DOI:
10.1039/D4AY02218A
(Paper)
Anal. Methods, 2025, Advance Article
Fluorogenic selective detection of Zn2+ using a pyrazole-ortho-vanillin conjugate: insights from DFT, molecular docking, bioimaging and anticancer applications†
Received
9th December 2024
, Accepted 9th February 2025
First published on 10th February 2025
Abstract
A fluorescent sensor, (E)-N′-(2-hydroxy-3-methoxybenzylidene)-3,5-dimethyl-1H-pyrazole-1-carbohydrazide (HMPC), was designed and synthesized for the selective fluorescence recognition of Zn2+ in semi-aqueous media. Notably, HMPC exhibited a red-shifted, two-fold fluorescence “turn-on” enhancement in response to Zn2+ at 490 nm, with a detection limit of 1.68 μM, which is significantly lower than the WHO guideline (76.0 μM). The binding constant of HMPC with Zn2+ was calculated to be 5 × 104 M−1. The fluorescence enhancement of HMPC in the presence of Zn2+ is attributed to the suppression of the PET process and the enhancement of ICT, leading to fluorescence via the CHEF mechanism. The sensing mechanism was demonstrated through UV-vis, fluorescence spectroscopy, Job plots, ESI-MS, and DFT calculations. For biological applications, cytotoxicity and cell imaging studies were performed using MCF-7 cells. Molecular docking studies revealed a high binding energy of HMPC (ΔG = −7.1 kcal mol−1) with the 4,5-diaryl isoxazole HSP90 chaperone protein, suggesting its potential as an anticancer agent. Additionally, its binding energy of −6.5 kcal mol−1 with the HDAC8 protein indicates greater efficacy than suberoylanilide hydroxamic acid (SAHA) in inhibiting HDAC, as it binds more strongly to the HDAC8 protein than SAHA (−7.4 kcal mol−1). Furthermore, due to its favorable ADME profile, HMPC may be suitable for oral administration, enhancing its potential as an anticancer drug.
1. Introduction
A chemosensor is an abiotic molecule that produces a measurable signal when interacting with matter or energy. Fluorescent chemosensors, a common type, emit a fluorescence signal upon binding with an analyte. These systems consist of two components: a fluorophore (signaling unit) and a receptor (recognition unit). Fluorescence occurs when a molecule absorbs photons from UV-visible light, transitioning to a higher energy state, and then emits photons while returning to its ground state, though alternative de-excitation pathways also exist.1 In 1867, F. Goppelsröder introduced the first fluorescent chemosensor, which was designed to detect aluminum ions (Al3+) by forming a highly fluorescent morin chelate. This pioneering work paved the way for the creation of numerous fluorescent chemosensors for detecting various metal ions over the following decades, contributing significantly to the emergence of modern analytical chemistry.2 Among the metal ions, zinc ion (Zn2+) has garnered significant attention due to its biological importance as the second most abundant transition metal in the human body after iron. Zinc influences a variety of physiological processes, including DNA synthesis,3 apoptosis,4,5 gene expression,6,7 neurotransmission,8–11 and signal transduction.12 Additionally, Zn2+ modulates several ion channels13 and serves as a crucial component in enzymes and transcription factors such as carbonic anhydrase and zinc finger proteins, where it plays both structural and catalytic roles.14 Zn2+ is vital for many biological processes, including brain function, gene transcription, immune response, and reproduction,15 and is implicated in pathological conditions such as Alzheimer's disease, epilepsy, ischemic stroke, and infantile diarrhea.16 While most Zn2+ is tightly bound to enzymes and proteins, free zinc pools exist in tissues such as the brain, intestine, pancreas, and retina. Given its biological significance, optical imaging using fluorescent sensors for Zn2+ has drawn considerable interest.17
The design and synthesis of molecular receptors for the selective detection of biologically important Zn2+ ions through fluorescence changes have gained considerable attention in recent years. This is because fluorescence signals are highly sensitive to alterations in the fluorophore's surrounding environment. Due to its 3d10 4s0 electronic configuration, Zn2+ lacks intrinsic spectroscopic or magnetic properties, making it incompatible with common analytical methods like UV-vis spectroscopy, Mössbauer spectroscopy, nuclear magnetic resonance (NMR), and electron paramagnetic resonance (EPR) spectroscopy.18 Although techniques such as atomic absorption spectroscopy offer highly accurate quantitative measurements of Zn2+, they typically destroy the sample being analyzed.19 In contrast, a fluorophore-based sensor that exhibits changes in fluorescence intensity or wavelength upon binding Zn2+ can provide not only the location of Zn2+ but also a semi-quantitative estimate in both in vivo and in vitro samples.20 The ability of Zn2+ to form complexes with various organic structures that can be linked to a fluorophore makes it possible to develop fluorescent probes for biological applications.21,22 Thus, target ligand was synthesized by normal Schiff base condensation reaction between 5-bromo-2-hydroxy-3-methoxybenzaldehyde with 3,5-dimethyl-1H-pyrazole-1-carbohydrazide to give the expected ligand (E)-N′-(2-hydroxy-3-methoxybenzylidene)-5-methyl-1H-pyrazole-3-carbohydrazide (HMPC) which has been shown in the Scheme 1. The target ligand HMPC has been characterized by 1H-NMR and mass spectra (Fig. S4–S6†).
