Fabrication of a novel palladium membrane sensor for its determination in environmental and biological samples
Received
15th February 2024
, Accepted 9th April 2024
First published on 13th April 2024
Abstract
A novel sensitive, specific, and reversible optical sensor for the palladium(II) ion was created by impregnating an agarose membrane with 4-(2-amino-3-hydroxypyridine-4-ylazo)1,5-dimethyl-2-phenyl-1,2-dihydropyrazol-3-one (AHDDO). Spectrophotometric studies of complex formation between the AHDDO base ligand and Mn2+, Cd2+, Co2+ Hg2+, Zn2+, Cu2+, Pd2+, Sr2+, Al3+, Fe3+, Au3+, and Ag+ metal ions in an ethanolic solution indicated a substantially larger stability for the palladium ion complex. Therefore, the AHDDO was immobilized on a clear agarose film and used as a suitable ionophore for building a selective Pd2+ optical sensor. By combining the sensing membrane with Pd2+ ions at pH 5.75, a transparent color change from orange to violet was observed. On the immobilization of AHDDO, the effects of ionophore concentration, pH, temperature, stirring, and reaction time were investigated. A linear relationship was observed between the membrane absorbance at 633 nm and Pd2+ concentrations in a range from 15 to 225 ng mL−1 with detection (3σ) and quantification (10σ) limits of 4.25 and 14.25 ng mL−1, respectively. For the determination of Pd2+ ions, no significant interference from at least 400-fold excess concentrations of a number of possibly interfering ions was found. The sensor exhibits remarkable selectivity for Pd2+ ions and can be regenerated through exposure to 0.15 M HNO3. The sensor has been successfully used to find palladium in biological, soil, road, and water samples.
Environmental significance
In the present study, a highly selective optical sensor for the measurement of Pd2+ was created by immobilizing 4-(2-amino-3-hydroxypyridine-4-ylazo)1,5-dimethyl-2-phenyl-1,2-dihydropyrazol-3-one (AHDDO) on an agarose membrane. The proposed sensor has excellent optical properties that make it suitable for use as an optical sensor, including good selectivity, low cost, appropriate lifetime, rapid and reproducible regeneration, and ease of manufacture and handling. The sensor also demonstrated a rapid response time, a suitable linear calibration curve, and low detection and quantification limits. By means of an easy and low-cost methodology, satisfactory experimental results were achieved for the determination of Pd2+ ions by the prepared sensor. Without any significant interference from other metal ions, the suggested sensor can be used for the quantitative and qualitative measurement of Pd2+ ions in various real samples.
|
1 Introduction
Noble metals, particularly palladium (Pd), are widely used in the electrical industry.1 Palladium's significance has expanded in recent years as new applications for dentistry, medical equipment, jewelry, and vehicle catalytic converters have emerged.2 Despite the evident benefits of automobile catalysts, palladium emissions into the environment are linked to the production and recovery of emissions controls in the manufacturing industries, as well as the functioning of car catalysts. Precious metals with unique physical and chemical properties are significant materials that have recently seen widespread application in industry and technology.3,4
Palladium is widely known for its capacity to treat viral infections (leukemia and AIDS) as well as its anti-cancer activity.5 Certain palladium compounds have been described as a potential threat to people, causing asthma, allergies, rhino conjunctivitis, and other major health problems, and are easily transmitted to biological material and eventually amplified along the food chain.6,7 Palladium salts have been proven to be highly toxic to aquatic plants even at low concentrations.8 According to a World Health Organization report,9 data on Pd2+ losses from automobile exhaust emission control catalysts is currently sparse.
Palladium monitoring in environmental samples is a crucial issue for assessing the dangers to the ecosystem and human health that may arise in the future due to its broad spectrum of applications.10 Concentrations of palladium in surface fresh waters and surface salt waters were found to be in the range of 0.4 to 22 ng L−1 and 19 to 70 ng L−1, respectively whereas in the dense traffic road soil samples, the concentration of palladium was found to be in the range of less than 0.7 to 47 μg kg−1. Palladium exposures are thought to pose a number of potential dangers to both human health and the environment, according to the World Health Organization. Palladium appears to be consumed by humans in diets up to 2.0 μg per day on average.11 A significant field of research now involves quantitative techniques for detecting Pd(II) and other contaminants in industrial, environmental, geophysical, and biological samples.10–13 Even while modern technology makes it feasible to detect analytes at extremely low concentrations, most samples contain far less palladium than would be necessary to make a direct determination. Consequently, before making the actual determination, proper enrichment procedures are necessary.14
To date, different kinds of conventional analytical techniques like atomic absorption spectroscopy (AAS),15 flame atomic absorption spectroscopy,16 electro-thermal atomic absorption spectroscopy,17 inductively coupled plasma mass spectrometry (ICP-MS),18,19 inductively coupled plasma atomic emission spectroscopy (ICP-AES),20 X-ray fluorescence (XRF),21 high performance liquid chromatography (HPLC),22 electrochemical methods23 and voltammetry24 have been widely adopted for quantitative and qualitative analysis of palladium. However, their affordability and effectiveness in detecting palladium are limited by high equipment costs and time-consuming sample pre-treatments. When Pd(II) is present in environmental samples, its low concentration (at μg L−1) combined with the high concentration of interfering matrix components frequently necessitates a matrix separation step accompanied by an enrichment step. This allows for an accurate and precise determination of Pd(II) in samples with very low analyte contents.25 As a result, there is a great need for designing and building novel instruments for the detection and identification of diverse contaminants in the environment.26–34
Optical chemical sensors (optodes), which are cost-effective and selective tools for determining the presence of heavy metal ions, have undergone significant development in recent years.32–36 To provide straightforward and quick measurement methods with improved selectivity and low detection limits, optodes are typically employed in conjunction with low-cost spectrophotometric techniques.37,38 One of the critical steps for building optical sensors is immobilization ionophores on transparent membranes. The ionophore immobilized is accomplished by either physical entrapment,39 sol–gel,40 multilayered films41 or chemical (covalent) bonding methods.42 On the other hand, covalently bonded sensors have reproducible responses and lengthy lifetimes.43 Different approaches to covalent immobilization can be found in the literature.44 Ionophores are essential components in the fabrication of optical sensors. Because azo dye ligands can create stable complexes with transition metal ions, they are commonly utilized as ionophores in the development of membrane sensors. For a particular ion, they exhibit extraordinary selectivity, sensitivity, and stability.45
The most widely used techniques are spectrophotometric ones, which are still very popular. Spectrophotometric techniques are appealing due to the instrumentation's widespread availability, the procedures' simplicity, and the technique's speed, precision, and accuracy. Optical sensors, which are crucial for biological imaging, provide relatively straightforward, sensitive, and affordable detection techniques for analytes of interest. As a result, numerous optical sensors for the colorimetric and fluorometric detection of palladium have been developed.46–50
Agarose is a strongly hydrophilic, chemically inert and microbiologically resistant material.51 It is quite stable in gel form and is the most widely used support in affinity chromatography.52 Chemical compounds containing primary aliphatic or aromatic amines can be covalently bonded to activated agarose beads. Agarose has been used for manufacturing of an optical fiber humidity sensor.53 Compared to PVC the use of hydrophilic agarose as a support for the fabrication of covalently immobilized optical sensors has the advantage of being easily manufactured and handled. According to our review of the literature, no species has yet used 4-(2-amino-3-hydroxypyridine-4-ylazo)1,5-dimethyl-2-phenyl-1,2-dihydropyrazol-3-one (AHDDO) as a sensing agent. In order to build a selective optical sensor for the spectrophotometric detection of Pd2+ in aqueous real sample solutions, AHDDO is covalently immobilized on an agarose membrane and used as an efficient ionophore with N and O donor atoms.
2 Materials and methods
2.1 Instrumentation
An Orion research model 601 A/digital ionalyzer pH meter was used for checking the pH of solutions. A Shimadzu model 670 atomic absorption spectrometer with flame atomization was used. The operating parameters were set as described by the manufacturer. A PerkinElmer (USA) graphite furnace atomic absorption spectrometry model A Analyst 300 was used in a nitrous oxide–acetylene flame for all GFAAS measurements. A UV–vis spectrophotometer model V 53 from JASCO (Tokyo, Japan) was used for recording the spectra and the absorbance measurements. The absorbance measurements were given by mounting the optical membrane sensor samples (1 × 9 × 50 mm3) inside a quartz cuvette. The absorbance measurements of the optical membrane sensor samples were obtained with respect to air as well as a blank sensor sample. A Hamilton syringe (10 μL) was used to transport minor Pd2+ ion volumes into the cell. The film thicknesses of the sensing slides were measured by using a digital microscope (Ray Vision Y 103) that was coupled with a video camera (JVC TK-C 751 EG) and a digital micrometer (Mitutoy, Japan) with an accuracy of ± 0.001 mm.
