Investigating Thermomyces lanuginosus and Purpureocillium lilacinum to produce advanced myco-materials through selective fungal decellularization
Received
3rd August 2023
, Accepted 19th July 2024
First published on 15th August 2024
Abstract
In healthcare and human life, and with the growing need for environmentally friendly materials to replace synthetic ones, biomaterials are essential. Desirable biomaterials may now be created using a wide range of extracted natural polymers. Mycelium-based biomaterials are being developed into more adaptable, inexpensive, and self-replicating products. Some fungal species, like Pleurotus ostreatus and Ganoderma lucidum, have been recognised as excellent sources of biomaterials with unique morphological, mechanical, and hydrodynamical characteristics. Thermomyces lanuginosus and Purpureocillium lilacinum are two fungal strains that may be used to create biomaterials. This article seeks to introduce these strains and use experimentation to identify their distinctive characteristics. The fungus was cultivated in a lab, and the growth kinetics of the fungus were estimated. The strains of P. lilacinum and T. lanuginosus had maximum specific growth rates (μmax) of 1.34 ± 0.024 and 3.09 ± 0.019 L−1 d−1, respectively. Decellularization of the fungal biomass was performed using 0.1% SDS solution, after which the scaffolds were created by drying the biomass in plastic moulds. Following that, analysis using scanning electron microscopy (SEM), energy dispersive X-ray spectroscopy (EDS), and Fourier transform infrared spectroscopy (FT-IR) was carried out. The porosity and swelling ratio were also determined and hydrodynamic characterization was performed for the samples. The results show that mycelia have the potential to serve as inexpensive, all-natural bio-scaffolds and T. lanuginosus-prepared materials have a larger swelling ratio and increased porosity, which makes them better myco-materials than those formed from P. lilacinum.
Environmental significance
In a world increasingly driven by environmental sustainability, the emergence of novel biomaterials assumes a pivotal role. Fungal biomaterials have a big impact on the environment and can help create a future that is more environmentally friendly and sustainable. Among the main advantages for the environment are biodegradability, reduced reliance on synthetic materials, less use of energy and resources, and sequestering carbon as the mycelium develops, which may aid in absorbing carbon from the atmosphere. Many fungal strains used for biomaterial production can be cultivated on waste products from forestry and agriculture, which minimizes the need to alter land use and extract new resources. Additionally, a variety of industries, including building, textiles, and medical devices, may employ fungal biomaterials. Their versatility in applications can lead to reduced environmental impacts across multiple industries. In summary, fungal biomaterials provide a noteworthy ecological substitute for traditional materials, aiding in the preservation of resources, mitigation of pollution, and the adoption of a more sustainable methodology for the manufacturing and use of materials. Their development and adoption correspond to the worldwide movement in many sectors toward more environmentally friendly and sustainable methods. Significantly, our study presents a sustainable and economically viable route to biocompatible material creation, ready to tackle urgent environmental issues and support long-term solutions across several sectors.
|
1. Introduction
Living creatures are now being carefully incorporated into the fields of materials science and nanotechnology in order to meet the demand for intelligent biological resources with high-end applications that cannot be produced synthetically. To achieve this, sufficient research involvement is needed to develop viable goods that are non-polluting and ecologically sound. Recently, the primary emphasis in this area of study has been on materials that are more widely accessible globally, are sustainable, have better physical properties, and have superb final uses. One of the biggest categories of living things on the planet is fungi. Mycelia, the vegetative portion of fungi, are becoming a highly adaptable, self-growing, and affordable biomaterial. It has so far been used experimentally for packaging, textile, and building applications.1 Mycelia are made up of hyphae, which are elongated cells developed by absorption of nutrients from their growth medium and naturally form fibrous mats. Mycelia have a generally porous structure that they have self-grown, and their hyphal outer membranes show both proteins and carbohydrates. It should be noted that altering both the fungus strain and the medium may fine-tune the shape, chemical makeup, hydrodynamics, and mechanical characteristics of mycelia.2,3
Many different scaffold materials have been created, and methods like electrospinning, freeze-drying, and 3D printing are available and widely used. Also, making these materials involves moulding: the nutritional substrate and mycelium spores were combined, put into a mould, and allowed to expand until the mycelium development was halted by heating.4 The mycelium multiplies rapidly and generates a large number of self-assembling connections in the form of minute threads known as hyphae, which cover the source completely, decompose it, and combine it into an enduring and organic substance. The primary elements of the mycelium are natural biopolymers like cellulose, chitin, various proteins, etc. Lignin, cellulose, pectin, various compounds from microbes or medicinal plants, protein products from both animals and plants, etc. are essential resources to create biopolymers among the various natural sustainable resources. These materials are all recyclable, easily biodegradable, and have many other beneficial qualities.5,6
This work focuses on the easy creation of a fungal scaffold and the investigation of its properties using various analytical techniques. Yeast-peptone-dextrose (YPD) is used as the foundation for the development of a mycelium-based compostable substance, and its various characteristics, including morphology, chemical composition, and porosity are assessed. Two filamentous fungus species were selected for the work, Purpureocillium lilacinum and Thermomyces lanuginosus.
Despite the fact that both T. lanuginosus and P. lilacinum have promising characteristics for scaffold creation, they each have their own set of beneficial characteristics. In addition to being an excellent producer of biomass, T. lanuginosus is also capable of producing a sturdy mycelium, which makes it possible to construct scaffolds that have certain structural characteristics that are desired. On the other hand, P. lilacinum stands out due to its capacity to release bioactive compounds that encourage the development and differentiation of cells. Enzymes that are capable of breaking down complex organic molecules are produced by T. lanuginosus, which survives at higher temperatures (up to 60 degrees Celsius) and generates them effectively. For the purpose of implementing certain features into the scaffold, this could prove to be advantageous. P. lilacinum, on the other hand, is able to grow at temperatures that are milder (between 26 and 30 degrees Celsius) and has a wide range of adaptability to different growing conditions. In addition, the surface chemistry of P. lilacinum has the ability to be modified in order to cater to the requirements of certain different kinds of cells. For this reason, the selection of one of these strains over another is contingent upon the particular needs of the tissue engineering application that is being targeted. If maintaining structural integrity is of utmost importance, T. lanuginosus could be the better option. P. lilacinum, on the other hand, would be a preferable option if the enhancement of cell growth and the customisation of the scaffold for certain cell types is the focal points of the investigation. On the other hand, more research is necessary to optimise the usage of both fungi in scaffold building and to evaluate their long-term performance for applications in tissue engineering.
Fungal biomaterials hold significant environmental implications that can contribute to a more sustainable and eco-friendly future. Some key environmental benefits include: bio-degradability, reduction of synthetic materials, lower energy and resource consumption, and carbon sequestration as the mycelium can help sequester carbon from the atmosphere as it grows. This process can contribute to mitigating climate change by capturing and storing carbon in the fungal biomass.7 Also, fungal biomaterials can be used in a wide range of applications, from packaging and construction to textiles and medical devices. The purpose of this research paper is to develop myco-materials from the biomass of the species P. lilacinum and T. lanuginosus, and then to investigate the morphological, mechanical, and hydrodynamical properties of the materials that are created.
