DOI:
10.1039/D4BM01027B
(Paper)
Biomater. Sci., 2025,
13, 193-209
Enhanced wound healing with biogenic zinc oxide nanoparticle-incorporated carboxymethyl cellulose/polyvinylpyrrolidone nanocomposite hydrogels
Received
3rd August 2024
, Accepted 29th September 2024
First published on 15th October 2024
Abstract
Contemporary wound dressings lack antibacterial properties, exhibit a low water vapour transmission rate, and demonstrate inadequate porosity. In order to overcome these limitations, scientists have employed water hyacinth to produce carboxymethyl cellulose (CMC). CMC/PVP nanocomposite films containing biogenic zinc oxide nanoparticles (nZnOs) were synthesised using cost effective solution-casting technique. As the proportion of nZnOs in the film increased, swelling and water permeability decreased, whereas mechanical stability improved. Dynamic light scattering testing and transmission electron microscopy confirmed that the particle size was around 50.7 nm. Field emission scanning electron microscopy (FESEM) images showed that nZnOs were distributed uniformly in the polymer matrix. Cell viability against Vero cells was greater than 94%, and a substantial zone of inhibition against S. aureus and E. coli bacteria was observed. Wounds of albino mice were treated with CMC/PVP and CMC/PVP/nZnO (6%) nanocomposite hydrogels and healed in 20 and 12 days, respectively, as demonstrated by wound healing assay and histological staining. In vitro and in vivo studies revealed that the novel nanocomposite hydrogels exhibit improved cell viability and wound healing features. Therefore, they could be exploited as promising skin wound dressing materials.
1 Introduction
Wounds, known as a “silent epidemic”, have a significant impact on global health, patients’ lives, and psychological well-being.1 Treatment of chronic wounds is still a challenge because of the prolonged time required for healing. Slow-healing wounds involve bacterial infections, pre-existing medical conditions (such as diabetes and cancer), malnutrition, obesity, and smoking. Oxidative stress is caused by an imbalance between reactive oxygen species (ROS) and a tissue's or cell's ability to neutralise these and repair itself. Low ROS levels are required for wound healing, whereas high levels of ROS impede the cellular and molecular processes of healing.2,3
Hydrogels are chemically or physically cross-linked 3D polymeric networks that can absorb significant volumes of water or biological fluids into their interstitial structures owing to the presence of hydrophilic groups.4,5 Moreover, superabsorbent polymers (SAPs) show enhanced water retention in saline and de-ionised water.6 Water is either bonded with polar hydrophilic groups or is free, filling the spaces between chains, pores, or holes.7 The ability of a hydrogel to absorb and retain water is determined by crosslinking as well as ionic strength, temperature, and pH conditions.8
Hydrogels have recently received substantial attention for their potential to be more effective in tissue engineering, drug delivery, self-healing, and biosensors than other alternatives.9–12 Natural polymeric hydrogels have fewer adverse effects and are more biocompatible than synthetic polymeric hydrogels.13 Natural and man-made hydrogels are blended to enhance longevity, water retention capacity, and rheological properties.5
The water hyacinth (Eichhornia crassipes) plant is a fast-growing invasive species. It has recently gained popularity because of its high biodegradability and cellulose content (64.51%). Its water-absorption capacity and ecological compatibility have attracted attention towards its hydrogel-making ability.14,15
Carboxymethyl cellulose (CMC) is extremely hydrophilic, which allows it to absorb wound exudates and maintain a moist environment around the wound.16,17 It is a cellulose derivative that creates macromolecular electrostatic repulsion, allowing water to be absorbed and is non-toxic.18,19
Polyvinylpyrrolidone (PVP) is non-toxic, highly water-soluble, biocompatible, biodegradable, heat-resistant, wettable, adhesive, and film-forming. As a result, it is extensively used in medicine, food, cosmetics, and other industries. In the pharmaceutical and biomedical fields, it has been approved by the US Food and Drug Administration as a safe and secure polymer for conducting biological studies.20–22
Superabsorbent hydrogels were successfully fabricated using epichlorohydrin as a crosslinker in a NaOH/urea aqueous system. However, urea exerts toxicity via reactive oxygen species (ROS) generation in various cells.12,23 CMC and HEC have been previously crosslinked in water with divinyl sulfone (DVS). Yet DVS must not be utilised in hydrogels because of its toxicity.8 Citric acid (CA), a crosslinking agent, has been designed to be non-toxic and cost-effective. It is widely used in the pharmaceutical and food sectors. It is a benign and intuitive chemical substance, suitable for making new functional biomaterials.24 The non-toxic nature of citric acid will help to develop biodegradable hydrogels which are harmless to the environment.8,25
Citric acid (CA) was used to crosslink sodium carboxymethylcellulose (Na-CMC) and hydroxypropylmethylcellulose (HPMC) hydrogel films.26 However, CMC hydrogels have a very low mechanical strength, especially in swollen states, which restricts their use. Blending CMC with PVP solves this problem, expanding its potential to be a new type of wound dressing material. Antimicrobial performance tests and in vivo experiments were not used to evaluate the clinical efficacy of the developed CMC/PVP films for wound healing applications.27,28 Boric acid, an antibacterial agent, was added to the CMC/PVP hydrogel for burn or cut wound healing, although antioxidant and in vivo tests were not done.29 Ultrasonication-assisted solution casting was used to develop CMC/Polyvinyl alcohol/nano-zinc-oxide/multilayer graphene nanoplatelet composite films for commercial food packaging without cytotoxicity testing.30 Some researchers prepared CMC/PVP/CuO nanocomposites,31 CMC/PVP/Au nanocomposites,32 CMC/PVP/activated carbon composites,33 and CMC/PVP/PEG/agar composites successfully, but their antibacterial activities were not tested. The CMC/PVP/CE (6%) composite hydrogel demonstrated reduced antibacterial efficacy. Additionally, mechanical and rheological tests were not executed for this composite hydrogel.34 Besides their superior swelling capacity and favourable elasticity, the PVP/CMC hydrogels exhibited additional features indicating their potential as wound dressing materials; however, no assessments of wound healing or biocompatibility had been carried out.35 A biocompatible, injectable, self-healing PVP/CMC hydrogel for drug release was synthesised.36 However, some indispensable assays for wound healing applications, such as cell viability, hemolytic activity, wound healing rate, H & E staining, antibacterial activity, and antioxidant activity were deficient.
In natural polymers, metal oxide nanoparticles enhance physicochemical zinc oxide nanoparticles’ mechanical and biological properties. Collagen is retrieved by metal oxide nanoparticles, which have a high level of antibacterial activity without causing any damage to eukaryotic cells.3 The microscopic size and high surface-to-volume ratio of zinc oxide nanoparticles (nZnOs) allow them to interact directly with microbial membranes, resulting in increased antimicrobial activity over zinc salts. Their antibacterial effect comes from reactive oxygen species, which damage cellular structures and release metal ions into solution.35 In addition, the zinc ions released by the nanoparticles encourage keratinocytes to migrate to the wound, which aids in healing. A potentially bioactive wound dressing, based on CMC/ZnO/MCM-41 nanocomposite hydrogels, was prepared. It showed far better results in tensile strength (12%) but less swelling (100%).36 The main advantages of using zinc oxide over silver nanoparticles were affordability and colourlessness. Zinc oxide nanoparticles (nZnOs) inhibit S. aureus and E. coli in wounds and are inexpensive and secure.37
Recent features related to wound dressings encompass restricted porosity, diminished antibacterial efficacy, challenges in dressing removal after healing, and instances of allergic reactions. Hydrogels made from CMC/PVP that incorporate zinc oxide nanoparticles (nZnOs) have not been developed to date. This study focuses on the assessment of biogenic nZnO-impregnated viscoelastic, antibacterial and novel CMC/PVP nanocomposite hydrogels as promising skin wound dressing materials.
2 Experimental
2.1 Materials
Water hyacinth (Eichhornia crassipes) plant stems were collected from a local pond in Rajshahi, Bangladesh. Polyvinylpyrrolidone (PVP, MW ∼40
000), sodium hydroxide (NaOH), 100% acetic acid (CH3COOH), sodium chlorite (NaClO2), methanol, 100% ethanol, citric acid and zinc acetate were purchased from Merck (Germany). DPPH (Sigma Aldrich), monochloroacetic acid (Qualikems, India), isopropanol (Riedel-deHaen, Germany), and Nebanol Plus ointment (Square Pharma, Bd.) were also purchased. All these chemicals were of analytical grade and used without further purification.