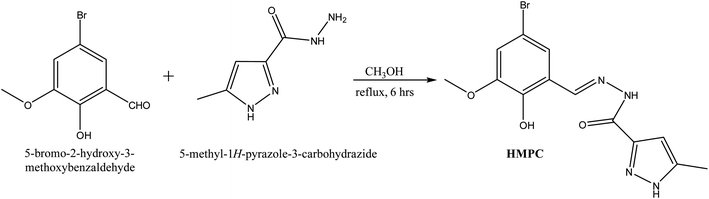 |
| Scheme 1 Synthesis of HMPC. | |
2. Experimental section
2.1 General
The chemicals and solvents were purchased from Sigma-Aldrich Chemicals Private Limited and were used without further purification. Melting points were determined on a hot-plate melting point apparatus in an open-mouth capillary and were uncorrected. 1H-NMR spectra were recorded on Brucker 400 MHz instruments. NMR titration was carried out in DMSO-D6 solvent on 400 MHz instrument with TMS as an internal standard. Chemical shifts are expressed in δ units and 1H–1H and 1H–C coupling constants in Hz. UV-vis and fluorescence titration experiments were performed on UV-spectrophotometer: PerkinElmer, Lambda 30 and Shimadzu spectrofluorophotometer RF-6000 using a fluorescence cell of 10 mm path respectively.
2.2 Synthesis of the ligand HMPC
5-Methyl-pyrazole-3-carbohydrazide was synthesized according to reported literature method.22 To the methanolic solution of 5-methyl-pyrazole-3-carbohydrazide (140 mg, 1.0 mmol), 5-bromo-2-hydroxy-3-methoxybenzaldehyde (152 mg, 1.0 mmol) was added and refluxed for 6 hours over oil bath (Scheme 1). The resulting solution is then concentrated to ∼15 mL, cooled and a pale yellow precipitated was separated out. The yellow precipitate was filtered and washed with chilled ethanol and dried over CaCl2 in a vacuum desiccator to get dry solid ligand as HMPC.
Yield: 150 mg, 64%. 1H NMR (DMSO-d6, 400 MHz): δ (ppm): 13.14 (s, 1H, –OH), 12.03 (s, 1H, –NH), 11.08 (s, 1H, –NH), 8.34 (s, 1H,
C–H), 7.29 (s, 1H), 7.15 (s, 1H), 6.52 (s, 1H) 3.84 (s, 3H), 2.30 (s, 3H). Mass (MS): M+ calculated for C13H13BrN4O3 is 352.0171; found: 354.9801 (M + 2H+).
3. Results and discussion
3.1 Binding study with Zn2+
The binding study of HMPC (c = 5 × 10−5 M) with various metal ions (c = 10−5 M) by UV-vis and fluorescence methods were carried out in an acetonitrile/aqueous (9
:
1, v/v) HEPES-buffered (1 mM, pH 7.4) solution. The UV-vis spectrum of HMPC showed a major absorption peak at 318 nm. Upon the gradual addition of Zn2+, a gradual increase in absorbance at 318 nm was observed, indicating complexation between HMPC and the Zn2+ ion (Fig. 1). However, this absorption characteristic did not result in any visible color change.
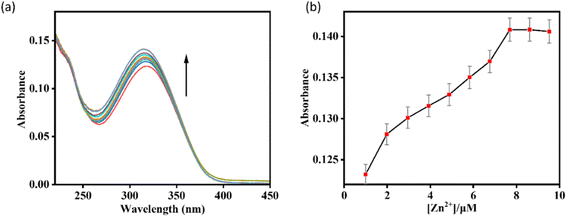 |
| Fig. 1 (a) UV-vis absorption spectra of HMPC (c = 5 × 10−5 M) in CH3CN–HEPES buffer (9 : 1, v/v, pH = 7.4) upon addition of 0 to 5 equiv. of Zn2+ (c = 10−5 M). (b) Changes of absorbance as a function of Zn2+ concentration including error bars (error amount, 1%; Y error bar for both [±] deviation). | |
In the fluorescence experiment, the receptor HMPC exhibited two emission peaks centered at 400 nm and 427 nm (λex = 318 nm). However, with the gradual addition of Zn2+ to the HMPC solution, a new red shifted emission peak generated at 490 nm with strong fluorescence enhancement by the appearance of naked eye blue color. As expected, HMPC initially exhibited weak fluorescence at 490 nm when excited at 318 nm, with a low quantum yield (Φ0 = 0.1415). Upon addition of increasing amounts of Zn2+, about a 2-fold increase in fluorescence quantum yield (Φ/Φ0 = 0.259/0.142 = 2, λem = 490 nm) was observed (Fig. 2).
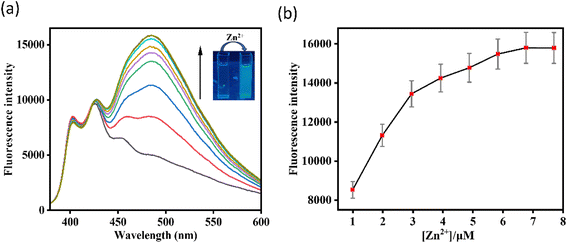 |
| Fig. 2 (a) Fluorescence spectra of HMPC (c = 5 × 10−5 M) in CH3CN–HEPES buffer (9 : 1, v/v, pH = 7.4) upon addition of 0 to 5 equiv. of Zn2+ (c = 10−5 M). Inset: changes of emission color on addition of Zn2+ ions (b) changes of emission intensity of HMPC as a function of Zn2+ concentration including error bars (error amount, 5%; Y error bar for both [±] deviation). | |
One of the challenges for Zn2+ probes is to develop systems that are selective over a wide range of potentially competing ions, such as Mn2+, Cr3+, Pb2+, Fe2+, Co2+, Ni2+, Hg2+, Cd2+, Fe3+, and Cu2+. HMPC showed almost negligible effects on its fluorescence behavior in the presence of these competing ions, thereby maintaining its selectivity toward Zn2+ by naked eye as well as in the fluorescence spectra (Fig. 3).