2. Materials and methods
A workflow with experiments, calculations and analytical techniques was planned and performed as described in detail below and also represented as a schematic diagram (Fig. 1).8,9
 |
| Fig. 1 Schematic representation of the workflow. | |
2.1 Strains, growth media and culture conditions
Purpureocillium lilacinum: Luangsa-Ard et al. classified the genus Purpureocillium in the Ophiocordycipitaceae family which is a type of filamentous fungus. It was often isolated from nematodes, insects and the rhizosphere of numerous crops in 2011 based on its medicinal value.10–12 The species may thrive in a broad range of temperatures between 8 and 38 °C; however 26 to 30 °C is ideal.13 It may thrive on any kind of surfaces and has a broad pH tolerance. The colonies are spherical, purple, and swollen. Conidia are single-celled, chain-like, oval to spindle-shaped, and are around 2.0–2.3 and 3.1–4.0 μm microns in size. A hyaline mycelium with well-developed septate and numerous branches is produced by P. lilacinum. This fungus has the potential to be an effective biocontrol agent for root-knot nematodes in crops. Like other fungi, Purpureocillium lilacinum's chemical and molecular composition may change based on a number of variables, including growing conditions, substrates, and genetic variants. Proteins involved in enzymatic reactions, structural support, signalling, and defense mechanisms; carbohydrates like glucose, fructose, and different polysaccharides; fatty acids, sterols, phospholipids, and other lipid derivatives; nucleic acids; and secondary metabolites like mycotoxins, antibiotics, pigments, and other bioactive substances make up the basic molecular makeup of fungi.14 It is well recognized that Purpureocillium lilacinum may parasitize and control a variety of nematodes, especially those that are damaging to plants or animals. In addition, it may infect and harm other living things like mites and insects. It is an essential component of biological pest management since it is an entomopathogenic fungus.15
Thermomyces lanuginosus (MTCC:9331): A widespread thermophilic fungus called Thermomyces lanuginosus (previously referred to as Humicola lanuginosa) is often isolated from self-heating piles of organic detritus. It is a thermophilic fungus since strains often develop between 20 °C and 62 °C, with 50 °C serving as the ideal growth temperature.16 The majority of the strains examined had a pH of 6.5 for optimal development. There have been reports of T. lanuginosus strains in dry and wet grassland, loamy garden soil, and aquatic sediments, but the fungus is more closely related to organic substrates like grass culms, roots, and leaves, composts made from a variety of plant materials, and the dung of different birds and mammals. T. lanuginosus was initially discovered in soil where it participated in the decomposition of compost before being discovered as a plant endophyte.17 Colonies begin as white and silky, usually less than 1 mm in height, but quickly change to a grey or green-grey color, beginning in the centre. The thick body compartments found in the hyphae of thermophilic moulds developed at high temperatures (above 50 °C) serve as storage structures, primarily for phospholipids.18 The filamentous components that make up the body of Thermomyces lanuginosus are often formed as branching, septate hyphae. Asexual spores known as conidia are produced by the hyphae, which may reach lengths of up to several centimetres. The capacity of Thermomyces lanuginosus to generate a variety of extracellular enzymes is well documented. Many hydrolytic enzymes, including as cellulases, hemicelluloses, amylases, proteases, lipases, and pectinases, are secreted by it. With the aid of these enzymes, the fungus is able to digest complex organic components and use them as a source of nutrition.19 It is used to make a variety of commercial enzymes, mainly in the manufacture of biofuels and biotechnology.20Table 1 provides a review of the numerous species that have been employed to create biomaterials to date.
Table 1 Summary of biomaterials produced using various species of organisms
S. no. |
Organism – species |
Biomaterials produced |
References |
1 |
Bacteria – Gluconacetobacter, Agrobacterium, Achromobacter, Acetobacter xylinum |
Bacterial cellulose, bioplastics, biofilms |
21,22
|
2 |
Yeast – Saccharomyces cerevisiae, Aureobasidium pullulans |
Yeast-derived proteins, bioactive peptides |
23,24
|
3 |
Algae – Chlamydomonas reinhardtii, Chlorella pyrenoidosa, Scenedesmus obliquus |
Algal polysaccharides, microalgae lipids |
25
|
4 |
Plants – Acacia pennata, Colocasia esculenta, Bambusa vulgaris, Gossypium herbaceum |
Plant fibers, plant-derived polymers |
26,27
|
5 |
Insects – Bombyx mori L., Manduca sexta |
Silk proteins, chitin-based materials |
28,29
|
6 |
Marine life – Axinella cannabina, Suberites carnosus, Arthrospira platensis, Chlorella pyrenoidosa |
Coral skeletons, fish scales, mollusc shells |
30,31
|
7 |
Animals – Tunicata, Araneae |
Collagen, gelatin, elastin, extracellular matrix |
32,33
|
Yeast-peptone-dextrose (YPD) is used as the foundation for the development of a mycelium-based compostable substance, and its various characteristics, including morphology, chemical composition, and porosity are assessed. To be cultivated, fungal stocks of Purpureocillium lilacinum and Thermomyces lanuginosus were gathered. Yeast peptone dextrose agar (YPDA) was made in the proportions 1
:
2
:
2 (yeast extract
:
peptone powder
:
dextrose). All materials and media were autoclaved for 15 minutes at 120 °C. Following that, a little inoculum of mycelium was inoculated onto the plates to allow the mycelia to germinate. Stock cultures were revived on plates incubated at 30 °C for 72 hours using YPDA. They were then isolated and sub-cultured in the same medium and growth conditions. The grown spores were put into 1000 mL Erlenmeyer flasks containing 750 mL culture media each (YPDB). For 8 days, the cultures were agitated at 30 °C and 80 rpm on an orbital shaker incubator (CIS-24 PLUS from Remi Elektrotechnik Ltd).
2.2 Fungal growth in liquid media
The YPD liquid culture medium was inoculated with the fungal mycelium and cultured at 30 °C for 168 hours. Growth curves were produced using absorbance data collected during the time, as detailed below. During strain development in liquid medium, inoculation Erlenmeyer flasks were removed from the orbital shaker one at a time at distinct time intervals of 12 hours. After every 12 hours, small samples of 3 ml were obtained from each species and their absorbance was evaluated against a blank medium using a UV-spectrophotometer (LabIndia) at 600 nm. Using the acquired data, a growth curve was constructed, with absorbance plotted versus incubation time.
2.3 Growth kinetics of fungal cultures
To predict fermentation time and cell mass obtained μmax was calculated. A higher “μmax” indicates increased cell production. 3 ml of culture sample and control were withdrawn after every 12 hours and the absorbance was recorded at 600 nm with control medium as blank. 1 ml culture was filtered through a pre-weighed Whatman filter paper and after filtration the dried cell weight was measured. The data collected was then plotted into graphs, studied and the slopes were determined.
2.4
μ
max estimation using biomass
The following equation was used to calculate the biomass for our experiment:34 |
 | (1) |
where Md is the weight of the filter with the dried biomass and Mi is the weight of the pre-weighed filter, and V is the volume of culture.
According to Huesemann et al. (2016),35 the values of BM were used to determine the fungi's specific growth rate (μ) as follows:
|
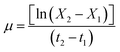 | (2) |
where X1 and X2 are the BM at two different time points, and t1 and t2 are the corresponding times in days.
