DOI:
10.1039/D4VA00244J
(Tutorial Review)
Environ. Sci.: Adv., 2024,
3, 1652-1668
Microplastics analysis: from qualitative to quantitative
Received
30th June 2024
, Accepted 29th September 2024
First published on 30th September 2024
Abstract
Microplastics (MPs) are a class of non-degradable pollutants of global concern. MPs ubiquitously exist in the natural environment and can get inevitably transferred to the human body. Although the impacts of MPs on the ecosystem are not clearly defined yet, their toxicity to human health is becoming a concern. The complexity of MPs caused by the presence of heavy metals and organic pollution further makes it a great challenge to analyze MPs rapidly and accurately. Demanding pretreatment and insufficient data acquisition seriously hinder the precise understanding of the risk of MPs to the ecosystem and human health. Herein, this review covers recent advances in the separation of MPs, identification, and quantification methods while discussing their mechanisms and efficacy. Furthermore, this review details the use of Fourier transform infrared spectroscopy, Raman spectroscopy, and mass spectrometry for the qualitative and quantitative analysis of MPs, offering a comprehensive overview of the up-to-date strategies that overcome current technological limitations. Finally, challenges and prospective outlooks for the rapid and accurate analysis of MPs are presented.
Environmental significance
The pervasive presence of microplastics in diverse environmental matrices poses significant threats to ecosystems and human health. This review, titled “Microplastics Analysis: From Qualitative to Quantitative,” provides a comprehensive overview of the methods used to identify and quantify microplastics, highlighting the advancements from qualitative assessments to quantitative analyses. By synthesizing current methodologies and addressing the challenges in microplastic detection and measurement, this paper underscores the urgent need for standardized approaches to better understand the distribution, sources, and impacts of microplastics. The insights provided herein are crucial for informing policy decisions, improving waste management practices, and developing mitigation strategies to protect environmental and public health.
|
1. Introduction
Plastics are essential materials for our lives and play an important part in the smooth operation of modern society and industry.1 However, insufficient plastic management, such as improper disposal or recycling, has resulted in the amount of plastic waste rising from 156 in 2000 to 353 million tons in 2019.2–6 Owing to their non-degradability, plastics can persist for centuries and undergo complex physical, chemical, and biological processes, producing plastic particles with tiny diameters. It is widely acknowledged in numerous studies that plastic particles smaller than 5 mm are MPs,7 which have made their way everywhere: in Arctic snow and Antarctic ice,8 in deep-sea sediments,9 in sea fishes,10 in tap and bottled water,11,12 in tomatoes,13 and even in human placenta14 and blood.15 The sources of MPs can be divided into primary and secondary MPs. Primary MPs refer to purposely manufactured plastics of microscopic sizes, such as microbeads found in facial cleansers and cosmetics as well as microscopic scrubbers in airborne spray media.16 Secondary MPs are formed when large plastic debris undergoes mechanical abrasion, photooxidation, or biological processes. This can usually result in the breakdown of plastic into smaller fragments, such as those released from mulch films into soil, and the MPs are discharged from plastic wastes in the ocean.17 The characteristics of MPs found in some representative matrices are detailed in Table 1.
Table 1 Typical characteristics of MPs found in representative matricesa
Classification |
Samples |
Abundance |
Size |
Composition |
Ref. |
PE for polyethylene; PP for polypropylene; PS for polystyrene; PES for polyethersulfone resin; PET for polyethylene terephthalate; PU for polyurethane; PA for polyamide/nylon; EAA for ethylene acrylic acid copolymer.
|
Environment matrice and organism |
Bohai sea |
0.33 ± 0.34 items per m3 |
0.05–235 mm |
PE, PP, PS |
18
|
Yangtze estuary (surface water) |
0.48 × 103–21.52 × 103 items per m3 |
<0.5 mm |
PES |
19
|
Air (Outdoor, Shanghai, China) |
0–4.18 items per m3 |
0.02–9.96 mm |
PET, PU, PA |
20
|
Soil (Shouguang, China) |
310–5698 items per kg |
<5 mm |
PP, EAA, PE, PS, PET |
16
|
Atlantic croaker (Texas Gulf coast) |
0.87 items per fish |
<5 mm |
PE, PP |
21
|
Crayfish |
16.1 items per crayfish |
— |
PE, PET |
22
|
Crassostrea gigas |
1.88 ± 1.58 items per g |
0.02–1.32 mm |
PE, PP, PA |
23
|
Food |
Milk powder |
70 ± 30 items per kg (boxed); 40 ± 30 items per kg (canned) |
— |
PE |
24
|
Egg |
11.67 ± 3.98 items per egg |
0.05–0.1 mm |
PE |
25
|
Milk |
6.5 ± 2.3 items per L |
0.1–5 mm |
PS, PES |
26
|
White wine |
5857 items per L |
— |
PE |
27
|
The extensive prevalence of MPs poses distinct risks to ecosystems and human health.28 The tiny size and large surface area of MPs make them easily adsorb heavy metal ions and organic pollutants,29,30 resulting in severe threats to plants and animals in aquatic and terrestrial environments.31–33 Phytophages and animals of higher trophic levels ingest these tiny plastic particles, enriching MPs in advanced organisms and cycling them.34 For instance, numerous aquacultured fish species, such as common carp, crucian carp, and silver carp, have been found to accumulate MPs, with average counts of 2.5 ± 1.3, 1.9 ± 1.0, and 3.8 ± 2.0 items per individual, respectively.35 MPs are also detected in 33 of 34 commercial fish species in the South Pacific. In each fish body, 2.4 ± 0.2 microplastic items were found on average.36
Furthermore, MPs present interrelated hazards to the health of ecosystems, humans, and animals.37 MPs enter the human body in three main ways, which are inhalation, ingestion, and dermal contact.38 Catarino et al. discovered that during the meal period, the potential of humans to inhale MPs resulting from household dust was 3 to 15 times more than that to ingest MPs from eating mussels.39 Cox et al. analyzed the American diet and estimated that individuals consume a range of 39
000 to 52
000 MPs annually through ingestion, whereas inhalation exposure is estimated to be between 35
000 and 69
000 MPs per year.40 Regarding dermal contact, the quantification of human exposure to MPs through the skin is still unclear due to factors such as ethical constraints, sample biosecurity, and methodological limitations.41 Numerous in vitro and in vivo studies have demonstrated that MPs can cause a range of biological effects, including metabolic disorders, oxidative stress, inflammation, tissue damage, and disruption of intestinal flora.42,43 Therefore, more research efforts should be made to investigate the risks associated with MPs.
The analytical methods for MPs in complex matrices are essential for fully understanding the ecological impacts and health risks of MPs.44 MPs as a complex include polymers and additives, heavy metals and/or organic pollutants adsorbed,45,46 colonized pathogenic and/or microorganisms,47 and fragmentation and degradation products (biodegradation, UV radiation, and mechanical abrasion).48 MPs also exhibit diverse forms, such as spheres, flakes, fibres, and threads.49 Thus, MPs are highly varied and complex composite contaminants, which poses significant challenges for their analysis across different matrices. Separating MPs from complex matrices, such as food, organisms, and environmental samples like sediment and effluent, is also difficult, particularly due to their tiny size effect.50 Recently, several studies have been conducted on analytical methods for MPs, with a part or whole emphasis on identification and quantification.51,52 However, a comprehensive understanding of the entire procedure involved in analyzing MPs in complex matrices, which is crucial for establishing scientific standards, has only been partially explored thus far.
Recently, some reviews have been published on MP analysis, with an emphasis on either separation or chemical identification.53,54 This review provides a critical overview of the literature on analytical techniques of MPs in environmental and food matrices, emphasizing the latest methods for MP separation, identification, and quantification (Fig. 1). It highlights the benefits and limitations of these methods, as well as the technological challenges. The review also offers perspectives on future challenges and opportunities and outlooks on strategic goals for rapid and precise quantitative analysis of MPs.
 |
| Fig. 1 Schematic representation that summarizes the separation, identification and quantification methods established for the analysis of MPs, which are introduced in the review. | |
2. Methods for separation of MPs
MPs exist ubiquitously in the environment and even in foods.55 A greater challenge is that MPs are persistent and pernicious despite their tiny size.56 Therefore, it is essential to develop and select suitable methods to separate MPs from complex matrices to understand their distribution and hazardous effects. Although no standardized separation method exists, various techniques for separating MPs from the environment and foods have been developed. The separation process usually involves pretreatment and purification using methods like density flotation, membrane filtration, oil leaching and pressurized fluid extraction, magnetic separation and electrostatic separation (Table 3). These methods will be discussed and compared in detail, focusing on operation steps, advantages, disadvantages, and application scope.
