (Hitachi High-Technologies Canada Inc., Etobicoke, Canada) with backscattered electrons imaging mode. Further details of SEM imaging can be found in the ESI.†
2.3 16S and 18S rDNA metabarcoding and bioinformatics
For DNA extraction, 1 g frozen subsamples per sample were prepared. Cryoconite hole and supraglacial sediment samples were centrifuged at 2 °C and 16
000 g for 30 min. The supernatant was removed, and the DNA was extracted from the corresponding pellets using the E.Z.N.A Soil DNA Kit (OMEGA Bio-Tec, Inc., U.S.A.) following the manufacturer's protocol. Ice samples were freeze-dried, and DNA extracted using the same E.Z.N.A Soil DNA extraction kit. The hypervariable V3–V4 region of the bacterial 16S rRNA gene and the V4 fragment of the eukaryotic 18S rRNA gene were amplified by polymerase chain reaction (PCR) using double-tagged PCR primer pairs. PCR products were checked for quality by agarose gel electrophoresis and pooled before undergoing library preparation using the NEBNext Ultra II DNA Library Prep Kit for Illumina (New England Biolab, U.K.). Finally, a 600-cycle paired-end sequencing run was performed on an Illumina® MiSeq sequencer (Illumina, San Diego, CA) using a 2 × 300 bp paired-end Illumina® chemistry kit. More details on the processing of samples for metabarcoding analyses can be found in the ESI.†
Quality control of the sequencing output was first conducted using FastQC.39 The sequences were pre-processed, including quality filtering, trimming, merging, dereplication, and denoising, resulting in a set of predicted biological sequences called zero-radius taxonomic units (ZOTUs). The taxonomic annotation for each ZOTU was assigned using the SILVA database.40 To enable a robust comparison of diversity matrices, rarefaction was applied. Further details on sequencing data processing are described in the ESI.† Sequencing data can be accessed at https://doi.org/10.20383/102.0528.
2.4 Polycyclic aromatic hydrocarbon characterization and nutrient profiling
Polycyclic aromatic hydrocarbons (PAH) were extracted by shaking 10 mL of melted ice, cryoconite, or sediment samples with an equal volume of dichloromethane. Isotope-labelled internal standards (500 mg L−1 acenaphthene-d10, chrysene-d12, and phenanthrene-d10 in acetone) were added at a level of 1 µg per sample. Extracts were reduced to a volume of 0.5 mL under a gentle stream of nitrogen gas and analyzed using gas chromatography-mass spectrometry (GC-MS). Analytes were separated on an Agilent DB-5ms column (60 m length, 250 µm diameter, 0.1 µm film thickness) using a Thermo Scientific Trace 1310 gas chromatograph. Full-scan spectra were obtained using a Thermo Scientific QExactive GC Orbitrap high-resolution/accurate-mass (HRAM) mass spectrometer. Concentrations were determined through an isotope dilution workflow based on internal standard recovery and calibration based on a 7-point calibration curve. Limits of detection/quantification ranged from 0.2/1.4 ng kg−1 (benzo[a]anthracene) to 1.2/7.4 ng kg−1 (dibenz[a,h]anthracene). Metrics inferred from PAH measurement included diagnostic ratios of PAHs that are indicative of the potential sources, specifically:1 anthracene (ANT) and phenanthrene (PHE); ANT/(ANT + PHE),2 fluorene (FLU) and pyrene (PYR); FLU/(FLU + PYR),3 and indeno[1,2,3-cd]pyrene (InP) and benzo[g,h,i]perylene (BgP); InP/(InP + BgP). PAHs are emitted as mixtures and diagnostic ratios can be used to specify emission sources as characteristic PAH profiles and they are found in the environment as a consequence of the combustion or processing of hydrocarbon fuels (e.g., burning of coal, oil, gas, wood, garbage, and tobacco).41 Typically, low molecular weight PAHs are generated during low-temperature processes like wood burning, whereas high-temperature processes like fuel combustion in engines tend to release PAH compounds with higher molecular weights.41 It should be noted that diagnostic ratios of PAHs should only be seen as a rough indication of the emission source, and further research is necessary to attribute the emissions to certain sources.
Total organic carbon (TOC), total dissolved phosphorus (TDP), and total dissolved nitrogen (TDN) were analyzed to assess the nutrient profiles of the glacier samples. TOC was quantified by a commercial lab (Bureau Veritas, Edmonton, Canada) and expressed as either percent or mass concentration, depending on sample characteristics. Further details for the measurement of TOC can be found in the ESI† (SI-4). For TDP and TDN analysis, samples were thawed and then centrifuged at 3500 rpm (∼2054 g) for 10 min to separate liquid and particulate fractions, with the liquid fraction being collected and filtered through 0.45 µm nylon syringe filters. Subsamples of the filtered water were then digested and analyzed on a SmartChem 170 autoanalyzer. Further details for the measurement of TDN and TDP can be found in the ESI (SI-5).†
2.5 Statistical analysis
The statistical analysis and data visualization was performed using R (version 4.1.2). Richness, Shannon diversity, and evenness were calculated and compared between different sample types. A Spearman correlation analysis was performed to test for correlations between alpha diversity metrics and PAH concentrations. For beta diversity, Bray–Curtis distances were calculated. Non-metric multidimensional scaling (NMDS) based on Bray–Curtis was performed to visualize taxonomic composition profiles of prokaryotic and eukaryotic communities. Permutational multivariate analysis of variance (adonis2 function) was used to test whether the differences among sample types were significant. The envfit function (vegan, version 2.5-7) was applied to compute correlations between taxa and ordination scores to obtain taxa that drive differences in community composition. The most discriminant phyla (p < 0.01, 999 permutations) were then fitted on the NMDS plot. If not mentioned otherwise, data are reported as mean ± standard deviation (SD) where the n ≥ 3, while results from duplicate measurements were averaged.