The capacity for biomass growth is reflected in the specific growth rate, however the doubling time offers a more useful and accessible metric. The time needed for the quantity of live cells to double is known as the “doubling time”. Slower growth is indicated by a longer doubling time. Eqn (3) was used to compute the doubling time (Td) in days.
|
 | (3) |
A fungal culture resembles a first-order chemical process during the exponential (or logarithmic) development phase when the rate of cell growth is inversely correlated with the number of fungi present. The growth rate constant, or constant of proportionality, is an indicator of the growth rate:
Rate of increase of cells = μ × number of cells |
2.5 Filtration, drying and scaffold preparation
Several distinct filtration methods are used in order to accomplish the separation of mycelial biomass or fungal cells from the liquid media in which they are suspended. To determine which filtering technology should be used the size and concentration of the fungal biomass, the level of purity of the end product that is required, and the extent to which the process can be carried out economically were determined. A typical technique for separating solids from liquids or eliminating solid contaminants from a liquid sample is filtering using Whatman filter paper. The biomass was seen to gather in the bottom of the flasks after 15 days of incubation. Then Whatman filter paper no. 1 was used to filter it. The broth was discarded and the biomass of the cultivated fungus was collected on the filter paper. Ethanol solutions with a gradient of 20%, 40%, 60%, and 80% were used to wash the biomass. After washing, formaldehyde was used to stabilise the biomass.
Drying fungal biomass and preparing scaffolds by employing fungal biomass are two techniques that are essential to prepare biomaterials. In these methods, the moisture is extracted from the fungal biomass, and structured scaffolds are created for a variety of different uses. Few techniques for drying fungal biomass are air drying, freeze drying and spray drying. The biomass collected after filtration were moulded using sterilised plastic moulds and dried in a hot air oven overnight at 50 °C. Further characterisation tests were performed using the dried and dead biomass (biomaterial thus synthesized).
2.6 Decellularization
Decellularization may be accomplished using a variety of approaches, such as enzymatic, chemical, and physical ones. These techniques attempt to disrupt and eliminate cells while causing the least amount of harm to the extracellular matrix (ECM) components, preserving tissue-specific bioactive chemicals necessary for cellular repopulation. The choice of procedure depends on the kind of tissue, planned uses, and desired results. Detergent or surfactant is used to decellularize the fungal tissue. Sodium dodecyl sulphate (SDS), Triton X, EDA, alkyl processing, acid, an ion-cleaning agent, a non-ionic detergent, an amphoteric detergent, or mixtures of these are examples of detergent in certain implementations. For each strain, three samples were taken and weighed in equal amounts. A 0.1% SDS solution was prepared. Each strain's samples were placed in the solution. 2 of the 3 samples for each strain were dipped in pH 7 maintained SDS; out of them 1 was sonicated for 15 min. The other sample was placed in pH 12 maintained SDS solution. The concentration of released DNA was estimated using a nanodrop spectrophotometer in triplicate (Thermoscientific ND-1000).
2.7 Chemical composition analysis: Fourier transform infrared spectroscopy (FT-IR) analysis
FTIR spectroscopy may be used for a variety of tasks, including substance identification, mixture composition analysis, chemical reaction monitoring, material quality assessment, and molecular structure and bonding investigation. For spectrum analysis of the biomaterials derived, a Bruker ALPHA II FTIR spectrophotometer was employed. With a resolution of 4 cm−1 along with forward and reverse rotating mirror speeds of 10 and 6.2 kHz, respectively, spectra in the 600–4000 cm−1 range were captured. Prior to examination, the samples were oven dried at 50 °C, and the dried biomaterial samples were used for spectrum analysis. All of the samples' infrared spectra were recorded, and the treated samples' infrared spectra were used to look for distinctive peaks that matched the functional groups in the materials made from P. lilacinum and T. lanuginosus.
2.8 Morphological characterization – scanning electron microscopy and energy dispersive X-ray spectroscopy (SEM-EDS)
This method combines the elemental analysis capabilities of energy-dispersive X-ray spectroscopy (EDS) with the imaging capabilities of a scanning electron microscope (SEM). The fungal scaffolds were analysed using Scanning Electron Microscopy (SEM) to visually evaluate the morphology. In order to prepare the samples, two 2 mm pieces of the scaffolds were dried for 10 minutes at 50 °C in a hot air oven. The samples had been coated with gold (50 nm) using sputter coating prior to SEM examination. The samples were studied using a ZEISS EVO Series scanning electron microscope, model EVO 18, before which a 5 nm thick gold coating was applied. 20 kV SEM was used to visualize the surface morphology of the materials. Analysis was also done on the EDS spectra. Individual spectra from 10 separate locations were combined to create an average spectrum.
2.9 Porosity
The porosity is defined as the proportion of the volume fraction (the volume of interior cavities) to the external sample volume. In order to keep the implant mechanically stable, porosity functions as a binding between the scaffold and the surrounding tissues.36 The porosity of a material may be assessed using a variety of techniques. The liquid-displacement technique is one of them. Ethanol is employed as the solvent in this procedure, and the solvent's initial volume (V1), volume during diffusion (V2) and residual volume (V3, solvent's volume after diffusion and removal of material) are determine to calculate the material's porosity.
Now, the following equation is used to calculate the biomaterial's porosity.
where
V1 = volume of the solvent before immersing the scaffold.
V2 = volume of the solvent with a scaffold after one hour.
V3 = volume of the solvent after scaffold removal.
Some simple ways to determine a material's porosity include utilising the plugins for tools like jPOR macro, OriginPro and ImageJ. The porosity here is assessed using SEM images. The porosity for the two scaffold samples generated from strains Purpureocillium lilacinum and Thermomyces lanuginosus was assessed by analyzing SEM images. To compute the same, SEM pictures for the samples were viewed in ImageJ software. The photographs were picked and in colour settings split channels were opted for. The best channel with the greatest contrast and finest quality was picked. The rectangle selection tool was used to choose the greatest rectangular piece of the coating feasible, exclusive of either interface. The selection was clipped and the threshold tool was used to pick the regions of porosity. The picture was transformed to a binary image of the porosity then the particles were evaluated. The results may be shown now. A summary for the findings was prepared and from here the area fraction value was taken. This figure represents the percentage of porosity.
2.10 Swelling ratio estimation
The swelling ratio is the proportional increase in weight and thickness of the material brought on by water absorption. In simple words, it is the ratio of the original weight/thickness of the dry material to the difference between the starting weight/thickness of the material and the weight/thickness of the completely swelled mass. To determine how much swelling tendency the samples possess for the correct transportation of molecules, a swelling ratio test is an essential methodology. First, samples of a fungal scaffold were dried to determine the swelling ratio. After drying, the samples were weighed. Each sample was then submerged in distilled water and 1× phosphate-buffered saline (PBS). After three hours of immersion, the samples were removed to be weighed and measured once again.
The swelling ratio percentage is measured using the following equation.
where
W1 is the dry weight of the sample and
W2 is the swelled weight of the sample.
37
2.11 Hydrodynamic characterization (hydrophilicity/hydrophobicity)
A scaffold's hydrophilic properties are necessary for cell adhesion and proliferation. Most of the time, hydrophilic scaffolds encourage cell growth and proliferation. The contact angle measuring methodology is used to determine if a scaffold is hydrophilic or hydrophobic. According to Law K. Y. (2014),38 a material is said to be hydrophilic if the contact angle is below 90° and hydrophobic if it is more than 90°. At room temperature, static water contact angle (CA) measurements were made using ImageJ software developed at the National Institutes of Health (NIH) and the Laboratory for Optical and Computational Instrumentation. 5 μL of distilled water were dropped onto the appropriate surfaces, and after 10 s, side view pictures of the drops were taken using a camera of 108 MP at 1×. Every sample was subjected to a maximum of ten contact angle measurements, which were carried out at random sites. The average values of these measurements were reported. By using the programme to suit the photographed drop form, the CA were computed automatically using “Contact Angle” plugins to measure the contact angle image clicked.