2.2 Preparation of water hyacinth (Eichhornia crassipes) cellulose
Water hyacinth (Eichhornia crassipes) plants were collected and cleaned. The plant stems were separated from the roots and leaves and air-dried for around 15 days. The air-dried water hyacinth was crushed into 60 mesh powder. It was heated in a Soxhlet system to remove the wax using a 2/1 ratio of a toluene/ethanol solvent. After dewaxing, the sample was hydrolysed with 3.5% (v/v) HNO3 solution at 70 °C for 2 h in a fibre-to-liquor ratio of 1/50. The lignin content was completely removed using a 0.7% (w/v) NaClO2 solution in a thermostatic water bath at 80 °C for 3 h, maintaining a fibre-to-liquor ratio of 1/50. The bleached cellulose was treated with 17.5% (w/v) NaOH solution in a fibre-to-liquor ratio of 1/30 to remove the hemicellulose content and then allowed to stand for 3 h at room temperature. After that, the obtained cellulose was rinsed with distilled water before neutralisation with 2% acetic acid. Then drying was carried out in an oven at 60 °C for 2–3 h.14,15,19
2.3 Na-CMC preparation
First, 9 grams of water hyacinth (WH) cellulose powder, 30 ml of 30% (w/v) NaOH, and 270 ml of isopropanol were mixed in a beaker under stirring and allowed to stand at room temperature for 30 min. After that, 10.8 g monochloroacetic acid was added to the beaker and stirred for 60 min. The beaker was then wrapped in aluminium foil and kept at 55 °C for 2 h. During this time, the reaction continued and the slurry was divided into two phases. To remove undesirable salts, the upper phase was discarded and sedimentary phases were suspended in 70% methanol (100 ml) and neutralized with glacial acetic acid, followed by filtration and washing for five times with 70% ethanol (300 ml). Afterwards, it was rinsed with 100% methanol and filtered once more. Na-CMC was obtained, dried in an oven at 55 °C and stored.38 The CMC synthesis reactions are shown in eqn (1) + eqn (2) + eqn (3). | Cell-OH + NaOH = Cell-ONa + H2O | (1) |
| Cl-CH2COOH + NaOH = Cl-CH2COONa + H2O | (2) |
| Cell-ONa + ClCH2COONa = Cell-O-CH2COONa + NaCl | (3) |
2.4 Biosynthesis of zinc oxide nanoparticles
First, 25 grams of freshly collected neem leaves (Azadirachta indica) were washed with 100 ml deionised water at 65 °C for 20 min to obtain an aqueous extract. The aqueous extract was filtered after agitation in a magnetic stirrer till the colour turns from orange to brown. The plant extract was kept in a cool place to prevent any degradation. ZnO nanoparticles were prepared by mixing 25 mL of the neem extract with 25 mL of zinc acetate. The pH of the solution was maintained at 7.0 by adding 0.5 M sodium hydroxide (NaOH) at room temperature, resulting in a white precipitate.
After washing the collected sample with water and ethanol several times, the impurities were eliminated using a centrifuge (Model: Pro-Analytical, CR 4000 Medium Prime Centrifuge) Finally, the precipitate was dried in an oven at 60 °C and calcined in a muffle furnace (Model: DMF-05) at 400 °C for an hour.39,40Eqn (4) displays the ZnO nanoparticle formation reactions.
| 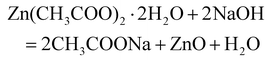 | (4) |
2.5 Preparation of CMC/PVP/nZnO nanocomposite hydrogels
The hydrogel films were prepared by a solution casting method using an aqueous solution consisting of Na-CMC, PVP and citric acid as a cross-linker. First, 5% (w/v) Na-CMC solution was prepared by dissolving 1 g Na-CMC in 20 ml deionized water and by thermally treating it with citric acid (10% based on the total polymer matrix) at 190 °C for 15 minutes in a hot air oven to achieve CA crosslinking of Na-CMC.41 Then, CMC/PVP blends were obtained by mixing 5% (w/v) CA crosslinked Na-CMC and 5% (w/v) PVP polymer solutions were separately prepared, maintaining a ratio of 80/20 (v/v). The mixture was then heated at 80 °C for 60 min under mild stirring.42
Nanocomposites were prepared by gradual addition of the nZnO suspension at different concentrations (2%, 4% and 6 wt%) to the CMC/PVP solution at the time of stirring. Then, 0.25 ml sorbitol per gram of polymeric mixture was added as a plasticiser, and the mixture was sonicated using a digital ultrasonic cleaner (Model: YJ5120-1) for homogenization at 25 °C for 15 min. The solution was poured into a glass mould and left for atmospheric evaporation at room temperature for 24 h. The homogeneous CMC/PVP/nZnO suspensions were poured into a transparent glass Petri dish and left at room temperature for 72 h, in order to evaporate the solvent, and a film was formed. Then the suspensions were transferred to a freeze-drier for drying.42,43 The incorporation of ZnO nanoparticles into the CMC/PVP polymer matrix occurs through the formation of dipole-dipole interactions and hydrogen bonds among nZnO, CMC, PVP and CA. The possible schematic diagram of the CMC/PVP/nZnO nanocomposite hydrogel is shown in Fig. 1.
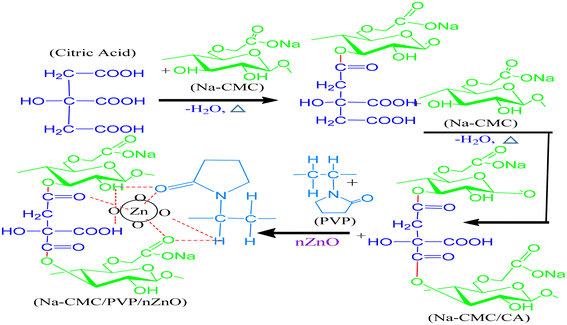 |
| Fig. 1 Reaction mechanism for the synthesis of the CMC/PVP/nZnO nanocomposite hydrogel. | |
2.6 Determination of the degree of substitution (DS) of the prepared CMC
Sodium carboxymethylcellulose (CMC) is a high-polymer cellulose ether. Each glucose unit contains three hydroxyl groups, namely C2, C3, and C6. The degree of substitution (DS) expresses the amount of hydrogens in the hydroxyl group of a glucose unit that have been replaced by carboxymethyl groups. The degree of substitution has a great influence on solubility, emulsibility, thickening ability, and stability of CMC.44 The conventional approach was used to determine the degree of substitution of CMC (ASTM, 1961). Then, 108 ml of 65% HNO3 was made up to one litre with methanol. Five grams of prepared CMC was shaken with 200 ml of dil. HNO3–methanol mixture, kept for 3 h and then filtered. The excess acid was washed with 70% methanol. Two grams of dried washed CMC was added to 200 ml of distilled water and 30 ml of 1 N NaOH. After dissolving, the mixture was titrated with 1 N HCl.45,46 Then DS of the prepared CMC was calculated using eqn (5). | DS = 0.162A/(1 − 0.058A) | (5) |
where A = (BC − DE)/F, A is the equivalent weight of alkali required per gram of sample, B (ml) is the amount of NaOH solution, C is the normality of NaOH solution, D (ml) is the amount of HCl solution, E is the normality of HCl solution and F (g) is the weight of the sample.
2.7 Determination of gel content and swelling behaviour
To measure the gel content, about 0.1 g (1 × 2 cm2, thickness 0.3 mm) of CMC/PVP/nZnO nanocomposite hydrogels were dipped in a phosphate buffer solution at 37 °C for 2 days. Every 12 h, the phosphate buffer solution was replaced in the sample container to remove the soluble parts from the hydrogel. Then swollen samples were taken out, dried in an oven at 50 °C, and weighed.15 The gel content of nanocomposites was calculated using eqn (6): | 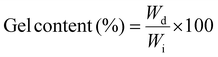 | (6) |
where Wd is the weight of the dried hydrogel after extraction and Wi is the initial weight of the hydrogel, respectively.
First, 0.1 g of dry sample (1 × 2 cm2, thickness 0.3 mm) was immersed into water at 25 °C and removed at predetermined time intervals. Surface water was absorbed by dabbing the sample with a filter paper. Swollen hydrogels were weighed, and the percentage of water uptake capacity was measured using eqn (7):
| 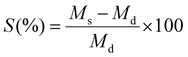 | (7) |
where
S is the equilibrium water absorbency (%) and
Ms and
Md are the weights of swollen hydrogel (g) at time
t and dry hydrogel (g), respectively in three different solutions, namely phosphate buffer of pH 6.8 and 7.4 and simulated gastric fluid,
i.e., HCl buffer of pH 1.2.
27
2.8 Water vapour transmission and porosity tests
The water vapour transmission rate (WVTR) was calculated using the European Pharmacopoeia (EP) standard. Hydrogel film samples (0.1 g, 1 × 2 cm2, thickness 0.3 mm) were cut into circular pieces and taped to the mouth of a container with 35 mm inner diameter containing 25 ml distilled water. The bottles were then kept in an oven at a temperature of 35 °C and a relative humidity of 35%. The formula (eqn (8)) was used to calculate the WVTR (g m−2 day): |  | (8) |
where A is the permeation area of samples, and Wi and Wf are the initial and final weights of bottles, respectively.47
Porosity is a measure of void (i.e., empty) spaces in a material that is expressed as a fraction of the volume of empty spaces over the entire volume, ranging from 0 to 1, or as a percentage ranging from 0 to 100.
The porosity of the nanocomposite hydrogels was found by the alcohol displacement method. In brief, the initial weight and volume (W and V) of the lyophilized gels were measured and recorded. The gels were then immersed in dehydrated alcohol until they are completely saturated. The gels were weighed again after the surface liquid had been removed. The porosities were calculated using eqn (9):
where
W1 and
W2 indicate the weight of the nanocomposite films before and after immersion, respectively and
V1 is the volume before immersion, with
ρ being the density of alcohol at room temperature.