2.2 Preparation of the reagent
The AHDDO azo dye under investigation was prepared via the common approach used for preparing azo dye derivatives of aromatic amines. The aromatic amine involved aminoantipyrane, where 0.01 mole of the aminoantipyrane was converted to the hydrochloric form by adding the lowest amount of 1
:
1 HCl and then diluting with water and cooling at −2.0 °C. A cooled solution of NaNO2 (0.01 mole) is added gradually with continuous stirring of the amine salt. The resulting diazonium salt solution is allowed to stand in an ice bath for 15 min with stirring at −2.0 °C and added gradually to a solution of 0.01 mole of 2-amino-3-hydroxy pyridine dissolved in 10% NaOH which is cooled at −2.0 °C. The resulting solution is allowed to stand for 15 min with constant stirring until the azo dye completely formed. The obtained azo is filtered off, dried and recrystallized in ethanol. The purity of the resulting azo compounds is checked by measuring the melting point constancy. The chemical structure of AHDDO is detected by elemental analysis (C, H, N), IR and 1H-NMR spectra. The separated azo has the following structural formula:
4-(2-amino-3-hydroxypyridine-4-ylazo)1,5-dimethyl-2-phenyl-1,2-dihydropyrazol-3-one (AHDDO).
2.3 Chemicals
All chemicals were of analytical-reagent quality and freshly deionized water was used throughout. For fabrication and preparation of the membrane, agarose and epichlorohydrine were supplied by Merck Chemical Company. The solutions of pH 2.75–10.63 universal buffer were prepared as described earlier.54
2.4 Procedures for spectrophotometric titrations
2.0 mL of the AHDDO ionophore solution (1 × 10−4 M) in ethanol was transferred into a quartz cell of the spectrometer. Microliter amounts of 1 × 10−3 M solution of each metal ion were transferred to the ionophore solution by a 50 μL microsyringe. Each spectrum was recorded immediately after vigorous mixing of the solution. All the absorbance data were corrected for the dilution.
A method described elsewhere55,56 was used for preparation and epoxy activation of agarose membranes with a thickness of 0.25 mm from 4.0% agarose aqueous solution. The transparent-viscose solution was dispersed between two 20 cm × 20 cm dust-free glass plates, applying a gentle pressure. The borders of one of the glass plates were already lined with a 0.20 mm thickness tape to adjust the thickness of the membrane. For the epoxy activation, an epichlorohydrine method was used.57 The membranes were cut into 1 cm × 3 cm pieces and stored at 4 °C under 50% ethanol solution before use. For immobilization of the AHDDO, the agarose membranes were treated with a 1 × 10−2 M AHDDO solution, in an alkaline medium, by a method described elsewhere.55 The resulting orange color membranes were thoroughly washed with 50% ethanol on a glass filter, soaked in 50% ethanol overnight, and washed vigorously with water to displace any non-bonded dye. A 1 cm × 3 cm piece of the fabricated membrane sensor was cut and mounted in a polyacrylamide holder and placed inside the quartz cell of the spectrophotometer. The cell was then used as usual for the absorbance determinations. All the measurements on the agarose membranes were performed in aqueous medium.
2.5 Procedure
The membrane was carefully placed into the spectrophotometer cell, which had been previously filled with 2.5 mL of buffer solution adjusted to pH 5.75. Subsequently, a solution containing Pd2+ ions at a specific concentration was introduced, and the absorption spectrum was recorded over the wavelength range of 350–750 nm at 10 mm intervals, using a reference membrane. All measurements were carried out at 35 °C. The calibration curve was generated by plotting the absorbance values obtained from a series of standard solutions with varying concentrations of Pd2+ ions. To determine the Pd2+ ion content in the sample, the calibration curve was employed. For membrane regeneration, a 2.0 min treatment in a 0.15 M HNO3 solution was conducted, preparing the sensor membrane for subsequent use.
2.6 Analysis of water samples
In Egypt's Benha during the month of November 2024, several water samples were taken. One-liter polyethylene bottles with a stopper are used to store the samples. The samples (1.0 L) were kept at 4.0 °C to collect particles in a refrigerator after being filtered using a membrane filter with a 0.45 m pore size and acidified with 1.0% v/v HNO3. The organic interferences in the samples oxidize when 1.0% H2O2 and strong nitric acid are present. In order to bring the pH to 5.75, a universal buffer solution is needed. The suggested approach and FAAS were used to calculate the analyte concentrations in the samples. The membrane is stored for two weeks in 50% ethanol/water (v/v) solution to be used without any effect on the water quality.
2.7 Road dust sample
Using a small brush and a plastic pan, road dust samples that may contain ultra-trace amounts of Pd2+ were collected from the free Benha–Cairo and Benha–Zagazig roads in Egypt. The samples were dried in a 100 °C oven for 24 hours without the need to sieve the substance first. In a PTFE vial, 0.2 g of the dust (road dust) sample was weighed. Aqua regia 3.0 mL was added. The mixture was nearly dried out through heating. The mixture was heated once again for two hours after 3.0 mL of aqua regia and 1.0 mL of 40% HF were added. Following the addition of 2.0 mL of 65% HNO3, the mixture was heated until it was dry. To ensure the complete elimination of HF, the final procedure was repeated twice. The residue was diluted to 50 mL with deionized water. The digest was centrifuged for 20 min at 3000 rpm in order to separate the solid residue. The sample acidity was adjusted to pH 5.75 with universal buffer. A blank solution characterizing the procedure was prepared in the same way.
2.8 Determination of palladium in soil samples
In Benha, Egypt, a soil sample was taken from the area surrounding Farid Nada Street. The material was ground after drying at 100 °C for two hours. A beaker was filled with 5.0 g of the soil sample or the certified reference material GPP-10 (generator: GEOSTATS PTY Ltd, Australia; sample type: reference ore). Following the addition of 15 mL of aqua regia, the mixture was boiled almost to dryness. Once more, 15 mL of aqua regia was added to the leftover to finish the digestion, and the mixture was boiled almost to dryness. The sample combination was cooled, added 20 mL of distilled water, and then filtered through Whatman filter paper. The pH of the solution under the filter was adjusted to 2.0–3.0, and then it was transferred into a 100 mL volumetric flask, and diluted with water. A fresh garlic sample was dried for some days and in an oven at 45 °C to a constant weight. 2.5 g of the dried sample was transferred into a beaker, to which 10.0 mL of concentrated nitric acid was added. It was placed on a hot plate to become semi-dried. Again, 10 mL of conc. HNO3 and 2.0 mL of H2O2 were added, and the solution was kept on the hot plate in order to complete the digestion. Following drying, it was cooled, 20 mL of water was added, and a Whatman filter paper was used to filter the mixture. The sample solution was brought to a pH of 5.75, transferred to a volumetric flask of 100 mL, and then diluted with water.
2.9 Determination of palladium in biological samples
A 100 mL volumetric flask was filled with approximately 2.0 mL of whole blood samples, 3.0 mL of 2.0 M HNO3 solution, and then 1.0 mL of hydrogen peroxide. The flask was manually shaken to promote sample oxidation before deionized water was added to bring it up to volume.
For the decomposition of the urine sample, 20 mL of urine and 1.0 mL of concentrated nitric acid are mixed in a 70 mL quartz vessel and slowly heated up in order to concentrate the volume to about 2.0 mL. Afterwards, a further 4.0 mL of concentrated nitric acid and 0.5 mL of 12 M hydrochloric acid are added and this sample is digested in a high pressure asher (HPA®) for 50 min at a temperature of 320 °C and a pressure up to 130 Pa. The volume is eventually lowered to roughly 0.5 mL in order to eliminate any additional acids from the resultant solution. Losses of the analytes could happen if evaporation to dryness is not prevented. The blank was created by substituting high-purity water for the human serum or urine sample, and it was then put through the same process as stated above.