2.1 Sample pretreatment
Pretreatment of the environment and food samples represents a crucial step toward separating MPs from real-world matrices, which can remove organic matters for reduced interference without affecting MPs. The pretreatment process can also concentrate MPs, making it easy to separate and analyze them. Sample pretreatment based on chemical reactions usually involves the digestion of organic matter using redox, enzyme digestion, alkaline digestion, and acid digestion, as shown in Table 2. The reagents usually employed in the redox method include 30% H2O2 and NaClO4. However, Fenton's reagent, which consists of 0.05 M Fe2+ and 30% H2O2, can produce hydroxyl radicals and other reactive oxidizing substances that exhibit significantly higher oxidation potential than H2O2.73,74 Enzyme digestion, utilizing proteases, cellulases, and oxidizing agents, is as effective for tougher organic matter.75 Unlike acid digestion by HNO3, which may hydrolyze or oxidize MPs, alkaline digestion using reagents like KOH and NaOH can effectively decompose organic matter without affecting MPs.76 A proper digestion method can improve the pretreatment efficiency depending on the characteristics of the sample. The redox and enzyme digestion methods are preferred for samples containing organic matter, such as soil, sediment and fish.16,58,59 Acid or alkaline digestion is suitable for easier-to-digest products, such as scallop soft tissues, fruits, and vegetables.15,16,59,67
Table 2 Detailed information on the sample pretreatment methods for MPs in representative matrices
Pretreatment methods |
Operation/mechanism |
Characteristics |
Applied sample |
Ref. |
Redox |
Reaction with 30% H2O2, NaClO4, or Fenton's reagent under stirring at above 50 °C for 12–24 h |
Simple operation procedure, high digestion efficiency, and wide application |
Soil, freshwater sediment, scallops, edible salt and fish |
16 and 57–59 |
Enzymatic digestion |
Reaction with protease and/or cellulase at suitable temperature and pH under shaking, followed by adding H2O2 or NaClO4 |
Applicable to marine organisms and their tissues, and high digestion efficiency |
Stormwater, sediment, cuttlefish, seaweed and nori |
60–63
|
Digestion by alkali and acid |
Incubation with 10% KOH (or NaOH), or 65% HNO3 for over 24 h, followed by adding 30% H2O2, NaClO4, or CH2Cl2 under stirring |
Applicable to easy-to-digest samples, and long treatment |
Sewage, soil, edible shellfish, farmed oysters, coastal mussels, fish, edible fruits and vegetables |
59 and 64–69 |
Ultrasound extraction |
Ultrasonic propagation under an appropriate ultrasonic frequency and power in water or organic solvents for generating strong shock waves and tiny jets, peeling off MPs from the original attached substrate or impurities |
Cost-effective, minimal sample destruction, no need for complex chemical reactions |
Wastewater, soil, honey, beer, milk |
16, 70 and 71 |
SPME |
Extracting target compounds by inserting coated fibers to adsorb MPs, and desorbing MPs under specific conditions for the subsequent chromatography-MS analysis |
High sensitivity, rapidity, solvent-free and usually in conjunction with chromatography-MS. |
Soil |
72
|
Table 3 Detailed information on purification of MPs in complex matrices
Method |
Reagent/tool |
Mechanism |
Advantage |
Disadvantage |
Applied sample |
Ref. |
Density flotation |
NaCl solution (1.2 g cm−3) |
Separation by utilizing density differences between substances and MPs |
Low cost, easy to operate, non-toxic to humans |
Poor separation of high-density MPs |
Soil deposits |
99
|
NaI solution(1.8 g cm−3) |
Good separation efficiency for high-density MPs |
Toxic, costly, and polluting |
100
|
ZnCl2 solution (1.55 g cm−3) |
Good separation efficiency for high-density MPs |
High cost and polluting |
85
|
Membrane filtration |
Glass fiber filter membrane (0.7–25 μm) |
Vacuum pumps are utilized to provide pressure to create a differential pressure that allows MPs to pass through the apertures in the membrane and be separated from the rest of the material |
The most commonly used filtration membrane, which effectively retains micro/nano plastics on the membrane |
Poor filtration of small particle-size MPs |
Tap water, beer, sea salt |
77 and 101 |
Small size filter membrane (nitrocellulose filter membrane 0.45 μm, aluminum oxide filter membrane 0.1 μm) |
Used for filtration of samples containing few impurities; can effectively filter out small plastic particles |
Can release fibers to contaminate samples |
Beer, mussels |
77
|
Polycarbonate (PC) filters (1.2–8 μm) |
Mostly used for filtration of MPs in municipal drinking water |
Interference with infrared signals, low filtration efficiency |
Drinking water in cities |
102
|
Oil extraction |
Rapeseed oil, castor oil, and olive oil |
Enrichment of MPs via hydrophobic interaction to the oil phase by shaking |
Rapid separation, and high recovery (up to 99%) |
Possible destruction of plastics by oil phase; poor separation efficiency for dense MPs |
Seawater and soil sediment |
53, 103 and 104 |
Magnetic separation |
Iron oxide nanoparticles (recommended size of separated MPs: d < 20 μm) |
Fewer applications are currently used to detect seawater samples and soil sediments |
Low cost, rapid separation and reusability |
Possible interference by magnetic nanoparticles with subsequent analysis |
Seawater |
53
|
Electrostatic separation |
KWS |
Electrostatic interaction with metal or plastic separator |
Rapid separation with independence on plastics size, and high recovery (up to 99%) |
High cost |
Drinking water, soil sediment |
5
|
Pressurized liquid extraction |
Pressurized fluid extractor |
Extraction by a high-pressure fluid and separation from the liquid by altering temperature or pressure |
Separation without dependence on particle size |
Possible morphological change of MPs |
Soil sediment, and urban compost |
94–96 and 105 |
Ultrasound extraction and solid phase microextraction (SPME) are representative pretreatment methods that avoid the need for complex chemical reactions. Both of the two methods exert minimal disruption effect on the sample. In particular, ultrasonic treatment is highly suggested for eliminating impurities that have been absorbed onto the surfaces of MPs, suitable for separating MPs from both environmental and food samples, including wastewater, soil, honey, and milk.16,71 SPME is solvent-free, and usually used in conjunction with chromatography-mass spectrometry (MS).77 SPME has been used for microextraction of MPs from soil.72
2.2 Density flotation
The density flotation method is capable of separating materials based on the density difference between MPs and other substances, as illustrated in Fig. 2a. This method involves the process of phase separation, in which a sample containing MPs is mixed with salt solutions like NaCl or NaI, allowing low-density MPs to rise while those with high density to settle. This can help successfully separate them and then filter them using a membrane.79 The U.S. National Oceanic and Atmospheric Administration (NOAA) has developed a standard method for separating marine MPs, which is applicable to the separation of MPs from wastewater. Typically, samples are digested with H2O2 using an aqueous ferrous solution (Fe(II)) as a catalyst. Subsequently, a NaCl or ZnCl2 solution is used to obtain an extracting solution containing MPs, which is then filtered through membranes with pore sizes ranging from 0.7 to 125 μm. It is worth noting that the efficacy of density flotation greatly depends on the type and concentration of the salt solution. Saturated NaCl solution (1.2 g cm−3) is suitable for separating MPs with relatively low density and is widely recommended because of its low cost, safety, and easy operation. However, it is less effective for high-density MPs, such as PET (1.38 g cm−3) and PVC (1.35 g cm−3).80 Saturated NaI (1.8 g cm−3) and ZnCl2 solutions (1.55 g cm−3) are efficient ways to separate high-density MPs. For example, a study introduced a portable device using a saturated ZnCl2 solution that yields a 95.8% separation efficiency.81 Nevertheless, ZnCl2 may react with carbonates in the sample, leading to sedimentation, and its corrosiveness may reduce flotation efficacy.82 Furthermore, this strategy has other drawbacks, including high costs, toxicity, and environmental damage owing to waste liquid disposal.65 Kedzierski et al. reduced the cost of NaI-based density flotation by recycling and reuse of NaI solution.78 In that work, the efficiency for MP extraction was as high as over 90%. Meanwhile, a loss of 4.8% of NaI after 10 times-recycling was observed (Fig. 2b), greatly reducing the cost and waste of the method.
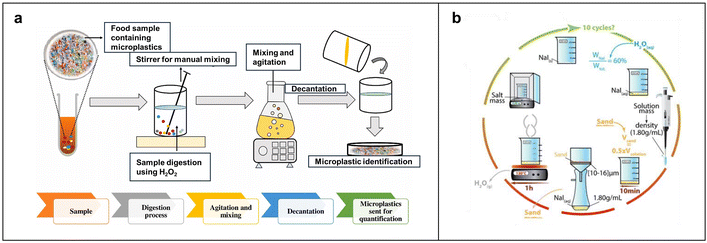 |
| Fig. 2 Flow charts of MPs separation based on density flotation. (a) Basic flow of density flotation. Reproduced with permission,77 Copyright 2021, Elsevier. (b) The detailed process of separating MPs via NaI-enabled density flotation. The recycling and reuse of NaI in the process can help reduce the cost and waste of the method. Reproduced with permission,78 Copyright 2016, Elsevier. | |
Therefore, the evaluation of a separation method for practical applications depends on many factors, including environmental impact, cost, and effectiveness.
2.3 Membrane filtration
Membrane filtration collects MPs on various membrane filter surfaces through a pressure difference generated by vacuum pumps.83 This method is commonly used to separate MPs from environment and food samples, due to its simple operation and lack of need for a complex device. Based on pore size selection, membrane filtration separates MPs from other substances, as shown in Fig. 3a. This process can be classified as ultrafiltration, microfiltration, nanofiltration, and reverse osmosis. Membrane filters are made of various materials, such as glass fiber, polycarbonate, and cellulose. Each material has unique characteristics. For example, glass fiber with rough surfaces can potentially retain impurities and release fibers that may contaminate the sample. Polycarbonate lacks hydrophilicity, reducing its filtration efficiency and effectiveness. This may result in prolonged water molecules on the surface, which in turn increases pollutant adsorption and clogging of the membrane, thus reducing its lifespan. It is worth noting that polycarbonate may interfere with infrared signals, making the detection and identification of MPs challenging. Nylon and alumina membranes lack chemical corrosion resistance.85
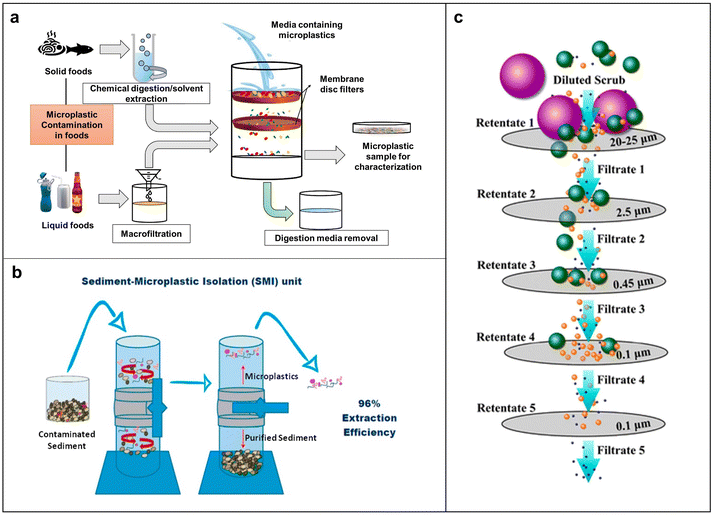 |
| Fig. 3 Working principle of various filtration methods for MP separation. (a) Mechanistic scheme for the separation of MPs via membrane filtration. Reproduced with permission,77 Copyright 2021, Elsevier. (b) Schematic of SMI. (c) Scheme of the filtration process with the complex mechanism. Reproduced with permission,84 Copyright 2017, American Chemical Society. | |
Compared with the abovementioned materials, cellulose acetate and cellulose nitrate offer superior overall performance. Depending on the characteristics of a sample, a membrane with different pore sizes may be used. For example, a pore size of 1 μm to 0.5 mm is suitable for small molecule liquid matrices such as seawater, and a 5 μm-pore size is suitable for food takeaway packaging.86 Coppock et al. developed the sediment-microplastic isolation (SMI) method, a small-scale process for MP sampling in marine sediments (Fig. 3b).87 The SMI process begins with placing the equipment under a laminar flow cover to extract MPs from sediments. Each trial involves adding a dry sample (30–50 g), a clean magnetic stirring bar, and ZnCl2 solution to the purged SMI unit. Then, the mixture was left to settle until the supernatant was clarified. The clarified supernatant was then vacuum filtered, transferred to a clean Petri dish, and examined under an optical microscope, showing the effectiveness of the SMI device with an average recovery of 95.8%. This method applies to various sediment types and is suitable for laboratory and field separation of MPs from benthic samples. A multi-aperture membrane filtration process is suggested to separate MPs from complex matrix composition. Typically, it starts with a larger pore-size filter, followed by a smaller one, to avoid clogging and enhance filtration efficiency and membrane lifespan. Hernandez et al. developed a filtration device that utilized polycarbonate membranes with different pore sizes, effectively separating nanoplastics (NPs) from facial scrubs, as illustrated in Fig. 3c.84 Besides using multi-aperture membranes, combining membrane filtration with treatments, such as disk filters, sand filtration, and ozone, can enhance efficiency. Through this strategy, a study conducted by Lee and co-workers successfully removed 75–91.9% MPs in a wastewater plant.88 Wang et al. developed a sustainable, highly porous, and ultra-light sponge to eliminate MPs from environmental and food samples.89 By either filtration using the sponge pores or simply pressing the sponge in a sample containing MPs, it is possible to obtain an efficiency of up to 90% without affecting the mechanical strength of the sponge. The sponge could rapidly degrade to glucose by enzymes and completely degrade within 12 days once buried in the soil. This work represents an important step forward in using degradable materials for sustainable separation of MPs. Overall, the membrane filtration method is easy to operate and adaptable to different treatment scales and water quality conditions. It can also be easily integrated with other separation methods. Nevertheless, this method also has some limitations, including strong dependence of removal efficiency on the size distribution of MPs, and frequent occurrence of membrane fouling.