3. Results and discussion
3.1 Characterization of ice and microbe communities by SEM
Overall, the glacier exhibited a pattern of darkening, which indicated potential deposition of organic material and growth of microbial organisms (Fig. S1A and B†).9,36 The persistence of low albedo conditions after a melt season suggests that there may be a bio-albedo feedback loop at play, whereby biological processes contribute to the retention of soot and further darkening of the glacier's snow and ice surface.9,42 SEM analyses revealed the formation of biofilms on all surfaces of the glacier sampled (Fig. 1A–F). The size of the microorganisms observed was relatively small, 50–400 nm (Fig. 1E and F).43,44
3.2 Glacier prokaryotic and eukaryotic microbial communities
Molecular richness, as measured by total ZOTUs (zero-radius operational taxonomic units) differed between glacier sample matrices. The glacier surface sediment sample (n = 1) showed the greatest molecular richness with 3094 ZOTUs for 16S and 1480 ZOTUs for 18S, while glacier ice (n = 3) exhibited the lowest bacterial (1245 ± 42.7; mean ± standard deviation (SD)) and eukaryotic (525 ± 68.4) molecular richness (Table S1†). Cryoconite holes (n = 3) exhibited an intermediate molecular richness for the bacterial (2035 ± 869.2) and eukaryotic microorganisms (837.3 ± 320.7).
In all sequenced samples, bacterial communities were mainly represented by the phyla Cyanobacteria (Table S2,† 38.6 ± 20.3% mean relative abundance ± SD), Proteobacteria (26.5 ± 10.4%), Bacteroidetes (18.1 ± 6.61%), and Actinobacteria (7.31 ± 1.90%) (Fig. 2A), which is consistent with samples from other glaciers (e.g., Northern Schneeferner Glacier, Germany;45 Qaanaaq Glacier, Greenland;46 cryoconites in the Artic and Antarctic47). Cyanobacteria were comprised primarily of the genus Leptolyngbyales (10.2 ± 7.34%) and Pseudoanabaenales (3.07 ± 2.19%), filamentous bacteria, which, due to production of EPS, play a pivotal role in formation of biofilms,21,26,46,48 and Nostocales (1.40 ± 1.22%), which are largely responsible for nitrogen fixation in glacier environments.48,49 Proglacial soils across the globe frequently contain heterocystous cyanobacteria of the Nostocales order.50 The ability of these cyanobacteria to fix atmospheric nitrogen makes them significant contributors to the buildup of organic matter in such sparse environments.50 This promotes the formation of aggregates, thus stabilizing the surface and protecting against physical damage, which allows the establishment of more widely distributed taxa primarily composed of heterotrophs.48,50–53
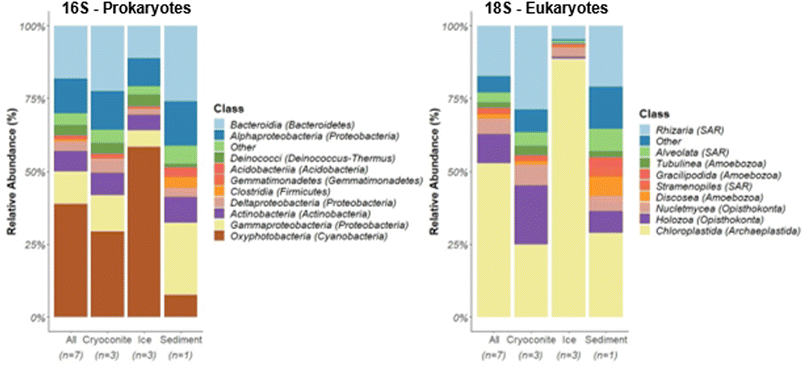 |
| Fig. 2 Composition of microbial communities grouped by sample types. Relative abundance (%) refers to the mean proportion of ZOTUs originating from each class. ZOTUs with mean relative abundances <1% are summarized in the category Other. | |
The assessment of the eukaryotic communities via 18S metabarcoding revealed Chloroplastida (52.9 ± 31.5%), members of the SAR (Stramenophiles, Alveolata, Rhizaria) supergroup (21.9 ± 14.2%), Opisthokonta (15.4 ± 11.1%), and Amoebozoa (7.59 ± 6.65%) as the most abundant eukaryotic taxa among samples (Fig. 2).
Comparing the microbial community composition between sampling types, significant differences were found (PERMANOVA: prokaryotes: p = 0.02; eukaryotes p = 0.02, also see Fig. S5 and S6†). Communities in ice were mainly composed of primary producers, with Cyanobacteria (58.3 ± 16.1% mean relative abundance of prokaryotes) and Chloroplastida (88.7 ± 7.56% mean relative abundance of eukaryotes) dominating. Chloroplastida were almost exclusively represented by the genus Ancylonema (86.8 ± 7.97% total mean relative abundance ± SD), a filamentous alga, which was tentatively confirmed using SEM (Fig. 1C). Accordingly, correlation analysis between taxa and NMDS ordination scores showed higher relative abundances of Zygnematales, which includes Ancylonema, in ice samples compared to the other glacier samples analyzed (Fig. S5†). These autotrophic organisms provide organic carbon for other members of these sparse ecosystems, representing the first steps in the carbon cycle.54,55 Algae belonging to this group have been detected to occupy vast areas of ice surfaces, producing strong secondary pigmentation during summer, further decreasing surface albedo.21 Similar low algal species diversity (>95% Ancylonema) have been seen in other studies with 2-3 species making up >95% of composition.56
Relative abundances of heterotrophic bacteria, including Actinobacteria, Proteobacteria (Alpha-, Gamma-, and Deltaproteobacteria), and Bacteroidetes, were greatest for the sediment sample (78.9%), followed by samples from cryoconite holes (60.8 ± 0.12%), and glacier ice (33.9 ± 9.67%; Fig. 2A). The envfit correlation analysis revealed cryoconites being associated with higher relative abundances in Nitrosomonadaceae, Streptomycetaceae, Bdellovibrionaceae, Blrii41, and Cytophagaceae (Fig S5†).
Furthermore, various taxa with higher relative abundances in sediments appeared to be significant driver for the differences in community composition including Sphingomonadaceae, Xanthomonadaceae, Micrococcaceae, Desulfobacteraceae, Methylococcaceae, Pseudonocardiaceae, Roseiflexaceae, Flavobacteriaceae, and Intrasporangiaceae.