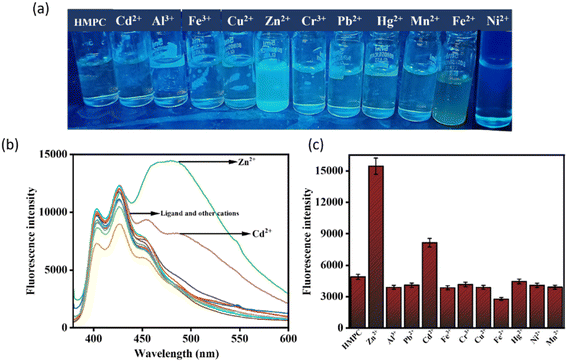 |
| Fig. 3 (a) Fluorescence color changes of HMPC in presence of various cations. (b) Fluorescence titration spectra of HMPC (c = 5 × 10−5 M) in HEPES buffered aqueous CH3CN (9 : 1, v/v) upon addition of 15 equiv. of different metal ions. (c) A comparative fluorescence response of HMPC by bar representation in presence of different cations. | |
However, Cd2+ exhibits slight interference because both ions share similar chemical and physical properties. Zn2+ and Cd2+ are in the same group (Group 12) of the periodic table and have comparable ionic radii, electronegativities, and coordination geometries resulting a slight interference in the fluorescence spectra of HMPC with Cd2+. Despite this, the sensor HMPC demonstrated higher selectivity and sensitivity toward Zn2+ compared to Cd2+.23
Competition studies were also carried out in the presence of other interfering cations to further investigate the selectivity of HMPC for Zn2+. The fluorescence enhancement of HMPC for Zn2+ was unaffected by the presence of other interfering cations. This indicates that the receptor HMPC is highly sensitive and selective for cation Zn2+ eventually in presence of other interfering analytes (Fig. 4). From the fluorescence titration experiments, the detection limit of HMPC for Zn2+ was calculated to be 1.68 μM using the formula DL = K × Sb1/S, where K = 3, Sb1 is the standard deviation of the blank solution, and S is the slope of the calibration curve, (Fig. S2†).24 Job plot analysis indicated 1
:
1 binding stoichiometry between HMPC with Zn2+ (Fig. S3†) and from the fluorescence titration experiment, the association constant (Ka) of HMPC with Zn2+ was calculated as 5 × 104 M−1 (error < 10%) (Fig. S1†).25
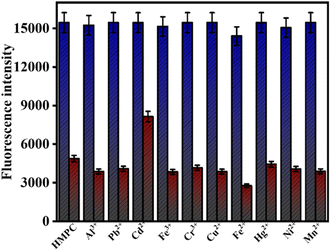 |
| Fig. 4 (Maroon bars) variation of fluorescence intensity of HMPC + 15 equiv. Mn+; (blue bars) fluorescence intensity of HMPC + 15 equiv. Mn+, followed by 10 equiv. Zn2+. | |
The reversible binding of HMPC with the metal ion Zn2+ has been proved by the reversibility experiment carried out by adding an aqueous solution of excess Na2EDTA (0–5.0 equiv.) in situ to the solution of HMPC-Zn2+ complex (Fig. 5). And it has been observed that the fluorescence intensity of HMPC-Zn2+ complex is quenched steadily in presence of excess Na2EDTA which indicates that Na2EDTA possibly strips away Zn2+ from the metal binding cavity.
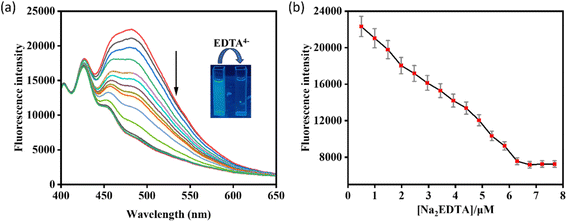 |
| Fig. 5 (a) Fluorescence titration spectra of HMPC-Zn2+ complex (c = 5 × 10−5 M) with Na2EDTA (0–5.0 equiv.) in CH3CN–HEPES buffer (9 : 1, v/v, pH = 7.4). Inset: bluish white colored emission observed with Zn2+ was disappeared in presence of Na2EDTA. (b) Change of emission intensity as a function of Na2EDTA concentration including error bars (error amount, 5%; Y error bar for both [±] deviation. | |
3.2 Probable binding mode in solution phase
Probable binding of HMPC with Zn2+ was demonstrated by UV-vis, fluorescence and mass spectrometry. Initially HMPC alone showed weak fluorescence exhibiting two emission signals at 400 nm and 427 nm but in the presence of Zn2+, a notable red shifted fluorescence enhancement was observed at 490 nm. This remarkable fluorescence enhancement of HMPC in presence of Zn2+ can be attributed due to the suppression of PET process within the molecule and enhancement of ICT for the charge transfer between ligand and metal, which results also generation of the fluorescence enhancement by chelation enhanced fluorescence (CHEF) mechanism. Before binding with Zn2+ (off-state), the fluorescence of free HMPC is very weak due to operating PET from electron donor phenolic hydroxy group to electron acceptor hydrazide coupled imine moiety and weak ICT (Scheme 2). In the presence of Zn2+, the ligand HMPC forms four coordination via the bond formation between O2 donors from hydroxy and hydrazide moieties and one nitrogen from imine nitrogen and one negative chloride counter anion resulting in fluorescence enhancement (on-state) by CHEF.26 On the other hand, the emission intensity of HMPC is notably very weak due to the radiationless deactivation of the excited state caused by conformational changes via the free rotation around the imine (C
N) bond, a phenomenon commonly observed in Schiff-base dyes. But, co-ordination with Zn2+ inhibits the isomerization which results the increase of fluorescence intensity of the ligand for enhanced ICT.27 The formation of a complex between HMPC and Zn2+ has been verified through the emergence of a mass peak at m/z = 450.6280, attributed to [HMPC + Zn2+ + Cl−] (Fig. S6† and Scheme 2), which has been justified also by 1
:
1 binding stoichiometric ratio in the Jobs plot analysis and theoretical calculation.