2.10 Determination of palladium in tea samples
Samples of tea grown on agricultural land that may contain small amounts of Pd2+ ions were tested. A 50 mL beaker containing 10 mg of dry tea that had been dried at 110 °C was then filled with 7.0 mL of concentrated nitric acid. The beaker was then covered with glass, and the contents were heated at 150 °C for 15 minutes. After cooling the sample, 3.0 mL of H2O2 was added, and the mixer was once more heated to 200 °C for roughly an hour, or until the solution was clear. After removing the glass watch, the acid was heated to 150 °C and evaporated to dryness. 5.0 mL of 1.0 M nitric acid was used to completely dissolve the white residue, and the resulting solution was then poured into a 100 mL volumetric flask. After that, the solution was properly neutralized with NaOH solution. The resulting solution was diluted to the mark and the pH adjusted to 5.75, and the recommended procedure was followed.
3 Results and discussion
3.1 Spectrophotometric studies
Pd2+ has a strong attraction for soft coordination centers like nitrogen and oxygen.58 The detection systems based on oxygen-containing ligands have generally demonstrated higher selectivity and sensitivity among the many types of palladium optode detection systems.59 As a result, the possibility of using AHDDO with an oxygen-containing site as an active ionophore for highly accurate and sensitive Pd2+ ion detection was explored. By using spectrophotometric titration, the complexation of Pd2+ with AHDDO in ethanol solution was studied. Fig. 1 depicts the absorption curves, which peak at about 444 nm due to AHDDO. A reduction in absorbance at this wavelength is seen with the addition of Pd2+. By decreasing the absorbance at 444 nm, a peak at about 567 nm is formed that corresponds to the formation of a Pd2+–AHDDO complex. For the other studied metal ions (Mn2+, Cd2+, Co2+ Hg2+, Zn2+, Cu2+, Pd2+, Sr2+, Al3+, Fe3+, Au3+, and Ag+) no effective complexion is detected. The resulting plots of the absorbance (at 567 nm) against metal ion/ligand mole ratios are shown in Fig. 2. From the sharp inflection point observed for Pd2+ at a mole ratio of 0.5, it can be immediately concluded that a 1
:
2 complex of [Pd–(AHDDO)2] is formed in ethanol solution. Using existing formulae and the nonlinear least-squares curve-fitting program KINFIT, the formation constant of the resultant complex between Pd2+ and AHDDO was calculated to be equivalent to 4.58 from the absorbance versus [Pd2+]/[AHDDO] mole ratio data.60,61 Evidently, the curves for the other metal ions in Fig. 2 indicate their negligible complex formation by AHDDO. Thus, based on the results obtained from solution studies, AHDDO was expected to act as a suitable and highly selective ionophore in the preparation of an agarose-based ion-selective optode for Pd2+ ion determination.
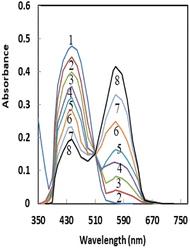 |
| Fig. 1 Absorbance spectra of 1-AHDDO (2 × 10−4 M) in ethanol in the presence of increasing [Pd2+] ions. The color change of solution upon complexation with Pd2+ ions. The numbers 2– 8 show the direction of absorbance changes by increasing [Pd2+] from 100–700 μg mL−1. | |
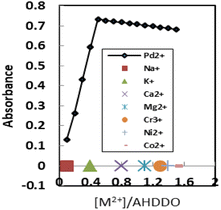 |
| Fig. 2 Absorbance of AHDDO (2 × 10−4 M) in ethanol at 567 nm, as a function of the [Pd2+]/[AHDDO] mole ratio for different metal ions. | |
3.2 Ligand immobilization on agarose membranes
The optical characteristics of AHDDO were somewhat altered by its immobilization on an agarose membrane. When AHDDO was immobilized, the absorbance maximum changed from 444 nm to roughly 458 nm in the redshift (Fig. 3), most likely as a result of the ligand's more flattened shape, which would make it easier for electrons to resonate. A similar shift (from 567 to 633 nm) was observed for an absorbance maximum of the Pd2+–AHDDO complex after the ligand immobilization on the agarose membrane (Fig. 3). During the titration, no measurable spectral shift was observed, which is typical for an absorption process involving a strong complex formation. The concentration of AHDDO as an ionophore, pH, and temperature all affect how much AHDDO can be loaded onto the agarose membrane. Due to the membrane-damaging effects of higher temperatures and the unsuitable immobilization at lower ones, 35 °C was chosen in this case. The influence of the ionophore concentration was investigated in the range of 5 × 10−5 to 1.5 × 10−2 M at λmax = 458 nm. As is obvious from Fig. 4, a continuous increase in the membrane absorbance was monitored by increasing the ionophore concentration up to 1 × 10−2 M. High loadings, however, may unacceptably decrease the absorbance of the membrane. Thus a concentration of 1 × 10−2 M of the AHDDO was chosen as an appropriate concentration in subsequent experiments. Fig. 5 shows the influence of pH of the reaction solution on the maximum absorbance of the membrane. By adding suitable amounts of universal buffer solution with a pH range of 2.75 to 10.63, the pH of the solution was adjusted. It was discovered that a pH 7.75 solution results in the highest immobilization of the AHDDO.
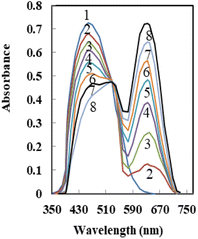 |
| Fig. 3 Absorbance spectra of the 1-AHDDO membrane in the presence of increasing [Pd2+] ions 2–8 for 40–225 μg mL−1. The color change upon complexation of AHDDO with Pd2+ ions. The numbers show the direction of absorbance changes by increasing Pd2+. | |
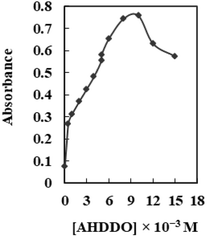 |
| Fig. 4 Effect of [AHDDO] on its loading on the agarose membrane. Experimental conditions: pH, 5.75; wavelength, 458 nm; temperature 35 °C. | |
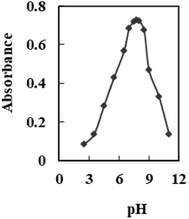 |
| Fig. 5 Effect of pH of the reaction solutions on the immobilization of AHDDO on the agarose membrane. Experimental conditions: [AHDDO], 1.0 × 10−2 M; λmax, 458 nm. | |
3.3 Effect of the pH of the test solution on the sensor response
The response of the suggested Pd2+ ion-selective optode is illustrated in Fig. 6 as being affected by the pH of the tested solution. The optode membrane's response characteristic was very pH dependent. Changing the pH from 3.56 to around 5.75 caused an immediate increase in absorbance, but pH values higher than 7.51 caused a drop in absorbance (Fig. 6). The diminished response at the low pH region may be explained by the extraction of H+ from the test solution into the membrane, via protonation of the nitrogen atom of AHDDO, resulting in an expected change in the formation of a Pd2+–AHDDO complex. On the other hand, the reduced optical response of the proposed sensor at pH > 7.51 could be due to a possible hydroxide formation of Pd2+ ions {Pd(OH)+ and Pd(OH)2}. Thus, in subsequent experiments, pH 5.75 was used for absorbance measurements.
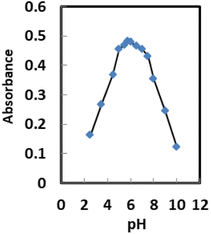 |
| Fig. 6 Effect of pH on the Pd2+ ion-selective sensor in the presence of 150 ng mL−1 Pd2+ at the optimum temperature. | |
3.4 Effect of temperature on the sensor reaction
The effect of temperature on sensor performance is investigated. The absorption spectra were recorded at 633 nm at a range of temperatures between 25 and 60 °C (Fig. 7). The absorbance at 633 nm rises with increasing Pd2+ sample temperature up to 35 °C, then falls due to thermal quenching associated with rising ion lattice vibrations58 and declining complex formation with the membrane. Upon increasing temperature to ≥60 °C, there is no variation in absorbance indicating no complex formation between Pd2+ and AHDDO. The best temperature to obtain highly sensitive and selective results was 35 ± 2.0 °C.
