Viability of elutriation for the extraction of microplastics from environmental soil samples†
Received
20th March 2024
, Accepted 27th May 2024
First published on 30th May 2024
Abstract
In this study, we evaluated the suitability of elutriation, a method successfully employed in the extraction of microplastics from marine sediments, for the extraction of microplastics from freshwater and terrestrial soils. Five soils were sampled throughout Oklahoma, USA in order to capture a range of sand, silt, clay, and organic matter composition. Each soil was subjected to microplastic extraction with and without elutriation, followed by digestion in 7.5% NaOCl, and then flotation in 6 M ZnCl2. The mass of each soil was measured after elutriation to determine sample mass reduction, and multiple methods including fluorescence imaging and automated particle counting through ImageJ, Attenuated Total Reflectence-Fourier Transfor Infrared Spectroscopy (ATR-FTIR), and Pyrolysis-coupled Gas Chromatography/Mass Spectrometry (py-GC/MS) were used to determine microplastic quantity, mass, and characteristics. T-test was used to check for statistically-significant differences between methods in terms of mass or particle quantity. For all tested soils, elutriation resulted in greater sample mass reduction than non-elutriated samples, and was between 59.0–97.3% for the tested soils. Furthermore, no statistically significant (p < 0.05) differences were observed in particle quantification or polymer mass between methods, and no differences were observed for polymer or size distribution. Additionally, 33% more polymers were positively identified (R2 = 70%) by ATR-FTIR analysis in elutriated samples compared to non-elutriated soils. The mass reduction provided by elutriation allows for the processing of larger sample volumes, leading to greater accuracy and sensitivity in detecting microplastics. As such, we recommend elutriation be performed as a pretreatment step to extract microplastics from soils.
Environmental significance
The presence of microplastics in the environment and their toxic effects have been well-documented in modern literature; however, the spatial and temporal distribution of these contaminants remains uncertain. As a major sink of microplastics in the environment, soils are a major factor in their fate and transport, so a rigorous understanding of soil microplastic concentrations will greatly aid in the modelling of microplastics in the environment and risk-exposure analysis. Due to the complex nature of many soils, a multi-step extraction protocol is often necessary to quantify and characterize microplastics in environmental samples. This study aims to determine if elutriation, a method successfully employed in marine sediment extractions, is viable as a pretreatment step in the extraction of microplastics from a broad range of soils.
|
1 Introduction
Microplastics are broadly defined as plastic polymer particles with a size in the range of 1–5000 μm and exhibit a wide range of physical and chemical properties.1 The majority of microplastics (91% of land-based output) are produced from the breakdown of larger plastic debris and enter the ocean through freshwater systems such as creeks and rivers.2–4
Once in the freshwater systems, microplastics degrade via UV radiation as long as they remain suspended in the water column.1,5,6 Degradation greatly diminishes when microplastics become entrapped in sediments, therefore, soils are likely a long-term sink for microplastics.3,7–9 As such, a rigorous understanding of microplastic fate and transport requires measurement of microplastic concentrations in soils.
A variety of methodologies are available for the extraction of microplastics in soils, though no standardized protocol is currently fully agreed upon.10,11 Optimal extraction of microplastics requires the separation of microplastics from a complex matrix of organic matter and soil grains. The most common methods for eliminating organic matter from samples involve incubation in an oxidizing solution at elevated temperatures.12,13 Among the most successful methods are oxidation with Fenton's reagent or NaOCl.13–15 Currently, less information regarding the efficacy of NaOCl for digestion is available compared with Fenton's reagent. Direct comparisons of the two, however, show superior organic matter destruction for NaOCl, with comparable alteration to plastics.13,14 After digestion, soil grains may be efficiently removed through flotation in a dense brine solution. Solutes such as ZnCl2 allow for sufficient density (at least 1.4 g mL−1) to float heavy polymers.16–18 The combined efficiency of these processes has been quantified by multiple groups, with estimates placing the combined extraction efficiency of both processes between 92–94%.17,19 This extraction procedure is rather efficient, though still presents some drawbacks.
Because digestion of organic matter is destructive, this step has the potential to consume large volumes of reagents; however, a pretreatment step involving the removal of larger pieces of organic matter has the potential to reduce the consumption of reagents. Furthermore, solutes such as ZnCl2, though reusable, are often expensive and hazardous, and flotation is often performed using a centrifuge, where space is limited.17,19,20 As such, the usage of brine solutions should be minimized. One possible way of reducing the volume of brine solution required for separation is by decreasing the sample mass prior to this flotation using some form of pretreatment. Both of these goals can be achieved through simple elutriation.
Elutriation is the process of separating mixtures of particles using an upward fluid stream. The principle works by exploiting Stokes' Law, which states that a particle settling in a fluid medium will settle at a given velocity determined by the characteristics of the fluid and of the particle.21,22 By suspending a heterogeneous mixture in an upward fluid stream, the upward velocity can be tuned to such a value that particles can be effectively separated from one another based on density, shape, and size.21,23 This method has been applied in many unique areas of research and industry but most importantly has been used for microplastic extractions.24–28
Elutriation has been successfully implemented to separate microplastics from marine sediments. This method was pioneered in 2013 using a simple, 15 cm diameter vertical column elutriator.26 The upflow velocity was optimized through experiment, and elutriation was followed by centrifugation in NaI solution and digestion in different chemicals.26 This method was improved on by fractionating soils prior to elutriation then running each mass fraction at a unique flowrate, optimized through experimentation to maximize microplastic recovery.28 Kedzierski et al. were able to achieve 90% recovery of heavier polymers, without using heavy brine solutions. The method was also replicated using a small glass column and was able to achieve 72% recovery for PET.27 Elutriation is a promising method for the extraction of microplastics from sandy marine sediments; however, the efficacy of this method for silty, loamy, or clayey soils is still unclear.