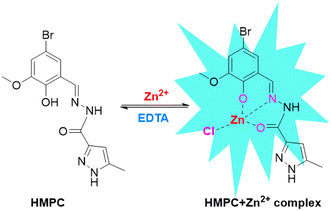 |
| Scheme 2 Probable binding mode in solution phase. | |
3.3 DFT study
The optimized geometry of HMPC and HMPC + Zn2+ complex through DFT is shown in Fig. 6. The HMPC + Zn2+ complex having metal centre Zn2+ are tetra-coordinated with neutral ligand with O2 donor system, one nitrogen and one negative chlorides counter anion, satisfy the tetrahedral geometry to form the mononuclear complex. The theoretical Zn–Cl and Zn–N (of imine) bond distances are as 2.175 Å and 2.003 Å respectively and Zn–O bond lengths are 2.199 Å (amide oxygen) and 1.947 Å (phenolic oxygen) for HMPC + Zn2+ complex. Moreover, it is found that the HOMO–LUMO energy gap are ΔE = 7.652 eV and 6.984 eV for HMPC and HMPC + Zn2+ complex respectively which is responsible for the stabilization of the complex formation (Fig. 7).
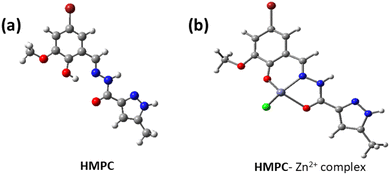 |
| Fig. 6 Geometry optimized molecular structure of (a) HMPC and (b) HMPC-Zn2+ complex. | |
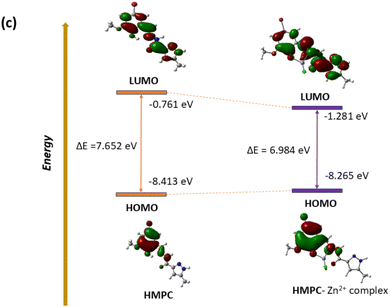 |
| Fig. 7 Frontier molecular orbital with energy difference of HMPC and complex. | |
Experimental absorbance of ligand HMPC at 328 nm at room temperature was increased by the gradual addition of Zn2+. The theoretical electronic spectra shows the corresponding calculated absorption band at 317 nm, demonstrating excellent agreement with the experimental data and these spectral bands can be attributed to the S0 → S7 transition.
3.4 Biological application
MCF-7 cells at sub-confluent volume were cultured in DMEM for 24 hours under standard conditions in a CO2 incubator. Following this, HMPC was administered to the cells at its determined IC50 concentration. After an appropriate incubation period, fluorescence images of the treated MCF-7 cells were captured at 40× magnification using a ZEISS fluorescence microscope (Germany). In parallel, the cells were also exposed to a 10 μM Zn2+ solution for one hour, and additional fluorescence images were captured at excitation wavelengths of 582 nm and 581 nm for the cells pre-treated with HMPC at the IC50 concentration, as described by Mabhai et al. (2019).28
3.4.1 Cell viability study on MCF 7 and human lymphocyte cells (HLCs). The cytotoxic effects of HMPC on MCF-7 and HCT-116 cells were assessed using the MTT assay, which revealed a significant dose-dependent reduction in cell viability, indicating potent cytotoxicity. The IC50 value of HMPC was found to be 19.26 μg mL−1 for MCF-7 cells and 21.31% μg mL−1 for HCT-116 cells, which are comparable to the IC50 of the standard anticancer drug 5-FU (16.14 μg mL−1 for MCF-7 and 10.21 μg mL−1 for HCT-116). These results further substantiate the potent cytotoxic activity of HMPC (Fig. 8A and B). A time-dependent study demonstrated that HMPC treatment resulted in a 48.47% reduction in cell viability for MCF-7 cells and a 51.49% reduction for HCT-116 cells at 24 hours. At 48 hours, the reduction in cell viability was 30.89% for MCF-7 cells and 38.23% for HCT-116 cells (Fig. 8C). These results underscore the strong cytotoxic potential of HMPC against MCF-7 and HCT-116 cell lines. Furthermore, HMPC exhibited minimal toxicity to human lymphocytes at concentrations up to 50 μg mL−1, with a slight reduction in cell viability, as the viability at 50 μg mL−1 was recorded at 52.32%. Overall, these findings suggest that HMPC could be a promising anticancer agent for targeting MCF-7 and HCT-116 cells (Fig. 8D, HLC).