48
2.9 Attenuated total reflectance-fourier transform infrared spectroscopy analysis
ATR-FTIR spectroscopy was carried out to analyse the Water Hyacinth Cellulose, CMC/PVP, and CMC/PVP/nZnO nanocomposite hydrogels. The spectra were recorded in the range of 4000–400 cm−1, using a PerkinElmer Fourier transform infrared (FTIR) spectrophotometer (Model: Paragon 500, PerkinElmer, UK) with a smart orbit Attenuated Total Reflectance (ATR) accessory. The ATR was fitted with a diamond crystal. The ATR-FTIR spectrum was ascertained in a transmittance mode15
2.10 UV-visible spectroscopy and dynamic light scattering analysis
UV-vis spectroscopy is an analytical technique that measures the number of discrete wavelengths of UV or visible light that a sample absorbs or transmits to a reference or blank sample. Then, 1 mL of nZnO suspension was prepared for UV-vis analysis and sonicated at 6000 rpm for 10 min. The UV-vis spectra were measured from 200 to 800 nm. UV-visible spectroscopy (Model: T60 UV-vis spectrophotometer) was also employed to evaluate the DPPH free radical scavenging activity.
Dynamic light scattering (DLS) is a popular technique for measuring particle size distributions in colloidal suspensions and emulsions. The particle size distribution of the biosynthesised nZnOs was measured by dispersing 10 mg of nZnOs in 100 ml of distilled water and sonicating for 30 min. Then its particle size was determined using a DLS apparatus (PSS, Santa Barbara, CA, USA).49
2.11 X-ray diffraction
XRD analysis was performed using a PAN Analytical X Pert PRO X-ray diffractometer (Model: Rigaku, SmartLab, Japan) to determine the phase and crystalline nature of the prepared nanocomposite hydrogel films. The crystallinity of extracted cellulose, PVP, CMC, CMC/PVP, and CMC/PVP/nZnO nanocomposites was investigated. Cu-Kα radiation of wavelength 1.5406 Å was used as the X-ray source. The scanning rate was 2 min−1 over a period with a scan angle ranging from 5° to 70°.48
2.12 Mechanical and rheological tests
The mechanical features of the developed nanocomposite hydrogel films (tensile strength (N mm−2)) were assessed using a texture analyzer (TA XT plus, Stable Microsystem, Godalming, UK) and a 5 kg loaded cell. Samples with 0.3 mm thicknesses (determined using a digital micrometer (Digimatic Micrometer, QuantuMike IP 65, Mitutoyo, Japan)) and no surface defects were chosen. A film of size 1 × 2 cm2 was cut and grasped between the clamps, which was then separated at a rate of 50 mm min−1. Mechanical tests on developed composites were carried out at 65% relative humidity and room temperature. The experiment was carried out in triplicate.50 Then, eqn (10) and (11) were used to calculate the TS and EB of the films: | 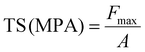 | (10) |
| 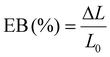 | (11) |
The dynamic rheological characteristics of the hydrogels were determined using a stress-controlled rheometer. Using a MARS III Haake Rotational Rheometer (MARS III, Germany), frequency sweeps were performed at angular velocities between 0.1 and 10 Hz. The storage moduli (G′) and loss moduli (G′′) of the hydrogel were analyzed.51
For hydrogels, we obtained the dependences of the storage modulus G′ and the loss modulus G′′ on the frequency of exposure to load γ.
The storage modulus defines the elastic characteristics of the hydrogel, whereas the mechanical loss modulus describes its viscosity properties (eqn (12) and (13)):
| G′ = (σ0/γ0)cos δ | (12) |
| G′′ = (σ0/γ0)sin δ | (13) |
where
σ0 is the stress,
γ0 is the strain magnitude and
δ is the phase angle between stress and strain.
The tangent phase angle was determined as follows:
| tan δ = G′/G′′. | (14) |
2.13 Surface morphology analyses
The images of the samples were acquired by ultra-high-resolution electron imaging and the scans were performed at 5.0 kV accelerating voltage using a field emission scanning electron microscope (FESEM; JEOL, Model-JSM7600 F, Japan).
The additional imaging of nZnOs was performed by Transmission Electron Microscopy (TEM) using a Talos S200 microscope (FEI, Hillsboro, OR, USA) at 200 kV to examine their size and morphology. The TEM images were acquired and subsequently annotated using Image-J (free software, Version 1.53q; NIH, Bethesda, MD, USA) to assess the size distributions and compute the average diameter.52
2.14 Antioxidant activity test
The antioxidant activity of the CMC-based composite films was investigated using a 2,2-diphenyl-1-picrylhydrazyl (DPPH) radical. About 50 mg of the film sample was dipped in 5 mL of 0.004% (w/v) DPPH, in methanol solution, and incubated at room temperature for 60 min. Its absorbance was measured at 517 nm using a UV-vis spectrophotometer. An identical DPPH solution was used without the film as a control. The antioxidant activity of the film samples was determined53 using eqn (15): |  | (15) |
where Ac and As are the absorbances of DPPH of the control and test films, respectively.
2.15 Antibacterial efficacy test
Escherichia coli and Staphylococcus aureus species of bacteria were cultured in a nutrient agar medium. The prepared films were then cut into a circle with a diameter of about 6 mm. After culturing the bacteria on the agar medium, the cut film pieces were placed on the culture surface. After storage at 37 °C for 48 h, the circular area of the inhibited zone around the film was measured 3 times and the average of these measurements was recorded.54
2.16 Experimental animal, ethical clearance and in vivo wound healing study
This investigation was conducted according to the “ARRIVE guidelines”55 and approved under license no: 455(12)/320/IAMEBBC/IBSc, 14 September 2023. First, male Swiss albino mice were sedated. Then their superior posterior areas were shaved. A full-thickness skin wound of 10 mm diameter was created on the shaved areas. The wounds were immediately treated with five substances: Nebanol (antibiotic), CMC/PVP, CMC/PVP/nZnO (2%), CMC/PVP/nZnO (4%) and CMC/PVP/nZnO (6%), respectively. They were allowed unlimited food and drink. To evaluate the wound healing capability of hydrogel dressings, the wound images were recorded at different time intervals to calculate the wound healing rate3 using eqn (16): | 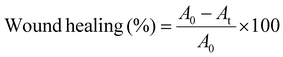 | (16) |
where At and A0 were the wound areas on the specified day and the day of operation, respectively.
On the 12th day, the mice were killed. The wound tissue samples were thoroughly biopsied. Then the tissue samples were fixed in 10% neutral buffer formalin for 24 hours, embedded in paraffin, and sliced at a thickness of 6–10 μm, using a microtome. The sections were deparaffinised, dehydrated through a series of graded ethanol, stained with hematoxylin and eosin (H&E), and viewed using an optical microscope (OLYMPUS, CX31, Tokyo, Japan).
2.17 Cytotoxicity and blood compatibility test
The cytotoxicity of the nanocomposite hydrogel films was assessed using Vero cells, derived from the kidney cells of African green monkeys (Vero, ATCC CCL-81). The nanocomposite hydrogel films, after drying, were pressed into circular samples with a diameter of 12 mm. The samples were sterilised in an autoclave at 121 °C for 10 min. Subsequently, the samples were transferred to 24-well growth plates aseptically. Each well was supplemented with 1 ml of minimal essential medium (MEM) (a culture medium). The medium was allowed to equilibrate with the samples for 30 min. In each well, a metallic ring and 0.5 metres of MEM culture medium (supplemented with 10% fetal calf serum) were introduced prior to cell seeding. Vero cells were cultured on 24-well plates with an initial density of 6 × 104 cells per well for both the nanocomposite hydrogel films and the control. The cells were incubated at a temperature of 37 °C in an environment containing 5% CO2, which had been humidified, for 16 h. Subsequently, the culture media were replaced with a serum-free minimum essential medium (MEM) for an additional 48 h, to facilitate the cultivation of Vero cells. Following the incubation period, the viable cells were counted by an MTT (3-[4,5-dimethylthiazol-2-yl]-2,5-diphenyl tetrazolium bromide) assay.56
The release of hemoglobin into the plasma is known as hemolysis. Hemolysis occurs when the erythrocyte membrane breaks. The hemolytic activity of nanocomposite hydrogels was tested.
The blood used for testing was diluted. Then 2 mL of fresh blood was mixed with 2.5 mL of a saline solution containing 0.9% sodium chloride. Nanocomposite hydrogel films, measuring approximately 1 × 1 cm2 and weighing 20 mg, were added with calibration into 4 mL of saline solution, for a duration of 30 min, at a temperature of 37 °C. Following this, a volume of 0.1 mL of dilute blood was introduced into each sample, which was then incubated for a period of 60 min at a temperature of 37 °C.
Positive and negative controls were conducted without nanocomposite hydrogels. The positive control was the addition of 0.1 mL of dilute blood to 4.0 mL of distilled water, resulting in 100% haemolysis. The negative control involved the addition of 0.1 mL of dilute blood to 4.0 mL of saline solution, resulting in 0% hemolysis. The solutions were placed in a centrifuge at a speed of 2500 rpm for 5 min. The absorbance at a wavelength of 545 nm was measured and hemolysis was calculated57 using eqn (17):
| 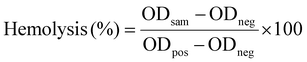 | (17) |
where OD
sam, OD
neg, and OD
pos are the adsorptions of the sample, negative control, and positive control, respectively.