3. Results and discussion
3.1 Growth kinetics and μmax calculation
To learn more about P. lilacinum and T. lanuginosus's growth rates in a lab setting, the two species were grown in conical flasks filled with YPD medium. For 15 days, batch cultivation was done. Fig. 2 shows the development curves for both species under the same environmental conditions. By measuring their absorbance and dry cell biomass in an interval of 12 hours, which has been provided in Table 2, mycelial culture development was studied in order to understand its growth pattern. The specific growth rate and maximum specific growth rate (μmax) were examined using the data. For the fungus P. lilacinum, the log phase was quite long; in the growth curve it began at 72 h and ended at 168 h. T. lanuginosus had an exponential phase ranging from 24 to 96 hours.
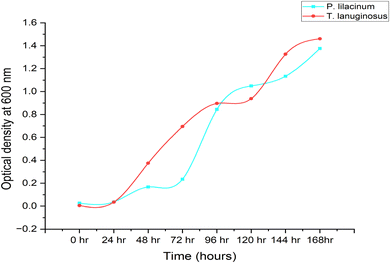 |
| Fig. 2 Plot for the growth pattern of the fungal strains. | |
Table 2 Absorbance and dry cell biomass of P. lilacinum and T. lanuginosus
Time (in hours) |
P. lilacinum
|
T. lanuginosus
|
OD |
Dry biomass (g l−1) |
OD |
Dry biomass (g l−1) |
0 |
0.026 |
0 |
0.005 |
0 |
12 |
0.032 |
46 |
0.017 |
38 |
24 |
0.037 |
70 |
0.035 |
60 |
36 |
0.101 |
72 |
0.179 |
72 |
48 |
0.167 |
74 |
0.375 |
80 |
60 |
0.194 |
77 |
0.561 |
93 |
72 |
0.235 |
80 |
0.695 |
110 |
96 |
0.844 |
60 |
0.896 |
80 |
120 |
1.049 |
40 |
0.938 |
50 |
144 |
1.134 |
20 |
1.326 |
30 |
168 |
1.376 |
20 |
1.461 |
30 |
A key determinant of the concentration of fungal biomass is its dry weight. It's interesting to note that on the 3rd day, both Purpureocillium lilacinum and Thermomyces lanuginosus showed a much greater biomass dry weight. The stationary phase was then followed by the fungus. P. lilacinum and T. lanuginosus had maximum biomass concentrations of 80 g L−1 and 110 g L−1, respectively. The particular growth rates for P. lilacinum and T. lanuginosus shown in Fig. 3 and 4 showed a decline on a daily basis, mostly because of nutrient depletion. The maximum specific growth rates (μmax) for the strains P. lilacinum and T. lanuginosus were 1.34 ± 0.024 and 3.09 ± 0.019 L−1 d−1, respectively.
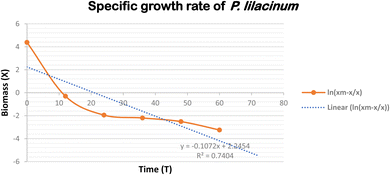 |
| Fig. 3 Graph depicting the specific growth rate of P. lilacinum. | |
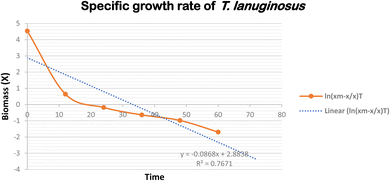 |
| Fig. 4 Graph depicting the specific growth rate of T. lanuginosus. | |
It is important to keep in mind that the maximal biomass and growth rate are both dependent on the doubling time of the fungus as well as the accessibility of key nutrients. When determining a fungal population's growth rate and rate of reproduction, doubling time is a critical quantity to consider. This term refers to the amount of time needed for the population of a fungus to increase by a factor of two. When researching the development dynamics and productivity of fungal cultures, the doubling time is a crucial measure. The doubling times of P. lilacinum and T. lanuginosus when they were grown in YPD medium were determined to be 0.517 ± 0.006 and 0.224 ± 0.006 day−1 respectively.
3.2 DNA content analysis after decellularization
Divalent saline solution, which may be used to separate out salt residue from solution/bracket precipitates, eliminates leftover SDS. Additionally, desalination residue and/or SDS micelles can be removed using dH2O, acetic acid, dimethylsulfoxide (DMSO), or ultrasonic treatment. MgCl2 and CaCl2 are two examples of the divalent salts that might be present in the divalent saline solution. The following criteria are given for use in evaluating how well various components may be removed from the system. The decellularized extracellular matrix (ECM) has to have: (1) fewer than 50 ng of double-stranded DNA (dsDNA) per mg of ECM dry weight; (2) less than 200 bp of DNA fragment length; and (3) no apparent nuclear material when stained with 4′,6-diamidino-2-phenylindole (DAPI).39 The amount of DNA that was released from the cells after they were decellularized was measured using a nanodrop spectrophotometer at regular intervals of 15 minutes over a period of four hours (Fig. 5). Under conditions of pH 7 and 12, and sonicated SDS, both types of material demonstrated a decellularization process that was approximatively exponential. The lowest degree of decellularization was seen for T. lanuginosus in a pH 7 solution, whereas the pH 12 solution gave the lowest degree of decellularization for P. lilacinum. Additionally, the maximum degree of decellularization was observed in sonicated SDS for both of these materials.
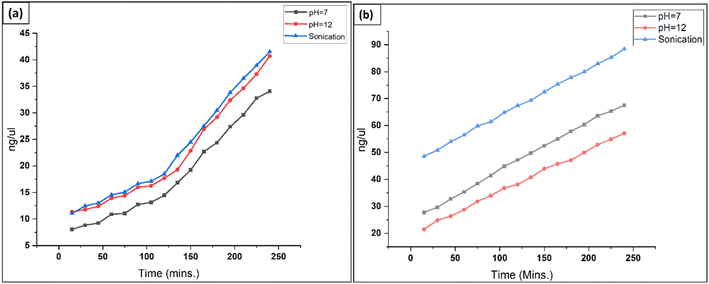 |
| Fig. 5 Graph depicting increased cellular contents in SDS solution over time and under different conditions: (a) T. lanuginosus, (b) P. lilacinum. | |