2.4 Oil leaching
Oil leaching as a density-independent method exploits the hydrophobic interaction between oil and MPs to separate MPs in soil and aquatic sediments. The separation involves shaking a mixture of oil, water, and the sample containing MPs until three-phase separation occurs. Due to their hydrophobic nature, MPs tend to migrate to the oil phase. Solvents like ethanol are then used to remove residual oil, facilitating the subsequent detection and identification of MPs. Crichton et al. introduced a petroleum-based protocol using rapeseed oil to extract MPs with a recovery rate of 96%.90 The process enriches MPs during the separation as other impurities are hydrophilic and hardly interfere with the separation. However, solid samples may clog dispensing funnels. The partition funnel limits the recovery of MPs from the upper side when hydrophilic particles are discharged as underflow. Also, residual oil traces may hinder the separation of oil film, which requires further cleaning with solvents like ethanol and hexane. Kononov et al. reported a simple method for extracting PE, PP and PVC MPs from soil using the buoyancy of canola oil and the density separation process using NaCl (Fig. 4a).92 In the system, the MPs in soil are extracted in the oil phase and separated after soil sedimentation. After ethanol rinsing and H2O2 digestion of organic adherents, the MPs can be recovered at high efficiency ranging from 76.0% to 98.7%, depending on MP composition. To reduce the amount of oil as well as to improve the efficiency of separation based on oil enrichment, Li et al. optimized the oil-water ratio, using sustainable olive oil and emulsifiers to create stable and well-dispersed olive oil microemulsions that are effective over a broad pH range and in high salt conditions.91 As depicted in Fig. 4b, upon addition of a demulsifier, hydrophobic interaction between the emulsion of olive oil with high surface area and MPs causes them to rise to the upper layer of the system, separating MPs from the aqueous environment with up to 87% efficiency. The components of MPs are further identifiable through Raman characterization. The strategy based on olive oil and rapeseed oil emulsions offers a straightforward, efficient, and sustainable approach to separating and identifying MPs.
 |
| Fig. 4 Separation and analysis of MPs based on the affinity of MPs and oil. (a) Schematic of extracting MPs from agricultural soil using rapeseed oil and unsaturated sodium chloride solution. (b) Schematic of MP separation through olive oil emulsion enrichment. Reproduced with permission,91 Copyright 2023, Elsevier. | |
2.5 Magnetic separation
Magnetic separation involves adsorbing magnetic nanoparticles onto MPs, making them magnetic, and then employing magnets to separate the composite of magnetic nanoparticles and MPs from the liquid. This method is widely used in biomedical environments and other fields because of its ease of separation, recyclability, large surface area, strong adsorption capacity, and cost-effectiveness. Grbic et al. developed a magnetic extraction method using cetyltrimethoxysilane-modified iron particles that bond to plastics through their hydrophobic surfaces to facilitate their recovery from the sample, as shown in Fig. 5a.93 A key feature of this method is its reliance on the surface area to volume ratio; smaller particles allow more iron nanoparticles to bind per unit mass of plastic, enhancing effectiveness. Shi et al. introduced a method using hydrophilic Fe3O4 magnetic nanoparticles for MP removal, which optimized the concentration and magnetization time of Fe3O4 magnetic nanoparticles, giving rise to a removal rate of 80% in environmental waters, as depicted in Fig. 5b.6 Based on the typical hydrophobic nature of plastics, magnetic Fe3O4 nanoparticles are often modified with hydrophobic ligands, such as polydopamine, carbon nanotubes, and linseed ash, which enhances their adhesion to MPs and further improves the separation efficiency. However, Fe3O4 nanoparticles with hydrophobic layers are difficult to disperse in water and costly to produce, highlighting the need for a more cost-effective and efficient magnetic separation method for MPs.
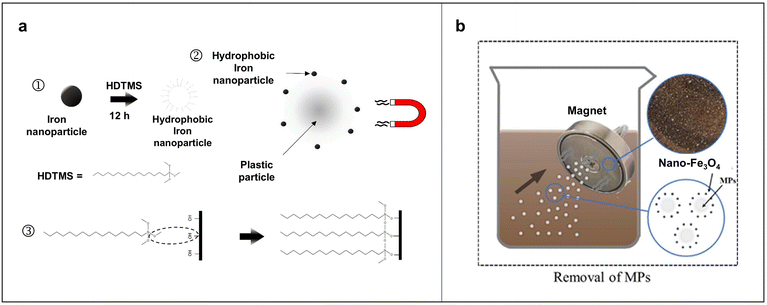 |
| Fig. 5 Typical examples of magnetic separation of MPs. (a) Schematic of the principle of using magnetic nanoparticles to remove microplastics. ① Fe nanoparticles were modified with cetyltrimethoxysilane (HDTMS) to produce hydrophobic Fe nanoparticles, which bind to the plastic through hydrophobic interactions. HDTMS binds to the nanoparticles to produce hydrophobic tails. ② The bound Fe nanoparticles allow for magnetic recycling of the MPs as the magnetic force acts on the particles. ③ Graphical representation of HDTMS bonded to OH groups on the natural oxide layer of Fe. Reproduced with permission,93 Copyright 2019, American Chemical Society. (b) Schematic representation of the process of MPs removal by magnetic nanoparticles. Reproduced with permission,6 Copyright 2021, Elsevier. | |
2.6 Pressurized liquid extraction (PLE)
PLE is often used to collect semivolatile organic compounds from solid materials at subcritical temperatures and pressures. Recently, scientists have applied this method to remove MPs from soils, sediments, and wastes. The basic PLE procedure, illustrated in Fig. 6a, is carried out at 180 °C and 1000 psi with methylene chloride as an extractant. The equipment and operating principles of PLE are depicted in Fig. 6b, where the solvent (S) and the sample (A) are first injected into the extraction cell. The extraction cell includes an oven (O) and a pressure valve (P), which together achieve the desired temperature and pressure to extract MPs from the sample. Extracted MPs are subsequently cooled and collected in a carousel. Studies have demonstrated that using PLE at 160 °C could efficiently separate MPs from samples, while extended loading at this temperature may cause the static valve to be clogged with 100 μm of glass material. To prevent this issue, it is recommended to raise extraction temperatures to the range of 180–190 °C and reduce the amount of plastic loaded.95,96 Derkies et al. developed an analytical method that combines PLE and pyrolysis gas chromatography-MS (GC-MS) for MPs in various environmental samples, including sediment, suspension, soil, and sewage sludge.94 In that work, sample separation involved a pre-extraction step with methanol, followed by PLE using tetrahydrofuran to realize the automated extraction of MPs. The limit of quantification for this method was as low as 0.007 mg g−1 for common synthetic polymers, such as PE, PP, and PS. Despite the high removal efficiency for MPs, PLE is a costly method. The primary cost of PLE at the beginning is equipment purchase. Solvent cost in this method is related to the type and quantity of organic solvents used during the extraction process, while energy expense arises from the electricity consumed to operate under high-temperature and high-pressure conditions.