Though microplastics are distributed worldwide, the spatiotemporal distribution of microplastics, which would typically be used to determine exposure risks, is not well known.3,29–31 The gap in modern knowledge of microplastic distributions is largely attributable to a total lack of standardized protocols for extraction, identification, and quantification of these contaminants.10,32,33 Given the prior success in implementing elutriation in marine sediments, the objective of this study is to investigate the viability of elutriation for the extraction of microplastics from soils of varying composition. This study proposes an improved procedure for the extraction of microplastics from environmental soil samples.26–28
2 Methods
2.1 Quality assurance and control
Prior to usage, all materials were sonicated for 30 min in DI water. Plastic usage was kept to a minimum, and whenever possible, procedures were performed in a laminar flow cabinet. Samples were handled in a clean lab while wearing 100% cotton lab coats.1,34 Negative controls were also performed to assess the background contamination during sampling and lab procedures.
2.2 Location and procedures
Four soils of varying texture and organic matter content were selected from local sources including creeks, rivers, and fields amended with applied biosolids. Arkansas River 1 (AR1) and Arkansas River 2 (AR2) were taken from the Arkansas River in Tulsa, Oklahoma, with AR2 being taken downstream from AR1. The Boomer Creek (BC) sample was taken from Boomer Creek in Stillwater, Oklahoma, and the Applied Biosolids 1 (AB1) sample was taken from the stillwater beneficial land application site, which contains agricultural soils amended with biosolids from the stillwater wastewater treatment facility. A fifth soil sample, Applied Biosolids 2 (AB2), was an additional sample produced by increasing the clay content of part of the AB1 sample by adding 70 g lab-grade bentonite to 200 g of AB1 sample. This was then wetted with DI water and thoroughly homogenized. Each sample was obtained using a shovel to sample the top 10 cm of soil just above the water line. An arbitrarily large sample mass was collected and placed in a clean glass jar, then labeled and sealed until further processing. Exact sampling locations are given in Table S1 in ESI.†
2.3 Soil preparation and analysis
2.3.1 Soil drying.
Approximately 1 kg of the total sample of each soil was spread out onto a sheet of aluminium foil and then placed into an oven at 50 °C. This temperature was selected in order to avoid surface oxidation of microplastics.35,36 Oven vents were fully opened and the samples allowed to dry for 24 h. Samples were then removed from the oven and manually worked through a 12in-diameter, 4.75 mm sieve to break up aggregates and remove large rocks and plant debris. Samples were then placed back into the oven at 50 °C for another 24 h. Once dried, samples were weighed out into 10 g aliquots. Samples were stored in glass beakers and capped with aluminium foil.
2.3.2 Texture analysis.
Approximately 300 g of dry soil as described in Section 2.3.1 from each sample was sent to Oklahoma State University's Soil, Water, and Forestry Analytical Laboratory (SWFAL) for a soil texture analysis. This provides the amount of clay, silt, and sand as a percentage of the mineral fraction of the soil.
2.3.3 Organic matter analysis.
Organic matter content was determined by loss on ignition. Three 10 g aliquots of each soil were weighed out in small aluminium dishes. The soil was then placed into an oven to dry at 105 °C for 24 h.37 The masses of each soil were recorded, and then placed into an oven at 550 °C for 30 min.37 The masses of each soil were again recorded, and the percentage of organic matter calculated.
2.3.4 Negative controls.
Fifty (50) mL of DI water was subjected to the extraction and quantification procedures as outlined in Section 2.3.5–2.5.1 in order to assess microplastic contamination during extraction.
2.3.5 Soil disaggregation.
Each 10 g sample was added to a 100 mL glass beaker. Fifty (50) mL of DI water was then added to achieve a mass-to-volume ratio of 1 g dry soil to 5 mL DI water.38 The mixture was stirred then allowed to sit overnight in order to thoroughly wet and soften soil aggregates. A probe-type sonicator was then lowered into the water to roughly half the depth of the water and centered in the beaker. In order to avoid fragmentation of microplastics, the sonication energy was selected such that organic matter integrity would be preserved, while still dispersing clay aggregates.38 At 20% power, 400 J were applied for every 1 g of dry soil.38 Once sonicated, the probe was lifted out of the sample and rinsed into the beaker with a wash bottle of DI water, and the sample was immediately transferred to pretreatment.
2.4 Extraction procedure
2.4.1 Pretreatment.
Each sonicated soil sample was subjected in triplicate to one of the following pretreatment steps:
Method 1, sieving: sonicated samples were each thoroughly rinsed through a 45 μm sieve. The passing fraction was discarded.
Method 2, elutriation: sonicated samples were added to a glass elutriation column (diameter = 5 cm, height = 30 cm) and elutriated using cold tap water at an upflow velocity of 1.3 cm s−1 for 15 min. The effluent of the column was passed through a 45 μm sieve, discarding the passing fraction. The sonicated samples were added while the column was in the process of filling, and the time was recorded from the point at which all sample had been added to the column. A time interval of 15 min was selected as this was sufficient to allow thorough separation of soil constituents.