3.3 Characterization
3.3.1 Fourier transform infrared spectroscopy (FTIR).
FTIR is an effective method for detecting structural alterations in compounds brought on by changes to functional groups, linkages and bonds. Typically distinct, a compound's fingerprint may be used to distinguish between various types of compounds. FTIR spectroscopy was used to examine the chemical composition and structural characteristics of biomaterials. The FTIR spectra were acquired for materials in the range of 400–4000 cm−1 designed using fungal species P. lilacinum and T. lanuginosus. The findings shed light on the functional groups and molecular bonds that are present in these materials. The molecular structure of the biomaterials could potentially be better understood by analysing their FTIR spectra. For instance, the high peaks at 2916.11 cm−1 in the P. lilacinum spectrum show the existence of –CH2– stretching vibrations, indicating the presence of long hydrocarbon chains. The peaks at 1653.51 cm−1 and 1386.60 cm−1 are the stretching vibrations of C
N and –CH, respectively. Strong S
O stretching is also seen from the spectral peak at 1031.49 cm−1, which signifies the presence of sulfoxide in the material. The FTIR spectra of the T. lanuginosus biomaterial contain a variety of spectral peaks, each of which is associated with a specific wavenumber. These spectral peaks include the following: 3257.29 (strong O–H stretching), 2920.75 (strong O–H stretching/weak O–H stretching/medium C–H stretching), and 1607.09 cm−1 (medium C
C stretching/medium N–H bending/strong C
C stretching). The P. lilacinum biomaterial's FTIR spectra include a variety of spectral peaks with associated wavenumbers, including those at 3282.82 (strong O–H stretching), 2916.11 (C–H stretching), 1653.51 (C
N stretching/C
C stretching), 1386.60 (C–H bending/S
O stretching/S
O stretching), and 1031.49 cm−1 (strong S
O stretching) (Fig. 6).
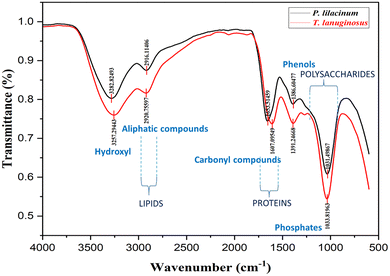 |
| Fig. 6 FTIR spectra for biomaterial samples generated from P. lilacinum and T. lanuginosus. | |
3.3.2 SEM image analysis and elemental composition.
The mycelium sheet is likely coated with cell detritus, as can be seen in Fig. 6(a) and (b) from SEM images. Both strains, however, allow for the visualization of the hyphae. P. lilacinum films have a special kind of compressed filament, as seen in Fig. 7(a). Here, it is evident that the feeding substrates have an impact on the filament's width. The hyphae are mechanically supported by internal hydrostatic pressure (turgor), which also promotes hyphal development by producing a mass movement of the cytoplasm to the hyphal ends. Due to internal hydrostatic pressure, the cell membrane prevents hyphal osmotic lysis. Since the filaments of T. lanuginosus are substantially smaller, any treatment will have a lower impact on their structure. Particularly, films of T. lanuginosus during its growth phase display a thread-like structure (Fig. 7(b)). During the early stages of development, short, heavily entangled tubes are more frequent, but later on, compact filaments become more prevalent.
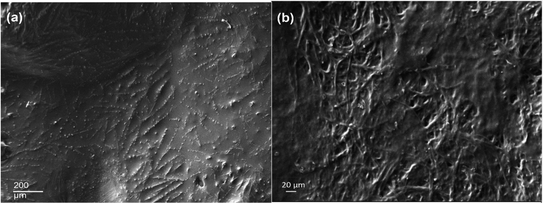 |
| Fig. 7 SEM image of (a) P. lilacinum and (b) T. lanuginosus. | |
As determined by EDS, the predominant elements in the P. lilacinum based biomaterial samples were potassium, oxygen, chlorine, and carbon in various compounds. Smaller amounts of the elements sodium, aluminium, and sulfur were observed. For T. lanuginosus based biomaterial samples the predominant elements were determined to be oxygen, chlorine, potassium and carbon. We found lower concentrations of sodium, phosphorus, and sulfur. Aluminum and phosphorus were the trace elements that were found; they were only detected in a small number of samples.
3.4 Porosity via SEM analysis
The morphological analysis of the decellularized P. lilacinum and T. lanuginosus based biomaterials was done by using the ImageJ software as mentioned earlier. By using the software, the pore size and porosity of the sample were determined. The average pore size of the P. lilacinum decellularized sample was 7.884 μm and the average porosity was 0.101%. For sample T. lanuginosus the average pore size was 109.676 μm and the average porosity was 20.023%. Thus, the biomaterial prepared from T. lanuginosus was determined to be of more porous nature. The segmented image in “ImageJ” for analysis is shown in Fig. 8.
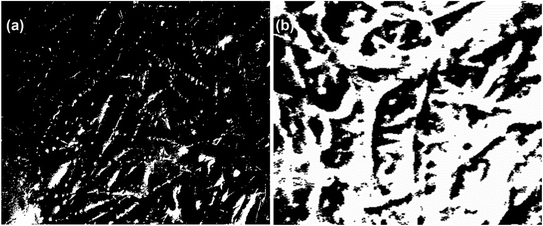 |
| Fig. 8 (a) Segmented image of P. lilacinum obtained from ImageJ. (b) Segmented image of T. lanuginosus obtained from ImageJ. | |
3.5 Swelling ratio analysis
Depending on the particular kind of material and its composition, the swelling ratio of fungal materials may change. The swelling ratio of fungal compounds tends to be rather high. This is because of their ability to absorb and hold moisture owing to their hygroscopic nature. The kind of fungus, the chemical makeup of the substance, and the surrounding environment all affect how much swelling develops. For P. lilacinum, the swelling ratio percentages were estimated to be, respectively, 33.99% with water and 52.60% with 1× PBS solution. According to calculations, T. lanuginosus's swelling ratio percentages with water and 1× PBS were 39.75% and 50.85%, respectively. Due to its great affinity for absorbing water, porous structure, larger amount of cellulose and hemicellulose in their cell walls, and structural integrity, the latter exhibits a higher swelling ratio with water. It is important to remember that variables other than the characteristics of the fungus itself might affect the swelling ratio of fungal materials. Temperature, pH, moisture content, and processing methods are all variables that might affect how fungal compounds expand (Fig. 9).