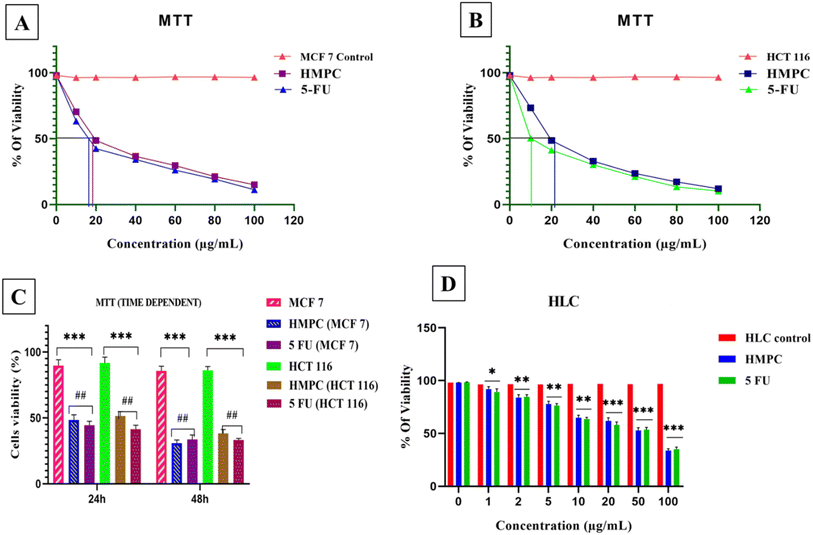 |
| Fig. 8 MTT assay: cytotoxic effects of HMPC on MCF-7 cells at different concentrations, with an IC50 value of 19.26 μg mL−1 (A). Cytotoxic effects of HMPC on HCT-116 cells at different concentrations, with an IC50 value of 21.31 μg mL−1 (B). Cell viability was assessed at 24 h and 48 h following treatment with HMPC at its IC50 concentrations (C). Cytotoxic study on HLC: a slight decrease in lymphocyte viability was observed at much higher concentration of HMPC (50 μg mL−1) (D). Data are presented as mean ± SEM of three independent experiments; **p < 0.01, ***p < 0.001, compared to the control group. | |
3.4.2 Fluorescence cell imaging study. During 1 hour incubation at 37 °C with HMPC at its IC50 concentration, less significant intracellular fluorescence was observed in fluorescent cell imaging on MCF-7 and HCT-116 cell lines. However, when the cells were incubated with an exogenous Zn2+ ion solution, a strong intracellular blue fluorescence was detected, with the fluorescence intensity of the HMPC-Zn2+ + complex being significantly higher (Fig. 9). This indicates that HMPC effectively penetrates the cell membrane and binds to intracellular Zn2+ ions, forming the HMPC-Zn2+ complex, as demonstrated by the fluorescence imaging. The fluorescence generation mechanisms of HMPC, Density Functional Theory (DFT) calculations were employed to analyze the electronic structure and frontier molecular orbitals of HMPC and the HMPC-Zn2+ complex. The results suggest that the fluorescence arises from the ligand-to-metal charge transfer mechanism facilitated by the coordination of Zn2+ ions with the HMPC molecule. In its free state, HMPC exhibits a less efficient intramolecular charge transfer due to the absence of metal ion coordination, which explains the low fluorescence intensity observed. Upon binding with Zn2+, the electronic transitions are significantly altered, as evidenced by leading to enhanced fluorescence.
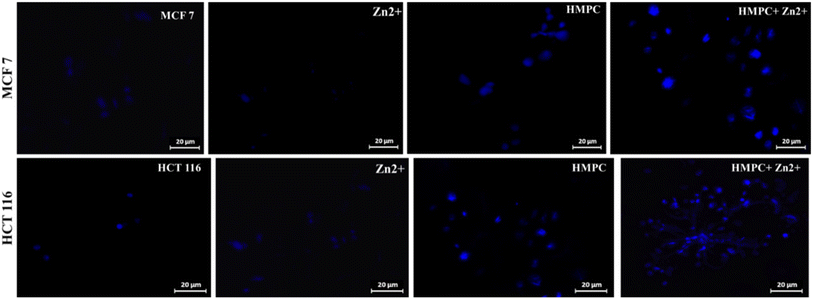 |
| Fig. 9 Intercellular biosensor cell imaging study was investigated on MCF 7 and HCT 116 cells. Cells were incubated alone with free HMPC and brighter blue fluorescence was observed HMPC-Zn2+ treated groups other than HMPC. The image was captured by a fluorescence microscope (ZEISS, Germany). | |
The strong blue fluorescence of the HMPC-Zn2+ complex highlights the specificity and efficiency of the Zn2+ binding. These findings demonstrate that HMPC could serve as a selective sensor for Zn2+ ions in bio-imaging applications under the given concentrations and incubation conditions, without inducing cytotoxic effects. By combining experimental fluorescence imaging and computational analysis, we provide a comprehensive understanding of HMPC have potential as a Zn2+ ion sensor against MCF-7 and HCT-116 cells.