2.18 Statistical analysis
All the experiments were conducted in triplicate, and the data are presented as mean values ± standard deviation. The resulting zones of inhibition for the antibacterial activity of the samples around the discs were measured in mm.
3 Results and discussion
3.1 Degree of substitution of the prepared Na-CMC
The degree of substitution (DS) was used to determine the solubility of the CMC. The DS values between 0.0 and 0.4 indicate that CMC is insoluble but swellable, whereas the values beyond this range indicate that CMC is completely water-soluble. Cellulose was extracted from water hyacinth (WH) and Na-CMC was synthesised from WH cellulose. Its average DS was 0.78.
3.2 Gel content and swelling behaviour
The gel content revealed the cross-linking of polymer chains in the hydrogel structure. The interaction of the hydrogel's components determines crystallinity and cross-linking. The gel content increased with the increase in the PVP ratio of the hydrogel structure and decreased with the increase in the CMC ratio. Thus, the interaction between PVP and other gel components changes the amount of crosslinking and can be used to control the flexibility and strength of the developed hydrogels.15
As shown in Fig. 2a, the composite hydrogel without nZnOs had the lowest gel content, which was 70%; the gel content increased to 74%, 77.5%, and 82% with additions of 2%, 4%, and 6% nZnOs, respectively. The interaction between the nanoparticles and the biopolymer chains exhibited an upward trend as the quantity of nZnO was augmented.58 These results confirmed that the addition of nZnOs to CMC/PVP promoted the cross-linking network and augmented the insolubility behaviour of the composite hydrogels in water.
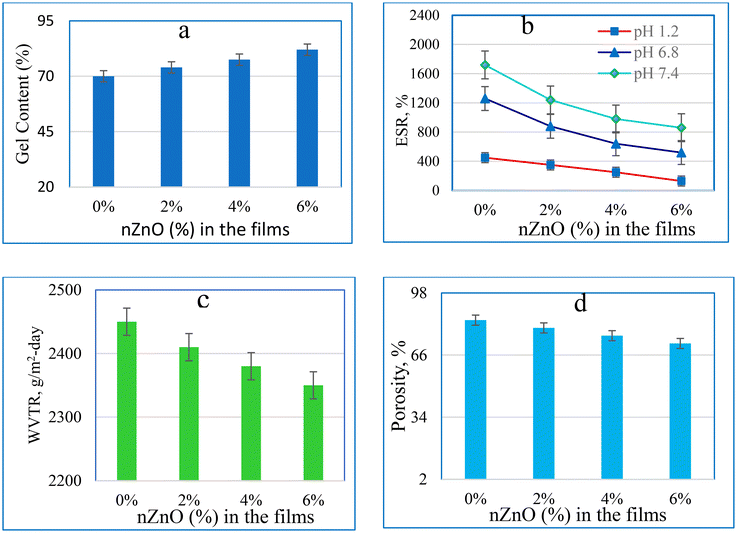 |
| Fig. 2 Effect of nZnOs on (a) the percentage of gel content, (b) percentage of ESR, (c) WVTR, and (d) percentage of porosity of the prepared CMC/PVP/nZnO nanocomposite hydrogels. | |
The swelling capacity of a hydrogel membrane plays a vital role in its antibacterial activity, wound healing capacity, and biomedical applications, because of water retention. The hydrogel membranes can absorb a significant quantity of wound exudates by swelling, resulting in more rapid wound healing.
The water uptake of the films was tested in three solutions, phosphate buffers of pH 7.4 and 6.8 and a simulated gastric fluid, i.e. a HCl buffer of pH 1.2. The purpose of these tests was to investigate the pH sensitivity of hydrogels.
Increased water intake was observed in all samples at higher pH levels, such as pH 7.4. At pH values higher than the pKa of carboxylic groups (pKa 4–5), the carboxylic acid groups become deprotonated, and increased electrostatic repulsion among the carboxylate ions expands the hydrogel matrix. This expansion allows more water to penetrate the hydrogel, which we see as enhanced swelling.59
Most carboxylate anions are protonated at low pH levels. The network decreases when the electrostatic repulsion between carboxylate groups is decreased, leading to lower water absorption levels. Furthermore, the reduced water uptake capacity of hydrogel films may be due to the availability of more hydrogen bonding connections among carboxylate groups, which implies greater physical crosslinking.60
As shown in Fig. 2b, the CMC/PVP film exhibits the greatest swelling, of 1720%, at pH 7.4. This capacity is assigned to the strong hydrogen bonding between the biopolymers of CMC and PVP. This interaction allows water absorption and swelling without dissolution. The swelling of the CMC/PVP film with nZnOs is thought to have been reduced because of the interaction between CMC/PVP and nZnOs. As a result, the films were more condensed and tighter. The second-greatest swelling capacity was 1240% for CMC/PVP/nZnOs (2%) at pH 7.4. The swelling capacity of the prepared nanocomposite hydrogels decreases with the increase in the concentrations of nZnOs and increases with the increase in pH values. The nZnOs have a propensity to form additional bonds with carboxyl groups, resulting in a reduction of free carboxyl groups and the binding of hydroxyl groups. The effect of this is to reduce the swelling of water molecules.61
3.3 Water vapour transmission rate and porosity test
High water vapour transmission rate (WVTR) causes the formation of scars, while low WVTR leads to the generation of exudates and increased bacterial growth. To prevent the wound from drying out and losing water, as well as the development of exudates in the wound bed that aid in healing, the ideal wound dressing should have a WVTR of 2000–2500 g m−2 day.62
The CMC/PVP composite hydrogel exhibited the highest WVTR value, specifically 2450 g m−2 day. However, the addition of 6% nZnOs led to a decrease in the WVTR value, reaching a minimum of 2350 g m−2 day. This reduction can be attributed to the occlusion of pores and an enhancement in the water barrier qualities,63 as shown in Fig. 2c.
Fig. 2d shows that the CMC/PVP film has 84% porosity of its total volume. The addition of nZnOs (2–6%) caused a reduction in the total porosity of the film, from 80% to 72%. This reduction is assigned to the interaction between CMC/PVP and nZnOs. Greater porosity would be helpful for the transfer of nutrients and oxygen to the interior of the bandages. Further, the porous nature would be helpful to absorb large amounts of exudates from the wound surface and reduce the infection due to such exudates.64
The addition of 0.4% nZnO increased pores and unoccupied volume. However, high nanofiller concentrations limit intermolecular movements and porosity at the knot-tying function, lowering the swelling ratio. Nanocomposites may have a lower swelling ratio due to the shielding effect of nZnOs reducing the matrix's net electrostatic charge, which reduces water affinity.1,65
The WVTR decreased with the increase in nZnO concentration due to the formation of hydrogen bonds among CMC, PVP, and nZnOs, which increased matrix compliance and decreased diffusion of water molecules into the films.61 and also the decrease was attributed to the occupied pore volume by nZnOs.1 With the increase in the nZnO content, there was a substantial enhancement in the film's ability to adhere to moisture. Increasing the nZnO content results in a stiffer pore structure in the film, making it more challenging for water to be released from these films.66 That is why, the inclusion of nZnO resulted in a reduction in porosity, WVTR, and ESR. Which are substantiated by the corresponding increase in gel content.
3.4 ATR-FTIR analysis
Fig. 3a displays the FT-IR spectra of WH cellulose, CMC, PVP, citric acid, CMC/PVP, and CMC/PVP/nZnO, covering the wavelength range of 400–4000 cm−1. Peaks between 3300 cm−1 and 3500 cm−1 wave numbers for three cellulose samples reveal the existence of hydroxyl groups (–OH), which imply hydrophilic properties and the ability of the hydrogel to absorb water. Peaks between 2800 cm−1 and 3000 cm−1 wave numbers for all samples indicate C–H stretching vibrations. Peaks at 1740 cm−1 were assigned to important components such as acetyl and carbonyl groups of lignin, 1606 cm−1 to the carbonyl group, 1430 cm−1 to the C
O stretching and aromatic skeletal vibrations of lignin, 1322 cm−1 to C–O stretching, and 1030 cm−1 to the C–O–C stretching vibration of cellulose. The absorption peaks appeared at 1416 cm−1, which showed the presence of –CH2– groups, and the regions of 1601 cm−1 indicate the presence of the carboxyl group (COO–) and more intense vibrations at 1060 cm−1 indicate the presence of ether linkage (C–O–C). It could be that water hyacinth, derived from cellulosic materials, underwent carboxymethylation to form CMC.