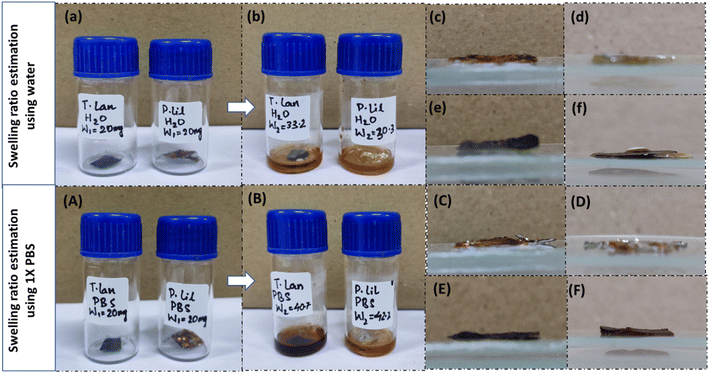 |
| Fig. 9 (a) Dried and weighed samples of the biomaterials. (b) Samples immersed in distilled water. (c) Side view of the dried P. lilacinum sample. (d) Side view of the water swelled P. lilacinum sample. (e) Side view of the dried T. lanuginosus sample. (f) Side view of the water swelled T. lanuginosus sample. (A) Dried and weighed samples of the biomaterials. (B) Samples immersed in 1× PBS solution. (C) Side view of the dried P. lilacinum sample. (D) Side view of the PBS swelled P. lilacinum sample. (E) Side view of the dried T. lanuginosus sample. (F) Side view of the PBS swelled T. lanuginosus sample. | |
3.6 Hydrodynamic characterization
Insightful information on the behaviour, structure, and characteristics of fungal biomaterials in various fluid environments is provided through hydrodynamic characterization methods. Using ImageJ software, the hydrodynamic properties of the materials were ascertained after calculating the contact angle of water droplets with the biomaterials' surfaces. For P. lilacinum and T. lanuginosus, the contact angles were found to be 147.3 ± 5.7° [Fig. 10(a)] and 143 ± 7.6° [Fig. 10(b)], respectively. With respect to the contact angles shown by the materials, they were determined to be hydrophobic in nature. Assessment of the stability and aggregation propensities of fungal biomaterials is aided by hydrodynamic characterization. This information is crucial for creating stable formulations and understanding the processes of aggregation or destabilization in varied settings. This feature is advantageous for bioplastics because it offers mechanical strength,40 water absorption capacity, and appropriateness for environmentally friendly applications such as food packaging.41
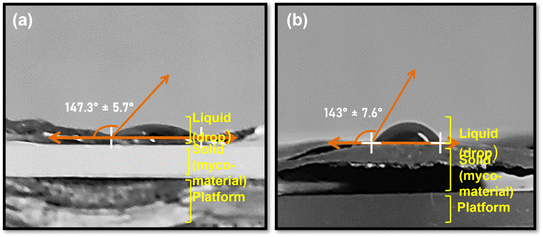 |
| Fig. 10 Contact angle measurement: (a) P. lilacinum, 147.3 ± 5.7° and (b) T. lanuginosus, 143 ± 7.6°. | |
4. Application of mycelium-based materials
Fungal biomaterials offer an environmentally significant alternative to conventional materials, contributing to resource conservation, reduced pollution, and a more sustainable approach to material production and consumption. Importantly, this study reveals a low-cost, environmentally favourable method for producing biocompatible materials that might be used to produce stronger, more robust, and eco-friendly construction materials. The biomaterial produced through our research has the potential to serve as a foundational concept for a material utilized in the construction industry, such as a restorative material for fractured concrete structures or the design of entire building structures. Several studies and examples pertaining to construction materials derived from mycelium are provided herein.
An emerging field that is garnering increasing interest is the utilization of fungi as building materials. Numerous articles have examined various advancements concerning the application of fungal materials in the field of architecture, which have significantly transformed our understanding of material utilization.42 Compared to conventional materials, mycelium-based products have a number of benefits, such as being less expensive, biodegradable, and having a lower density and smaller environmental effect. For particular purposes, a wide variety of substrates may be used in conjunction with carefully regulated processing methods to produce materials obtained from mycelium that have the required structure and functionality. Due to cracking, concrete, a crucial element of the built environment, has problems with stability and longevity. Concrete may fracture due to a variety of physical, chemical, and biological factors, such as freeze–thaw cycles, overload stress, physico-chemical weathering, and bio-weathering.43–45 Water and oxygen increase corrosion when the passivation layer has deteriorated. Coating may be thought of as a preventive technique utilized before any cracks emerge and would function as a barrier to guard against liquids and gases as opposed to restorative procedures.46 Adding admixtures or reducing the water to cement ratio, surface treatment is a practical and economical solution to enhance the quality of the concrete's surface layer while safeguarding the structure.47,48 The mechanical properties of mycelial composites support compression-based structural designs. Using mycelium-based building materials makes novel technical, aesthetic, and sustainable construction approaches conceivable. For instance, one bio-composite, a mycelium brick, is composed of mycelium and agricultural waste and is capable of forming a three-dimensional structure. Such bricks may be employed as construction materials that are both robustly flexible and fire-resistant.
A review of patent documents reveals that bio-foam composites and intricate structures comprised of mycelium-based composites may be utilized as structural materials, interior finishing materials, components for mobile home furnishings, and packaging materials in the construction of buildings. Their low thermal conductivity makes them suitable for sound absorption or insulation applications. Applications within the construction industry are primarily limited to impermeable thermal and acoustic insulators.49
While myco-materials are a relatively new technology, there is a dearth of understanding about their potential. Many individuals, including architects, engineers, and builders, are just unaware of the potential that they possess. The fact that myco-materials may be more costly than conventional concrete, despite the fact that the cost is decreasing as the technology advances, is one of the reasons why they are not very popular among the general public. Although there is some question about the long-term performance of myco-materials, such as their resistance to fire and durability, there is still some uncertainty. Due to the fact that the manufacture of building materials based on mycelium is currently restricted, it may be challenging to use these materials for construction projects of a significant size. It is anticipated that myco-materials will gain more widespread acceptance in the years to come as a result of the efforts of researchers who are striving to overcome these problems. Nevertheless, it will take some time for the building sector to completely accept this technology when it has been introduced.
5. Conclusion and future perspective
Within the scope of this investigation, we have proposed a method for the cultivation and harvesting of the fungal mycelium as well as the molding of this mycelium into a scaffold. The purpose of this investigation was to offer a method for the cultivation and harvesting of the fungal mycelium as well as the formation of a scaffold using these materials. Growth dynamics for two fungal species, namely T. lanuginosus and P. lilacinum, were investigated; they exhibit exponential growth during the first seven days after they are cultured. T. lanuginosus has a faster growth rate than P. lilacinum. P. lilacinum shows the presence of vibrations that are characteristic of hydroxyl and alkyl groups, imine or oxime compounds or alkene compounds, a mixture of carbon–hydrogen bonds and sulphate groups or sulfonyl chloride groups in the sample. T. lanuginosus shows the presence of functional groups such as hydroxyl groups, additional hydroxyl groups, alkyl groups, alkenes, and amines. It can be concluded after seeing the porosity and swelling ratio (%) that the P. lilacinum sample (average porosity: 0.101%; swelling ratio 15.75% with water and 25.44% with 1× PBS solution) would form a less advantageous material for applications such as drug release, whereas the materials prepared using T. lanuginosus (average porosity: 20.023%; swelling ratio 69.18% with water and 61.76% with PBS) can aid in maintaining a moist environment conducive to tissue regeneration, as well as controlled drug, fertiliser or pesticide release. Decellularized tissues may also be used as bio-scaffolds for cell-based therapeutics, disease modelling, and drug testing. The presence of a wide variety of chemical functionalities on their surface makes mycelia an interesting platform for tissue engineering applications. Cells preferably attach onto carbohydrate and protein moieties, namely by interacting with the numerous proteoglycans and proteins present in the extracellular matrix (ECM). Therefore, the observed wide variety of chemical functionalities on the fungal surface indicates a promising path towards the development of fully fungal-based biomaterials.
However, the potential applications of myco-materials go far beyond the realm of building materials. Because the mycelium has a naturally fibrous structure, it is a good option for the creation of scaffolds that may be used in tissue engineering and drug delivery applications. The idea of harnessing mesenchymal stem cells to build new tissues, transport medicine directly to specific locations of the body, or even construct implanted devices is among the possibilities that researchers are investigating. The resilience of myco-materials to fire is now a barrier that prevents their broad implementation in the building industry. In the future, research may concentrate on the creation of fire-retardant additives or treatments for the mycelium itself, or it might examine the possibility of designing the material to have qualities that are naturally resistant to fire. In addition, research directed towards enhancing the mechanical strength, durability, and water resistance of myco-materials would further expand the usefulness of these materials. The mycelium has an innate capacity to heal itself on its own. It is possible that in the future, research may investigate methods to make use of this capability to produce self-healing bioconcrete. This has the potential to result in structures that are able to heal cracks or small damage on their own, hence lowering the amount of money spent on maintenance and prolonging the lifetime of the building. It may be possible to include sensors into myco-materials so that they can keep track of their own health and performance. The early discovery of issues would be made possible as a result of this, which might assist in the prevention of catastrophic failures. It is possible to widen the scope of study on myco-materials in order to investigate mycelium-based composites for purposes other than structural building. For example, the development of bio-packaging materials, components for furniture, or even automobile parts derived from the mycelium might fall under this category. It is possible for scientists and engineers to continue the development of myco-materials and unleash their full potential as a material that is both sustainable and adaptable for the future if they investigate these and other prospective research avenues.