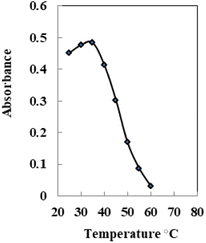 |
| Fig. 7 Effect of temperature on the Pd2+ ion-selective sensor in the presence of 150 ng mL−1 Pd2+ at the optimum temperature. | |
3.5 Effect of stirring
The response of the produced sensor is significantly influenced by stirring the Pd2+ solution. When the Pd2+ solution was stirred in comparison to the non-stirred solution, an improvement of almost seven times was obtained (Fig. 8). This observation can be explained by the movement of Pd2+ ions towards the immobilized AHDDO. The stirring process has accelerated the Pd2+ ion–AHDDO reaction as well as the Pd2+ ion diffusion across the membrane to the AHDDO (Fig. 8). The concentration gradient is the only variable that affects the diffusion of Pd2+ ions across the membrane during the non-stirring procedure.62
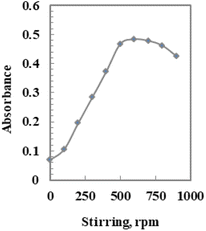 |
| Fig. 8 Effect of stirring on the Pd2+ ion-selective sensor in the presence of 150 ng mL−1 Pd2+ at the optimum temperature. | |
3.6 Effect of membrane thickness
Various membrane thicknesses were tested to choose the optimum ones. The absorption spectra were recorded at 633 nm at a membrane thickness between 15 and 50 mm. The thickness of the membrane was exactly assessed during the experiments using a digital microscope (Ray Vision Y 103) that was connected to a video camera (JVC TK-C 751 EG). The absorbance at 633 nm rises with increasing thickness up to 25 mm, and remains constant until it reaches 35 nm, then falls after 35 nm due to the extraction mechanism63 and declining complex formation with the membrane. The optimum thickness of the sensor membrane prepared was established to be 25 ± 2 mm. This is because the membrane is not too thick (>100 mm) and/or not too thin (<5.0 mm) and is reasonable to be applied as a transducer for the sensor membrane depending on the extraction mechanism.63
3.7 The sensor response time
It is clear that for 150 ng mL−1 Pd2+ at pH 5.75, the time required to reach the 97% steady state response is less than 5.0 min. The concentration of the analyte ion, membrane thickness, membrane diffusion, the rate of complex formation between the Pd2+ ion and AHDDO, and the rate of complex dissociation are some of the factors that affect this type of optode membrane's reaction time. At high concentrations of Pd2+ ions a rapid response was achieved, which resulted in a large change in response (3.0 min for 1.0 μg mL−1). The optode membrane's response time was shown to be slower at low Pd2+ ion concentrations (5.0 min for 150 ng mL−1). The response time typically ranged between 4.0 and 6.0 min and decreased as the analyte concentration increased. With a thicker membrane, it took noticeably longer to get a lower Pd2+ concentration.64,65 The proposed mechanism for the preparation and interaction between the AHDDO sensor and the Pd2+ ions and its complexation is depicted in Scheme 1.
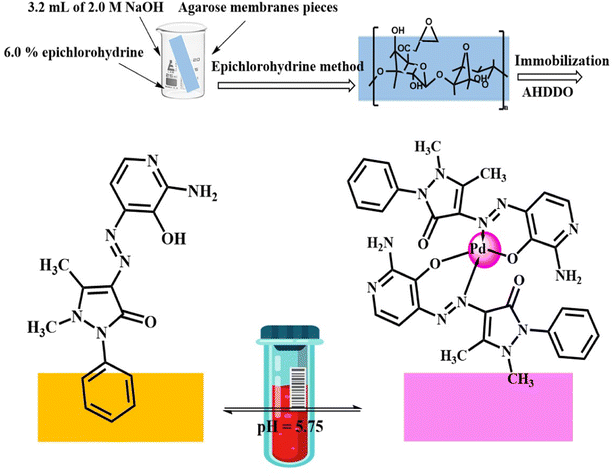 |
| Scheme 1 Schematic representation for the preparation and complexation of the formed optode. | |
3.8 Reproducibility, short-term stability and lifetime
When assessing a membrane optode's appropriateness for selectively determining an ion of interest, reproducibility and stability are two crucial factors to consider. The numerous applications of the optode for Pd2+ monitoring in test solutions at two concentration levels of 100 and 150 ng mL−1 were used to assess the reproducibility of the response. Following each absorbance test, the membrane was restored in less than 2.0 minutes by soaking in a solution of 0.03 M EDTA and 0.02 M HCl, respectively. Good reproducibilities were obtained at both Pd2+ concentration levels. The calculated relative standard deviation (RSD) values from the results were 1.68% and 1.82%, respectively, which are satisfactory and highly accurate. The short-term stability of the optode membrane was investigated by monitoring its absorbance values during its contact with a 150 ng mL−1 solution of Pd2+ at pH 5.75 over a period of 12 h. From the absorbance measurements in 30 min intervals (n = 24), it was found that the response was almost unchanged with only a 3.5% increase in absorbance at 633 nm after the 12 h period. Meanwhile, it was found that the membrane optode could be stored in 20% ethanol at 4 °C, without any measurable change in its absorbance value at 633 nm over a period of one month. Therefore, there is no ionophore leaching from the membrane during this period and it is quite stable over at least one month.
3.9 Efficient sensor membrane regeneration
Complete recovery of the sensor membrane's absorbance was not achieved upon transitioning from high to low concentrations of Pd2+ ions. To restore a sensor membrane previously immersed in Pd2+, various reagents, including NaOH, H2SO4, HCl, HNO3, and EDTA, were evaluated. Notably, among these options, 0.15 M HNO3 proved to be the most effective, fully regenerating the sensor after a brief 2.0 min treatment. It's important to note that following the regeneration step, and before subsequent analyses, the sensor membrane must be immersed in a buffer with a pH of 5.75 for a minimum of 3.0 min.
3.10 Selectivity
A membrane optode's selectivity, which indicates its relative response for the principal ion over various ions present in solution, may be one of its most crucial properties. Thus, it was investigated how several common metal ions affected the absorbance of the proposed Pd2+ sensor. To study the selectivity of the proposed Pd2+ sensor membrane, the absorbance of a fixed concentration of Pd2+ ions, at the 150 ng mL−1 level, in a solution of pH 5.75 was recorded before (Ao) and after (A) addition of some potentially interfering ions such as Ag+, Cd2+, Mn2+, Zn2+, Co2+, Hg2+, Cu2+, Ni2+, Sr2+, Al3+, Fe3+, and Au3+, and at concentrations up to 400 times of the analyte ion. The resulting relative error is defined as RE(%) = [(A − Ao)/Ao] × 100. The findings recorded in Table 1 clearly show that the relative error for all of the metal ions under investigation is less than 4.0%, which is regarded as tolerable. Further investigation revealed that anions such as C2O42−, NO3−, NO2−, CO32−, IO3− and CH3COO− present at high concentrations up to 200 μg mL−1 also do not show an obvious interfering effect on the Pd2+ assay (Table 1). The presented results demonstrated the tolerance limits of the ion concentrations added resulting in less than ± 5.0% relative error. The results show that the suggested optode membrane is extremely selective towards Pd2+ ions and may be used to detect levels of Pd2+ ions in natural environmental samples, even when other co-existing cationic and anionic species are present.
Table 1 Tolerance ratio (TR = ion/Pd2+ mass ratio) for various interfering ions in the determination of 150 ng mL−1 Pd2+
Ion |
TR |
Error% |
Ion |
Error% |
TR |
Na+, K+, Li+, acetate |
7500 |
+2.4 |
Al3+, Fe3+, CO32− |
−2.9 |
2500 |
Ca2+, Mg2+, succinate |
6000 |
−1.8 |
Fe2+, oxalate |
−3.0 |
2000 |
Ag+, Tl+, citrate |
5500 |
−3.1 |
Cu2+, Ni2+, SO42− |
+4.0 |
1750 |
Sr2+, Ba2+, NO2−, Cl− |
5000 |
−2.7 |
Hg2+, Co2+, HCO32− |
−3.5 |
1300 |
Ge4+, Ti4+, NO3− |
4500 |
+ 3.8 |
Sn4+, Sn2+ |
+3.9 |
1000 |
Bi2+, Mn2+, IO3− |
4000 |
−3.3 |
Zn2+, Pb2+, SCN− |
+3.9 |
750 |
Zr4+, Cr6+, S2O32− |
3500 |
+ 3.6 |
Au3+, La3+, Cr3+ |
+2.4 |
500 |
Mo6+, W6+, Br− |
3250 |
−3.0 |
Se4+, Te4+ |
−3.2 |
200 |
Th4+, UO22+, B4O72− |
3000 |
+ 3.5 |
Cd2+, Pt4+ |
+4.0 |
60 |
3.11 Calibration curve
As recorded in Table 2, the sensor response, in the form of a change in absorbance at 633 nm, towards Pd2+ ion concentration was obtained within 15–225 ng mL−1. The blank absorbance at 633 nm was assessed after equilibrating the sensor sample with blank solution at pH 5.75. The absorbance linearly differs as a function of Pd2+ ion concentration in the range of 15–225 ng mL−1. The equilibrating solution's minimum Pd2+ ion concentration was found to be 4.25 ng mL−1, which was sufficient to generate a distinct color change in the sensor. A larger amount of aqueous sample, however, can further reduce the detection limit of Pd2+ ion concentration. The detection and quantification limits,66 defined as CL = 3SB/m and CQ = 10SB/m (where CL, CQ, SB, and m are the detection limit, quantification limit, standard deviation of the blank and slope of the calibration graph, respectively), were 4.25 and 14.25 ng mL−1.