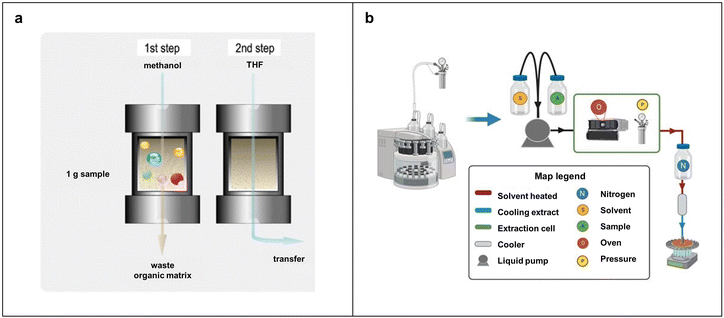 |
| Fig. 6 Pressurized liquid extraction methods for the separation of MPs. (a) Basic flow of pressurized fluid extraction method. Reproduced with permission,94 Copyright 2019, Springer-Verlag GmbH Germany, part of Springer Nature. (b) Schematic of pressurized liquid extraction equipment and the operation flow. | |
2.7 Electrostatic separation
Electrostatic separation utilizes electrically charged MPs to separate them from other impurities of samples using an electrically charged metal or plastic separator. The Hamos Electrostatic Metal/Plastic Separator, also known as a Korona–Walzen–Scheider (KWS) system manufactured by Hamos GmbH, has been studied in previous works for the electrostatic separation of MPs.97 In the usual procedure, dry samples containing MPs are fed into the KWS through a filling funnel and slowly dispersed onto a rotating metal drum via a vibrating conveyor belt, as shown in Fig. 7a. The drum and conveyor belt speeds are individually adjustable. As the drum rotates, particles enter a high-voltage corona field, which discharges materials at different speeds based on their conductivity. The particles are then divided into non-conducting sample parts (MPs and some residues) and discarded materials. Fig. 7b illustrates the functional principle of the corona roller separator, where a vibrating conveyor applies the mixture separated to a grounded roll and electrostatically charged by corona electrodes of 15
000–30
000 volts. This charge makes the particles stick to the roll surface due to mirror forces. Once particles leave the electrostatic field, they are discharged onto the surface of the grounded roller. Metal particles quickly lose their charge and fall off the roller in a parabolic path. Plastic particles, being excellent insulators, release their charge very slowly and remain on the drum until brushed off. The distinct behavior of metal and plastic particles facilitates their separation. Adjustable separation plates are used to prevent remixing of conductive and non-conductive materials post separation. Unlike other separation methods like density separation, the KWS system offers a 99% recovery rate with minimal dependence on particle size.5Fig. 7c shows a digital photograph of a typical KWS equipment. It handles large on-site samples efficiently and operates safely with corrosive liquids, enhancing its efficiency. However, KWS can only separate dry materials. The sample should be dried before separation.
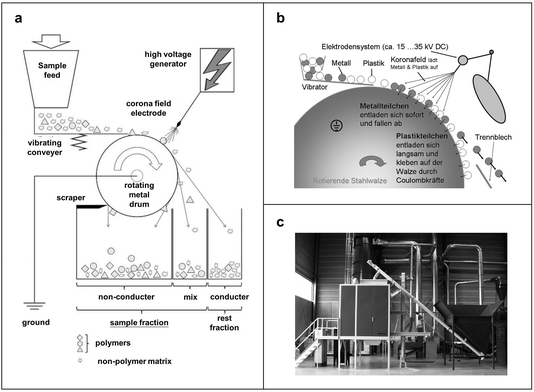 |
| Fig. 7 Schemes of the mechanism and process for separation of MPs based on electrostatic interaction. (a) Schematic of the electrostatic separator. Reproduced with permission,97 Copyright 2017, Elsevier. (b) Working mechanism of the corona roller separator type hamos KWS. Reproduced with permission,98 Copyright 2019, Springer-Verlag. (c) Physical view of a KWS electrostatic separator (hamos 1521-1). Reproduced with permission,98 Copyright 2019, Springer-Verlag. | |
3. Identification and quantification of MPs
Identification and quantification of MPs after separation can help us better understand their sources and distribution in the environment and foods, providing essential information for environmental protection, food processing and packing improvement, and health effect assessment. From the management and policy standpoint, effective monitoring and identification of MPs are also important for developing regulations and standards to control and mitigate pollution by MPs. Nevertheless, people are facing difficulties in identifying and quantifying MPs from environmental and food samples. First, the tiny sizes of MPs, ranging from millimeters and even micrometers to nanometers, make them challenging to detect and analyze using standard laboratory equipment and tools. Second, MPs occur in varying compositions and types, each requiring specific analytic methods due to their different properties. Third, environmental and food samples contain a complex mix of organic and inorganic matter, which are difficult to completely remove and may interfere with the identification and quantification of MPs. Careful handling is needed to avoid any background contamination of intrinsic MPs from air, reagents and vessels. To date, there is still no widely accepted standard for the detection and identification of MPs, leading to variability in results from different laboratories and from different studies. The following discussion will describe the state-of-the-art analytical techniques for MPs concerning identification and quantification.
3.1 Optical microscopic identification and quantification methods
Microscopic observation is the simplest and most commonly used method for quantitative detection of MPs. Researchers use various microscopes, such as the stereo microscope, fluorescence microscope, 3D confocal microscope, and atomic force microscope, to identify and quantify the number, size, shape, and color of MPs, as detailed in Table 4. Image analysis is also necessary and is performed using Image J or Motic Image Plus 2.0 to measure the size and count of microscopic particles.114,115 A stereo microscope equipped with a digital camera is a standard method for quantitatively detecting MPs in food. However, stereo microscopes cannot accurately differentiate between natural and synthetic particles. Visual identification is ineffective for particles smaller than 500 μm. Therefore, studies often combine microscopy with analytical methods like FTIR spectrometry to reduce errors. Fluorescence microscopy and 3D confocal microscopy are also used to quantify trace MPs in food.116–119
Table 4 Detailed information on microscopic methods for analysis of MPs
Microscopic method |
Mechanism and operation |
Advantage |
Disadvantage |
Applied sample |
Ref. |
Stereoscopic microscope |
Direct observation of MPs under the microscope based on the characteristics of MPs |
Easy operation, visual representation of size, shape, and color |
Interference by impurities, subjectivity depending on observers, and unavailability for <500 μm MPs |
Fish, shellfish, chicken, seaweed nori, and marine fish |
137 and 138 |
Fluorescence microscope |
Staining of MPs with a hydrophobic dye and observation under the microscope |
Direct measurement of particle number and size of MPs |
Unavailability for <50 μm MPs |
Bottled water and milk |
116 and 117 |
3D confocal microscope |
Staining of MPs with a hydrophobic dye, and observation and tracking under the microscope |
Spatial observation, applicability to in vivo transport, and cellular uptake of MPs |
Complicated operation and processing, and high-cost |
Wheat and lettuce seeds |
119
|
Atomic force microscope |
Probing and scanning of MPs on a flat surface or a plastic film under the microscope |
Precise morphological observation |
Complex operation, unavailability of size and color analysis |
Pure MPs and plastic film |
118 and 139 |
3.2 Chemical identification and quantification methods
Detailed analysis of the molecular structure of microplastic polymers allows for precise identification of the chemical composition of MPs. Currently, there are several chemical identification and quantification techniques, as shown in Table 5. First, spectroscopic techniques, such as infrared spectroscopy and Raman spectroscopy, are commonly used due to their fingerprinting characteristics and good applicability in many contexts.120 Second, the combined use of thermal analysis and chromatographic techniques, such as thermal extraction-thermal desorption GC-MS (TEDE-GC-MS), represents another powerful platform for precise analysis of MPs.121 Furthermore, the combination of microscopy and spectroscopy, such as scanning electron microscopy coupled with energy dispersive X-ray spectroscopy (SEM-EDS), can usually provide highly resolved morphological information for MPs.122
Table 5 Detailed information on the identification and quantification methods for analysis of MPs in complex matrices
Method |
Category |
Principle |
Advantage |
Disadvantage |
Applied sample |
Ref. |
FTIR |
FTIR |
Measurement of MPs' absorption or transmission of infrared radiation to provide vibrational bands and chemical information |
High sensitivity, reliability and no destructive effect to samples |
Difficulty in operation; unavailability for small (>100 μm) MPs |
Edible shellfish, hot water |
106
|
ATR-FTIR |
Attenuated total reflection for complete interaction between the incident infrared radiation and MPs |
Simple and rapid analysis, available for analyzing irregularly shaped MPs |
Rigorous pretreatment; complicated instrument operation |
Chicken, marine fish, cuttlefish, squid, and bottled water |
107 and 108 |
Micro-FTIR |
Combination of microscopic observation with Fourier infrared spectroscopy |
Applicability to MPs analysis in many situations |
No available for analyzing MPs smaller than 5 μm; complicated instrument operation |
Oysters, wild fish from urban estuaries, seaweed, and table salt |
108
|
Raman spectroscopy |
Micro-Raman |
Raman scattering from MPs by laser irradiation that provides vibrational information on functional groups |
Reliability in chemical composition analysis, easy to operate, and possible to analyze small MPs (<1 μm) |
Possible interference by fluorescence background and time-consuming |
Dried fish, canned sardines, city drinking water, sea salt, honey |
109
|
SERS |
Enhancement of Raman signal by sample adsorption onto rough plasmonic metal surface |
High sensitivity |
Cumbersome pretreatment process |
Pure water and seawater |
110
|
Raman tweezers |
Raman spectroscopy coupled with an optical tweezer for detecting individual MPs captured in an optical trap |
Precise analysis of small MPs (50 nm−20 μm), fast, and high-precision trace analysis |
Low popularity of the instrument |
Crab and fish |
111
|
Thermal analysis |
Pyr-GC-MS |
Pyrolysis of MPs in a thermal cracking oven for GC-MS analysis |
High recovery rate for airborne MPs |
No morphological information |
Fish, lake water, soil, air and oyster |
107
|
TED-GC-MS |
Indirect determination of MPs by GC-MS via plastic thermal cracking gas captured by solid phase adsorption |
Direct identification and quantification of MPs in environmental samples without pretreatment |
No morphology information |
Sewage, ferment residue, river water |
112
|
TGA-DSC |
Qualitative and quantitative analysis based on cracking and weight loss curves of plastics at different temperatures |
Easy operation, suitable for analyzing PP and PE MPs |
Possible errors for analysis of complex polymers |
Lake water |
113
|
Electron microscopy |
SEM coupled with EDS |
Qualitative and quantitative analysis of composition based on SEM imaging and X-ray scattering information |
High magnification and high resolution |
Difficulty in analyzing small MPs |
Fish |
110
|
3.2.1 Fourier transform infrared (FTIR) spectroscopy.
FTIR spectroscopy is developed from the principle of Fourier transform of interfered infrared light. FTIR spectroscopy is commonly used in MP characterization studies due to its high sensitivity and efficiency. FTIR spectroscopy identified MPs by comparing the infrared spectra of samples with a known reference polymer spectrum library, offering accurate analysis of small-sized MPs. FTIR spectroscopy can detect particles larger than 100 μm, while micro-FTIR, which combines FTIR spectroscopy with microscopy, reduces the detection limit to 5 μm, making it ideal for MP characterization.106 Additionally, the attenuated total reflection (ATR) technique applied to FTIR facilitates quicker and easier analysis, particularly for irregularly shaped MPs.107 Liu et al. developed a method to identify MPs in Chinese coastal mussels by combining thermogravimetric analysis (TGA), FTIR, and GC/MS, improving the capability of discriminating MPs.123 Recycling rates could be up to 97% without degrading MPs. However, the high cost of Fourier spectroscopy-related instruments and extensive operation training hinder the widespread detection of MPs using FTIR.