The retained fraction of all samples was rinsed three times from the 45 μm mesh into a 250 mL beaker and capped with aluminium foil until further processing. Soils at this stage are referred to as “pretreated soils”. “Fully-extracted samples” were subsequently subjected to digestion and flotation as outlined in Section 2.4.2–2.5.4.
2.4.2 Digestion.
The pretreated sample was vacuum-filtered through a 47 mm diameter, 20 μm stainless steel mesh, and the beaker was thoroughly rinsed into the filtration apparatus three times with DI water to minimize losses. Once fully filtered, the funnel and mesh were rinsed into a 250 mL Erlenmeyer flask using a rinse bottle containing 7.5% NaOCl.13,14 The Erlenmeyer flask was then filled to 50 mL with 7.5% NaOCl, and the flask was capped with aluminium foil. The sample was then placed into an orbital incubator at 50 °C and 300 rpm for 24 h.
2.4.3 Flotation and filtration.
After digestion procedures, the digested sample was vacuum-filtered through a 47 mm diameter, 20 μm stainless steel mesh. Once completely filtered, the sample was rinsed thoroughly with DI water to remove any remaining NaOCl. The funnel and mesh were then rinsed into two 15 mL polypropylene vials (Falcon) using approximately 30 mL of 6 M ZnCl2 solution. Each vial was then vortexed until the soil was thoroughly dispersed. The vials were then placed in a centrifuge at 4900 rpm for 5 min.17,19 The vials were removed from the centrifuge, taking care to not agitate or invert the tubes. The supernatant of each vial was then vacuum-filtered through a 47 mm diameter, 20 μm stainless steel mesh. The vials were then refilled with 6 M ZnCl2 solution, dispersed, and the process repeated a total of three times. The 20 μm mesh and 47 mm funnel were then rinsed into a 13 mm vacuum filtration apparatus with a 25.4 mm diameter, 0.2 μm Al2O3 membrane. The walls were then rinsed with DI water to ensure the sample was retained on the Al2O3 membrane and not the filter funnel. The apparatus was then carefully disassembled, and the Al2O3 membrane placed in a glass Petri dish and labelled with name, date, and description.
2.5 Quantification
2.5.1 Nile red quantification.
Three to four drops of 2 μg mL−1 Nile red-methanol solution were placed onto the extracted microplastics to thoroughly wet them.39 Next, the microplastics were stained for 10 min, and then the filter was carefully transferred to a 47 mm glass frit filter unit with no funnel. The vacuum was turned on and 300 μL of 95% ethanol was used to rinse the excess Nile red dye from the filter. This was achieved by dropping the ethanol around the perimeter of the filter in such a way as to not disrupt the microplastics. Once dry, the filter was then transferred to a 6-well plate (Greiner) and capped with aluminium foil to avoid the disturbance of microplastics due to static charge on the acrylic plate lid. Each filter was then imaged at 4× magnification with the RFP channel using a fluorescence microscope (Cytation 5 Imager; Biotek). The images of the filters were then exported as PNG files at 1
:
1 pixel resolution. The particles in each image were then counted using the Microplastic Visual Analysis Tool (MP-VAT) through ImageJ.39 The average number of particles and standard error for each method and soil was calculated, and then t-test was then used to check for statistically-significant differences between methods.
2.5.2 Pyrolysis gas chromatography/mass spectrometry.
Each of the pretreated soils described in Section 2.4.1 was analyzed by py-GC/MS after pretreatment and after full extraction for both protocols. Pyrolysis gas chromatography/mass spectrometry (py-GC/MS) was conducted using an Agilent 6890 GC/5975 MS system (Agilent, Santa Clara, CA) with an EGA/PY-3030D Pyrolysis unit (Frontier Labs, Koriyama, Japan) in “single shot” mode and a UAMP column kit for microplastics analysis. The system was calibrated and configured according to Agilent's guidelines and integrated with the Frontier pyrolysis unit. Microplastic samples of interest (≈1 mg) were placed in a stainless steel Eco-cup SF sample cup (Frontier Labs, Koriyama, Japan), loaded into the pyrolysis unit and subjected to defined pyrolysis parameters. The pyrolysis process heated the sample to 600 °C, generating volatile and semi-volatile products for GC separation over a 45 min runtime. The UAMP column separated the products to target twelve polymers of interest: Polyethylene (PE), Polypropylene (PP), Polystyrene (PS), acrylonitrile-butadiene-styrene (ABS), Styrene-butadiene rubber (SBR), polymethyl methacrylate (PMMA), polycarbonate (PC), polyvinyl chloride (PVC), polyurethane (PU), polyethylene terephthalate (PET), nylon 6 (N6), and nylon 6,6 (N66). Quantification was performed using F-Search MPs 2.1 software (Frontier Labs, Koriyama, Japan), cross-referencing mass spectra and retention times with calibration curves constructed from polymer standards (MPs-CaCO3 Calibration standard) at five different weights (0.1 mg, 0.2 mg, 0.5 mg, 2 mg, and 4 mg) for each tissue sample.
The measured masses of microplastics were normalized by original sample mass to give units of mg (microplastics) kg (dry soil)−1.