Table 2 Analytical characteristics of the offered sensor membrane
Parameters |
Proposed sensor |
For six replicate determinations of 150 ng mL−1 Pd2+.
|
pH |
5.75 |
λ
max (nm) |
633 |
Beer's range (ng mL−1) |
15–225 |
Ringbom range (ng mL−1) |
40–200 |
Molar absorptivity (L mol−1 cm−1) |
3.23 × 107 |
Detection limit (ng mL−1) |
4.25 |
Quantification limit (ng mL−1) |
14.25 |
Reproducibility (RSD%)a |
1.82 |
Regression equation |
|
Slope |
3.82 |
Intercept |
−0.11 |
Correlation coefficient (r) |
0.9975 |
The intra-day and inter-day precision and accuracy analytical results show that the attained approach is highly repeatable and reproducible, with coefficients of variation ranging between 1.88 and 2.15%.
Table 3 contains the findings of a comparison between optical sensor detection of Pd2+ ions and spectrophotometric methods.67–78 Unfortunately, there aren't many publications on optical sensors for evaluating Pd2+ ions. It was established that the achieved optode is significantly better than a number of available sensors for the monitoring of Pd2+ ions. In addition, the suggested sensor has a lower detection limit than spectrophotometric techniques. The use of AHDDO as a chromophoric reagent for an optode membrane for the preconcentration and evaluation of Pd2+ ions is described here for the first time.
Table 3 Comparison of the proposed optode method with spectrophotometric methods for Pd(II) determinationa
Reagent |
Conditions |
λ
max, nm |
ε
max, L mol−1 cm−1 |
M : L |
Linear range, μg mL−1 |
Interfering ions |
Ref. |
CETAB: cetyltrimethyl ammonium bromide; PW: present work.
|
3,4-Dihydroxy-benzaldehydeisonicotinoyl hydrazone |
pH = 3.0, acetate buffer |
380 |
5.3 × 103 |
1 : 1 |
0.5–20.0 |
Cu2+ |
67
|
4-(4′-Antipyriyl azo)-2-bromophenol |
pH = 4.0–7.0 |
498 |
2.1 × 103 |
1 : 2 |
0.2–3 |
Cu2+, Co2+, V5+, Ag+, Fe3+ |
68
|
α-Furildioxim |
0.1–1.4 M HCl, CHCl3 |
380 |
2.2 × 104 |
1 : 2 |
1–3 |
— |
69
|
4-(2′-Furalidene-imino)-3-methyl-5-mercapto-1,2,4-thiazole |
pH = 5.4, n-butanol |
410 |
1.4 × 103 |
1 : 1 |
17–50 |
Cu2+, Cr+6, Mn+7, S2O32−, CH4N2S |
70
|
Sulphochlorophenol-azorhodanine |
8 M H3PO4 + 5 M H2SO4; 1 M HCl, 1–2 h |
520; 520 |
1.2 × 105; 5.0 × 104 |
— |
— |
Pt4+, Rh3+ |
71
|
2-(2-Quinolylazo)-5-diethylaminobenzoic acid |
1.0 M HCl, 1% CETAB |
625 |
1.51 × 105 |
1 : 2 |
0.01–0.6 |
Co2+, Ni2+ |
72
|
4-(6-Bromo-2-benzothiazolylazo)pyrogallol |
Dimethylformamide |
580 |
— |
— |
0.6–15.0 |
— |
73
|
1-(2-Benzothiazolyl-azo)-2-hydroxy-3-naphthoic acid |
5.0 M HCl and CETAB |
669 |
2.61 × 105 |
1 : 2 |
0.0–0.9 |
Ni2+, Co2+ |
74
|
Benzyloxybenzaldehydethiosemicarbazone |
pH = 5.0, cyclohexanol |
365 |
4.0 × 103 |
1 : 21 |
5–60 |
Ni2+, Cu2+ |
75
|
4-(N′-(4-Imino-2-oxo-thiazolidine-5-ylidene)-hydrazino)-benzoic acid |
pH = 7.0, universal buffer |
450 |
4.3 × 103 |
1 : 1 |
0.6–10.6 |
Pt4+, Ru4+, |
76
|
4-(N′-(4-Imino-2-oxo-thiazolidin-5-ylidene)-hydrazino)-benzene-sulfonic acid |
pH = 7.6–8.3 |
438 |
7.5 × 103 |
1 : 2 |
0.2–2.2 |
Ir4+, Ru4+ |
77
|
Cefixime |
pH = 2.6 Na2HPO4-citric acid buffer |
352 |
1.22 × 104 |
2 : 1 |
0.8–16.5 |
Cu2+, Zn2+, Cd2+, Ni2+ |
78
|
AHDDO |
pH = 5.75 |
633 |
3.23 × 107 |
1 : 2 |
15–225 |
— |
PW |
3.12. Analytical applications
The suggested approach was used to determine the presence of Pd in various road dust, soil, water, food, and biological materials after digestion in order to demonstrate its reliability. In order to measure the matrix effect, the samples were spiked. The results showed good agreement between the amounts of added and measured analytes. Table 4 presents the outcomes. It is clear from the results of three analyses of each sample that Pd was successfully recovered. Table 4 lists all the results that were obtained. The recoveries between 97.74% and 102.29% that were obtained, as demonstrated in this table, validated the correctness of the developed procedure. The statistical analysis of these results using the Student's t-test showed that there was no significant difference between the experimentally measured and captured/detected concentrations at the 95% confidence level.79
Table 4 Results of Pd2+ determination in real samples after digestion (95% confidence level; n = 6)
Samples |
Added [ng mL−1] |
Foundb [ng mL−1] |
Recovery (%) |
t-testc |
F-valuec |
Sensor |
ETAAS |
DL: detection limit.
: Mean ± SD.