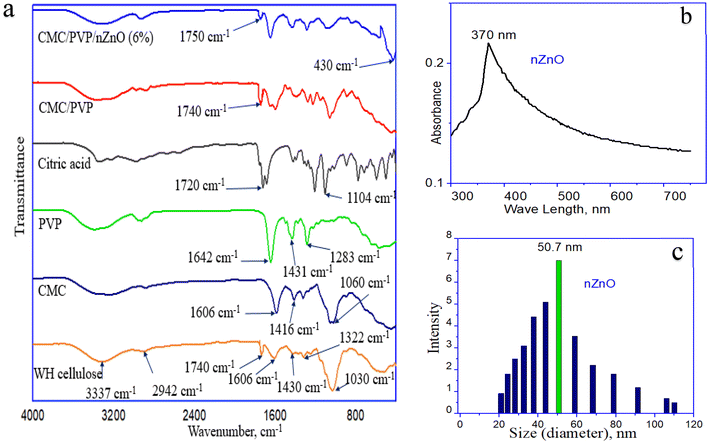 |
| Fig. 3 (a) FTIR spectra of WH cellulose, CMC, PVP, citric acid, CMC/PVP and CMC/PVP/nZnO (6%) nanocomposite hydrogels. (b) UV-visible spectrum of the bio-synthesised nZnOs using Azadirachta indica. (c) DLS of the bio-synthesised nZnOs. | |
Some characteristic peaks in the PVP spectrum could be assigned as follows: 1283 cm−1 for C–N stretching vibration, 1431 cm−1 for –CH2– wagging, and 1642 cm−1 for C
O stretching vibration. For citric acid, peaks at 1104 cm−1 were assigned to C–O stretching and 1720 cm−1 to C
O stretching.
For the CMC/PVP hydrogel, there was a new peak at 1740 cm−1, indicating ester linkage among CMC by CA, and 1283 cm−1 assigned to C–N stretching due to the crosslinking of PVP with CMC. Ester linkage also appears at 1750 cm−1 and a new peak is assigned to the presence of nZnO at 430 cm−1 in the developed CMC/PVP/nZnO nanocomposite hydrogels.31,33,67
3.5 UV-visible spectroscopic analysis and dynamic light scattering analysis
The best method to use for confirming nZnOs is UV-vis spectroscopy, which is depicted in Fig. 3b. Due to the surface plasmon resonance consequence, the strong absorption range in the order of 370 nm confirms the existence of nZnO hydrogel networks.64 This can be attributed to the intrinsic bandgap absorption of zinc oxide, which results from electron transitions from the valence band to the conduction band (O 2p–Zn 3d). The assessed value is lower than that of bulk zinc oxide, which is 380 nm, and there is a blue shift in excitonic absorption.68 DLS is a recently discovered and extensively utilised technique for determining the hydrodynamic diameter of nanoparticle suspensions by analysing the Brownian motion of the particles. The produced nZnOs exhibit stability, as depicted in Fig. 3c. The histogram analysis reveals that the particles exhibit a maximum volume fraction at a diameter of 50.7 nm. This is also confirmed by the UV-visible test of the prepared nanocomposite hydrogels.
3.6 X-ray diffraction
In Fig. 4a, X-ray diffraction (XRD) patterns of Water Hyacinth WH cellulose showed characteristic peaks at 2θ = 15.6°, 22.7° and 34.7°, which indicate the crystalline structure of water hyacinth cellulose after the removal of lignin and hemicellulose components from it by treatment with NaClO2 and 17.5% NaOH. CMC peaks at 22.6° with a lower intensity mean that there has been a decrease in crystallinity. With the increase in degree of substitution, the X-ray diffraction of CMC shows less crystallinity because of the cleavage of the hydrogen bonds among cellulose molecules as a result of the alkalisation of cellulose. Characteristic peaks were obtained at 2θ = 11.2° and 20.5° for PVP. The XRD pattern of hydrogel CMC/PVP loaded with nZnO (6%) showed coexistence of both peaks of nZnO and CMC/PVP. This confirms the presence of nZnO in the hydrogel membranes.31,33,69
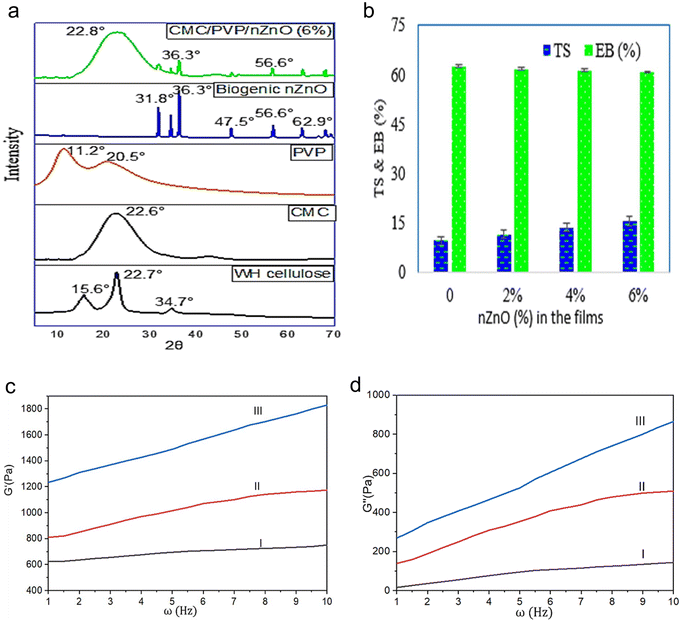 |
| Fig. 4 (a) XRD patterns of WH-cellulose, CMC, PVP, CMC/PVP hydrogel, and CMC/PVP/nZnO (6%) hydrogel; (b) tensile strength (TS) and elongation at break (EB%); and (c) storage moduli (G′) and (d) loss moduli (G′′) of i. CMC, ii. CMC/PVP and iii. CMC/PVP/nZnO (6%) nanocomposite hydrogels. | |
3.7 Mechanical and rheological behaviours
The mechanical properties of the CMC/PVP and CMC/PVP loaded with nZnO were recorded and explained as presented in Fig. 4b. All hydrogel membranes exhibited high elongations with a lower percentage of tensile strength. The tensile strengths of the membranes were in the range from 9.5 to 15.5 N mm−2 and elongations at the break between 62.6 and 60.9%. That is to say, the mechanical properties of the CMC/PVP/nZnO composite hydrogel did not change regularly with the content of nZnO.70
To elucidate the rheological properties of the CMC/PVP hydrogels, the storage modulus (G′) and loss modulus (G′′) of the synthesised hydrogels were evaluated, as illustrated in Fig. 4(c) and (d). The G′ curves of all synthesised hydrogels displayed a plateau-like behaviour, as demonstrated in Fig. 4(c), signifying the rubbery plateau region of the gel network. This phenomenon can be ascribed to the cross-linking structure formed by CMC, PVP, CA, and nZnOs. G′ of the CMC/PVP hydrogels exceeded G′′, indicating the presence of a gel network characterised by elastic properties rather than viscous behaviours. In contrast to G′′ at elevated frequencies, G′′ exhibits a reduced value at lower frequencies. Consequently, the CMC/PVP hydrogels are likely to possess adequate time for relaxation. The incorporation of PVP and nZnOs resulted in a progressive increase in the modulus of the hydrogels. Higher storage modulus indicates elevated elastic property, but among all the test samples, the value of storage modulus is highest for the CMC/PVP/nZnO (6%) hydrogel. The presence of an increased number of carboxyl and hydroxyl groups in CMC enhances the potential for hydrogen bond formation with PVP and nZnO. Furthermore, the increase in the degree of cross-linking serves to limit the mobility of macromolecular chains. Therefore, it can be inferred that the effects of storage modulus (G′) play a significant role in the flow behaviour of the hydrogels, indicating that the flow of the gels examined in this study is predominantly elastic rather than viscous.
Both the XRD and tensile strength test results confirm existence of the amorphous structure in the developed nanocomposites, as shown by XRD and moderate tensile strength tests, essential for their ability to adsorb water or biological fluids such as wound exudates.
3.8 Surface morphology analyses
Field emission scanning electron microscopy (FESEM) was performed to examine the shape and size of the prepared materials. The surface morphology of CMC, PVP and hydrogel, with and without nZnOs, was analysed by FESEM, as shown in Fig. 5a. CMC shows a crystalline and needle-like structure and PVP shows a globular structure. However, the CMC/PVP hydrogel shows a homogeneous blended structure and confirms successful crosslinking among the constituents of the nanocomposite hydrogels. The FESEM images of the cross-section of the CMC/PVP/nZnO composite film reveal that there are no voids in the nanocomposite films and that the zinc oxide nanofillers are distributed evenly in the polymer matrix without aggregation. This indicates that the fillers are consistent with the polymer matrix and have potentially good adhesion, intermolecular binding, and affinity. The hydrogel including nZnO exhibited a transformation in morphology, resulting in the formation of porous structures with different sizes and shapes. Additionally, the inclusion of nZnOs into the hydrogel revealed the presence of a crystalline, fibre-like morphology resembling CMC.