Alphaproteobacteria were mainly composed of the orders Rhizobiales (cryoconite holes 2.5 ± 0.9%; ice 1.0 ± 0.3%, sediment 0.88%) and Sphingomonadales (cryoconite holes 2.2 ± 0.6%; ice 1.2 ± 0.4%, sediment 4.4%), which have been previously detected on other glaciers.57–59 The order Sphingomonadales is known for its ability to degrade a wide range of aromatic hydrocarbons, particularly PAHs.60 Differences between samples were also seen in their eukaryotic composition. Besides Tubulinea, no other taxonomic group within phylum Amoebozoa exceeded a relative abundance of 0.1% within ice samples. In contrast, higher relative abundances for phylum Amoebozoa (Classes Tubulinea, Gracilipodida, Discosea) were measured within the cryoconite holes and the sediment sample. In addition, the greatest abundance of Eutardigrada (Metazoa), eukaryotic grazers, was found in cryoconite (11.4 ± 2.24%), followed by sediment (6.20%), and ice samples (0.30 ± 0.35%). Cryoconite samples were therefore characterized not only by higher relative abundances of heterotrophic bacteria but also higher relative abundances of metazoan (tardigrades) as top grazers, suggesting a self-contained multi-level trophic web within cryoconite holes.47 The analysis of correlations between relative abundances in eukaryotic taxa and NMDS ordination scores indicated that the sediment sample was characterized by higher relative abundances in Chlorellales, Camptobasidiceae, and Heterotrichea, which were identified as significant drivers of differences in eukaryotic community composition.
3.3 Characterization of PAHs and diagnostic ratios
Surface sediment had the greatest quantity of PAHs, with sum concentrations of 737 ng kg−1 (Table 1), followed by ice (268 ± 43.6 ng kg−1) and cryoconite hole samples (134 ng kg−1). Microbial activity could lead to microbial degradation, and thus removal of PAHs (Table S2†). Results of previous studies have indicated degradation of the organophosphorus insecticide chlorpyrifos by microbial communities found in cryoconite.61 FLU was the dominant PAH found in glacier samples, with ANT and PYR being the next two dominant PAHs measured (ESI, Table S3†). Diagnostic ratios of PAHs were applied as a first approach to try and identify sources of these contaminants. It should be noted that diagnostic ratios should be interpreted with caution, especially in new environments. Our findings might indicate that the dominant source of PAHs was the incomplete combustion of fossil fuels and organic materials. The ratio of the PAHs (ANT
:
PHE) was consistently >0.1 which is often considered to be indicative of pyrogenic sources (combustion) over petrogenic sources (fossil fuels) (Table 1; ANT/(ANT + PHE)).41,62,63 Concentration ratios of FLU/(FLU + PYR) with values >0.5, indicated that the fraction of PAHs originating from the combustion of fossil fuels was primarily derived from the combustion of diesel fuel.41,63 The congener ratio InP/(InP + BgP), with values between 0.2 and 0.5, indicated PAHs were derived from the combustion of petroleum.63 These findings are consistent with the operation of a fleet of diesel engine vehicles that carry tourists on the Athabasca glacier in summer and the large number of motor vehicles that travel along the near Icefields Parkway. In addition, the PAHs on the Athabasca Glacier could be attributed to long-distance atmospheric transport from urban areas. Although samples were taken after one season of significant wildfires in British Columbia, west of the Columbia Icefield, there is an indication that the combustion of petroleum appears to be the major input of PAHs on the Athabasca Glacier. Spearman correlation analysis revealed that Shannon diversity and evenness of microbial eukaryotes and prokaryotes, as well as the richness of prokaryotes were positively correlated with the concentration benzo[a]pyrene, indeno[123-cd]pyrene, chrysene, benzo[ghi]perylene, and Dibenz[ah]anthracene (ESI Fig. S7†). The significant impact of PAH contamination on the microbial community composition suggests that surface albedo could be affected in unforeseen ways. Future studies should focus on quantifying a greater diversity of combustion-derived contaminants on the glacier, including aliphatic compounds, and using non-targeted methods to capture this diversity as comprehensively as possible.
Table 1 Polycyclic aromatic hydrocarbon (PAH) profiling of the collected samples normalized to 1 kg of sample material. Select PAH congener ratios were used to help distinguish sources of PAH in measured samples
|
Total PAH (ng kg−1) |
ANT/(ANT + PHE) |
FLU/(FLU + PYR) |
InP/(InP + BgP) |
n = 2 was used for the calculation of this PAH congener ratio.
|
Ice (n = 3) |
268 ± 43.6 |
1.000 |
0.892 ± 0.016 |
0.314a |
Cryoconite hole (n = 2) |
134 |
1.000 |
0.654 |
0.491 |
Sediment (n = 1) |
737 |
1.000 |
0.757 |
0.209 |
Previous research has indicated that cryoconite samples in select Tibetan Plateau glaciers had an InP/(InP + BgP) ratio of >0.5, indicating PAHs derived from grass, wood, and coal combustion.41,64 Cryoconite holes had an InP/(InP + BgP) ratio of 0.491 ± 0.110, broadly indicating that they originated from diesel, coal, or wood combustion. In summary, significant PAH contaminations were found on the surface of the Athabasca Glacier. In comparison to prior investigations into PAH contaminations on diverse glaciers globally, the concentrations observed in this study were both of lesser and greater magnitude when compared with values reported in the literature. For instance, while the Mt. Everest glacier in the central Himalayas was previously reported to have 100 µg L−1 of melted ice65 and the Alpine glacier was found to have 168 µg L−1 of melted ice,66 the current study revealed lower levels of PAH contamination. In contrast, concentrations of PAHs observed in this study were greater than those reported in some other studies, such as the Tibetan Plateau (20.54–60.57 ng L−1)67 and various mountains in Europe (5.6–81 ng L−1).68 Glaciers are considered low-carbon ecosystems from an ecological point of view, where most of the organic carbon present is derived from allochthonous inputs.69 However, the accumulation of organic pollutants, as shown in this study, represents an important source of carbon and therefore may have a significant impact on microbial communities of these ecosystems.70,71
3.4 Nutrient profiling of glacier samples
Amounts of total organic carbon (TOC) varied among samples of glacier materials (Table 2). Surface sediment had the greatest TOC percentage (16.0%), with cryoconite holes having a similar percentage of 13.7%. The TOC content of cryoconite samples previously taken from the Athabasca Glacier was 10.4 ± 0.3%,27 while relative proportions in cryoconite samples from select Tibetan Plateau glaciers were between 0.1 and 6.7%,72 indicating an overall increase relative to previous and different located samples. Glacier ice contained TOC concentrations of 229 ± 185 mg L−1, which were greater than concentrations of dissolved organic carbon (DOC), albeit a different measurement of organic carbon, in surface glacier water collected from ice sheets and various mountain glaciers in Greenland and Antarctica with concentrations ranging from 0.20 ± 0.1 to 0.49 ± 0.07 mg L−1.73 Along with this trend, snow samples taken from the Greenland Ice Sheet, at Summit, Greenland, had average TOC concentrations of 0.064 mg L−1.74 Water collected from cryoconite hole environments of Antarctica and Himalaya glacier surfaces had average TOC concentrations of 0.022 to 1.21 mg L−1 and 0.264 to 16.7 mg L−1, respectively.75 Overall, there is an indication of elevated organic carbon in collected samples from this study.