Data availability
Data for this article including analytical observations are available in the tables and figures submitted along with the manuscript.
Conflicts of interest
The authors declare that they have no known competing financial interests or personal relationships that could have appeared to influence the work reported in this paper.
Acknowledgements
We acknowledge the National Institute of Technology Raipur for Seed Grant, Project No: NITRR/Seed Grant/2021-22/30. We gratefully accepted the research support of Science & Engineering Research Board (SERB) vide grant number SRG/2022/000348 from the Department of Science and Technology, India.
References
- H. A. B. Wösten, Filamentous fungi for the production of enzymes, chemicals and materials, Curr. Opin. Biotechnol., 2019, 59, 65–70 CrossRef PubMed.
- M. E. Antinori, L. Ceseracciu, G. Mancini, J. A. Heredia-Guerrero and A. Athanassiou, Fine-Tuning of Physicochemical Properties and Growth Dynamics of Mycelium-Based Materials, ACS Appl. Bio Mater., 2020, 3(2), 1044–1051, DOI:10.1021/acsabm.9b01031.
- M. E. Antinori, M. Contardi, G. Suarato, A. Armirotti, R. Bertorelli and G. Mancini,
et al., Advanced mycelium materials as potential self-growing biomedical scaffolds, Sci. Rep., 2021, 11(1), 12630 CrossRef CAS PubMed.
- E. Soh, Z. Y. Chew, N. Saeidi, A. Javadian, D. Hebel and H. Le Ferrand, Development of an extrudable paste to build mycelium-bound composites, Mater. Des., 2020, 195, 109058 CrossRef CAS.
- M. Niaounakis, Recycling of biopolymers – The patent perspective, Eur. Polym. J., 2019, 114, 464–475 CrossRef CAS.
- M. Hong and E. Y. X. Chen, Completely recyclable biopolymers with linear and cyclic topologies via ring-opening polymerization of γ-butyrolactone, Nat. Chem., 2016, 8(1), 42–49 CrossRef CAS PubMed.
- P. Mohamad and I. T. M. Juki, The use of Fungus as CO2 Sequestration: A Systematic Review, Recent Trends Civ. Eng. Built. Environ., 2021, 3(1), 631–640 Search PubMed . Available from: https://publisher.uthm.edu.my/periodicals/index.php/rtcebe/article/view/3237.
- M. Ruggeri, D. Miele, M. Contardi, B. Vigani, C. Boselli and A. Icaro Cornaglia,
et al., Mycelium-based biomaterials as smart devices for skin wound healing, Front. Bioeng. Biotechnol., 2023, 11, 1–10 Search PubMed.
- M. Haneef, L. Ceseracciu, C. Canale, I. S. Bayer, J. A. Heredia-Guerrero and A. Athanassiou, Advanced Materials From Fungal Mycelium: Fabrication and Tuning of Physical Properties, Sci. Rep., 2017, 7(1), 41292 CrossRef CAS PubMed.
- J. Luangsa-ard, J. Houbraken, T. van Doorn, S. B. Hong, A. M. Borman and N. L. Hywel-Jones,
et al., Purpureocillium, a new genus for the medically important Paecilomyces lilacinus, FEMS Microbiol. Lett., 2011, 321(2), 141–149 CrossRef CAS PubMed.
- M. I. Hamid, M. Hussain, Y. Wu, X. Zhang, M. Xiang and X. Liu, Successive soybean-monoculture cropping assembles rhizosphere microbial communities for the soil suppression of soybean cyst nematode, FEMS Microbiol. Ecol., 2017, 93(1), fiw222 CrossRef PubMed.
- S. D. Silva, R. M. D. G. Carneiro, M. Faria, D. A. Souza, R. G. Monnerat and R. B. Lopes, Evaluation of Pochonia chlamydosporia and Purpureocillium lilacinum for Suppression of Meloidogyne enterolobii on Tomato and Banana, J. Nematol., 2017, 49(1), 77–85 CrossRef CAS PubMed.
- S. Singh and B. K. Bajaj, Potential application spectrum of microbial proteases for clean and green industrial production, Energy Ecol. Environ., 2017, 2(6), 370–386 CrossRef.
- V. M. Corbu, I. Gheorghe-Barbu, D. A. Ştefania, C. O. Vrâncianu and T. E. Şesan, Current Insights in Fungal Importance—A Comprehensive Review, Microorganisms, 2023, 11(6), 1384 CrossRef CAS PubMed.
- A. Moreno-Gavíra, V. Huertas, F. Diánez, B. Sánchez-Montesinos and M. Santos, Paecilomyces and Its Importance in the Biological Control of Agricultural Pests and Diseases, Plants, 2020, 9(12), 1746 CrossRef PubMed.
- N. P. Mchunu, K. Permaul, A. Y. Abdul Rahman, J. A. Saito, S. Singh and M. Alam, Xylanase Superproducer: Genome Sequence of a Compost-Loving Thermophilic Fungus, Thermomyces lanuginosus Strain SSBP, Genome Announc., 2013, 1(3), 1–2 Search PubMed.
- M. V. Novas and C. C. Carmarán, Studies on diversity of foliar fungal endophytes of naturalised trees from Argentina, with a description of Haplotrichum minutissimum sp, Nov. Flora:
Morphol. Distrib. Funct. Ecol. Plants, 2008, 203(7), 610–616 CrossRef.
- S. Singh, A. M. Madlala and B. A. Prior, Thermomyces lanuginosus : properties of strains and their hemicellulases, FEMS Microbiol. Rev., 2003, 27(1), 3–16 CrossRef CAS PubMed.
- S. Singh, A. M. Madlala and B. A. Prior, Thermomyces lanuginosus : properties of strains and their hemicellulases, FEMS Microbiol. Rev., 2003, 27(1), 3–16 CrossRef CAS PubMed.
- F. J. Contesini, M. G. Davanço, G. P. Borin, K. G. Vanegas, J. P. G. Cirino and R. R. d. Melo,
et al., Advances in Recombinant Lipases: Production, Engineering, Immobilization and Application in the Pharmaceutical Industry, Catalysts, 2020, 10(9), 1032 CrossRef CAS.
- C. Chen, W. Ding, H. Zhang, L. Zhang, Y. Huang, M. Fan, J. Yang and D. Sun, Bacterial cellulose-based biomaterials: From fabrication to application, Carbohydr. Polym., 2022, 278(0144-8617), 118995 CrossRef CAS PubMed.
- F. Jabbari, V. Babaeipour and S. Bakhtiari, Bacterial cellulose-based composites for nerve tissue engineering, Int. J. Biol. Macromol., 2022, 217(0141-8130), 120–130 CrossRef CAS PubMed.