3.5 Molecular docking study
The link between the mark protein (PDB-ID: 2VCJ) and the ligands is assessed by molecular docking.28–34 The designs reveal the free energy change of ligand HMPC for the interactions with protein 2VCJ, ΔG = −7.1 kcal mol−1 (Table 1) inside a grid box of 28.6 × 12.7 × 19.82 Å with dimensions 25 × 25 × 25 Å along three axes (Fig. 10a). The 2D diagram shows that ligand HMPC forms one hydrogen bonding interaction with the 51st asparagine residue, along with a pi–sulfur bond with the 98th methionine residue of the A chain in the binding site of the protein 2VCJ (Fig. 10b).
Table 1 Results of the docking of ligands and target protein 2VCJ
Name of ligands |
Docking score (kcal mol−1) |
HMPC |
−7.1 |
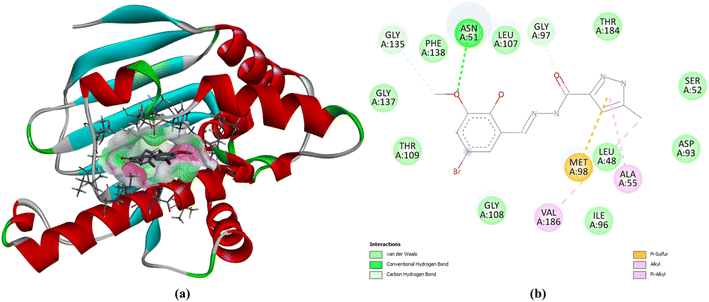 |
| Fig. 10 Docking poses of HMPC with 2VCJ, (a) at active site, (b) 2D diagram of the docked complex. | |
The link between the mark protein (PDB-ID: 1T69) and the ligand HMPC is assessed by molecular docking. The designs reveal the free energy change of ligand HMPC for the interaction with protein 1T69, ΔG = –6.5 kcal mol−1 (Table 2) inside a grid box of 30.09 × −3.16 × −13.64 Å with dimensions 22 × 22 × 22 Å along three axes (Fig. 11a). The 2D diagram (Fig. 11b) shows that ligand HMPC forms two hydrogen bonding interactions with the 33rd lysine residue and 180th histidine residue of chain A in the binding site of the protein 1T69, along with van der Waals and pi–pi stacked interactions as shown in the Fig. 11b.
Table 2 Results of the docking of ligands and target protein 1T69
Name of ligands |
Docking score (kcal mol−1) |
HMPC |
−6.5 |
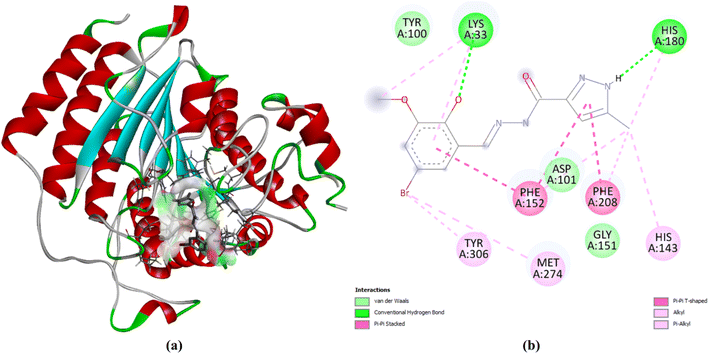 |
| Fig. 11 Docking poses of HMPC with 1T69, (a) at active site, (b) 2D diagram of the docked complex. | |
3.6 ADME prediction results
The result from SWISS-ADME35 is agreeable as HMPC offers no violation of Lipinski's, Veber's, and Egan's rule of drug-likeness properties and it has high gastrointestinal absorption (Fig. S7a†), no blood–brain barrier (BBB) permeant property (Fig. S7a†), it has a good bio-radar (Fig. S7b†) which makes it orally admissible to people. HMPC does not inhibit any of the cytochrome P50 enzymes except the CYP1A2 inhibitor, and is not a P-gp substrate. It has a good log
P value of 1.97, essential for showing drug-like properties. It has a Total Polar Surface Area (TPSA) of 99.60 Å2 (Table S1†).
4. Conclusion
In conclusion, the fluorescent sensor HMPC has demonstrated high selectivity and sensitivity for Zn2+ detection, with a detection limit well below the WHO guideline. Its fluorescence enhancement is driven by a CHEF mechanism through the suppression of PET and enhancement of ICT, as validated by comprehensive spectroscopic analyses and computational studies. Additionally, HMPC shows promising biological applications, including low cytotoxicity and potential anticancer activity, as supported by molecular docking studies with HSP90 and HDAC8 proteins. With a favorable ADME profile and strong binding affinities, HMPC presents potential not only as a fluorescent probe for Zn2+ but also as a candidate for anticancer drug development. Furthermore, the cytotoxicity and cell imaging studies conducted with MCF-7 cells indicate the potential of HMPC for biological applications, paving the way for further exploration in the field of chemosensing and biomedicine.
Data availability
The data supporting this article have been included as part of the ESI.†
Conflicts of interest
The authors declare no competing financial interest.