Table 2 Total organic carbon (TOC) and nutrient (total dissolved nitrogen, TDN; and total dissolved phosphorus, TDP) values in matrices from glacier samples
Sample |
TOC (%) |
TOC (mg L−1) |
TDN (mg L−1) |
TDP (µg L−1) |
n = 3 was analyzed for total organic carbon, with limited material for additional analyses of PAHs and nutrients of the additional glacier cryoconite sample.
n = 2 was used for the calculation of TDN because of insufficient sample volume.
|
Ice (n = 3) |
NA |
229 ± 185 |
7.62b |
85.7 ± 94.9 |
Cryoconite hole (n = 2) |
13.7a |
NA |
6.96 |
67.5 |
Sediment (n = 1) |
16.0 |
NA |
11.5 |
54.7 |
Concentrations of total dissolved nitrogen (TDN) and phosphorus (TDP) differed among types of samples from the glacier. TDN was greatest for surface sediment (Table 2; 11.5 mg L−1), while ice and cryoconite holes had similar values of 7.62 ± 5.17 and 6.96 mg L−1, respectively. In comparison with another glacier, ice samples collected from the Svalbard Glacier contained TDN concentrations of 0.0383 ± 0.0156 mg L−1, which was 100-fold less than what was observed in this study.76 Similarly, Hans and Werenskoid glacier (Svalbard glaciers) ice samples had total nitrogen (TN) of 0.2–0.23 mg L−1 and cryoconite was found to contain 1.17-3.7 mg TN/L.77 Glacier samples from this study all had relatively similar total dissolved phosphorus (TDP), with ice having the greatest (85.7 ± 94.9 µg L−1), surface sediment having the lowest (54.7 µg L−1), and cryoconite holes having a value of 67.5 µg L−1. Previously analyzed grab samples of meltwater from the Athabasca Glacier contained lesser concentrations of total phosphorus of 16 ± 10 µg L−1,32 suggesting dilution by precipitation and early-season snowmelt. TDP concentrations detected in collected samples were exceptionally high when compared to total phosphorus (TP) in ice samples from the two Svalbard glaciers (0.11–0.14 mg L−1).77 Comparison of nutrients between glaciers can be difficult due to the use of various endpoints and sample processing, however, overall elevated amounts were detected in our study samples.
4. Summary
In summary, all samples from the Athabasca Glacier indicated elevated PAH, nutrient, and TOC concentrations relative to studies conducted on various glaciers around the world. Ice samples from the Athabasca Glacier surface had lower nutrient and PAH concentrations and were associated with lower ZOTU richness, but higher relative abundance of autotrophic organisms. In contrast, cryoconite holes and supraglacial sediment hosted highly diverse bacterial and eukaryotic communities, including greater relative abundances of heterotrophic bacteria and eucaryotic grazers, possibly forming a multilevel trophic network.
Studies have shown that cryoconite holes are not spatially isolated, as they can be interconnected and represent dynamic habitats linked to the near-surface ice sheet, which in turn are widely connected to other glacial and extraglacial environments.78 Understanding the dynamics of these communities better is critical, as they have been shown to influence surface energy balance and glacier melt rates. Related studies on the Athabasca Glacier and Columbia Icefield could demonstrate strong and persistent radiative forcing for increased melt due to soot and algae on the snow and ice surfaces.9 In this context, increased deposition of organic matter and nutrients may further increase microbial biodiversity, biomass, and EPS production, leading to a reduction in surface albedo and possibly increased retention of dark sediments, further promoting glacier melt.
Eventually, the inclusion of glacier microbial community dynamics in predictive climate and hydrologic models that explicitly account for glacier melt will have huge potential. Although the extent of the present study is not sufficient to compare the different matrices, this initial assessment provides an important insight into the bacterial and eukaryotic composition of different supraglacial habitats of the Athabasca Glacier. A better understanding of the environmental contaminants and nutrients that may be affecting these communities and their influence on glacial ecosystem processes is urgently needed to better understand the consequences of global climate change and its impact on melt rates and meltwater quality for ecosystems and downstream human use.
Data availability
Sequencing data can be accessed at https://doi.org/10.20383/102.0528. The data used to support the findings of this study are available from the corresponding author upon request.
Author contributions
All authors contributed to the study's conception and design. Samples were taken by Caroline Aubry-Wake. Material preparation, sample processing and analysis were performed by Milena Esser and Phillip Ankley. The first draft of the manuscript was written by Milena Esser and Phillip Ankley and all authors commented on previous versions of the manuscript. All authors read and approved the final manuscript.
Conflicts of interest
The authors declare no competing financial interest.