2.5.3 Mass reduction.
Each of the pretreated soils described in Section 2.5.2 was dried overnight at 50 °C, and the dry mass of each soil was determined using a digital balance. Taking care to assure that each soil is well-mixed, both methods will result in identical soil mass reductions due to the passing fraction through the 45 μm sieve, denoted by ΔmSieving. However, pretreatment with elutriation will also result in an additive mass reduction due to the retention of heavy sediments within the column, denoted by ΔmElutriation. Mass reduction by elutriation primarily acts on high-density, large-diameter particles, and mass reduction by sieving affects small-diameter particles, so it can be assumed that mass reduction by sieving and mass reduction by elutriation are mutually exclusive. As such, the mass removal of each method, ΔmTotal, is given by the equation.
ΔmTotal = ΔmSieving + ΔmElutriation |
where ΔmElutriation is equal to zero for sieved soils, and ΔmSieving is constant for each soil sample and equal to ΔmTotal for sieved soils. All Δm values are reported as a percentage of the original sample mass.
2.5.4 Characterization.
One extracted sample from each soil and method was analyzed using Scanning Electron Microscopy (SEM) and Energy Dispersive X-ray Spectroscopy (EDS), at a primary energy of 20 keV, collection time of 5 min, and working distance of 10 mm.
Attenuated Total Reflectance – Fourier Transformed Infrared (ATR-FTIR) spectroscopy analysis was performed on one sample from each full extraction protocol following similar steps carried out by Quiambao et al.40 That is, for each particle of interest, the aperture was set to encompass the largest area of the particle possible without including the filter background or other nearby particles. Using the cooled detector, a background spectrum was collected before each particle, followed immediately by a 1.5 min, high-resolution scan while applying 2 psi to the germanium tip ATR. The number of particles analyzed was set at 10% of the total particle quantification from Section 2.5.1, with a minimum set at 5 particles and a maximum set at 30 particles. The threshold for positive identification was set at R2 = 70%. An image and IR spectrum was saved for each particle.
3 Results and discussion
3.1 Soil characteristics
The five soils differed in texture and organic matter content, and generally followed a trend whereby sandy soils were low in organic matter and clayey soils were higher in organic matter. That is, the AR2 sample contained the highest percentage of sand and minimal organic matter (96.3% sand, and 0.1% organic matter). Conversely, the sample AB2 showed the highest percentage of clay and a higher content of organic matter as well (50% clay and 6% organic matter). Finally, the AB1 sample contained the highest amount of organic matter (8.6% organic matter). The selected soil types were classified by soil texture as sand (AR2), sandy loam (AR1), loamy sand (BC), clay loam (AB1), and clay (AB2). Exact soil compositions are shown in Table S1.†
3.2 Sample mass reduction from elutriation
Elutriation resulted in greater soil mass reduction in all samples compared to sieving alone and this discrepancy was most pronounced in sandy samples (Fig. 1A–C). All samples experienced mass reduction under both sieving and elutriation. For sieving, this reduction was solely due to the passing fraction through the 45 μm sieve (Fig. 1A). As such, fine-grained soils experienced greater mass reduction than coarse-grained soils. For example, the most clayey soil, AB2, experienced an 87.6% reduction in mass from sieving alone, while the sandiest sample, AR2, only experienced a 2.50% reduction.
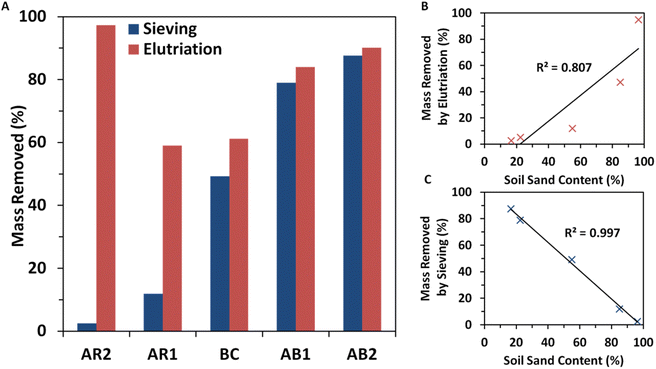 |
| Fig. 1 Total sample mass removal by pretreatment for each sample and method (A), and sample mass removal solely by elutriation (B) and sieving (C) as a function of soil sand content. Arkansas River 2 (AR2), Arkansas River 1 (AR1), Boomer Creek (BC), Applied Biosolids 1 (AB1), and Applied Biosolids 2 (AB2). | |
For elutriation, however, the mass was removed by two mechanisms: the first is due to the passing fraction though the 45 μm sieve, and the second is due to the retention of heavy sediments in the elutriation column. Therefore, all samples experienced greater sample mass reduction with elutriation compared to sieving alone, with the difference being most pronounced for sandy samples, resulting in high sample mass reduction in both sandy and clayey soils, and lesser reduction for loamy soils. For example, the sandiest soil, AR2, experienced a 97.3% reduction in sample mass, and the most clayey soil, AB2, experienced a 90.1% reduction in mass. The lowest sample mass reduction with elutriation was recorded for AR1 (59.0%) which was still 4.96 times greater than the reduction achieved from sieving alone (11.9%).