Acknowledgements
MD acknowledges to Science & Engineering Research Board (SERB), Govt. of India (ref. No. PDF/2016/000334). AHS thankful to the Researchers Supporting Project number (RSP2025R373) at King Saud University, Riyadh, Saudi Arabia for supporting this research. AKD specially acknowledges State University Research Excellence (SERB-SURE) of the Science and Engineering Research Board (SERB) (File Number: SUR/2022/ 002461) under Anusandhan National Research Foundation (ANRF) and Department of Science and Technology (DST), Government of India, for the financial support by the research grant. The authors express their gratitude to Christ University, Bangalore, for providing research facilities, and Center for Research, Christ University for the seed money grant (grant approval number CU-ORS-SM-24/09). Malavika S. Kumar extends her appreciation to the University Grants Commission (UGC), Government of India, for the Savitribai Jyotirao Phule Fellowship for Single Child (SJSGC), F. No. 82-7/2022(SA-III).
References
- A. W. Czarnik, Acc. Chem. Res., 1994, 27, 302–308 CrossRef.
- A. W. Czarnik, J. Org. Chem., 1993, 58, 5797–5801 CrossRef.
- J. Fraústo da Silva and R. J. P. Williams, in The Biological Chemistry of the Elements, Clarendon Press, Oxford, 1991, p. 302 Search PubMed.
- P. D. Zalewski, I. J. Forbes, G. Mazdai and C. Giannakis, Biochem. Inter., 1991, 24, 1093–1101 Search PubMed.
- S. J. Martin, G. Mazdai, J. J. Strain, T. G. Cotter and B. M. Hannigan, Clin. Exp. Immunol., 1991, 83, 338–344 CrossRef PubMed.
- D. E. Epner and H. R. Herschman, J. Cell. Physiol., 1991, 148, 68–74 CrossRef.
- R. J. Cousins and L. M. Lee-Ambrose, J. Nutr., 1992, 122, 56–64 CrossRef.
- X. M. Xie and T. G. Smart, Nature, 1991, 349, 521–524 CrossRef PubMed.
- C. J. Frederickson, Int. Rev. Neurobiol., 1989, 31, 145–238 CrossRef PubMed.
- L. M. T. Canzoniero, S. L. Sensi and D. W. Choi, Neurobiol. Dis., 1997, 4, 275–279 CrossRef.
- D. W. Choi and J. Y. Koh, Annu. Rev. Neurosci., 1998, 21, 347–375 CrossRef PubMed.
- F. Grummt, C. Wienmann-Dorsch, J. Schneider-Schaulies and A. Lux, Exp. Cell Res., 1986, 163, 191–200 CrossRef.
- N. L. Harrison and S. Gibbons, J. Neuropharmacology, 1994, 33, 935–952 CrossRef.
- B. L. Vallee and K. H. Falchuk, Physiol. Rev., 1993, 73, 79–118 CrossRef PubMed.
-
(a) C. J. Frederickson, J.-Y. Koh and A. I. Bush, Nat. Rev. Neurosci., 2005, 6, 449 CrossRef PubMed;
(b) S. Goswami, S. Maity, A. C. Maity, A. K. Das, K. Khanra, T. K. Mandal and N. Bhattacharyya, Tetrahedron Lett., 2014, 55, 5993–5997 CrossRef;
(c) S. Maity, A. C. Maity, A. K. Das and N. Bhattacharyya, Anal. Methods, 2022, 14, 2739–2744 RSC.
-
(a) A. I. Bush, W. H. Pettingell, G. Multhaup, M. d Paradis, J. P. Vonsattel, J. F. Gusella, K. Beyreuther, C. L. Masters and R. E. Tanzi, Science, 1994, 265, 1464–1467 CrossRef;
(b) F. Joy, K. P. Chaithra, A. Nizam, A. Deepti, P. S. Baby Chakrapani, A. K. Das, T. P. Vinod and Y. Nair, Chem. Eng. J., 2023, 453, 139798 CrossRef;
(c) G. C. Das, A. Kumar Das, D. Das, T. Raj Maity, A. Samanta, F. Ali Alasmary, A. Salem Almalki, A. Iqbal and M. Dolai, J. Photochem. Photobiol., A, 2023, 440, 114663 CrossRef.
-
(a) Z. Xu, J. Yoon and D. R. Spring, Chem. Soc. Rev., 2010, 39, 1996–2006 RSC;
(b) S. Goswami, A. K. Das, K. Aich, A. Manna, S. Maity, K. Khanra and N. Bhattacharyya, Analyst, 2013, 138, 4593–4598 RSC.
- P. Jiang and Z. Guo, Coord. Chem. Rev., 2004, 248, 205–229 CrossRef.
-
(a) D. A. Skoog, F. James Holler and S. R. Crouch, Principles of Instrumental Analysis, Cengage Learning, 2017, vol. 27, pp. 992 Search PubMed;
(b) S. Sogra, V. Aishwarya, P. S. Chaithra, L. Suchi, S. Abhishek, S. Vishnu and A. K. Das, J. Fluoresc., 2024 DOI:10.1007/s10895-023-03552-1;
(c) A. K. Das and S. Goswami, Sens. Actuators, B, 2017, 245, 1062–1125 CrossRef;
(d) R. Pandey, A. Kumar, Q. Xu and D. S. Pandey, Dalton Trans., 2020, 49, 542–568 RSC.
- W.-K. Dong, S. Folaranmi Akogun, Y. Zhang, Y.-X. Sun and X.-Y. Dong, Sens. Actuators, B, 2017, 238, 723–734 CrossRef CAS.
- Q.-Y. Yu, C.-W. Wei, X.-J. Wang, S.-Q. Gao, X.-Y. Tong and Y.-W. Lin, J. Biol. Inorg. Chem., 2023, 28, 205–211 CrossRef PubMed.