Acknowledgements
The authors would like to thank Prof. Darren P. Korber (University of Saskatchewan, College of Agriculture and Bioresources) for assistance with the identification of microorganisms in electron microscopy images. The research was supported by a Discovery Grant from the Natural Science and Engineering Research Council of Canada (Project #326415-07) and a grant from Western Economic Diversification Canada (Project #6578, 6807 and 000012711). The authors wish to acknowledge the support of an instrumentation grant from the Canada Foundation for Infrastructure. Prof. Giesy was supported by the Canada Research Chair program and a Distinguished Visiting Professorship in the Department of Environmental Sciences, Baylor University in Waco, TX, USA. The research in this paper is part of the project titled “Next generation solutions to ensure healthy water resources for future generations” funded by the Global Water Futures program, Canada First Research Excellence Fund. Additional information is available at http://www.globalwaterfutures.ca/. Alberta Innovates, Canada Research Chairs, NSERC Discovery Grants and NSERC Vanier Scholarships are gratefully appreciated.
References
- A. M. Anesio and J. Laybourn-Parry, Glaciers and ice sheets as a biome, Trends Ecol. Evol., 2012, 27, 219–225 CrossRef PubMed , available from: http://www.cell.com/article/S0169534711002722/fulltext.
- J. R. Lee, B. Raymond, T. J. Bracegirdle, I. Chadès, R. A. Fuller and J. D. Shaw,
et al., Climate change drives expansion of Antarctic ice-free habitat, Nature, 2017, 547(7661), 49–54 CrossRef CAS PubMed.
- S. Cauvy-Fraunié and O. Dangles, A global synthesis of biodiversity responses to glacier retreat, Nat. Ecol. Evol., 2019, 3(12), 1675–1685 CrossRef PubMed.
- M. Huss and R. Hock, Global-scale hydrological response to future glacier mass loss, Nat. Clim. Change, 2018, 8(2), 135–140 CrossRef , available from: http://www.nature.com/natureclimatechange.
- R. L. Wessels, J. S. Kargel and H. H. Kieffer, ASTER measurement of supraglacial lakes in the Mount Everest region of the Himalaya, Ann. Glaciol., 2002, 34, 399–408 CrossRef , available from: https://www.cambridge.org/core.
- M. Zemp, M. Huss, E. Thibert, N. Eckert, R. McNabb and J. Huber,
et al., Global glacier mass changes and their contributions to sea-level rise from 1961 to 2016, Nature, 2019, 568(7752), 382–386, DOI:10.1038/s41586-019-1071-0.
- G. K. C. Clarke, A. H. Jarosch, F. S. Anslow, V. Radić and B. Menounos, Projected deglaciation of western Canada in the twenty-first century, Nat. Geosci., 2015, 8(5), 372–377 CrossRef CAS , available from: https://www.nature.com/articles/ngeo2407.
- D. Pradhananga and J. W. Pomeroy, Recent hydrological response of glaciers in the Canadian Rockies to changing climate and glacier configuration, Hydrol. Earth Syst. Sci., 2022, 26(10), 2605–2616 CrossRef.
- C. Aubry-Wake, A. Bertoncini and J. W. Pomeroy, Fire and Ice: The Impact of Wildfire-Affected Albedo and Irradiance on Glacier Melt, Earth's Future, 2022, 10(4), e2022EF002685, DOI:10.1029/2022EF002685.
-
D. M. Gray and D. H. Male, Handbook of Snow : Principles, Processes, Management & Use, ed. D. M. Gray and D. H. Male, Pergamon Press, Toronto, New York, 1981 Search PubMed.
- A. J. Hodson, Understanding the dynamics of black carbon and associated contaminants in glacial systems, Wiley Interdiscip. Rev.: Water, 2014, 1(2), 141–149, DOI:10.1002/wat2.1016.
- R. E. Brandt, S. G. Warren and A. D. Clarke, A controlled snowmaking experiment testing the relation between black carbon content and reduction of snow albedo, J. Geophys. Res., 2011, 116, D08109 CrossRef.
- A. D. Clarke and K. J. Noone, Soot in the arctic snowpack: a cause for perturbations in radiative transfer, Atmos. Environ., 2007, 41, 64–72 CrossRef.
- J. M. Cook, A. J. Hodson, A. J. Taggart, S. H. Mernild and M. Tranter, A predictive model for the spectral “bioalbedo” of snow, J. Geophys. Res.: Earth Surf., 2017, 122(1), 434–454, DOI:10.1002/2016JF003932.
- O. L. Hadley and T. W. Kirchstetter, Black-carbon reduction of snow albedo, Nat. Clim. Change, 2012, 2(6), 437–440 CrossRef CAS . http://www.nature.com/natureclimatechange.
- Y. Li, S. Kang, X. Zhang, J. Chen, J. Schmale and X. Li,
et al., Black carbon and dust in the Third Pole glaciers: Revaluated concentrations, mass absorption cross-sections and contributions to glacier ablation, Sci. Total Environ., 2021, 789, 147746 CrossRef CAS PubMed . https://linkinghub.elsevier.com/retrieve/pii/S0048969721028175.
- B. Di Mauro, A darker cryosphere in a warming world, Nat. Clim. Change, 2020, 10(11), 979–980 CrossRef . https://www.nature.com/articles/s41558-020-00911-9.
- T. C. Bond, S. J. Doherty, D. W. Fahey, P. M. Forster, T. Berntsen and B. J. Deangelo,
et al., Bounding the role of black carbon in the climate system: A scientific assessment, J. Geophys. Res.: Atmos., 2013, 118(11), 5380–5552, DOI:10.1002/jgrd.50171.
-
Sturm M. H. G. Jones, J. W. Pomeroy D. A. Walker and R.W. Hoham (eds), 2001. Snow ecology: an interdisciplinary examination of snow-covered ecosystems. Cambridge, Cambridge University Press. 378 pp. ISBN 0-521-58483-3, hardback. £50/US$80, J. Glaciol., 2001, 47, 157, 347, available from: https://www.cambridge.org/core/journals/journal-of-glaciology/article/h-g-jones-j-w-pomeroy-d-a-walker-and-rw-hoham-eds-2001-snow-ecology-an-interdisciplinary-examination-of-snowcovered-ecosystems-cambridge-cambridge-university-press-378-pp-isbn-0521584833-har.