3.2.2 Raman spectroscopy.
Raman spectroscopy, which is based on the inelastic scattering of incident radiation through its interaction with vibrating molecules, has demonstrated the potential for detection of MPs smaller than 50 μm. Although it is complicated in principle, the availability of automated equipment and dedicated algorithms makes Raman spectroscopy a user-friendly and convenient method for analysis of MPs. In general, substances in samples are characterized by matching over 70% of their characteristic peaks with the reference database.124 Currently, Raman spectroscopy is exploited in different formats for MP analysis, including micro-Raman spectroscopy, surface-enhanced Raman spectroscopy (SERS), and Raman tweezers (RTs). Micro-Raman spectroscopy, which combines Raman spectroscopy with a microscope, is ideal for qualitative detection of MPs in food down to 1 μm, which is complementary to micro-FTIR spectroscopy (>50 μm).110 The integration of Raman and infrared spectroscopy as a new trend in MP detection enables the detection of plastic particles as small as 3 μm.125 SERS and RTs have also been employed for the qualitative analysis of MPs. SERS can detect MPs by adsorbing MPs onto corrugated plasmonic metal surfaces (e.g., gold or silver nanoparticles), which boosts the Raman signal of MPs and allows for detecting small-sized (∼450 nm) MPs at low concentration levels. Regarding RTs, they can perform both optical trapping and chemical identification, allowing for the detection of MPs at the single-particle level, with a diameter down to the 50 nm range.111 Based on the above comparison, it is suggested that micro-Raman featured with portability is suitable for convenient and field analysis of MPs; despite the need for an enhancement substrate and relatively complicated instrumentation compared with micro-Raman, SERS usually possesses much higher detection sensitivity. Although RTs can analyze small single MPs, suitable extraction and concentration protocols are required for their practical applications. In addition, the presence of microorganisms, organic or inorganic substances on MPs may produce interfering signals that affect the Raman spectra. Therefore, careful sample collection is essential to minimize background signals for Raman spectroscopic analysis of MPs.
3.2.3 Quantification based on thermal treatment.
Thermal treatment of MPs exploits the thermal characteristics of polymers and facilitates the analysis of their physical and chemical properties to identify their components. Primary thermal analysis techniques include pyrolysis GC-MS (Pyr-GC-MS), thermal extraction-thermal desorption GC-MS (TED-GC-MS), and thermogravimetric analysis coupled with differential scanning calorimetry (TGA-DSC). Pyr-GC-MS involves sending samples containing MPs to a pyrolysis chamber, analyzing their composition using GC, and simultaneously analyzing polymers and additives, which can avoid mechanical pre-selection and background contamination.126 Compared to FTIR and Raman spectroscopy, Pyr-GC-MS can achieve a higher recovery rate (97–110%) and excellent sensitivity for detecting airborne MPs, with fewer errors in observing and detecting small-sized MPs.127 For TED-GC-MS, the sample is placed in a horizontal thermal balance for thermogravimetric analysis, with pyrolysis gases adsorbed by a polydimethyl siloxane twister as a solid phase sorbent for the subsequent GC-MS analysis.128,129 TED-GC-MS offers a quick and straightforward way to detect and quantify MPs, and can directly identify and quantify polymers in environmental samples without the need for pre-treatment. TGA-DSC identifies chemicals by measuring the melting temperature of samples. TGA-DSC provides both qualitative and quantitative information on the gaseous products of MPs,28 requiring minimal sample preparation and being the most economical among the three methods. The accuracy of TGA-DSC is influenced by factors like additives, impurities, and polymerization chain segments, which can interfere with the identification of complex polymers. Therefore, TGA-DSC is not suitable for analysis of samples containing high contents of organic matter.113 Note that size and morphological information of MPs is not available from all the methods based on thermal treatment due to the thermal disruption in the analysis procedures.
3.3 Integrated techniques
Due to the difficulty in precise and detailed analysis of MPs, recently, some techniques with integrated functions have also been applied to analysis MPs. For instance, electron microscopy is of high resolution and accuracy in the morphological analysis of small particles, while the chemical information of particles is lost. One can use SEM integrated with EDS for chemical information acquisition. The details are discussed below.
3.3.1 Scanning electron microscopy and energy dispersive spectroscopy (SEM-EDS).
SEM-EDS reveals the morphology of MPs, like surface fractures and cracks, and provides detailed information on their elemental composition and inorganic additives. The heterogeneity of samples, which often combine organic and inorganic substances with MPs, results in detected emission spectra that can differ significantly from those in the reference libraries, a notable limitation of FTIR. As for SEM/EDS, it is not affected by this limitation. SEM-EDS provides highly magnified, high-resolution images that minimize the likelihood of misclassifying tiny organics as MPs, a significant improvement over optical microscopy.130 Wang et al. employed two types of microscopes for MP analysis: a JSM-6480LV scanning electron microscope with a Sirius SD energy dispersive X-ray spectrometer for initial morphological assessment and detailed microanalysis and a Sigma 300 VP field emission scanning electron microscope for high-resolution imaging.122 High-resolution images of the particle surfaces of small plastic fibers and fragments and their elemental composition characteristics were attained based on these techniques. EDS perfectly reflect the wide variety of MPs currently found in the natural environment. In addition to the observed peaks for carbon and oxygen, some metal elements, such as Ti, Ba, and Zn, were also detected, and these are considered additives in some plastics. Indeed, TiO2 is widely used as a colorant and filler to enhance the whiteness, gloss, and color stability of plastics, while also improving hardness, durability and mechanical properties, which plays an essential role in the plastics industry.131 Embedding tetrapod-shaped zinc oxide whiskers and barium titanate nanoparticles into a PP matrix can also create a ternary nanocomposite material with enhanced dielectric properties.132 Some fragments and fibers containing nitrogen were identified as non-plastics (e.g., natural fibers, mollusc shell fragments, and plant fragments), since nitrogen is one of the main compositions in biological compounds.133
3.3.2 Other techniques.
Wang et al. introduced a new technique termed artificial intelligence-assisted nano digital inline holographic microscopy (AI-Assisted Nano-DIHM) for in situ detection of MPs and NPs in aquatic systems.134 The technique generated an interference hologram by interacting with a reference wave and the sample scattering. Equipped with thousands of raw holograms of MPs and non-plastic particles found in rivers or lakes, it allowed for automated particle characterization and classification in milliseconds using a deep neural network with nano-DIHM without sample pretreatment. Zhang et al. proposed an approach that combines SERS and stimulated Raman scattering (SRS) for qualitative and quantitative analysis of MPs and NPs in salt samples with different origins.135 After filtering a salt solution and undergoing a digestion process, the enriched MPs were subjected to SERS using Au-loaded anodized aluminum oxide substrates, while SRS was used to image and quantify MPs. Using this method, it is estimated that a person may ingest up to an average of 6 million MPs per year. Qian et al. developed a hyperspectral SRS imaging platform incorporating an automated plastic identification algorithm to analyze MPs and NPs with high chemical specificity and throughput.136 The proposed method enabled the quantification of NPs through particle counting and estimation, including NPs smaller than 100 nm on a single particle level. The results revealed that the total concentration of MPs and NPs in bottled water was around 2.4 × 105 per liter, with NPs accounting for 90%.
4. Quality assurance and control for MPs
Quality assurance and control for MPs primarily revolve around standardized procedures for sample preparation, detection, and identification to minimize the contamination of foreign MPs and enhance the precision and accuracy of qualitative and quantitative analysis. In practice, we can control several entry points that may influence the interpretation of sample data, such as MPs the experiment itself carried, residues from experimental equipment, and airborne plastic fibers.
The presence of foreign MPs in the laboratory environment should be minimized to ensure the sample separation of MPs. For example, all extraction cells and collection bottles should be pre-cleaned with HPLC-grade acetone and heated for approximately 1 h. All glassware should be cleaned with HPLC-grade ethanol using a dead-end process before and after oil extraction. Sieves used in preparation should be cleaned with an air gun to remove any remaining MPs and prevent contamination of subsequent samples. Additionally, a complete program blank should be run alongside each sample series to identify and quantify any secondary contamination. During experiments, lab coats without plastic materials like PVC and PE and nitrile gloves are used to prevent cross-contamination when handling samples and glassware.103
Regarding the detection and identification of MPs, air pollution should be treated seriously owing to the presence of plastic microfibers in air.140 A combination of an air shower room and an ultra-clean stainless steel room is highly recommended to prevent the contamination of plastic microfibers. Unlike a fume hood, this combination effectively filters fine fibers in addition to replacing the air, making it particularly suitable for experiments that are sensitive to air factors.141,142 Verifying contamination control can be done by exposing a blank glass dish to air post operation and subsequently checking for the presence of MPs on its surface.
5. Conclusion and outlook
In conclusion, this review offers a reference for the reliable and representative analysis of MPs in typical matrices by reviewing state-of-the-art methods of sample separation, identification and quantification (Fig. 8). It discusses the advantages, disadvantages, and application scenarios of detection and identification methods, with particular attention to their size limitation and detection sensitivity. A clear trend in the field is also demonstrated towards quantitative analysis of MPs by integrating various techniques. Still, the analysis of MPs is complicated by their varied environmental interactions that interfere with their detection and identification, posing challenges for applying a standardized operation protocol across different studies.
 |
| Fig. 8 Summary of the established methods for separation, identification, and quantification of MPs. | |
Direct in situ detection of MP-containing samples is an ideal way to conduct rapid analysis. However, it is a great challenge to realize high sensitivity and accuracy due to the complexity and low MPs concentration in complex matrices. In most cases, the interference signal from the sample background is much stronger than the characteristic signal of plastics (for instance, IR and Raman). In this regard, signal enhancement technology is critical for the development of novel in situ analysis strategies. Although the indirect ex situ strategy has a relatively complex sample processing and associated costs, it is now considered as an easily realizable strategy because of its high data reliability.