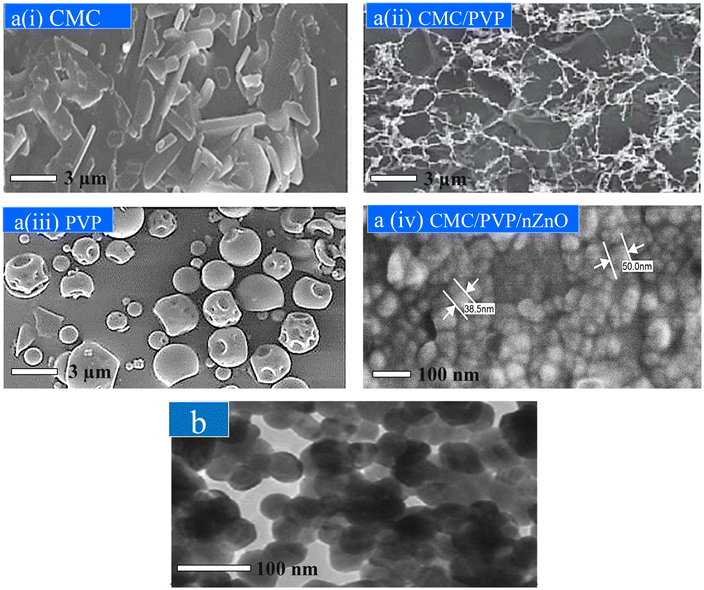 |
| Fig. 5 (a) FESEM images of CMC, PVP, CMC/PVP, and CMC/PVP/nZnO (6%). (b) TEM image of the bio-synthesised ZnO nanoparticles. | |
Fig. 5b illustrates the histogram of the average diameter distributions, with the Lorentz curve employed for fitting, alongside the morphology of nZnOs. The mean diameter was determined to be 56 ± 1 nm. The nZnOs demonstrated the presence of spherical, hexagonal, and cubic structures. The nanoparticles exhibited elongated crystals, revealing subtle interactions between zinc ions on the nZnO surface and phenolic groups (–OH), which led to the aggregation of the nanoparticles. Comparable aggregations have been documented in earlier research involving green nanoparticles.52
3.9 Antioxidant activity
2,2-Diphenyl-1-picrylhydrazyl (DPPH) is a simple and quick way to calculate the antioxidant activity. The colour changes from purple to yellow during reduction, as measured by the decreased absorbance at 517 nm. The DPPH free radical scavenging activities of the prepared samples were 35 ± 1%, 40.5 ± 2%, and 48.2 ± 1% for the CMC/PVP hydrogel loaded with 2%, 4% and 6% nZnO content composite hydrogel, respectively. Ascorbic acid was used as a control in this investigation and the results (in the same concentration as nZnOs) were 45.0 ± 2.0%, 50.5 ± 1%, and 60.4 ± 1.5%, which were higher. The presence of hydroxyl groups on the surface of the nZnOs contributed to their antioxidant activity. This is because of their ability to transport electrons from the oxygen atom to the odd electron of the nitrogen atom in DPPH.3 Therefore, the prepared nanocomposite biodegradable films had better antioxidant properties.
3.10 Antibacterial efficacy
The antibacterial efficacy of the CMC/PVP, CMC/PVP/nZnOs (2%, 4%, 6%), and antibiotic (positive control) formulations was assessed by testing them against two types of bacteria: Gram-negative Escherichia coli (E. coli) and Gram-positive Staphylococcus aureus (S. aureus). The results presented in Fig. 6a and b indicate that the zone of inhibition (ZoI) varied for different samples, against S. aureus and E. coli, after the incubation period. The ZoI values were observed to be 6 mm, 16 mm, 22 mm, 27 mm and 30 mm for S. aureus, and 6 mm, 13 mm, 15 mm, 20 mm, and 25 mm for E. coli. Therefore, it is concluded that the nanocomposite hydrogels containing nZnOs have antibacterial characteristics, as evidenced by the increased ZoI with higher concentrations of nZnOs. Zinc oxide nanoparticles induce oxidative stress by disrupting cellular membranes via a photo-catalytic process, resulting in the liberation of hydrogen peroxide (H2O2). The findings suggest that the hydrogels that were created exhibited greater efficacy against S. aureus compared to against E. coli. This disparity can be attributed to the fact that the thick peptidoglycan layer of S. aureus bacteria facilitates the penetration of antibacterial agents, whereas the thin peptidoglycan network of E. coli is shielded by its lipopolysaccharide (LPS) layer.3,64
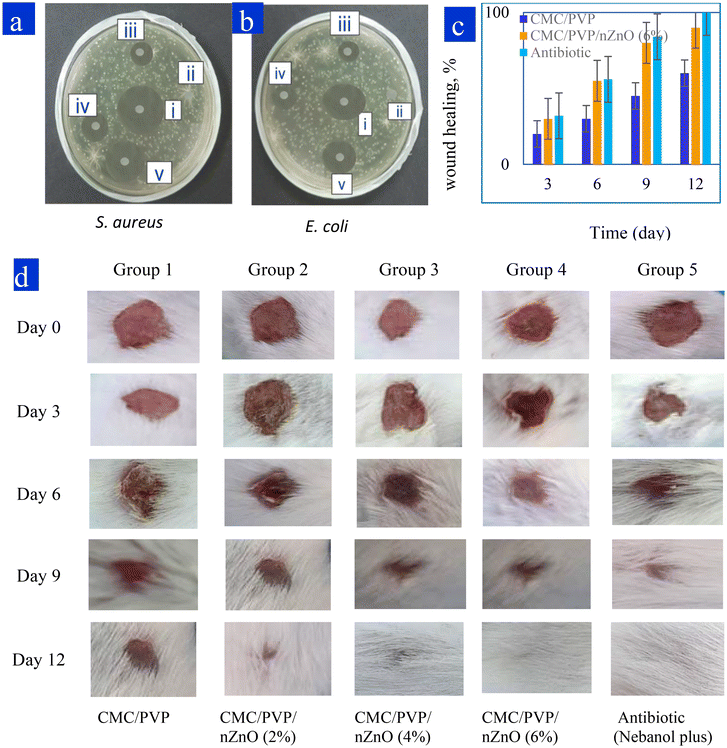 |
| Fig. 6 (a and b) ZoI against S. aureus and E. coli for i. antibiotic, ii. CMC/PVP, iii. CMC/PVP/nZnO (2%), iv. CMC/PVP/nZnO (4%), and v. CMC/PVP/nZnO (6%). (c) Wound healing percentage at different time periods for the prepared nanocomposite hydrogels and antibiotic. (d) Photographs of wounds treated with the CMC/PVP, CMC/PVP/nZnO (2%), CMC/PVP/nZnO (4%), and CMC/PVP/nZnO (6%) nanocomposite hydrogels at different time periods. | |
Out of all the prepared nanocomposite films, the CMC/PVP/nZnO (6%) film exhibits the best antibacterial efficacy. Therefore, this film has the best potential to be utilised as a common dressing for skin wounds.
3.11
In vivo wound healing study
Fig. 6c and d show the results of in vivo wound healing in albino mice. The extent of wound healing was significantly higher on day 3, day 6, day 9 and day 12 in the CMC/PVP/nZnO (6%) nanocomposite hydrogel and Nebanol-antibiotic-treated wounds, compared with those treated with CMC/PVP, CMC/PVP/nZnO (2%) and the CMC/PVP/nZnO (4%). The results indicate that the prepared hydrogels with nZnOs accelerated wound healing. The CMC/PVP showed 20%, 30%, 45%, and 60% wound healing on days 3, 6, 9, and 12, respectively, while the CMC/PVP/nZnO (6%) composite hydrogel showed 30%, 55%, 80% and 97% wound healing for the same time periods. However, Nebanol antibiotic showed 32%, 56%, 84% and 100% wound healing for the same respective time periods, as shown in Fig. 6c and d. Zinc oxide nanoparticles have better ability to heal wounds because they have antibacterial features, no effect on normal cells and the zinc ions which they release can promote keratinocyte migration to the wound site, which enhances wound healing.71
The wounds addressed with nZnO hydrogels exhibited a continuous epidermis, and the regenerated dermis demonstrated a notable increase in thickness compared to the control group. A significant quantity of epidermal appendages was observed in the regenerated skin that had been treated with the nZnO-containing hydrogel. On day 12, the wounds in the control group exhibited partial coverage with the epidermis, whereas those in the hydrogel and nZnO-incorporated hydrogel groups demonstrated complete coverage with the epidermis. nZnOs demonstrate an exceptional capacity for wound healing attributed to their antibacterial properties. Interestingly, the nZnO does not impact normal cells, and the zinc ions it generates can promote keratinocyte migration to the wound area, thereby enhancing the wound healing process.72
CMC is a cellulose derivative that is soluble in water and exhibits low crystallinity, undergoing slow degradation in vivo via biological, chemical, and physical processes. CMC-based films maintained a moist environment for wounds, promoted the growth of granulation tissue, and reduced the formation of scars. The films consist of polysaccharide chains. These chains enhance wound healing by maintaining a moist environment around the wound and promoting tissue granulation, ultimately facilitating hemostasis.73 Ion exchange could enable CMC films to take in wound fluids. This absorption can promote the production of granulation tissue, accelerate epithelialization, and facilitate healing. The mechanisms involved facilitate a more rapid healing of wounds. The accelerated wound healing observed with the films can be linked to the degradation of polymers at the wound site, potentially stimulating the aggregation of inflammatory cells and enhancing the migration of epithelial, vascular endothelial, and fibroblast cells.