-
(a) P. Bera, N. Saha, S. Kumar, D. Banerjee and R. Bhattacharya, Transition Met. Chem., 1999, 24, 425 CrossRef;
(b) B. Das, K. Chandra Murmu, S. K. Dey, S. M. Chaudhuri, Z. M. Almarhoon, M. Z. Ansari, P. P. Bag and M. Dolai, Inorg. Chem. Commun., 2024, 170, 113108 CrossRef;
(c) S. Goswami, S. Maity, A. C. Maity, A. K. Das, K. Khanra, T. K. Mandal and N. Bhattacharyya, Tetrahedron Lett., 2014, 55, 5993–5997 CrossRef;
(d) S. Goswami, A. K. Das, B. Pakhira, S. B. Roy, A. K. Maity, P. Saha and S. Sarkar, Dalton Trans., 2014, 43, 12689–12697 RSC.
-
(a) K. Komatsu, K. Kikuchi, H. Kojima, Y. Urano and T. Nagano, J. Am. Chem. Soc., 2005, 127, 10197–10204 CrossRef PubMed;
(b) H. H. Wang, Q. Gan, X. J. Wang, L. Xue, S. H. Liu and H. Jiang, Org. Lett., 2007, 9, 4995–4998 CrossRef PubMed;
(c) J. Du, J. Fan, X. Peng, H. Li and S. Sun, Sens. Actuators, B, 2010, 144, 337–341 CrossRef.
- M. Shortreed, R. Kopelman, M. Kuhn and B. Hoyland, Anal. Chem., 1996, 68, 1414–1418 CrossRef PubMed.
-
(a) H. A. Benesi and J. H. Hildebrand, J. Am. Chem. Soc., 1949, 71, 2703–2707 CrossRef;
(b) Y. Shiraishi, Y. Kohno and T. Hirai, Ind. Eng. Chem. Res., 2005, 44, 847–851 CrossRef.
- H. Niu, J. Liu, H. M. O'Connor, T. Gunnlaugsson, T. D. James and H. Zhang, Chem. Soc. Rev., 2023, 52, 2322–2357 RSC.
-
(a) M. A. Haidekker and E. A. Theodorakis, Org. Biomol. Chem., 2007, 5, 1669–1678 RSC;
(b) A. K. Chibisov, G. V. Zakharova and H. Görner, J. Chem. Soc., Faraday Trans., 1996, 92, 4917–4925 RSC;
(c) M. S. A. Abdel-Mottaleb, R. O. Loutfy and R. Lapouyade, J. Photochem. Photobiol., A, 1989, 48, 87–93 CrossRef;
(d) M. S. Kumar, V. S., M. Dolai, A. Nag, Y. Bylappa and A. K. Das, Anal. Methods, 2024, 16, 676–685 RSC.
-
(a) S. Pakrashy, P. K. Mandal, J. Nanda Goswami, S. K. Dey, S. Maiti Choudhury, B. Bhattacharya, F. Emmerling, F. A. Alasmary and M. Dolai, ACS Omega, 2022, 7, 48572–48582 CrossRef PubMed;
(b) S. Mabhai, M. Dolai, S. K. Dey, A. Dhara, S. M. Choudhury, B. Das, S. Dey and A. Jan, Spectrochim. Acta, Part A, 2019, 219, 319–332 CrossRef PubMed.
- S. Pakrashy, P. K. Mandal, S. K. Dey, S. M. Choudhury, F. A. Alasmary, A. S. Almalki, M. A. Islam and M. Dolai, ACS Omega, 2022, 7, 33408–33422 CrossRef PubMed.
- S. Misra, S. Pakrashy, S. Paul, P. K. Maurya, P. S. Sardar, A. Bose and A. Majhi, Interaction of a new triazole compound with Serum albumins and Proteolytic enzyme Bromelain by Steady state fluorescence and Molecular docking techniques, J. Biochem., Mol. Biol. Biophys., 2024, 61, 305–313 Search PubMed.
- S. Misra, S. Paul, S. Pakrashy, S. Ghosh, S. Naskar, P. K. Maurya, P. S. Sardar, K. Venkateswarlu, A. Bose and A. Majhi, Indian J. Chem., 2023, 62, 1001–1011 Search PubMed.
- M. Dolai, S. Pakrashy, A. K. Ghosh, S. Biswas, S. Konar, F. A. Alasmary, A. S. Almalki and M. A. Islam, J. Mol. Struct., 2023, 1274, 134584 CrossRef.
- B. Das, S. Pakrashy, G. C. Das, U. Das, F. A. Alasmary, S. M. Wabaidur, M. A. Islam and M. Dolai, J. Fluoresc., 2022, 32, 1263–1277 CrossRef PubMed.
- S. Pakrashy, S. Chakraborty, S. Manna, J. Nanda Goswami, B. Bhattacharya, F. Emmerling, J. Mandal, S. Misra, S. Maiti Choudhury, M. K. Okla, A. Bose, P. K. Maurya, A. Majhi and M. Dolai, ACS Appl. Bio. Mater., 2024, 7, 3414–3430 CrossRef PubMed.
- A. Daina, O. Michielin and V. Zoete, Sci. Rep., 2017, 7, 42717 CrossRef PubMed.
|
This journal is © The Royal Society of Chemistry 2025 |
Click here to see how this site uses Cookies. View our privacy policy here.