Sample mass reduction by sieving alone, ΔmSieving, was tightly negatively correlated to sand content (R2 = 0.997) (Fig. 1B). This is to be expected as the 45 μm mesh used for sieving is close to the 53 μm lower limit for what constitutes sand grains. Because the sieve size was so close to this cutoff, nearly all soil grains retained must have been sand grains. Therefore, soils with 100% sand content should demonstrate no mass removal by sieving alone, whereas soils with 0% sand content should experience nearly 100% mass removal. The tight correlation also confirms that the power and amplitude of sonication was sufficient to fully disrupt clay aggregates at least down to the size of 45 μm, or else this correlation would be broken due to the retention of clay aggregates in the sieve. Furthermore, this correlation supports the hypothesis that elutriation primarily acts on the sand fraction. Because sand is the main constituent left after sieving, the large discrepancy in mass reduction must be due to removal of some fraction of sand grains.
The sample mass removed solely by retention within the elutriation column was greatest for sandy samples (Fig. 1C). For example, elutriation alone removed 94.7% of the soil mass of the sandiest sample, AR2, but only removed 2.52% of the soil mass of the least sandy sample, AB2, with the remaining 87.6% reduction being solely attributable to the finer fraction passing through the 45 μm sieve. This is still a 20.3% decrease in sample mass compared to sonication and sieving alone. The hypothesis that sand content is responsible for mass reduction by the elutriation mechanism is further supported by the strong positive correlation between sample mass reduction by elutriation, ΔmElutriation, and soil sand content (R2 = 0.807). Unlike sieving, however, grain size is not the only important factor in elutriation performance, as other properties such as density, sphericity, and surface roughness all play important roles in settling behavior. Furthermore, the grain size distribution would need to be considered to predict the mass removal of this process. A more precise analytical model of mass reduction by elutriation would likely follow a more complicated function than a simple line of best fit.
3.3 Effects of elutriation on particle quantity and size distribution
No statistically significant differences were observed between the two extraction methods (sieving + digestion + flotation and elutriation + digestion + flotation) with regards to extracted particle quantity, size distribution, or visual presentation (Fig. 2A–C). In most samples, elutriated soils resulted in slightly greater particle yield compared with the elutriated soils (Fig. 2A). The BC sample was a notable exception as it showed a modest improvement over the sieving (p = 0.085). The results here suggest that pretreatment with elutriation prior to a typical extraction protocol involving digestion and flotation does not significantly affect the extracted quantity of microplastics across a wide range of soil types, provided sufficient upflow velocity.
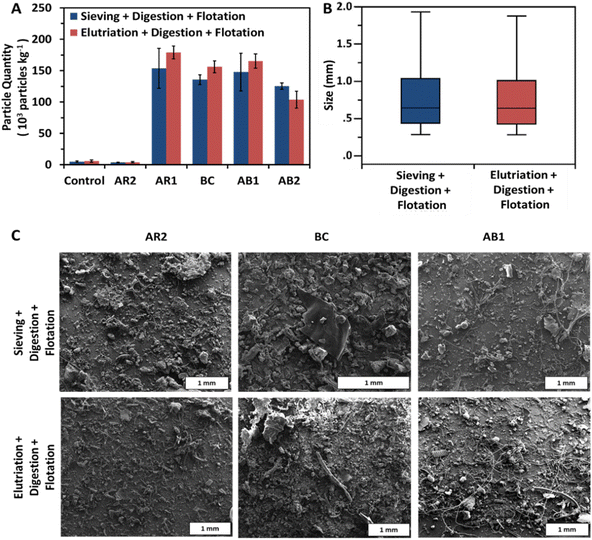 |
| Fig. 2 Fluorescent particle quantity measured by fluorescence microscopy and automated particle counting (A), microplastic size distribution for all particles for each method, measured by longest dimension (B), and SEM images comparing extraction methods for AR2, BC, and AB1 samples, scale bar in each picture denotes 1 mm. Error bars denote standard error. Arkansas River 2 (AR2), Arkansas River 1 (AR1), Boomer Creek (BC), Applied Biosolids 1 (AB1), and Applied Biosolids 2 (AB2). | |
Particle size distributions reveal little to no difference between methods (Fig. 2B). Similar numbers of particles were observed across all samples (n = 15
292 for sieving and n = 15
135 for elutriation). The distribution of particle sizes for both methods was skewed toward large particles and displayed nearly identical medians (0.641 mm for sieving and 0.637 mm for elutriation). Furthermore, the variation was similar between methods with almost identical interquartile ranges (0.591 mm for sieving and 0.581 mm for elutriation). These data suggest that this elutriation method does not bias samples toward smaller particles as may be expected from a gravity-based separation technique.
The qualitative similarities between methods are further corroborated by SEM imaging, indicating no visual differences between elutriated and non-elutriated extracted samples (Fig. 3). No marked differences are noted in terms of filter cleanliness, polymer size, polymer shape, or abundance.