The results indicated that the rate and extent of wound healing were significantly enhanced in the group treated with CMC films compared to the control group. The wounds treated with CMC/PVP/nZnO (6%) exhibited rapid healing via re-epithelialization, as illustrated in Fig. 7, with minimal scarring observed. nZnO, derived from the synthesised hydrogels, plays a crucial role in the formation of novel matrices in wound healing.73
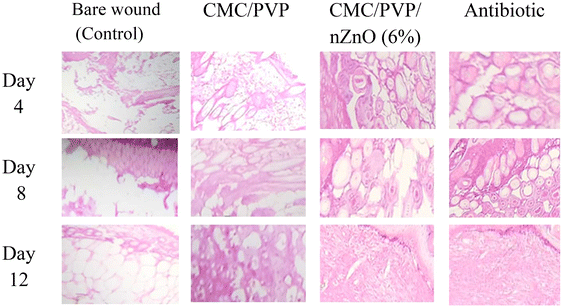 |
| Fig. 7 H&E staining of the wound sections collected on days 4, 8 and 12 in an albino mouse model. The control group comprises mice with untreated bare wounds, and the experimental group comprises mice treated with two different films (CMC/PVP), CMC/PVP/nZnO (6%), and the antibiotic. | |
3.12 Effect of zinc oxide nanoparticles on cytotoxicity and blood compatibility test
The cytotoxicity of the biosynthesised zinc oxide nanoparticle-incorporated nanocomposite against Vero cells was tested in vitro. Fig. 8a displays the cell viability results after 72 h for the hydrogel film containing 6% nZnOs, which was found to be 94.07 ± 0.681. In contrast, the control group, consisting of dimethyl sulfoxide (DMSO), had a cell viability of 96.97 ± 0.737, as shown in Fig. 8b. In previous studies, the nZnOs, synthesised using chemical methods, exhibited detrimental effects on cells and living tissues. These effects were attributed to an elevation in the formation of reactive oxygen species (ROS) and the induction of genotoxicity.3,74 The green-synthesised nZnOs used in this study, however, had no significant toxicity against Vero cells. It is conceivable that the chemicals that adhere to the surface of nZnOs during chemical synthesis procedures cause toxicity in living cells in some cases. However, in green synthesis methods, these toxic materials are replaced by non-toxic phytochemicals, as capping and reducing agents, making green-synthesised nZnOs less harmful. Therefore, cell viability was not significantly changed as compared to the control (dimethyl sulfoxide solution) at the tested concentration.
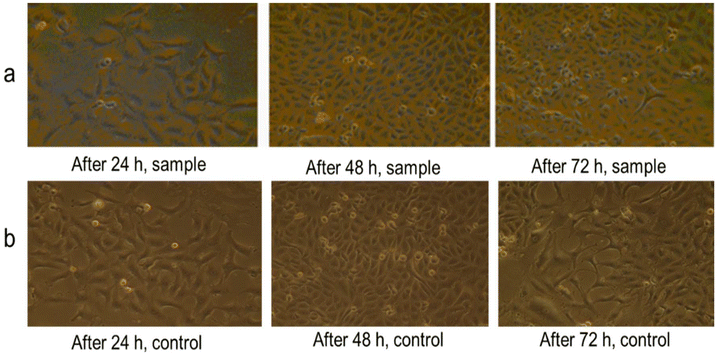 |
| Fig. 8 (a) Effect of zinc oxide nanoparticles on cell viability against Vero cells. (b) Effect of DMSO on cell viability against Vero cells. | |
Hemolytic activity increases with the increase in nZnO composition in CMC/PVP films. It was 1.1%, 1.74%, 2.26% and 2.76% for CMC/PVP (control), CMC/PVP/nZnO (2%), CMC/PVP/nZnO (4%), and CMC/PVP/nZnO (6%). According to the American Society for Testing and Materials, it is of minor hemolytic properties. The results indicate that the developed bio-composites can be considered as non-hemolytic materials and can be used frequently for skin wound healing application.70
4 Conclusion
The gel strength of the nanocomposite hydrogels was enhanced by fabricating them with CMC-embedded zinc oxide nanocomposites and PVP, together with nontoxic citric acid as a crosslinking agent for CMC. UV-vis spectroscopy, FTIR spectroscopy, TGA, XRD, FESEM, TEM, and other techniques were used to characterise the hydrogels. The swelling was maximum for CMC/PVP gels at pH 7.4, but it decreased with the increase in the percentage of nZnO in the CMC/PVP polymer matrix. nZnO aided microbial penetration in CMC/PVP gels, and the preparations demonstrated antibacterial activity against Gram-negative (E. coli) and Gram-positive (S. aureus) bacteria. However, the CMC/PVP/nZnO nanocomposite hydrogels were more effective against Gram-positive S. aureus than Gram-negative E. coli. The best antioxidant activity was found in nanocomposite hydrogels with 6% nZnOs. Using albino mice as test animals, skin wounds treated with the CMC/PVP/nZnO (6%) nanocomposite hydrogel healed more quickly than wounds treated with CMC/PVP hydrogel. Moreover, the results of the histological analysis confirmed the findings of faster tissue regeneration, together with a reduction in inflammatory cells and an improvement in angiogenesis in the surrounding skin. They are pliable, partially translucent, provide a chilly sensation, are easily removable, and allow for observing the healing process. The results validate and demonstrate the wide range of possible uses of novel CMC/PVP/nZnO (6%) nanocomposite hydrogels in the biomedical sciences, particularly in promising wound healing applications.
Ethical statement
The study was performed according to the institutional guidelines of the Animal, Medical Ethics, Biosafety and Biosecurity Committee (IAMEBBC) of the Institute of Biological Science, Rajshahi University, Bangladesh, Approval no. (Memo no. 455(13/320/IAMEBBC/IBSc)).
Author contributions
Md. Ibrahim H. Mondal: conceptualization, supervision, funding acquisition, formal analysis, writing – reviewing and editing. Md. Monirul Islam: investigation, methodology, formal analysis, visualization, writing – original draft. Firoz Ahmed: investigation, formal analysis, visualization, writing – reviewing and editing.
Data availability
All data and materials are included within the manuscript.
Conflicts of interest
The author declares no conflicts of interest.
References
- P. Pino, F. Bosco, C. Mollea and B. Onida, Antimicrobial nano-zinc oxide biocomposites for wound healing applications: A Review, Pharmaceutics, 2023, 15(3), 970, DOI:10.3390/pharmaceutics15030970.
- Z. Abdollahi, E. N. Zare, F. Salimi, I. Goudarzi, F. R. Tay and P. Makvandi, Bioactive carboxymethyl starch-based hydrogels decorated with CuO nanoparticles: antioxidant and antimicrobial properties and accelerated wound healing in vivo, Int. J. Mol. Sci., 2021, 22(5), 2531, DOI:10.3390/ijms22052531.
-
M. I. H. Mondal and M. O. Haque, Cellulosic Hydrogels: A Greener Solution of Sustainability, in Cellulose-Based Superabsorbent Hydrogels, ed. M. I. H. Mondal, Springer Nature, Switzerland, 2019. DOI:10.1007/978-3-319-77830-3_4.
- M. I. H. Mondal, M. O. Haque, F. Ahmed, M. N. Pervez, V. Naddeo and M. B. Ahmed, Super-Adsorptive biodegradable hydrogel from simply treated sugarcane bagasse, Gels, 2022, 8(3), 177, DOI:10.3390/gels8030177.
- Q. Chai, Y. Jiao and X. Yu, Hydrogels for Biomedical Applications: Their Characteristics and the Mechanisms behind Them, Gels, 2017, 3(1), 6, DOI:10.3390/gels3010006.
- M. N. Alam, M. S. Islam and L. P. Christopher, Sustainable Production of Cellulose-Based Hydrogels with Superb Absorbing Potential in Physiological Saline, ACS Omega, 2019, 4(5), 9419–9426, DOI:10.1021/acsomega.9b00651.
- F. Esposito, M. A. Del Nobile, G. Mensitieri and L. Nicolais, Water sorption in cellulose-based hydrogels, J. Appl. Polym. Sci., 1996, 60(13), 2403–2407, DOI:10.1002/(sici)1097-4628(19960627)60:13<2403::aid-app12>3.0.co;2-5.
- A. Sannino, C. Demitri and M. Madaghiele, Biodegradable Cellulose-based Hydrogels: Design and Applications, Materials, 2009, 2(2), 353–373, DOI:10.3390/ma2020353.
- M. Qamruzzaman, F. Ahmed and M. I. H. Mondal, An overview on starch-based sustainable hydrogels: Potential applications and aspects, J. Polym. Environ., 2022, 30(1), 19–50, DOI:10.1007/s10924-021-02180-9.
- L. Li, Y. Wang, L. Pan, Y. Shi, W. Cheng, Y. Shi and G. Yu, A Nanostructured Conductive Hydrogels-Based Biosensor Platform for Human Metabolite Detection, Nano Lett., 2015, 15(2), 1146–1151, DOI:10.3390/ma2020353.1021/nl504217p.
- P. Chen, W. Zhang, W. Luo and Y. Fang, Synthesis of superabsorbent polymers by irradiation and their applications in agriculture, J. Appl. Polym. Sci., 2004, 93(4), 1748–1755, DOI:10.3390/ma2020353.1002/app.20612.