-
J. Laybourn-Parry, M. Tranter and A. J. Hodson, The Ecology of Snow and Ice Environments, Oxford University Press, 2021 Search PubMed.
- A. Boetius, A. M. Anesio, J. W. Deming, J. A. Mikucki and J. Z. Rapp, Microbial ecology of the cryosphere: sea ice and glacial habitats, Nat. Rev. Microbiol., 2015, 13(11), 677–690 CrossRef CAS PubMed , available from: https://www.nature.com/articles/nrmicro3522.
- J. L. Wadham, J. R. Hawkings, L. Tarasov, L. J. Gregoire, R. G. M. Spencer and M. Gutjahr,
et al., Ice sheets matter for the global carbon cycle, Nat. Commun., 2019, 10(1), 1–17 CrossRef PubMed , available from: https://www.nature.com/articles/s41467-019-11394-4.
-
P. K. Mishra, S. Joshi, S. Gangola, P. Khati, J. K. Bisht and A. Pattanayak, Psychrotolerant Microbes: Characterization, Conservation, Strain Improvements, Mass Production, and Commercialization, Springer, Singapore, 2020, pp. 227–246, DOI:10.1007/978-981-15-1902-4_12.
- L. G. Benning, A. M. Anesio, S. Lutz and M. Tranter, Biological impact on Greenland's albedo, Nat. Geosci., 2014, 7, 691 CrossRef CAS , available from: http://www.nature.com/naturegeoscience.
- N. Takeuchi, S. Kohshima and K. Seko, Structure, Formation, and Darkening Process of Albedo-reducing Material (Cryoconite) on a Himalayan Glacier: A Granular Algal Mat Growing on the Glacier, Arct. Antarct. Alp. Res., 2001, 33(2), 115–122 CrossRef , available from: https://www.tandfonline.com/action/journalInformation?journalCode=uaar20.
- A. Hodson, K. Cameron, C. Bøggild, T. Irvine-Fynn, H. Langford and D. Pearce,
et al., The structure, biological activity and biogeochemistry of cryoconite aggregates upon an Arctic valley glacier: Longyearbreen, Svalbard, J. Glaciol., 2010, 56(196), 349–362 CrossRef CAS.
- Y. Xu, A. J. Simpson, N. Eyles and M. J. Simpson, Sources and molecular composition of cryoconite organic matter from the Athabasca Glacier, Canadian Rocky Mountains, Org. Geochem., 2010, 41(2), 177–186 CrossRef CAS.
- F. Pittino, R. Ambrosini, R. S. Azzoni, G. A. Diolaiuti, S. Villa and I. Gandolfi,
et al., Post-depositional biodegradation processes of pollutants on glacier surfaces, Condens. Matter, 2018, 3, 1–10 Search PubMed , available from: http://www.mdpi.com/journal/condensedmatter.
- J. M. Blais, D. W. Schindler, M. Sharp, E. Braekevelt, M. Lafreniěre and K. McDonald,
et al., Fluxes of semivolatile organochlorine compounds in Bow Lake, a high-altitude, glacier-fed, subalpine lake in the Canadian Rocky Mountains, Limnol. Oceanogr., 2001, 46(8), 2019–2031, DOI:10.4319/lo.2001.46.8.2019.
- M. J. Lafrenière, J. M. Blais, M. J. Sharp and D. W. Schindler, Organochlorine Pesticide and Polychlorinated Biphenyl Concentrations in Snow, Snowmelt, and Runoff at Bow Lake, Alberta, Environ. Sci. Technol., 2006, 40(16), 4909–4915 CrossRef.
- L. M. Campbell, D. W. Schindler, D. C. Muir, D. B. Donald and K. A. Kidd, Organochlorine transfer in the food web of subalpine Bow Lake, Banff National Park, Can. J. Fish. Aquat. Sci., 2000, 57(6), 1258–1269 CrossRef CAS.
- K. J. Staniszewska, C. A. Cooke and A. v Reyes, Quantifying Meltwater Sources and Contaminant Fluxes from the Athabasca Glacier, Canada, ACS Earth Space Chem., 2021, 5(1), 23–32 CrossRef CAS.
-
D. B. Donald, J. Syrgiannis, R. W. Crosley, G. Holdsworth, D. C. G. Muir, B. Rosenberg, et al., Delayed Deposition of Organochlorine Pesticides at a Temperate Glacier, 1999 Search PubMed.
- A. M. Grannas, C. Bogdal, K. J. Hageman, C. Halsall, T. Harner and H. Hung,
et al., Atmospheric Chemistry and Physics The role of the global cryosphere in the fate of organic contaminants, Atmos. Chem. Phys., 2013, 13, 3271–3305 CrossRef , available from: http://www.atmos-chem-phys.net/13/3271/2013/.
-
H. G. Jones, The Ecology of Snow-Covered Systems: a Brief Overview of Nutrient Cycling and Life in the Cold, 1999 Search PubMed.
- A. Bertoncini, C. Aubry-Wake and J. W. Pomeroy, Large-area high spatial resolution albedo retrievals from remote sensing for use in assessing the impact of wildfire soot deposition on high mountain snow and ice melt, Remote Sens. Environ., 2022, 278, 113101 CrossRef.
-
Statistics & Geospatial Data – Province of British Columbia., available from: https://www2.gov.bc.ca/gov/content/safety/wildfire-status/about-bcws/wildfire-statistics.
- A. Hynninen, M. Külaviir and K. Kirsimäe, Air-drying is sufficient pre-treatment for in situ visualization of microbes on minerals with scanning electron microscopy, J. Microbiol. Methods, 2018, 146, 77–82 CrossRef CAS PubMed.
-
S. Andrews, Babraham Bioinformatics – FastQC A Quality Control Tool for High Throughput Sequence Data, 2010, available from: https://www.bioinformatics.babraham.ac.uk/projects/fastqc/ Search PubMed.