Theoretical values for t- and F-values at the 95% confidence level for five degrees of freedom are 2.57 and 5.05, respectively.79
|
Free Benha–Cairo road |
— |
<DLa |
<DLa |
|
|
|
75 |
75.7 ± 0.08 |
74.0.00 ± 0.77 |
100.93 |
1.89 |
|
150 |
148.6 ± 0.13 |
153.2.00 ± 1.05 |
99.07 |
|
3.71 |
Benha–Zagazig road |
<DLa |
<DLa |
<DLa |
|
|
|
100 |
98.8 ± 0.11 |
102.5 ± 1.20 |
98.80 |
1.62 |
|
200 |
201.6 ± 0.18 |
196.5 ± 0.95 |
100.80 |
|
3.96 |
Soil – 1 |
— |
<DLa |
<DLa |
|
|
|
60 |
60.5 ± 0.09 |
61.0 ± 1.05 |
100.83 |
2.18 |
|
120 |
118.8 ± 0.10 |
123.6 ± 1.30 |
99.00 |
|
4.04 |
Siol – 2 |
— |
<DLa |
<DLa |
|
|
|
90 |
88.9 ± 0.07 |
91.7 ± 0.80 |
98.78 |
1.85 |
|
180 |
182.3 ± 0.12 |
177.2 ± 1.00 |
101.28 |
|
3.96 |
Sea water |
— |
<DLa |
<DLa |
|
|
|
80 |
80.5 ± 0.08 |
78.8.00 ± 1.25 |
100.63 |
1.44 |
|
160 |
158.3 ± 0.13 |
163.6 ± 0.75 |
98.94 |
|
3.78 |
Underground water |
— |
<DLa |
<DLa |
|
|
|
70 |
69.1.25 ± 0.16 |
72.0 ± 0.95 |
98.71 |
|
4.07 |
140 |
143.2 ± 0.20 |
137.4 ± 0.85 |
102.29 |
1.93 |
|
Tap water |
— |
<DLa |
<DLa |
|
|
|
110 |
107.5 ± 0.21 |
106.4 ± 1.45 |
97.73 |
|
3.71 |
220 |
223.7 ± 0.13 |
217.0 ± 0.95 |
101.68 |
2.07 |
|
Serum |
— |
<DLa |
<DLa |
|
|
|
85 |
84.1 ± 0.11 |
86.5 ± 1.30 |
98.94 |
1.83 |
|
170 |
167.8 ± 0.17 |
173.0 ± 1.55 |
98.71 |
|
3.79 |
Urine |
— |
<DLa |
<DLa |
|
|
|
65 |
65.7 ± 0.14 |
64.0 ± 1.00 |
101.08 |
2.17 |
|
130 |
128.6 ± 0.19 |
132.3 ± 1.35 |
98.92 |
|
4.18 |
Green tea |
— |
<DLa |
<DLa |
|
|
|
105 |
106.6 ± 0.11 |
103.2 ± 2.05 |
101.52 |
2.08 |
|
210 |
214.0 ± 0.13 |
215.0 ± 1.85 |
101.90 |
|
3.85 |
— |
<DLa |
<DLa |
|
|
|
Black tea |
55 |
55.5 ± 0.18 |
54.0 ± 0.19 |
100.92 |
1.81 |
|
110 |
108.7.7 ± 0.20 |
112.4 ± 0.19 |
98.81 |
|
3.68 |
4 Conclusion
In this study, a highly selective optical sensor for the measurement of Pd2+ was created by immobilizing 4-(2-amino-3-hydroxypyridine-4-ylazo)1,5-dimethyl-2-phenyl-1,2-dihydropyrazol-3-one (AHDDO) on an agarose membrane. The proposed sensor has excellent optical properties that make it suitable for use as an optical sensor, including good selectivity, low cost, appropriate lifetime, rapid and reproducible regeneration, and ease of manufacture and handling. The sensor also demonstrated a rapid response time, a suitable linear calibration curve, and low detection and quantification limits. By means of an easy and low-cost methodology, satisfactory experimental results were achieved for the determination of Pd2+ ions by the prepared sensor. Without any significant interference from other metal ions, the suggested sensor can be used for the quantitative and qualitative measurement of Pd2+ ions in various real samples.
Ethical approval
This article does not contain any studies with human participants or animals performed by any of the authors.
Author contributions
Adil Bahathiq and Ahmad Babalghith: conceptualization, data curation, investigation, methodology, visualization, validation, writing – original draft, writing – review & editing. Alaa Amin: conceptualization, methodology, data curation, investigation, supervision, validation, writing – original draft, writing – review & editing. Abdelrazek Askar: conceptualization, investigation, methodology, validation, writing – original draft, writing – review & editing.
Conflicts of interest
There is no potential conflict of interest.
Acknowledgements
The authors would like to acknowledge financial support from Umm Al-Qura University and Benha University for providing instrumental facilities.
References
- S. Daniel, J. M. Gladis and T. P. Rao, Synthesis of imprinted polymer material with palladium ion nanopores and its analytical application, Anal. Chim. Acta, 2003, 488, 173–182 CrossRef CAS.
- E. A. Azooz, R. K. Ridha and H. A. Abdulridha, The fundamentals and recent applications of micellar system extraction for nanoparticles and bioactive molecules: A review, Nano Biomed. Eng., 2021, 13, 264–278 CAS.
- G. Singhal, R. Bhavesh, K. Kasariya, A. Sharma and R. Singh, Biosynthesis of silver nanoparticles using Ocimum sanctum (Tulsi) leaf extract and screening its antimicrobial activity, J. Nanopart. Res., 2011, 13, 2981–2988 CrossRef CAS.
- S. A. Moreno-Alvarez, G. A. Martinez-Castanon, N. Nino-Martinez, J. F. Reyes-Macias, N. Patino-Marin, J. P. Loyola-Rodriguez and F. Ruiz, Preparation and bactericide activity of gallic acid stabilized gold nanoparticles, J. Nanopart. Res., 2010, 12, 2741–2746 CrossRef CAS.
-
E. Berman, Toxic Metals and Their Analysis, John Wiley and Sons, New York, 1990 Search PubMed.
- K. A. Nabieh, W. I. Mortada, T. E. Helmy, I. M. M. Kenawy and Y. G. A. El-Reash, Chemically modified rice husk as an effective adsorbent for removal of palladium ions, Heliyon, 2021, 7, e06062 CrossRef CAS PubMed.
- B. Majidi and F. Shemirani, Solvent-based de-emulsification dispersive liquid–liquid microextraction of palladium in environmental samples and determination by electrothermal atomic spectrometry, Talanta, 2012, 93, 245–251 CrossRef CAS PubMed.
- K. Leopold, M. Maier, S. Weber and M. Schuster, Long-term study of palladium in road tunnel dust and sewage sludge ash, Environ. Pollut., 2008, 156, 341–347 CrossRef CAS PubMed.
- A. N. Anthemidis, D. G. Themelis and J. A. Stratis, Stopped-flow injection liquid–liquid extraction spectrophotometric determination of palladium in airborne particulate matter and automobile catalysts, Talanta, 2001, 54, 37–43 CrossRef CAS PubMed.
- M. Bujdoš, I. Hagarová, P. Matúš, L. Čanecká and J. Kubová, Optimization of determination of platinum group elements in airborne particulate matter by inductively coupled plasma mass spectrometry, Acta Chim. Slov., 2012, 59, 124–128 Search PubMed.
- F. Zereini, B. Skerstupp, F. Alt, E. Helmers and H. Urban, Geochemical behaviour of platinum-group elements (PGE) in particulate emissions by automobile exhaust catalysts: experimental results and environmental, Sci. Total Environ., 1997, 206, 137–146 CrossRef CAS.
- T. Shamspur, Solid phase extraction of trace amounts of palladium ions using multi-walled carbon nanotube modified by N,N'-bis(2-hydroxy-benzylidene)-2, 2'(aminophenylthio)ethane prior to determination by flame atomic absorption spectrometry, Bull. Chem. Soc. Ethiop., 2012, 26, 19–26 CrossRef CAS.
- M. Imamoglu, A. O. Aydin and M. S. Dundar, Determination of gold, palladium and copper by flame atomic absorption spectrometry after preconcentration on silica gel modified with 3-(2-aminoethylamino) propyl group, Cent. Eur. J. Chem., 2005, 3, 252–262 CAS.
- E. Yilmaz, R. M. Alosmanov and M. Soylak, Magnetic solid phase extraction of lead(II) and cadmium(II) on a magnetic phosphorus-containing polymer (M-PhCP) for their micro-sampling flame atomic absorption spectrometric determinations, RSC Adv., 2015, 5, 33801–33808 RSC.
- A. V. Volzhenin, N. I. Petrova, N. S. Medvedev, D. S. Irisov and A. I. Saprykin, Determination of gold and palladium in rocks and ores by atomic absorption spectrometry using two-stage probe atomization, J. Anal. Chem., 2017, 72, 156–162 CrossRef CAS.
- S. Z. Mohammadi, D. Afzali, M. A. Taher and Y. M. Baghelan, Determination of trace amounts of palladium by flame atomic absorption spectrometry after ligandless-dispersive liquid–liquid microextraction, Microchim. Acta, 2010, 168, 123–128 CrossRef CAS.
- K. S. Patel, P. C. Sharma and P. Hoffmann, Graphite furnace-atomic absorption spectrophotometric determination of palladium in soil, Fresenius. J. Anal. Chem., 2000, 367, 738–741 CrossRef CAS PubMed.
- K. Kińska, J. Jiménez-Lamana, J. Kowalska, B. Krasnodębska-Ostręga and J. Szpunar, Study of the uptake and bioaccumulation of palladium nanoparticles
by Sinapis alba using single particle ICP-MS, Sci. Total Environ., 2018, 615, 1078–1085 CrossRef PubMed.