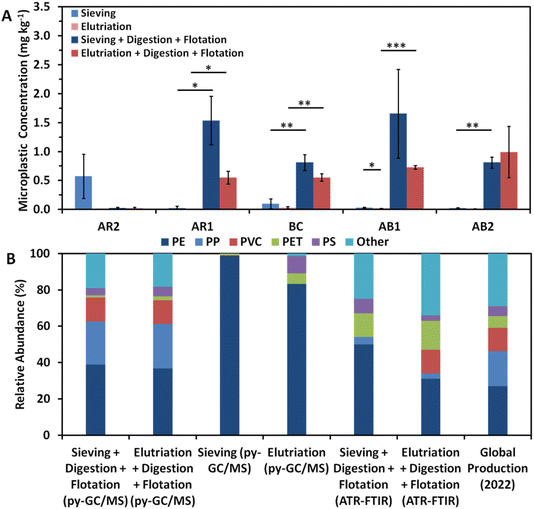 |
| Fig. 3 Soil microplastic concentration (mg kg−1) measured by py-GC/MS for all methods (A). Relative abundance for all methods in terms of concentration (py-GC/MS) and number of identified polymers (ATR-FTIR) compared to 2022 global polymer production (B). * denotes p < 0.05, ** denotes p < 0.01, *** denotes p < 0.005. Error bars denote standard error. | |
3.4 Comparison of extraction methods
3.4.1 Microplastic concentration.
The measured concentrations of microplastics (mg microplastic kg dry soil−1) were greater, sample variability was lower, and polymer distribution was more representative in the extracted samples (both in terms of mass and quantity) compared to bulk samples (Fig. 3A–B). The calculated soil microplastic concentrations were, on average, 32 times greater when samples were extracted prior to analysis with py-GC/MS, and of the ten samples, six of these values were statistically significant (Fig. 3A). Furthermore, the coefficient of variation was 58% for extracted samples and 83% for only pretreated samples. The greater variability was likely due to the relatively low concentration of microplastics and small sample mass which was analyzed by py-GC/MS, leading to unrepresentative measurements of polymer mass in pretreated soils.
No statistically significant differences in concentration were observed between methods in fully extracted samples (Fig. 2A). Unlike particle quantity, however, sieved soils generally yielded more microplastic mass than elutriated soils with the exception of AB2, though the high standard error makes this difference difficult to meaningfully discern. Though no statistically significant (p < 0.05) differences were observed, it is worth mentioning that sieving outperformed in nearly all samples, especially AR1 (p = 0.068) and BC (p = 0.097), but our study may be underpowered for such conclusions.
3.4.2 Polymer distribution.
The relative abundance of polymers in fully extracted samples more closely resembled global production of polymers (Plastics Europe, 2023) than did pretreated soils, though neither matched exactly (Fig. 3B). Differential half-lives of polymers in the environment may explain some discrepancy between production and environmental contamination. The observed distributions in extracted samples show some departure from global production, but are similar to one another. It should be noted that current global production is only a proxy for what may be expected in the environment and is not necessarily representative of the distribution of polymers present in the selected locations. Pretreated soils showed little resemblance to either global production or the extracted samples. This is likely due to the small mass of sample analyzed by py-GC/MS, leading to results not representative of the bulk soils.
More polymers were identified and a more representative distribution was observed in elutriated samples than sieved samples with ATR-FTIR analysis (Fig. 3B). It should be noted that ATR-FTIR could only be performed on fully-extracted samples. Across all samples, 33% more polymers were confirmed through ATR-FTIR analysis (n = 24 for sieving and n = 32 for elutriation). Furthermore, the distribution more closely mirrored that of expected concentration, and ATR-FTIR failed to identify any PVC particles in sieved samples, whereas four PVC particles were identified in elutriated samples.41 One hypothesis is that sample cleanliness (in terms of Si/C ratio) impacts the ability of ATR-FTIR to accurately identify polymers, as silicon dioxide has the potential to interfere with ATR-FTIR measurements. This hypothesis is supported by the negative correlation (R2 = 0.59) between Si/C ratio and percentage of particles positively identified by ATR-FTIR analysis (Fig. S1†). This would directly imply that elutriated samples contain a lower quantity of silicon; however, it was observed that both extraction methods exhibited broad, overlapping ranges of Si/C ratios, though the mean was higher for elutriation (Si/C = 0.140) compared to sieving (Si/C = 0.095) (Fig. S2†).
4 Conclusions
The data provided here demonstrate that pretreatment with elutriation provides greater soil mass reduction compared to sieving. Furthermore, elutriation did not result in any statistically-significant differences in extraction efficiency across a broad range of soil textures and organic matter contents, nor did it affect the particle size or polymer distributions compared to non-elutriated soils. These data also emphasize the importance of extracting microplastics from samples prior to analysis with py-GC/MS, fluorescent imaging, or ATR-FTIR. More positive identifications of polymers were made with ATR-FTIR analysis for elutriated samples compared to sieving as well. The mass reduction provided by elutriation allows for the processing of larger sample volumes, leading to more representative samples, greater sensitivity, and the potential to lessen reagent usage in subsequent extraction steps due to the decrease in sand grains and large pieces of organic matter.
Author contributions
Kyle Forsythe: conceptualization, data curation, formal analysis, investigation, methodology, validation, writing-original draft, visualization; Mason Egermeier: investigation; Marcus Garcia: investigation; Rui Liu: Investigation, Matthew Campen: resources; writing – review & editing, Matteo Minghetti: resources, writing – review & editing; Andrea Jilling: methodology, writing – review & editing; and Jorge Gonzalez-Estrella: conceptualization, data curation, formal analysis, funding acquisition, project administration, resources, supervision, visualization, writing – review & editing.
Conflicts of interest
There are no conflicts to declare.
Acknowledgements
This study was funded by the National Institute on Minority Health and Health Disparities (Award Number P50MD015706), the Center for Metals in Biology and Medicine (Award Number NIGMS P20GM130422), and the U.S. Geological Survey (Grant/Cooperative Agreement G21AP10597). The content is solely the responsibility of the authors and does not necessarily represent the official views of the National Institutes of Health nor of the U.S. Geological Survey. Marcus Garcia was supported by ASERT number K12 GM088021. The content is solely the responsibility of the authors and does not necessarily represent the official views of the National Institutes of Health nor of the U.S. Geological Survey.