- C. Quast, E. Pruesse, P. Yilmaz, J. Gerken, T. Schweer and P. Yarza,
et al., The SILVA ribosomal RNA gene database project: Improved data processing and web-based tools, Nucleic Acids Res., 2013, 41(D1), D590–D596 CrossRef CAS PubMed available from: https://pubmed.ncbi.nlm.nih.gov/23193283/.
- M. Tobiszewski and J. Namieśnik, PAH diagnostic ratios for the identification of pollution emission sources, Environ. Pollut., 2012, 162, 110–119 CrossRef CAS PubMed.
-
Jones HG, Pomeroy JW, Walker DA. H. G. Jones, J. W. Pomeroy D. A. Walker and R.W. Hoham (eds), 2001. Snow ecology: an interdisciplinary examination of snow-covered ecosystems. Cambridge, Cambridge University Press. 378 pp. ISBN 0-521-58483-3, hardback. £50/US$80, Journal of Glaciology, 2001, 47(157), 347–347, https://www.cambridge.org/core/journals/journal-of-glaciology/article/h-g-jones-j-w-pomeroy-d-a-walker-and-rw-hoham-eds-2001-snow-ecology-an-interdisciplinary-examination-of-snowcovered-ecosystems-cambridge-cambridge-university-press-378-pp-isbn-0521584833-har.
- L. A. J. Ghuneim, D. L. Jones, P. N. Golyshin and O. V. Golyshina, Nano-Sized and Filterable Bacteria and Archaea: Biodiversity and Function, Front. Microbiol., 2018, 9, 1971 CrossRef PubMed.
- Z. Ren, N. Martyniuk, I. A. Oleksy, A. Swain and S. Hotaling, Ecological Stoichiometry of the Mountain Cryosphere, Front. Ecol. Evol., 2019, 7 DOI:10.3389/fevo.2019.00360.
- C. Simon, A. Wiezer, A. W. Strittmatter and R. Daniel, Phylogenetic diversity and metabolic potential revealed in a glacier ice metagenome, Appl. Environ. Microbiol., 2009, 75(23), 7519–7526 CrossRef CAS PubMed , available from: http://aem.asm.org/cgi/content/full/75/23/7519.
- J. Uetake, S. Tanaka, T. Segawa, N. Takeuchi, N. Nagatsuka and H. Motoyama,
et al., Microbial community variation in cryoconite granules on Qaanaaq Glacier, NW Greenland, FEMS Microbiol. Ecol., 2016, 92(9), 127 CrossRef PubMed , available from: https://academic.oup.com/femsec/article/92/9/fiw127/2197710.
- K. A. Cameron, A. J. Hodson and A. M. Osborn, Structure and diversity of bacterial, eukaryotic and archaeal communities in glacial cryoconite holes from the Arctic and the Antarctic, FEMS Microbiol. Ecol., 2012, 82(2), 254–267 CrossRef CAS PubMed.
- K. Kastovská, J. Elster, M. Stibal and H. Santrůcková, Microbial assemblages in soil microbial succession after glacial retreat in Svalbard (high arctic), Microb. Ecol., 2005, 50(3), 396–407 CrossRef PubMed , available from: https://link.springer.com/article/10.1007/s00248-005-0246-4.
- J. E. Knelman, S. K. Schmidt and E. B. Graham, Cyanobacteria in early soil development of deglaciated forefields: Dominance of non-heterocytous filamentous cyanobacteria and phosphorus limitation of N-fixing Nostocales, Soil Biol. Biochem., 2021, 154, 108127 CrossRef CAS.
- I. S. Pessi, E. Pushkareva, Y. Lara, F. Borderie, A. Wilmotte and J. Elster, Marked Succession of Cyanobacterial Communities Following Glacier Retreat in the High Arctic, Microb. Ecol., 2019, 77(1), 136–147 CrossRef CAS PubMed , available from: https://link.springer.com/article/10.1007/s00248-018-1203-3.
-
J. Elster, Ecological Classification of Terrestrial Algal Communities in Polar Environments, Springer, Berlin, Heidelberg, 2002, pp. 303–326, https://link.springer.com/chapter/10.1007/978-3-642-56318-8_17 Search PubMed.
- I. D. Hodkinson, S. J. Coulson and N. R. Webb, Community assembly along proglacial chronosequences in the high Arctic: Vegetation and soil development in north-west Svalbard, J. Ecol., 2003, 91(4), 651–663, DOI:10.1046/j.1365-2745.2003.00786.x.
- H. Langford, A. Hodson, S. Banwart and C. Bøggild, The microstructure and biogeochemistry of Arctic cryoconite granules, Ann. Glaciol., 2010, 51(56), 87–94 CrossRef CAS , available from: http://www.scaweb.org/.
- A. M. Anesio, A. J. Hodson, A. Fritz, R. Psenner and B. Sattler, High microbial activity on glaciers: Importance to the global carbon cycle, Glob. Change Biol., 2009, 15(4), 955–960, DOI:10.1111/j.1365-2486.2008.01758.x.
-
A. Quesada and W. F. Vincent, Cyanobacteria in the Cryosphere: Snow, Ice and Extreme Cold, in Ecology of Cyanobacteria II, Springer Netherlands, Dordrecht, 2012 Search PubMed.
- S. Lutz, A. M. Anesio, A. Edwards and L. G. Benning, Microbial diversity on Icelandic glaciers and ice caps, Front. Microbiol., 2015, 6, 307 Search PubMed.
- R Ambrosini, F Pittino, M. Maglio, R. S. Azzoni, G. Diolaiuti and A. Franzetti, Bacterial communities of cryoconite holes of the Forni Glacier (Italian Alps) show both seasonal trends and year-to-year variability, Geophys. Res. Abstr., 2018, 10610 Search PubMed.
- Y. Liu, T. J. Vick-Majors, J. C. Priscu, T. Yao, S. Kang and K. Liu, Biogeography of cryoconite bacterial communities on glaciers of the Tibetan Plateau, FEMS Microbiol. Ecol., 2017, 93(6), fix072 Search PubMed.
- F. Pittino, M. Seeger, R. Azzoni, R. Ambrosini, A. Franzetti and F. Santa María, Geographical variability of bacterial communities of cryoconite holes of Andean glaciers, Sci. Rep., 2021 DOI:10.1101/2021.01.14.426633.