- A. Milheiro, J. Muris, C. J. Kleverlaan and A. J. Feilzer, Influence of shape and finishing on the corrosion of palladium-based dental alloys, J. Adv. Prosthodont., 2015, 7, 56–61 CrossRef PubMed.
- Y. Chen, W. Jin and Y. Yang, ICP-AES determination of catalyst palladium residue in nebivolol hydrochloride crude product, Chin. J. Pharm. Anal., 2012, 32, 2226–2228 CAS.
- M. K. Van, A. Smekens, M. Behets, P. Kazandjian and G. R. Van, Determination of platinum palladium, and rhodium in automotive catalysts using high-energy secondary target X-ray fluorescence spectrometry, Anal. Chem., 2007, 79, 6383–6389 CrossRef PubMed.
- Y. J. Dong, Liquid-liquid microextraction coupled with high performance liquid chromatography for determination of palladium, J. Chin. Chem. Soc., 2008, 55, 567–571 CrossRef CAS.
- B. K. Ostręga, M. Sadowska and K. Miecznikowski, Selective determination of Pd nanostructures in environmental matrices - Application of a carbon monoxide probe, Electrochim. Acta, 2022, 429, 140999 CrossRef.
- D. Amorello, S. Orecchio, S. Barreca and S. Orecchio, Voltammetry for monitoring platinum, palladium and rhodium in environmental and food matrices, ChemistrySelect, 2023, 8, e202300200 CrossRef CAS.
- T. A. Kokya and K. Farhadi, Optimization of dispersive liquid–liquid microextraction for the selective determination of trace amounts of palladium by flame atomic absorption spectroscopy, J. Hazard. Mater., 2009, 169, 726–733 CrossRef CAS PubMed.
- A. Khanmohammadi, A. J. Ghazizadeh, P. Hashemi, A. Afkhami, F. Arduini and H. Bagheri, An overview to electrochemical biosensors and sensors for the detection of environmental contaminants, Iran. Chem. Soc., 2020, 17, 2429–2447 CrossRef CAS.
- A. Motalebizadeh, H. Bagheri, S. Asiaei, N. Fekratc and A. Afkhami, New portable smartphone-based PDMS microfluidic kit for the simultaneous colorimetric detection of arsenic and mercury, RSC Adv., 2018, 8, 27091–28100 RSC.
- M. M. Bordbar, A. Sheini, P. Hashemi, A. Hajian and H. Bagheri, Disposable paper-based biosensors for the point-of-care detection of hazardous contamina-tions- A review, Biosensors, 2021, 11, 316–357 CrossRef CAS PubMed.
-
M. Ahmadi, A. Afkhami and T. Madrakian, Magnetic Nanomaterials in Analytical Chemistry, Chapter 9 -Application of Magnetic Nanomaterials as Colorimetric Sensors, Elsevier Inc., 2021, pp. 213–225 Search PubMed.
- P. Karami, H. Khoshsafar, M. Johari-Ahar, F. Arduini, A. Afkhami and H. Bagheri, Colorimetric immunosensor for determination of prostate specific antigen using surface plasmon resonance band of colloidal triangular shape gold nanoparticles, Spectrochim. Acta, Part A, 2019, 222, 117218 CrossRef CAS PubMed.
- R. F. Alshehri, A. S. Amin and E. R. Darwish, Ultrasensitive and highly selective detection of nickel ion by two novel optical sensors, Anal. Bioanal. Chem., 2023, 415, 5695–5707 CrossRef CAS PubMed.
- A. S. Amin, S. M. El-Bahy and A. M. E. Hassan, Construction of an optical sensor for molybdenum determination based on a new ionophore immobilized on a polymer membrane, J. King Saud Univ., Sci., 2023, 35, 102592 CrossRef.
- E. M. I. Moustafa, A. S. Amin and E. R. Darwish, Optical chemical sensor of Gd(III) based on 5-(2`-bromophenyl-azo)-6-hydroxypyrimidine-2,4-dione immobilized on poly(methyl methacrylate) and 2-nitrophenyl octyl ether matrix, RSC Adv., 2022, 12, 26090–26098 RSC.
- A. S. Amin, H. H. El-Feky and N. Hassan, Novel sensor for selective monitoring of trace Ytterbium ions based on an agarose based optical membrane, RSC Adv., 2022, 12, 26620–26629 RSC.
- H. H. El-Feky, A. S. Amin and E. M. I. Moustafa, Utilization of a plasticized PVC optical sensor for the selective and efficient detection of cobalt(II) in environmental samples, RSC Adv., 2022, 12, 18431–18440 RSC.
- E. M. I. Moustafa, A. S. Amin and M. A. El-Attar, A highly selective bulk optode based on 6-{4-(2, 4-dihydroxy-phenyl) diazenyl) phenyl}-2-oxo-4-phenyl-1,2-di-hydropyridine-3-carbonitrile incorporating chromoionophore V for determination of nano levels of cadmium, Anal. Biochem., 2022, 654, 114835 CrossRef CAS PubMed.
- A. S. Amin, S. El-Bahy and H. H. El-Feky, Utility of 5-(2′,4′-dimethylphenyl-azo)-6-hydroxy-pyrimidine-2,4-dione in PVC membrane for a novel green optical chemical sensor to detect zinc ion in environmental samples, Anal. Biochem., 2022, 643, 114579 CrossRef CAS PubMed.
- H. Hesham, S. M. El-Bahy, A. M. E. Hassan and A. S. Amin, Utility of a novel optical sensor design for ultra-trace detection of chromium colorimetrically in real environmental samples, Int. J. Environ. Anal. Chem., 2023, 103, 4031–4048 CrossRef.
- H. Xu, J. W. Aylott, R. Kopelman, T. J. Miller and M. A. Philbert, A real-time ratiometric method for the determination of molecular oxygen inside living cells using sol–gel-based spherical optical nanosensors with applications to rat C6 glioma, Anal. Chem., 2001, 73, 4124–4133 CrossRef CAS PubMed.
- S. Dong, M. Luo, G. Peng and W. Cheng, Broad range pH sensor based on sol–gel entrapped indicators on fibre optic, Sens. Actuators, B, 2008, 129, 94–98 CrossRef CAS.
- Y. Egawa, R. Hayashida and J. Anazi, Multilayered assemblies composed of brilliant yellow and poly(allylamine) for an optical pH sensor, Anal. Sci., 2006, 22, 1117–1119 CrossRef CAS PubMed.
- R. Guell, C. Fontas, V. Salvado and E. Antico, Development of a selective optical sensor for Cr(VI) monitoring in polluted waters, Anal. Chim. Acta, 2007, 594, 162–168 CrossRef PubMed.
- H. Hisamoto, Y. Manabe, H. Yanai, H. Tohma, T. Yamada and K. Suzuki, Molecular design, characterization, and application of multi information dyes for multidimensional optical chemical sensings. 2. Preparation of the optical sensing membranes for the simultaneous measurements of pH and water content in organic media, Anal. Chem., 1998, 70, 1255–1261 CrossRef CAS PubMed.
- E. Bakker, P. Bühlmann and E. Pretsch, Carrier-based ion-selective electrodes and bulk optodes. 1. General characteristics, Chem. Rev., 1997, 97, 3083–3132 CrossRef CAS PubMed.
- H. H. El-Feky, A. M. Askar and A. S. Amin, Quantification of silver in several samples using a new ionophore polymer membrane as an optical sensor, RSC Adv., 2021, 11, 35300–35310 RSC.
- L. Wang, M. Ren, Z. Li, L. Dai and W. Lin, Development of a FRET-based ratiometric fluorescent probe to monitor the changes in palladium(II) in aqueous solution and living cells, New J. Chem., 2019, 43, 552–555 RSC.
- S. Mondal, S. K. Manna, S. Pathak, A. A. Masum and S. Mukhopadhyay, A colorimetric and “off–on” fluorescent Pd2+ chemosensor based on a rhodamine-ampyrone conjugate: synthesis, experimental and theoretical studies along with in vitro applications, New J. Chem., 2019, 43, 3513–3519 RSC.
- J. Yan, X. Wang, Q. Tan, P. Yao, J. Tan and L. Zhang, A colorimetric and fluorescent dual probe for palladium in aqueous medium and live cell imaging, Analyst, 2016, 141, 2376–2379 RSC.