The as-presented ex situ analysis strategy contains an individual separation procedure where redox or alkali/acid digestion treatment is employed to eliminate the interference of most organic compounds from chemically inert plastics, i.e. PP, PS, and PE. However, strong redox, alkaline, and/or acidic conditions may not be suitable for the separation of some degradable plastics, such as polylactic acid, polyvinyl alcohol, and polyethylene glycol. Similarly, other separation methods, such as density flotation and oil leaching, are commonly available to purify and separate specific MPs. When the sample contains unknown and/or various kinds of MPs, it is difficult to accurately separate and quantitatively analyze all types of MPs. To this regard, it is necessary to develop non-targeted analysis methods. However, the environmental and health impacts may vary significantly depending on the unique structures and properties of MPs, such as stability, degradation rate, conditions, released monomers, or additives. Therefore, it is of significance to establish standard and universal separation, identification, and quantification methods for a certain size range, especially for each type of plastic, like PET or PS, contributing to precise identification of the hazards from the environment. Realization of this goal requires development of targeted methods that leverage specific properties such as density, hydrophilic and lipophilic characteristics, or specific functional groups like ester group and benzene ring.
Although people have understood the widespread presence and serious harmfulness of MPs in the environment and food, the detection and identification of MPs still require time-consuming and labor-intensive sample processing. State-of-the-art detection and identification methods typically can only identify plastic particles at the micrometer scale. Also, they have poor specificity in polymer identification. Efforts are badly needed to enhance precise identification and quantification of MPs through the following aspects.
(1) Detection and polymer-specific identification methods for MPs are in high demand. Although GC-MS is a powerful method for identifying functional chemical groups, it may not be suited for size determination and morphological characterization. Raman microscopy can help observe both the morphology and spectral information of MPs, while observation and spectral detection of smaller sized MPs may still be challenging with sample pretreatment and purification.
(2) A globally recognized standardized protocol for MPs in typical matrices such as the environment and foods should be established. Each protocol for a given sample may encompass sample pretreatment, detection procedure, and equipment required for chemical identification.
(3) Data analysis in conjunction with artificial intelligence (AI) is highly recommended for performing MP analysis tasks. Starting by gathering a large image dataset of MPs with different shapes, sizes and types, for instance, a deep learning model can be trained to detect and classify MPs in microscopic images. AI algorithms for autonomous microscope scanning further prioritize regions with a higher probability of containing MPs. Furthermore, machine learning algorithms trained on FTIR and Raman spectra may achieve accurate identification of MP chemical composition and types.
(4) Automation, miniaturization and optimization of the analytical workflow based on AI are important for the cost reduction of MP analysis. Smartphone-based microscopy may be sufficient for preliminary screening, provided that a pre-processing algorithm is developed for denoising and contrast enhancement of low-resolution microscopic images. Similarly, AI-integrated portable spectrometers, including FTIR and Raman systems, should be less expensive and easier to use than the traditional lab-scale counterparts. It is also suggested that the development and improvement of high-throughput analytical methods based on AI.
Data availability
No primary research results, software or code has been included and no new data were generated or analysed as part of this review.
Conflicts of interest
There are no conflicts of interest to declare.
Acknowledgements
We thanks Dr Jianxin Fu, Ms Yinan Li and Mr Qingsong Zhang from Ocean University of China for helpful discussion. This work is supported by the National Natural Science Foundation of China (No. 32373172), a Special Fund for the Qingdao Science and Technology Program of Public Wellbeing (22-3-7-cspz-4-nsh), and a Shenzhen Science and Technology Program (JCYJ20210324094000001).
References
- A. L. Andrady and M. A. Neal, Philos. Trans. R. Soc., B, 2009, 364, 1977–1984 CrossRef CAS PubMed.
- T. Wang, M. Hosseinzadeh, A. Cuccagna, R. Alakenova, P. Casademunt, R. A. Reyes, A. Lopez-Rubio and C. Porte, J. Hazard. Mater., 2023, 459, 132123 CrossRef CAS PubMed.
- N. Singh and T. R. Walker, npj Mater. Sustainability, 2024, 2, 17 CrossRef PubMed.
- S. Kubowicz and A. M. Booth, Environ. Sci. Technol., 2017, 51, 12058–12060 CrossRef CAS PubMed.
- S. S. R. Köhnlechner, BHM Berg-Hüttenmännische Monatsh., 2009, 154, 136–139 CrossRef.
- X. Shi, X. Zhang, W. Gao, Y. Zhang and D. He, Sci. Total Environ., 2022, 802, 149838 CrossRef CAS PubMed.
- Q. Zhang, E. G. Xu, J. Li, Q. Chen, L. Ma, E. Y. Zeng and H. Shi, Environ. Sci. Technol., 2020, 54, 3740–3751 CrossRef CAS PubMed.
- M. Bergmann, F. Collard, J. Fabres, G. W. Gabrielsen, J. F. Provencher, C. M. Rochman, E. van Sebille and M. B. Tekman, Nat. Rev. Earth Environ., 2022, 3, 323–337 CrossRef CAS.
- D. Zhang, X. Liu, W. Huang, J. Li, C. Wang, D. Zhang and C. Zhang, Environ. Pollut., 2020, 259, 113948 CrossRef CAS PubMed.
- B. Li, L. Su, H. Zhang, H. Deng, Q. Chen and H. Shi, Sci. Total Environ., 2020, 727, 138662 CrossRef CAS PubMed.
- M. I. Muhib, M. K. Uddin, M. M. Rahman and G. Malafaia, Sci. Total Environ., 2023, 865, 161274 CrossRef CAS PubMed.
- Y. Li, L. Peng, J. Fu, X. Dai and G. Wang, Analyst, 2022, 147, 1099–1105 RSC.
- R. Shi, W. Liu, Y. Lian, A. Zeb and Q. Wang, Sci. Total Environ., 2023, 859, 160025 CrossRef CAS PubMed.
- A. Ragusa, A. Svelato, C. Santacroce, P. Catalano, V. Notarstefano, O. Carnevali, F. Papa, M. Rongioletti, F. Baiocco, S. Draghi, E. D'Amore, D. Rinaldo, M. Matta and E. Giorgini, Environ. Int., 2021, 146, 106274 CrossRef CAS PubMed.
- H. A. Leslie, M. van Velzen, S. H. Brandsma, A. D. Vethaak, J. J. Garcia-Vallejo and M. H. Lamoree, Environ. Int., 2022, 163, 107199 CrossRef CAS PubMed.
- L. Yu, J. Zhang, Y. Liu, L. Chen, S. Tao and W. Liu, Sci. Total Environ., 2021, 756, 143860 CrossRef CAS PubMed.
- H. Shi, J. Frias, A. El-Din, H. Sayed, G. E. De-la-Torre, M. Jong, S. A. Uddin, R. Rajaram, S. Chavanich, A. Najii, M. D. Fernández-Severini, Y. S. Ibrahim and L. Su, Trac. Trends Anal. Chem., 2023, 168, 117308 CrossRef CAS.
- W. Zhang, S. Zhang, J. Wang, Y. Wang, J. Mu, P. Wang, X. Lin and D. Ma, Environ. Pollut., 2017, 231, 541–548 CrossRef CAS PubMed.
- L. Hu, M. Chernick, D. E. Hinton and H. Shi, Environ. Sci. Technol., 2018, 52, 8885–8893 CrossRef CAS PubMed.
- K. Liu, X. Wang, T. Fang, P. Xu, L. Zhu and D. Li, Sci. Total Environ., 2019, 675, 462–471 CrossRef CAS PubMed.
- C. A. Peters, P. A. Thomas, K. B. Rieper and S. P. Bratton, Mar. Pollut. Bull., 2017, 124, 82–88 CrossRef CAS PubMed.
- A. R. Dent, D. Chadwick, L. Eagle, A. N. Gould, M. Harwood, C. D. Sayer and N. L. Rose, Evol. Ecol., 2023, 13, e10041 CrossRef PubMed.
- V. M. Do, T. T. Dang, X. T. T. Le, D. T. Nguyen, T. V. Phung, D. N. Vu and H. V. Pham, Mar. Pollut. Bull., 2022, 180, 113800 CrossRef CAS PubMed.
- Q. Zhang, L. Liu, Y. Jiang, Y. Zhang, Y. Fan, W. Rao and X. Qian, Environ. Pollut., 2023, 323, 121225 CrossRef CAS PubMed.
- Q. Liu, Z. Chen, Y. Chen, F. Yang, W. Yao and Y. Xie, Food Chem., 2022, 397, 133771 CrossRef CAS PubMed.
- G. Kutralam-Muniasamy, F. Perez-Guevara, I. Elizalde-Martinez and V. C. Shruti, Sci. Total Environ., 2020, 714, 136823 CrossRef CAS PubMed.
- J. C. Prata, A. Paco, V. Reis, C. J. Da, A. Fernandes, C. F. Da, A. C. Duarte and T. Rocha-Santos, Food Chem., 2020, 331, 127323 CrossRef CAS PubMed.
- S. Abbasi, N. Razeghi, M. R. Yousefi, B. Podkoscielna and P. Oleszczuk, Environ. Sci. Pollut. Res., 2023, 30, 67008–67018 CrossRef CAS PubMed.
- J. Fu, Y. Li, L. Peng, W. Gao and G. Wang, Colloids Surf., A, 2022, 648, 129337 CrossRef CAS.
- Y. Cao, M. Zhao, X. Ma, Y. Song, S. Zuo, H. Li and W. Deng, Sci. Total Environ., 2021, 788, 147620 CrossRef CAS PubMed.
- X. Chang, Y. Fang, Y. Wang, F. Wang, L. Shang and R. Zhong, Sci. Total Environ., 2022, 850, 157857 CrossRef CAS PubMed.
- A. Ahmed, M. M. Billah, M. M. Ali, M. Bhuiyan, L. Guo, M. Mohinuzzaman, M. B. Hossain, M. S. Rahman, M. S. Islam, M. Yan and W. Cai, Sci. Total Environ., 2023, 876, 162414 CrossRef CAS PubMed.
- Y. Zhai, J. Bai, P. Chang, Z. Liu, Y. Wang, G. Liu, B. Cui, W. Peijnenburg and M. G. Vijver, Trac. Trends Anal. Chem., 2024, 174, 117667 CrossRef CAS.
- F. Wang, H. Wu, W. Wu, L. Wang, J. Liu, L. An and Q. Xu, Sci. Total Environ., 2021, 789, 148027 CrossRef CAS PubMed.
- A. A. Mamun, T. Prasetya, I. R. Dewi and M. Ahmad, Sci. Total Environ., 2023, 858, 159834 CrossRef CAS PubMed.
- A. Markic, C. Niemand, J. H. Bridson, N. Mazouni-Gaertner, J. C. Gaertner, M. Eriksen and M. Bowen, Mar. Pollut. Bull., 2018, 136, 547–564 CrossRef CAS PubMed.