-
M. A. Kertesz, A. Kawasaki and A. Stolz, Aerobic Hydrocarbon-Degrading Alphaproteobacteria: Sphingomonadales 6, 2019, DOI:10.1007/978-3-030-14796-9_9.
- C. Ferrario, F. Pittino, I. Tagliaferri, I. Gandolfi, G. Bestetti and R. S. Azzoni,
et al., Bacteria contribute to pesticide degradation in cryoconite holes in an Alpine glacier, Environ. Pollut., 2017, 230, 919–926 CrossRef CAS PubMed , available from: https://pubmed.ncbi.nlm.nih.gov/28738304/.
- H. Budzinski, I. Jones, J. Bellocq, C. Piérard and P. Garrigues, Evaluation of sediment contamination by polycyclic aromatic hydrocarbons in the Gironde estuary, Mar. Chem., 1997, 58(1–2), 85–97 CrossRef CAS.
- M. B. Yunker, R. W. Macdonald, R. Vingarzan, R. H. Mitchell, D. Goyette and S. Sylvestre, PAHs in the Fraser River basin: a critical appraisal of PAH ratios as indicators of PAH source and composition, Org. Geochem., 2002, 33(4), 489–515 CrossRef CAS.
- Q. Li, S. Kang, N. Wang, Y. Li, X. Li and Z. Dong,
et al., Composition and sources of polycyclic aromatic hydrocarbons in cryoconites of the Tibetan Plateau glaciers, Sci. Total Environ., 2017, 574, 991–999 CrossRef CAS PubMed.
- X. Wang, P. Xu, B. Qing, K. S. Chang, C. Z. Yuan and Y. T. Dong, The historical residue trends of DDT, hexachlorocyclohexanes and polycyclic aromatic hydrocarbons in an ice core from Mt. Everest, central Himalayas, China, Atmos. Environ., 2008, 42(27), 6699–6709 CrossRef CAS.
- F. Cappa, N. Suciu, M. Trevisan, S. Ferrari, E. Puglisi and P. S. Cocconcelli, Bacterial diversity in a contaminated Alpine glacier as determined by culture-based and molecular approaches, Sci. Total Environ., 2014, 50(9), 497–498 Search PubMed , available from: https://pubmed.ncbi.nlm.nih.gov/25117971/.
- Q. L. Li, N. L. Wang, X. B. Wu, J. C. Pu, J. Q. He and C. W. Zhang, Sources and distribution of polycyclic aromatic hydrocarbons of different glaciers over the Tibetan Plateau, Sci. China Earth Sci., 2011, 54(8), 1189–1198 CrossRef CAS , available from: https://link.springer.com/article/10.1007/s11430-010-4047-3.
- G. Carrera, P. Fernández, R. M. Vilanova and J. O. Grimalt, Persistent organic pollutants in snow from European high mountain areas, Atmos. Environ., 2001, 35(2), 245–254 CrossRef CAS.
-
M. Stibal, M. Tranter, L. G. Benning and J. Ř. Ehák, Brief Report Microbial Primary Production on an Arctic Glacier Is Insignificant in Comparison with Allochthonous Organic Carbon Input, 2008 Search PubMed.
- M. S. Shamurailatpam, J. Telling, J. L. Wadham, A. L. Ramanathan, C. A. Yates and N. J. Raju, Factors controlling the net ecosystem production of cryoconite on Western Himalayan glaciers, Biogeochemistry, 2023, 162(2), 201–220 CrossRef CAS.
- M. Stibal, M. Šabacká and K. Kaštovská, Microbial communities on glacier surfaces in Svalbard: Impact of physical and chemical properties on abundance and structure of cyanobacteria and algae, Microb. Ecol., 2006, 52(4), 644–654 CrossRef , available from: https://link.springer.com/article/10.1007/s00248-006-9083-3.
- Q. Li, N. Wang, C. Barbante, S. Kang, A. Callegaro and D. Battistel,
et al., Biomass burning source identification through molecular markers in cryoconites over the Tibetan Plateau, Environ. Pollut., 2019, 244, 209–217 CrossRef CAS PubMed.
- E. Hood, T. J. Battin, J. Fellman, S. O'Neel and R. G. M. Spencer, Storage and release of organic carbon from glaciers and ice sheets, Nat. Geosci., 2015, 8(2), 91–96 CrossRef CAS , available from: https://www.nature.com/articles/ngeo2331.
- E. von Schneidemesser, J. J. Schauer, M. M. Shafer, G. S. W. Hagler, M. H. Bergin and E. J. Steig, A method for the analysis of ultra-trace levels of semi-volatile and non-volatile organic compounds in snow and application to a Greenland snow pit, Polar Sci., 2008, 2(4), 251–266 CrossRef.
- A. Sanyal, R. Antony, G. Samui and M. Thamban, Microbial communities and their potential for degradation of dissolved organic carbon in cryoconite hole environments of Himalaya and Antarctica, Microbiol. Res., 2018, 208, 32–42 CrossRef CAS PubMed.
- J. D. Zarsky, M. Stibal, A. Hodson, B. Sattler, M. Schostag and L. H. Hansen,
et al., Large cryoconite aggregates on a Svalbard glacier support a diverse microbial community including ammonia-oxidizing archaea, Environ. Res. Lett., 2013, 8(3), 035044, DOI:10.1088/1748-9326/8/3/035044.
- J. Grzesiak, D. Górniak, A. Świątecki, T. Aleksandrzak-Piekarczyk, K. Szatraj and M. K. Zdanowski, Microbial community development on the surface of Hans and Werenskiold Glaciers (Svalbard, Arctic): a comparison, Extremophiles, 2015, 19(5), 885–897 CrossRef PubMed.
- J. Cook, A. Edwards and A. Hubbard, Biocryomorphology: Integrating microbial processes with ice surface hydrology, topography, and roughness, Front. Earth Sci., 2015, 3, 1–6 Search PubMed.
|
This journal is © The Royal Society of Chemistry 2024 |
Click here to see how this site uses Cookies. View our privacy policy here.