References
- A. A. Koelmans, N. N. H. Mohamed, E. Hermsen, M. Kooi, S. M. Mintenig and J. De France, Microplastics in freshwaters and drinking water: Critical review and assessment of data quality, Water Res., 2019, 155, 410–422 CrossRef CAS PubMed.
-
L. An, Q. Liu, Y. Deng, W. Wu, Y. Gao and W. Ling, Sources of microplastic in the environment, In Microplastics in terrestrial environments: emerging contaminants and major challenges, The Handbook of Environmental Chemistry, 2020, vol. 95, pp. 143–159 Search PubMed.
- Q. T. Birch, P. M. Potter, P. X. Pinto, D. D. Dionysiou and S. R. Al-Abed, Sources, transport, measurement and impact of nano and microplastics in urban watersheds, Rev. Environ. Sci. Bio/Technol., 2020, 19(2), 275–336 CrossRef CAS PubMed.
-
M. A. Browne, Sources and Pathways of Microplastics to Habitats, In Marine Anthropogenic Litter, ed. M. Bergmann, L. Gutow and M. Klages, Springer International Publishing, Cham, 2015, pp. 229–244 Search PubMed.
- P. Kay, R. Hiscoe, I. Moberley, L. Bajic and N. McKenna, Wastewater treatment plants as a source of microplastics in river catchments, Environ. Sci. Pollut. Res., 2018, 25(20), 20264–20267 CrossRef CAS PubMed.
- K. Zhang, A. H. Hamidian, A. Tubić, Y. Zhang, J. K. H. Fang and C. Wu,
et al., Understanding plastic degradation and microplastic formation in the environment: A review, Environ. Pollut., 2021, 274, 116554 CrossRef CAS PubMed.
- J.-J. Guo, X.-P. Huang, L. Xiang, Y.-Z. Wang, Y.-W. Li and H. Li,
et al., Source, migration and toxicology of microplastics in soil, Environ. Int., 2020, 137, 105263 CrossRef CAS PubMed.
- Y.-N. Kim, J.-H. Yoon and K.-H. J. Kim, Microplastic contamination in soil environment – a review, Soil Sci. Annu., 2020, 71(4), 300–308 CrossRef CAS.
- C. M. Rochman, Microplastics research—from sink to source, Science, 2018, 360(6384), 28–29 CrossRef CAS PubMed.
- A. Besley, M. G. Vijver, P. Behrens and T. Bosker, A standardized method for sampling and extraction methods for quantifying microplastics in beach sand, Mar. Pollut. Bull., 2017, 114(1), 77–83 CrossRef CAS PubMed.
- N. N. Phuong, L. Poirier, F. Lagarde, A. Kamari and A. Zalouk-Vergnoux, Microplastic abundance and characteristics in French Atlantic coastal sediments using a new extraction method, Environ. Pollut., 2018, 243, 228–237 CrossRef CAS PubMed.
- M. Lavoy and J. Crossman, A novel method for organic matter removal from samples containing microplastics, Environ. Pollut., 2021, 286, 117357 CrossRef CAS PubMed.
- F. Pfeiffer and E. K. Fischer, Various Digestion Protocols Within Microplastic Sample Processing—Evaluating the Resistance of Different Synthetic Polymers and the Efficiency of Biogenic Organic Matter Destruction, Front. Environ. Sci., 2020, 8 DOI:10.3389/fenvs.2020.572424.
- A. Bottone, J.-F. Boily, A. Shchukarev, P. L. Andersson and J. Klaminder, Sodium hypochlorite as an oxidizing agent for removal of soil organic matter before microplastics analyses, J. Environ. Qual., 2022, 51(1), 112–122 CrossRef CAS PubMed.
- R. R. Hurley, A. L. Lusher, M. Olsen and L. Nizzetto, Validation of a Method for Extracting Microplastics from Complex, Organic-Rich, Environmental Matrices, Environ. Sci. Technol., 2018, 52(13), 7409–7417 CrossRef CAS PubMed.
- R. L. Coppock, M. Cole, P. K. Lindeque, A. M. Queirós and T. S. Galloway, A small-scale, portable method for extracting microplastics from marine sediments, Environ. Pollut., 2017, 230, 829–837 CrossRef CAS PubMed.
- G. Grause, Y. Kuniyasu, M.-F. Chien and C. Inoue, Separation of microplastic from soil by centrifugation and its application to agricultural soil, Chemosphere, 2022, 288, 132654 CrossRef CAS PubMed.
- B. Quinn, F. Murphy and C. Ewins, Validation of density separation for the rapid recovery of
microplastics from sediment, Anal. Methods, 2017, 9(9), 1491–1498 RSC.
- S. S. Monteiro, T. Rocha-Santos, J. C. Prata, A. C. Duarte, A. V. Girão and P. Lopes,
et al., A straightforward method for microplastic extraction from organic-rich freshwater samples, Sci. Total Environ., 2022, 815, 152941 CrossRef CAS PubMed.
- M. O. Rodrigues, A. M. M. Gonçalves, F. J. M. Gonçalves and N. Abrantes, Improving cost-efficiency for MPs density separation by zinc chloride reuse, MethodsX, 2020, 7, 100785 CrossRef CAS PubMed.