- K. Xiang, Z. He, J. Fu, G. Wang, H. Li, Y. Zhang, S. Zhang and L. Chen, J. Hazard. Mater., 2022, 438, 129454 CrossRef CAS PubMed.
- M. F. Hughes, H. M. Clapper, R. M. Burgess and K. T. Ho, Curr. Opin. Toxicol., 2021, 28, 43–48 CrossRef CAS PubMed.
- A. I. Catarino, V. Macchia, W. G. Sanderson, R. C. Thompson and T. B. Henry, Environ. Pollut., 2018, 237, 675–684 CrossRef CAS PubMed.
- K. D. Cox, G. A. Covernton, H. L. Davies, J. F. Dower, F. Juanes and S. E. Dudas, Environ. Sci. Technol., 2019, 53, 7068–7074 CrossRef CAS PubMed.
- Y. Li, L. Chen, N. Zhou, Y. Chen, Z. Ling and P. Xiang, Sci. Total Environ., 2024, 946, 174215 CrossRef CAS PubMed.
- A. Gonzalez-Acedo, E. Garcia-Recio, R. Illescas-Montes, J. Ramos-Torrecillas, L. Melguizo-Rodriguez and V. J. Costela-Ruiz, Chemosphere, 2021, 280, 130826 CrossRef CAS PubMed.
- J. Fu, L. Zhang, K. Xiang, Y. Zhang, G. Wang and L. Chen, Biomater. Sci., 2023, 11, 4298–4307 RSC.
- F. Mushtak, J. Prakash and S. S. Katoch, Micro Nano Eng., 2024, 22, 100237 CrossRef CAS.
- X. Chen, X. Yu, L. Zhang, W. Zhao and Q. Sui, J. Hazard. Mater., 2024, 465, 133225 CrossRef CAS PubMed.
- N. Rafa, B. Ahmed, F. Zohora, J. Bakya, S. Ahmed, S. F. Ahmed, M. Mofijur, A. A. Chowdhury and F. Almomani, Environ. Pollut., 2024, 343, 123190 CrossRef CAS PubMed.
- X. Qiu, Z. Qi, Z. Ouyang, P. Liu and X. Guo, Gondwana Res., 2022, 108, 102–119 CrossRef CAS.
- P. Pfohl, M. Wagner, L. Meyer, P. Domercq, A. Praetorius, T. Huffer, T. Hofmann and W. Wohlleben, Environ. Sci. Technol., 2022, 56, 11323–11334 CrossRef CAS PubMed.
- D. Tatsii, S. Bucci, T. Bhowmick, J. Guettler, L. Bakels, G. Bagheri and A. Stohl, Environ. Sci. Technol., 2024, 58, 671–682 CrossRef CAS PubMed.
- D. He, X. Zhang and J. Hu, J. Hazard. Mater., 2021, 409, 124640 CrossRef CAS PubMed.
- Y. Meng, F. J. Kelly and S. L. Wright, Environ. Pollut., 2020, 256, 113445 CrossRef CAS PubMed.
- J. La Nasa, T. Lomonaco, E. Manco, A. Ceccarini, R. Fuoco, A. Corti, F. Modugno, V. Castelvetro and I. Degano, Chemosphere, 2021, 270, 128612 CrossRef CAS PubMed.
- A. Tirkey and L. Upadhyay, Mar. Pollut. Bull., 2021, 170, 112604 CrossRef CAS PubMed.
- I. Nabi, A. Bacha and L. Zhang, J. Cleaner Prod., 2022, 337, 130458 CrossRef CAS.
- B. Udovicki, M. Andjelkovic, T. Cirkovic-Velickovic and A. Rajkovic, Int. J. Food Contam., 2022, 9, 1–16 CrossRef.
- A. Hasan Anik, S. Hossain, M. Alam, M. Binte Sultan, M. T. Hasnine and M. M. Rahman, Environ. Nanotechnol. Monit. Manag., 2021, 16, 100530 CAS.
- S. Zhao, M. Danley, J. E. Ward, D. Li and T. J. Mincer, Anal. Methods, 2017, 9, 1470–1478 RSC.
- R. R. Hurley, A. L. Lusher, M. Olsen and L. Nizzetto, Environ. Sci. Technol., 2018, 52, 7409–7417 CrossRef CAS PubMed.
- H. Lee, S. Kim, A. Sin, G. Kim, S. Khan, M. N. Nadagouda, E. Sahle-Demessie and C. Han, Sci. Total Environ., 2023, 871, 161718 CrossRef CAS PubMed.
- Q. Li, Z. Feng, T. Zhang, C. Ma and H. Shi, J. Hazard. Mater., 2020, 388, 122060 CrossRef CAS PubMed.
- W. Courtene-Jones, B. Quinn, F. Murphy, S. F. Gary and B. E. Narayanaswamy, Anal. Methods, 2017, 9, 1437–1445 RSC.
- K. B. Olesen, D. A. Stephansen, N. van Alst and J. Vollertsen, Water, 2019, 11, 1466 CrossRef CAS.
- K. Enders, A. Kappler, O. Biniasch, P. Feldens, N. Stollberg, X. Lange, D. Fischer, K. J. Eichhorn, F. Pollehne, S. Oberbeckmann and M. Labrenz, Sci. Rep., 2019, 9, 15207 CrossRef CAS PubMed.
- M. Scheurer and M. Bigalke, Environ. Sci. Technol., 2018, 52, 3591–3598 CrossRef CAS PubMed.
- J. Gauquie, L. Devriese, J. Robbens and B. De Witte, Chemosphere, 2015, 138, 348–356 CrossRef CAS PubMed.
- Q. Qiu, Z. Tan, J. Wang, J. Peng, M. Li and Z. Zhan, Estuarine, Coastal Shelf Sci., 2016, 176, 102–109 CrossRef CAS.
- A. Dehaut, A. L. Cassone, L. Frere, L. Hermabessiere, C. Himber, E. Rinnert, G. Riviere, C. Lambert, P. Soudant, A. Huvet, G. Duflos and I. Paul-Pont, Environ. Pollut., 2016, 215, 223–233 CrossRef CAS PubMed.
- L. I. Devriese, M. D. van der Meulen, T. Maes, K. Bekaert, I. Paul-Pont, L. Frere, J. Robbens and A. D. Vethaak, Mar. Pollut. Bull., 2015, 98, 179–187 CrossRef CAS PubMed.
- X. Li, L. Chen, Y. Ji, M. Li, B. Dong, G. Qian, J. Zhou and X. Dai, Water Res., 2020, 171, 115379 CrossRef CAS PubMed.
- M. F. Diaz-Basantes, J. A. Conesa and A. Fullana, Sustainability, 2020, 12, 5514 CrossRef.
- M. Simon, N. van Alst and J. Vollertsen, Water Res., 2018, 142, 1–9 CrossRef CAS PubMed.
- U. Sunta, P. Trebse and M. B. Kralj, Molecules, 2021, 26, 1840 CrossRef CAS PubMed.
- K. Hu, P. Zhou, Y. Yang, T. Hall, G. Nie, Y. Yao, X. Duan and S. Wang, ACS ES&T Eng., 2022, 2, 110–120 Search PubMed.
- E. Neyens and J. Baeyens, J. Hazard. Mater., 2003, 98, 33–50 CrossRef CAS PubMed.
- P. Solanki, C. Putatunda, A. Kumar, R. Bhatia and A. Walia, 3 Biotech, 2021, 11, 428 CrossRef PubMed.
- W. Lao, S. Dial, M. Salmon and C. S. Wong, Sci. Total Environ., 2024, 917, 170528 CrossRef CAS PubMed.
- A. Sridhar, D. Kannan, A. Kapoor and S. Prabhakar, Chemosphere, 2022, 286, 131653 CrossRef CAS PubMed.
- M. Kedzierski, V. Le Tilly, G. Cesar, O. Sire and S. Bruzaud, Mar. Pollut. Bull., 2017, 115, 120–129 CrossRef CAS PubMed.
- T. T. Duong, P. T. Le, T. Nguyen, T. Q. Hoang, H. M. Ngo, T. O. Doan, T. P. Q. Le, H. T. Bui, M. H. Bui, V. T. Trinh, T. L. Nguyen, L. N. Da, T. M. Vu, T. Tran, T. C. Ho, N. N. Phuong and E. Strady, Environ. Monit. Assess., 2022, 194, 65 CrossRef CAS PubMed.
- D. Debraj and M. Lavanya, Sci. Total Environ., 2023, 893, 164878 CrossRef CAS PubMed.
- L. Li, M. Li, H. Deng, L. Cai, H. Cai, B. Yan, J. Hu and H. Shi, Sci. Total Environ., 2018, 639, 367–373 CrossRef CAS PubMed.
- D. Elkhatib and V. Oyanedel-Craver, Environ. Sci. Technol., 2020, 54, 7037–7049 CrossRef CAS PubMed.
- B. Quinn, F. Murphy and C. Ewins, Anal. Methods, 2017, 9, 1491–1498 RSC.
- L. M. Hernandez, N. Yousefi and N. Tufenkji, Environ. Sci. Technol. Lett., 2017, 4, 280–285 CrossRef CAS.
- M. B. Zobkov and E. E. Esiukova, Limnol Oceanogr. Methods, 2017, 15, 967–978 CrossRef CAS.
- V. Vatanpour, M. E. Pasaoglu, H. Barzegar, O. O. Teber, R. Kaya, M. Bastug, A. Khataee and I. Koyuncu, Chemosphere, 2022, 295, 133914 CrossRef CAS PubMed.
- R. L. Coppock, M. Cole, P. K. Lindeque, A. M. Queirós and T. S. Galloway, Environ. Pollut., 2017, 230, 829–837 CrossRef CAS PubMed.
- H. Hidayaturrahman and T. Lee, Mar. Pollut. Bull., 2019, 146, 696–702 CrossRef CAS PubMed.
- J. Fu, N. Liu, Y. Peng, G. Wang, X. Wang, Q. Wang, M. Lv and L. Chen, J. Hazard. Mater., 2023, 456, 131685 CrossRef CAS PubMed.
- E. M. Crichton, M. Noël, E. A. Gies and P. S. Ross, Anal. Methods, 2017, 9, 1419–1428 RSC.
- Y. Li, J. Fu, L. Peng, X. Sun, G. Wang, Y. Wang and L. Chen, Chem. Eng. J., 2023, 469, 143992 CrossRef CAS.