-
S. U. Tekale, A. B. Kanagare, A. V. Dhirbassi, A. J. Domb and R. P. Pawar, Polysaccharide-based Biomaterials: Overview, Polysaccharide-based Biomaterials: Delivery of Therapeutics and Biomedical Applications, The Royal Society of Chemistry, 2022, pp. 1–26 Search PubMed.
- A. Chaturvedi, N. Zhang, A. Challa and T. Thontepu, Synthesis of a Yeast-Derived Collagen. 10.21203/rs.3.rs-1840394/v1, J. Biotechnol. Biomater., 2022 DOI:10.21203/rs.3.rs-1840394/v1..
-
K. Iqbal, A. Chaudhary, S. Sharma, A. Varma, I. S. Thakur and A. Mishra, Algae-based biomaterials for biomedicines, Biomass, Biofuels, and Biochemicals, ed. H. Ngo, W. Guo, A. Pandey, J.-S. Chang, and D.-J. Lee, Elsevier, 2022, pp. 251–276 Search PubMed.
- A. A. Baba, A. K. Das and A. Gupta, Efficacies of four plant-based biomaterials in removal of Pb (II) from aqueous solution, Arabian J. Geosci., 2022, 15 DOI:10.1007/s12517-022-09904-8.
- R. Tarrahi, A. Khataee, A. Karimi and Y. Yeojoon, The latest achievements in plant cellulose-based biomaterials for tissue engineering focusing on skin repair, Chemosphere, 2022, 288(0045-6535) DOI:10.1016/j.chemosphere.2021.132529..
- C. Lujerdean, G.-M. Baci, A.-A. Cucu and D. S. Dezmirean, The Contribution of Silk Fibroin in Biomedical Engineering, Insects, 2022, 13(3) DOI:10.3390/insects13030286.
- S. M. Letcher, N. R. Rubio, R. N. Ashizawa, M. K. Saad, M. L. Rittenberg, A. McCreary, A. Ali, O. P. Calkins, B. A. Trimmer and D. L. Kaplan,
In vitro Insect Fat Cultivation for Cellular Agriculture Applications, ACS Biomater. Sci. Eng., 2022, 8(9) DOI:10.1021/acsbiomaterials.2c00093.
- A. Rahman and T. H. Silva, Collagens from Marine Organisms towards Biomedical Applications, Mar. Drugs, 2022, 20(3) DOI:10.3390/md20030170.
- G. Nayak, A. Sahu, S. Bhuyan and D. Kar, Review on Biomedical Applications of Marine Algae-Derived Biomaterials, Univers. J. Public Health, 2022, 10(1), 15–24, DOI:10.13189/ujph.2022.100102.
- M. S. Hasanin, Cellulose-Based Biomaterials: Chemistry and Biomedical Applications, Starch, 2022 DOI:10.1002/star.202200060.
- Z. U. Arif, M. Y. Khalid, R. Noroozi, M. Hossain, H. H. Shi, A. Tariq, R. Seeram and R. Umer, Additive manufacturing of sustainable biomaterials for biomedical applications, Asian J. Pharm. Sci., 2023, 18(3) DOI:10.1016/j.ajps.2023.100812.
- L. Qin, L. Liu, Z. Wang, W. Chen and D. Wei, The mixed culture of microalgae Chlorella pyrenoidosa and yeast Yarrowia lipolytica for microbial biomass production, Bioprocess Biosyst. Eng., 2019, 42(9), 1409–1419 CrossRef CAS PubMed.
- M. Huesemann, B. Crowe, P. Waller, A. Chavis, S. Hobbs and S. Edmundson,
et al., A validated model to predict microalgae growth in outdoor pond cultures subjected to fluctuating light intensities and water temperatures, Algal Res., 2016, 13, 195–206 CrossRef.
- Q. L. Loh and C. Choong, Three-Dimensional Scaffolds for Tissue Engineering Applications: Role of Porosity and Pore Size, Tissue Eng., Part B, 2013, 19(6), 485–502 CrossRef CAS PubMed.
- Z. Cai and J. Kim, Preparation and Characterization of Novel Bacterial Cellulose/Gelatin Scaffold for Tissue Regeneration Using Bacterial Cellulose Hydrogel, J. Nanotechnol. Eng. Med., 2010, 1(2), 1–6 Search PubMed.
- K. Y. Law, Definitions for Hydrophilicity, Hydrophobicity, and Superhydrophobicity: Getting the Basics Right, J. Phys. Chem. Lett., 2014, 5(4), 686–688 CrossRef CAS PubMed.
- A. Gilpin and Y. Yang, Decellularization Strategies for Regenerative Medicine: From Processing Techniques to Applications, BioMed Res. Int., 2017, 2017, 1–13 CrossRef PubMed.
- S. G. Jin, A. M. Yousaf, K. S. Kim, D. W. Kim, D. S. Kim and J. K. Kim,
et al., Influence of hydrophilic polymers on functional properties and wound healing efficacy of hydrocolloid based wound dressings, Int. J. Pharm., 2016, 501(1–2), 160–166 CrossRef CAS PubMed.
- S. Guzman-Puyol, G. Tedeschi, L. Goldoni, J. J. Benítez, L. Ceseracciu and A. Koschella,
et al., Greaseproof, hydrophobic, and biodegradable food packaging bioplastics from C6-fluorinated cellulose esters, Food Hydrocolloids, 2022, 128, 107562 CrossRef CAS.
- D. Almpani-Lekka, S. Pfeiffer, C. Schmidts and S. Seo, A review on architecture with fungal biomaterials: the desired and the feasible, Fungal Biol. Biotechnol., 2021, 8(1), 17 CrossRef PubMed.
- T. Siegmund, A numerical study of transient fatigue crack growth by use of an irreversible cohesive zone model, Int. J. Fatigue, 2004, 26(9), 929–939 CrossRef.
- H. S. Shang and Y. P. Song, Experimental study of strength and deformation of plain concrete under biaxial compression after freezing and thawing cycles, Cem. Concr. Res., 2006, 36(10), 1857–1864 CrossRef CAS.
- M. Fomina, V. S. Podgorsky, S. V. Olishevska, V. M. Kadoshnikov, I. R. Pisanska and S. Hillier,
et al., Fungal Deterioration of Barrier Concrete used in Nuclear Waste Disposal, Geomicrobiol. J., 2007, 24(7–8), 643–653 CrossRef CAS.
- G. M. Gadd and T. D. Dyer, Bioprotection of the built environment and cultural heritage, Microb. Biotechnol., 2017, 10(5), 1152–1156 CrossRef PubMed.
- H. W. Song, C. H. Lee and K. Y. Ann, Factors influencing chloride transport in concrete structures exposed to marine environments, Cem. Concr. Compos., 2008, 30(2), 113–121 CrossRef CAS.
- P. A. M. Basheer, L. Basheer, D. J. Cleland and A. E. Long, Surface treatments for concrete: assessment methods and reported performance, Constr. Build. Mater., 1997, 11(7–8), 413–429 CrossRef.
- M. Sydor, A. Bonenberg, B. Doczekalska and G. Cofta, Mycelium-Based Composites in Art, Architecture, and Interior Design: A Review, Polymers, 2021, 14(1), 145 CrossRef PubMed.
|
This journal is © The Royal Society of Chemistry 2024 |
Click here to see how this site uses Cookies. View our privacy policy here.