- I. C. Frost, An elutriating tube for the specific gravity separation of minerals, Am. Mineral., 1959, 44(7–8), 886–890 CAS.
- M. Colakyan and O. Levenspiel, Elutriation from fluidized beds, Powder Technol., 1984, 38(3), 223–232 CrossRef CAS.
- J. H. E. Cartwright, Stokes' law, viscometry, and the Stokes falling sphere clock, Philos. Trans. R. Soc., A, 2020, 378(2179), 20200214 CrossRef PubMed.
- A. Bouzas, J. Ribes, J. Ferrer and A. Seco, Fermentation and elutriation of primary sludge: Effect of SRT on process performance, Water Res., 2007, 41(4), 747–756 CrossRef CAS PubMed.
- J. W. Seinhorst, Modifications of the Elutriation Method for Extracting Nematodes From Soil, Nematologica, 1962, 8(2), 117–128 Search PubMed.
- M. Claessens, L. Van Cauwenberghe, M. B. Vandegehuchte and C. R. Janssen, New techniques for the detection of microplastics in sediments and field collected organisms, Mar. Pollut. Bull., 2013, 70(1), 227–233 CrossRef CAS PubMed.
- E. Hengstmann, M. Tamminga, C. vom Bruch and E. K. Fischer, Microplastic in beach sediments of the Isle of Rügen (Baltic Sea) - Implementing a novel glass elutriation column, Mar. Pollut. Bull., 2018, 126, 263–274 CrossRef CAS PubMed.
- M. Kedzierski, V. Le Tilly, P. Bourseau, H. Bellegou, G. César and O. Sire,
et al., Microplastics elutriation from sandy sediments: A granulometric approach, Mar. Pollut. Bull., 2016, 107(1), 315–323 CrossRef CAS PubMed.
- J. Tibbetts, S. Krause, I. Lynch and G. H. Sambrook Smith, Abundance, Distribution, and Drivers of Microplastic Contamination in Urban River Environments, Water, 2018, 10(11), 1597 CrossRef CAS.
- S. Uddin, S. W. Fowler, M. F. Uddin, M. Behbehani and A. Naji, A review of microplastic distribution in sediment profiles, Mar. Pollut. Bull., 2021, 163, 111973 CrossRef CAS PubMed.
- S. Wang, H. Chen, X. Zhou, Y. Tian, C. Lin and W. Wang,
et al., Microplastic abundance, distribution and composition in the mid-west Pacific Ocean, Environ. Pollut., 2020, 264, 114125 CrossRef CAS PubMed.
- M. A. Cashman, K. T. Ho, T. B. Boving, S. Russo, S. Robinson and R. M. Burgess, Comparison of microplastic isolation and extraction procedures from marine sediments, Mar. Pollut. Bull., 2020, 159, 111507 CrossRef CAS PubMed.
- F. Radford, L. M. Zapata-Restrepo, A. A. Horton, M. D. Hudson, P. J. Shaw and I. D. Williams, Developing a systematic method for extraction of microplastics in soils, Anal. Methods, 2021, 13(14), 1695–1705 RSC.
- V. Hidalgo-Ruz, L. Gutow, R. C. Thompson and M. Thiel, Microplastics in the marine environment: a review of the methods used for identification and quantification, Environ. Sci. Technol., 2012, 46(6), 3060–3075 CrossRef CAS PubMed.
- L. Ding, R. Mao, S. Ma, X. Guo and L. Zhu, High temperature depended on the ageing mechanism of microplastics under different environmental conditions and its effect on the distribution of organic pollutants, Water Res., 2020, 174, 115634 CrossRef CAS PubMed.
- K. Munno, P. A. Helm, D. A. Jackson, C. Rochman and A. Sims, Impacts of temperature and selected chemical digestion methods on microplastic particles, Environ. Toxicol. Chem., 2018, 37(1), 91–98 CrossRef CAS PubMed.
- J. I. Santisteban, R. Mediavilla, E. López-Pamo, C. J. Dabrio, M. B. R. Zapata and M. J. G. García,
et al., Loss on ignition: a qualitative or quantitative method for organic matter and carbonate mineral content in sediments?, J. Paleolimnol., 2004, 32(3), 287–299 CrossRef.
- M. J. Morra, R. R. Blank, L. L. Freeborn and B. Shafii, Size Fractionation of Soil Organo-Mineral Complexes Using Ultrasonic Dispersion, Soil Sci., 1991, 152(4), 294–303 CrossRef CAS.
- J. C. Prata, J. P. da Costa, A. C. Duarte and T. Rocha-Santos, Methods for sampling and detection of microplastics in water and sediment: A critical review, TrAC, Trends Anal. Chem., 2019, 110, 150–159 CrossRef CAS.
- J. Quiambao, K. Z. Hess, S. Johnston, E. El Hayek, A. Noureddine and A.-M. S. Ali,
et al., Interfacial Interactions of Uranium and Arsenic with Microplastics: From Field Detection to Controlled Laboratory Tests, Environ. Eng. Sci., 2023, 40(11), 562–573 CrossRef CAS PubMed.
-
PlasticsEurope, Plastics Europe: Plastics – the Facts 2022, PlasticsEurope AISBL, Brussels, Belgium, 2002 Search PubMed.
|
This journal is © The Royal Society of Chemistry 2024 |
Click here to see how this site uses Cookies. View our privacy policy here.