- A. Kononov, M. Hishida, K. Suzuki and N. Harada, Soil Syst., 2022, 6, 54 CrossRef CAS.
- J. Grbic, B. Nguyen, E. Guo, J. B. You, D. Sinton and C. M. Rochman, Environ. Sci. Technol. Lett., 2019, 6, 68–72 CrossRef CAS.
- G. Dierkes, T. Lauschke, S. Becher, H. Schumacher, C. Foldi and T. Ternes, Anal. Bioanal. Chem., 2019, 411, 6959–6968 CrossRef CAS PubMed.
- L. Barp, A. M. Visnjevec and S. Moret, Foods, 2023, 12, 2017 CrossRef CAS PubMed.
- A. Perez-Vazquez, M. Carpena, P. Barciela, L. Cassani, J. Simal-Gandara and M. A. Prieto, Antioxidants, 2023, 12, 612 CrossRef CAS PubMed.
- S. Felsing, C. Kochleus, S. Buchinger, N. Brennholt, F. Stock and G. Reifferscheid, Environ. Pollut., 2018, 234, 20–28 CrossRef CAS PubMed.
- R. Köhnlechner and S. Sander, BHM Berg-Hüttenmännische Monatsh., 2009, 154, 136–139 CrossRef.
- R. C. Thompson, Y. Olsen, R. P. Mitchell, A. Davis, S. J. Rowland, A. W. John, D. McGonigle and A. E. Russell, Science, 2004, 304, 838 CrossRef CAS PubMed.
- M. T. Nuelle, J. H. Dekiff, D. Remy and E. Fries, Environ. Pollut., 2014, 184, 161–169 CrossRef CAS PubMed.
- L. Pittura, C. G. Avio, M. E. Giuliani, G. D’Errico, S. H. Keiter, B. Cormier, S. Gorbi and F. Regoli, Front. Mar. Sci., 2018, 5, 103 CrossRef.
- Q. Liu, Y. Chen, Z. Chen, F. Yang, Y. Xie and W. Yao, Sci. Total Environ., 2022, 851, 157991 CrossRef CAS PubMed.
- S. Lechthaler, L. Hildebrandt, G. Stauch and H. Schuttrumpf, Anal. Methods, 2020, 12, 5128–5139 RSC.
- X. Rong, X. Chen, P. Li, C. Zhao, S. Peng, H. Ma and H. Qu, Chemosphere, 2022, 299, 134493 CrossRef CAS PubMed.
- J. Kamp, G. Dierkes, P. N. Schweyen, A. Wick and T. A. Ternes, Environ. Sci. Technol., 2023, 57, 4806–4812 CrossRef CAS PubMed.
- Y. Chen, D. Wen, J. Pei, Y. Fei, D. Ouyang and H. Z. A. Y. Luo, Curr. Opin. Environ. Sci. Health, 2020, 18, 14–19 CrossRef.
- C. Shi, C. Penrose, J. E. Pitts, P. Gowda, I. J. Luxmoore and G. R. Nash, Nanoscale Adv., 2019, 1, 476–480 RSC.
- A. Dilshad, M. Taneez, F. Younas, A. Jabeen, M. T. Rafiq and H. Fatimah, Environ. Monit. Assess., 2022, 194, 511 CrossRef CAS PubMed.
- S. Gundogdu, Food Addit. Contam.: Part A, 2018, 35, 1006–1014 CrossRef CAS PubMed.
- G. Xu, H. Cheng, R. Jones, Y. Feng, K. Gong, K. Li, X. Fang, M. A. Tahir, V. K. Valev and L. Zhang, Environ. Sci. Technol., 2020, 54, 15594–15603 CrossRef CAS PubMed.
- R. Gillibert, G. Balakrishnan, Q. Deshoules, M. Tardivel, A. Magazzu, M. G. Donato, O. M. Marago, D. L. C. M. Lamy, F. Colas, F. Lagarde and P. G. Gucciardi, Environ. Sci. Technol., 2019, 53, 9003–9013 CrossRef CAS PubMed.
- J. H. Lee, M. J. Kim, C. S. Kim, S. J. Cheon, K. I. Choi, J. Kim, J. Jung, J. K. Yoon, S. H. Lee and D. H. Jeong, Environ. Pollut., 2023, 333, 122017 CrossRef CAS PubMed.
- M. Majewsky, H. Bitter, E. Eiche and H. Horn, Sci. Total Environ., 2016, 568, 507–511 CrossRef CAS PubMed.
- J. C. Prata, V. Reis, J. Matos, C. J. Da, A. C. Duarte and T. Rocha-Santos, Sci. Total Environ., 2019, 690, 1277–1283 CrossRef CAS PubMed.
- T. S. Mahadevan, M. Milosevic, M. Kojic, F. Hussain, N. Kojic, R. Serda, M. Ferrari and A. Ziemys, J. Nanopart. Res., 2013, 15, 1477 CrossRef.
- E. Hengstmann and E. K. Fischer, Environ. Monit. Assess., 2019, 191, 612 CrossRef PubMed.
- L. S. Galvao, R. R. Ferreira, E. Fernandes, C. A. Correia, T. S. Valera, S. R. D. Dos and H. Wiebeck, J. Hazard. Mater., 2023, 443, 130217 CrossRef CAS PubMed.
- F. Akhatova, I. Ishmukhametov, G. Fakhrullina and R. Fakhrullin, Int. J. Mol. Sci., 2022, 23, 806 CrossRef CAS PubMed.
- M. Jiao, S. Cao, L. Ren and R. Li, J. Hazard. Mater. Adv., 2021, 3, 100016 CrossRef CAS.
- J. S. Boke, J. Popp and C. Krafft, Sci. Rep., 2022, 12, 18785 CrossRef PubMed.
- E. Duemichen, P. Eisentraut, M. Celina and U. Braun, J. Chromatogr. A, 2019, 1592, 133–142 CrossRef CAS PubMed.
- Z. M. Wang, J. Wagner, S. Ghosal, G. Bedi and S. Wall, Sci. Total Environ., 2017, 603–604, 616–626 CrossRef CAS PubMed.
- Y. Liu, R. Li, J. Yu, F. Ni, Y. Sheng, A. Scircle, J. V. Cizdziel and Y. Zhou, Environ. Pollut., 2021, 272, 115946 CrossRef CAS PubMed.
- K. Miserli, C. Lykos, A. G. Kalampounias and I. Konstantinou, Appl. Sci., 2023, 13, 9705 CrossRef CAS.
- S. Klingler, J. Hniopek, R. Stach, M. Schmitt, J. Popp and B. Mizaikoff, ACS Meas. Sci. Au, 2022, 2, 157–166 CrossRef CAS PubMed.
- S. T. Anuar, R. S. Altarawnah, A. A. Mohd, B. Q. Lee, W. Khalik, K. Yusof and Y. S. Ibrahim, Polymers, 2022, 14, 3054 CrossRef CAS PubMed.
- P. Luo, M. Bai, Q. He, Z. Peng, L. Wang, C. Dong, Z. Qi, W. Zhang, Y. Zhang and Z. Cai, Anal. Chem., 2023, 95, 3556–3562 CrossRef CAS PubMed.
- M. H. Cho, Y. J. Song, C. J. Rhu and B. R. Go, Polymers, 2023, 15, 241 CrossRef CAS PubMed.
- D. Sorolla-Rosario, J. Llorca-Porcel, M. Perez-Martinez, D. Lozano-Castello and A. Bueno-Lopez, Talanta, 2023, 253, 123829 CrossRef CAS PubMed.
- M. Gniadek and A. Dąbrowska, Mar. Pollut. Bull., 2019, 148, 210–216 CrossRef CAS PubMed.
- T. J. Kemp and R. A. McIntyre, Prog. React. Kinet. Mech., 2000, 26, 337–374 CrossRef.
- J. Hu, L. Zhang, Z. Dang and D. Wang, Compos. Sci. Technol., 2017, 148, 20–26 CrossRef CAS.
- G. Furfaro, M. D'Elia, S. Mariano, E. Trainito, M. Solca, S. Piraino and G. Belmonte, Sci. Rep., 2022, 12, 10244 CrossRef CAS PubMed.
- Z. Wang, D. Pal, A. Pilechi and P. A. Ariya, Environ. Sci. Technol., 2024, 58, 8919–8931 CrossRef CAS PubMed.
- X. Ruan, J. Ao, M. Ma, R. R. Jones, J. Liu, K. Li, Q. Ge, G. Xu, Y. Liu, T. Wang, L. Xie, W. Wang, W. You, L. Wang, V. K. Valev, M. Ji and L. Zhang, Environ. Sci. Technol., 2024, 58, 9091–9101 CrossRef CAS PubMed.
- N. Qian, X. Gao, X. Lang, H. Deng, T. M. Bratu, Q. Chen, P. Stapleton, B. Yan and W. Min, Proc. Natl. Acad. Sci. U. S. A., 2024, 121, e1994385175 Search PubMed.
- J. Nawab, H. Khan, J. Ghani, M. I. Zafar, S. Khan, S. Toller, L. Fatima and A. Hamza, Chemosphere, 2023, 330, 138572 CrossRef CAS PubMed.
- Y. Cheng, R. Zhang, L. Tisinger, S. Cali, Z. Yu, H. Y. Chen and A. Li, Gondwana Res., 2022, 108, 22–30 CrossRef CAS.
- S. D. Burrows, S. Frustaci, K. V. Thomas and T. Galloway, Trac. Trends Anal. Chem., 2020, 130, 115993 CrossRef CAS.
- S. M. Brander, V. C. Renick, M. M. Foley, C. Steele, M. Woo, A. Lusher, S. Carr, P. Helm, C. Box, S. Cherniak, R. C. Andrews and C. M. Rochman, Appl. Spectrosc., 2020, 74, 1099–1125 CrossRef CAS PubMed.
- S. Ziajahromi and F. Leusch, Environ. Pollut., 2022, 294, 118629 CrossRef CAS PubMed.
- K. Liu, T. Wu, X. Wang, Z. Song, C. Zong, N. Wei and D. Li, Environ. Sci. Technol., 2019, 53, 10612–10619 CrossRef CAS PubMed.
Footnote |
† The authors contributed equally. |
|
This journal is © The Royal Society of Chemistry 2024 |
Click here to see how this site uses Cookies. View our privacy policy here.