- H. Nie, J. Geng, J. Jing, Y. Li, W. Yang and X. Zhang, Triphenylphosphine-assisted highly sensitive fluorescent chemosensor for ratiometric detection of palladium in solution and living cells, RSC Adv., 2015, 5, 97121–97126 RSC.
- L. Zhou, S. Hu, H. Wang, H. Sun and X. Zhang, A novel ratiometric two-photon fluorescent probe for imaging of Pd2+ ions in living cells and tissues, Spectrochim. Acta, Part A, 2016, 166, 25–30 CrossRef CAS PubMed.
-
A. S. Medin, Studies on Structure and Properties of Agarose, PhD Thesis, Uppsala University, 1995.
- M. Wilchek and T. Miron, Thirty years of affinity chromatography, React. Funct. Polym., 1999, 41, 263–268 CrossRef CAS.
- C. Bariain, I. R. Matias, F. J. Arregui and M. Lopez-Amo, Optical fiber humidity sensor based on a tapered fiber coated with agarose gel, Sens. Actuators, B, 2000, 69, 127–131 CrossRef CAS.
-
H. T. S. Britton, Hydrogen Ions, Chapman and Hall, London, UK, 4th edn, 1952, p. 1168 Search PubMed.
- P. Hashemi, M. M. Abolghasemi, K. Alizadeh and R. A. Zarjani, A calmagite immobilized agarose membrane optical sensor for selective monitoring of Cu2+, Sens. Actuators, B, 2008, 129, 332–338 CrossRef CAS.
- P. Hashemi and M. M. Abolghasemi, Preparation of a novel optical sensor for low pH values using agarose membranes as support, Sens. Actuators, B, 2006, 115, 49–53 CrossRef CAS.
- I. Matsumoto, Y. Mizuno and N. Seno, Activation of sepharose with epichloro-hydrine and subsequent immobilization of ligand for affinity adsorbent, J. Biochem., 1979, 85, 1091–1098 CrossRef CAS PubMed.
- H. Tavallali and M. G. P. Jahromi, A novel optode sensor for the determination of palladium(II) in water and a hydrogenation catalyst, J. Serb. Chem. Soc., 2009, 74, 311–315 CrossRef CAS.
- M. C. Aragoni, M. Arca, F. Demartin, F. A. Devillanova, F. Isaia, A. Garau, V. Lippolis, F. Jalali, U. Papke, M. Shamsipur, L. Tei, A. Yari and G. Verani, Fluorometric chemosensors interaction of toxic heavy metal ions Pb(II), Cd(II) and Hg(II) with novel mixed-donor phenanthroline-containing macrocycles: spectrofluorometric, conductometric and crystallographic studies, Inorg. Chem., 2002, 41, 6623–6632 CrossRef CAS PubMed.
- K. Akhbari, K. Alizadeh, A. Morsali and M. Zeller, A new two-dimensional thallium(I) coordination polymer with 4-hydroxybenzylidene-4-aminobenzoate: thermal, structural, solution and solvatochromic studies, Inorg. Chim. Acta, 2009, 362, 2589–2594 CrossRef CAS.
- K. Saidi, W. Chaabani and M. Dammak, Highly sensitive optical temperature sensing based on pump-power-dependent upconversion luminescence in LiZnPO4: Yb3+–Er3+/Ho3+ phosphors, RSC Adv., 2021, 11, 30926–30936 RSC.
- M. Ahmad and R. Narayanaswamy, Optical fiber Al(III) sensor based on solid surface fluorescence measurement, Sens. Actuators, B, 2002, 81, 259–266 CrossRef CAS.
- C. C. Li and M. S. Kuo, Application of the acetylacetone chelation solid-phase extraction method to measurements of trace amounts of beryllium in human hair by GFAAS, Anal. Sci., 2002, 18, 607–609 CrossRef CAS PubMed.
- E. Antico, M. Lerchi, B. Rusterholz, N. Achermann, M. Badertscher, M. Valiente and E. Pretsch, Monitoring Pb2+ with optical sensing films, Anal. Chim. Acta, 1999, 388, 327–338 CrossRef CAS.
- M. Noroozifar, M. K. Motlagh, A. Taheri and R. Z. Dorabei, Diphenylthiocarbazone immobilized on the triacetyl cellulose membrane as an optical silver sensor, Turk. J. Chem., 2008, 32, 249–257 CAS.
-
H. M. N. H. Irving, H. Freiser, and T. S. West, IUPAC Compendium of Analytical Nomenclature: Definitive Rules, Pergamon Press, Oxford, UK, 1981 Search PubMed.
- S. Viennot, M. Lissac, G. Malquarti, F. Dalard and B. Grosgogeat, Influence of casting procedures on the corrosion resistance of clinical dental alloys containing palladium, Acta Biomater., 2006, 2, 321–330 CrossRef PubMed.
- H. J. Mohammed, A. Y. Muhi and H. A. Meisslemaw, Determination of trace amount of Pd(II) and Ni(II) with newly synthesized pyrozlon azo bromo phenol by spectrophotometric methods, E-j. Chem., 2011, 8, 381843 Search PubMed.
-
S. I. Ginzburg, N. A. Yezerskaya, I. V. Prokofevaet al., Analiticheskaya Khimiya Platinovykh Metallov, p. 614, Nauka, Moscow, Russia, 1972 Search PubMed.
- S. H. Gaikwad, T. N. Lokhande and M. A. Anuse, Extraction spectrophotometric determination of micro amounts of palladium(II) in catalysts, Indian J. Chem., 2005, 44A, 1625–1630 CAS.
- R. F. Gureva and S. B. Savvin, Spectrophotometric methods for determining noble metals, J. Anal. Chem., 2002, 57, 980–996 CrossRef CAS.
- Z. Huang, Q. Wei and X. Yang,
et al., Spectrophotometric determination of palladium by the colouration with 2-(2-quinolylazo)-5-diethylaminobenzoic acid, Bull. Korean Chem. Soc., 2005, 26, 1623–1626 CrossRef CAS.
- N. A. Naser, K. H. Kahdim and D. N. Taha, Synthesis and characterization
of an organic reagent 4-(6-bromo-2-benzothiazolylazo) pyrogallol and its analytical application, J. Oleo Sci., 2012, 61, 387–392 CrossRef CAS PubMed.
- N. Hassan and A. S. Amin, Solid phase extraction and spectrophotometric determination of palladium with 1-(2-benzothiazolylazo)-2-hydroxy-3-naphthoic acid, Anal. Chem. Lett., 2017, 7, 724–736 CrossRef CAS.
- S. L. Narayana, K. J. Reddy, S. A. N. Reddy, J. R. Kumar and A. V. Reddy, Synthesis of new reagent benzyloxybenzaldehyde thiosemicarbazone (BBTSC): selective, sensitive and extractive spectrophotometric determination of Pd(II) in water samples and synthetic mixtures, J. Chin. Chem. Soc., 2007, 54, 1233–1241 CrossRef CAS.
- O. S. Tymoshuk, O. S. Fedyshyn, L. V. Oleksiv, P. V. Rydchuk and V. S. Matiychuk, Spectrophotometric determination of palladium(II) ions using a new reagent: 4-(N’-(4-Imino-2-oxo-thiazolidine-5-ylidene)-hydrazino)-benzoic acid (p-ITYBA), J. Chem., 2020, 8141853 CAS.
- L. Lozynska, O. Tymoshuk and T. Chaban, Spectrophotometric studies of 4-[N’-(4-imino-2-oxo-thiazolidin-5-ylidene)-hydrazino]-benzenesulfonic acid as a reagent for the determination of palladium, Acta Chim. Slov., 2015, 62, 159–167 CrossRef CAS PubMed.
- S. N. H. Azmi, B. Iqbal, B. H. K. Al Ruqishi, S. A. M. Al Sayabi, N. M. K. Al Quraini and N. Rahman, Optimized and validated spectrophotometric method for the determination of palladium(II) in synthetic mixture and automobile workshop area samples, J. Assoc. Arab Univ. Basic Appl. Sci., 2016, 19, 29–36 Search PubMed.
-
J. N. Miller and J. C. Miller, Statistics and Chemometrics for Analytical Chemistry, Pearson/Prentice Hall, Harlow, UK, 5th edn, 2005, pp 58–67 Search PubMed.
|
This journal is © The Royal Society of Chemistry 2024 |
Click here to see how this site uses Cookies. View our privacy policy here.