- C. Chang, B. Duan, J. Cai and L. Zhang, Superabsorbent hydrogels based on cellulose for smart swelling and controllable delivery, Eur. Polym. J., 2010, 46(1), 92–100, DOI:10.3390/ma2020353.1016/j.eurpolymj.2009.04.033.
- J. Shang, Z. Shao and X. Chen, Chitosan-based electroactive hydrogel, Polymer, 2008, 49(25), 5520–5525, DOI:10.3390/ma2020353.1016/j.polymer.2008.09.067.
- L. Setyaningsih, E. Satria, H. Khoironi, M. Dwisari, G. Setyowati, N. Rachmawati, R. Kusuma and J. Anggraeni, Cellulose extracted from water hyacinth and the application in hydrogel, IOP Conf. Ser.: Mater. Sci. Eng., 2019, 673, 012017, DOI:10.3390/ma2020353.1088/1757-899x/673/1/012017.
- I. Gholamali and M. Yadollahi, Doxorubicin-loaded carboxymethyl cellulose/Starch/ZnO nanocomposite hydrogel beads as an anticancer drug carrier agent, Int. J. Biol. Macromol., 2020, 160, 724–735, DOI:10.1016/j.ijbiomac.2020.05.232.
- M. Naseri-Nosar and Z. M. Ziora, Wound dressings from naturally-occurring polymers: A review on homopolysaccharide-based composites, Carbohydr. Polym., 2018, 189, 379–398, DOI:10.1016/j.carbpol.2018.02.003.
- Y. Zhong, J. Wang, Z. Yuan, Y. Wang, Z. Xi, L. Li, Z. Liu and X. Guo, A mussel-inspired carboxymethyl cellulose hydrogel with enhanced adhesiveness through enzymatic crosslinking, Colloids Surf., B, 2019, 179, 462–469, DOI:10.1016/j.colsurfb.2019.03.044.
- M. I. H. Mondal and M. S. Yeasmin, Toxicity study of food-grade carboxymethyl cellulose synthesized from maize husk in Swiss albino mice, Int. J. Biol. Macromol., 2016, 92, 965–971, DOI:10.1016/j.ijbiomac.2016.08.012.
- A. H. Saputra and N. H. Apriliana, Polyvinyl Alcohol (PVA) Partially Hydrolyzed Addition in Synthesis of Natural Hydrogel Carboxymethyl Cellulose (CMC) Based from Water Hyacinth, MATEC Web Conf., 2018, 156, 01007, DOI:10.1051/matecconf/201815601007.
- N. Saha, R. Shah, P. Gupta, B. B. Mandal, R. Alexandrova, M. D. Sikiric and P. Saha, PVP-CMC hydrogel: An excellent bioinspired and biocompatible scaffold for osseointegration, Mater. Sci. Eng., C, 2018, 95, 440–449, DOI:10.1016/j.msec.2018.04.050.
- M. Teodorescu and M. Bercea, Poly(vinylpyrrolidone) – A Versatile Polymer for Biomedical and Beyond Medical Applications, Polym.-Plast. Technol. Eng., 2015, 54(9), 923–943, DOI:10.1080/03602559.2014.979506.
- M. Teodorescu, M. Bercea and S. Morariu, Biomaterials of PVA and PVP in medical and pharmaceutical applications: Perspectives and challenges, Biotechnol. Adv., 2018, 37(1), 109–131, DOI:10.1016/j.biotechadv.2018.11.008.
- I. Giardino, M. D′apolito, M. Brownlee, A. B. Maffione, A. L. Colia, M. Sacco, P. Ferrara and M. Pettoello-Mantovani, Vascular toxicity of urea, a new “old player” in the pathogenesis of chronic renal failure induced cardiovascular diseases, Turk Arch. Pediatr., 2017, 52(4), 187, DOI:10.5152/TurkPediatriArs.2017.6314.
- R. Salihu, S. I. A. Razak, N. A. Zawawi, M. R. A. Kadir, N. I. Ismail, N. Jusoh, M. R. Mohamad and N. H. M. Nayan, Citric acid: A green cross-linker of biomaterials for biomedical applications, Eur. Polym. J., 2021, 146, 110271, DOI:10.1016/j.eurpolymj.2021.110271.
- C. Chang and L. Zhang, Cellulose-based hydrogels: Present status and application prospects, Carbohydr. Polym., 2011, 84(1), 40–53, DOI:10.1016/j.carbpol.2010.12.023.
- K. Dharmalingam and R. Anandalakshmi, Fabrication, characterization and drug loading efficiency of citric acid crosslinked NaCMC-HPMC hydrogel films for wound healing drug delivery applications, Int. J. Biol. Macromol., 2029, 134, 815–829, DOI:10.1016/j.ijbiomac.2019.05.027.
- H. Chopra, S. Bibi, S. Kumar, M. S. Khan, P. Kumar and I. Singh, Preparation and evaluation of chitosan/PVA based hydrogel films loaded with honey for wound healing application, Gels, 2022, 8(2), 111, DOI:10.3390/gels8020111.
- M. Wang, L. Xu, H. Hu, M. Zhai, J. Peng, Y. Nho, J. Lia and G. Wei, Radiation synthesis of PVP/CMC hydrogels as wound dressing, Nucl. Instrum. Methods Phys. Res., Sect. B, 2007, 265(1), 385–389, DOI:10.1016/j.nimb.2007.09.009.
- N. Saha, A. Saarai, N. Roy, T. Kitano and P. Saha, Polymeric Biomaterial Based Hydrogels for Biomedical Applications, J. Biomater. Nanobiotechnol., 2011, 02(01), 85–90, DOI:10.4236/jbnb.2011.21011.
- T. Ji, R. Zhang, X. Dong, D. E. Sameen, S. Ahmed, S. Li and Y. Liu, Effects of ultrasonication time on the properties of polyvinyl alcohol/sodium carboxymethyl cellulose/nano-ZnO/multilayer graphene nanoplatelet composite films, Nanomaterials, 2020, 10(9), 1797, DOI:10.3390/nano10091797.
- N. S. Alghunaim, Effect of CuO nanofiller on the spectroscopic properties, dielectric permittivity and dielectric modulus of CMC/PVP nanocomposites, J. Mater. Res. Technol., 2019, 8(4), 3596–3602, DOI:10.1016/j.jmrt.2019.05.022.
- M. A. Morsi, A. Rajeh and A. A. Menazea, Nanosecond laser-irradiation assisted the improvement of structural, optical and thermal properties of polyvinyl pyrrolidone/carboxymethyl cellulose blend filled with gold nanoparticles, J. Mater. Sci.: Mater.
Electron., 2018, 30, 2693–2705, DOI:10.1007/s10854-018-0545-4.
- P. Ramadoss, T. Regi, M. I. Rahman and D. Arivuoli, Low–cost and biodegradable cellulose/PVP/activated carbon composite membrane for brackish water treatment, J. Appl. Polym. Sci., 2019, 137(22), 48746, DOI:10.1002/app.48746.
- O. Z. Higa, S. O. Rogero, L. D. B. Machado, M. B. Mathor and A. B. Lugão, Biocompatibility study for PVP wound dressing obtained in different conditions, Radiat. Phys. Chem., 1999, 55(5–6), 705–707, DOI:10.1016/s0969-806x(99)00215-7.
- R. K. Dutta, B. P. Nenavathu, M. K. Gangishetty and A. V. R. Reddy, Studies on antibacterial activity of ZnO nanoparticles by ROS induced lipid peroxidation, Colloids Surf., B, 2012, 94, 143–150, DOI:10.1016/j.colsurfb.2012.01.046.
- R. Rakhshaei and H. Namazi, A potential bioactive wound dressing based on carboxymethyl cellulose/ZnO impregnated MCM-41 nanocomposite hydrogel, Mater. Sci. Eng., C, 2017, 73, 456–464, DOI:10.1016/j.msec.2016.12.097.
-
M. I. H. Mondal, F. Ahmed, M. M. Islam, M. N. Pervez and J. Saha, 11-Metal and metal oxides nanoparticles in healthcare and medical textiles, in Medical Textiles from Natural Resources, ed. M. I. H. Mondal, Woodhead Publishing, UK, 2022, pp. 341–371. DOI:10.1016/B978-0-323-90479-7.00010-5.
- M. S. Yeasmin and M. I. H. Mondal, Synthesis of highly substituted carboxymethyl cellulose depending on cellulose particle size, Int. J. Biol. Macromol., 2015, 80, 725–731, DOI:10.1016/j.ijbiomac.2015.07.040.
- S. Ramamoorthy, S. Surendhiran, D. S. Kumar, G. Murugesan, M. Kalaiselvi, S. Kavisree, S. Muthulingam and S. Murugesan, Evaluation of photocatalytic and corrosion properties of green synthesized zinc oxide nanoparticles, J. Mater. Sci.: Mater. Electron., 2022, 33(12), 9722–9731, DOI:10.1007/s10854-022-07776-y.
- D. T. Handago, E. A. Zereffa and B. A. Gonfa, Effects of Azadirachta Indica Leaf Extract, Capping Agents, on the Synthesis of Pure and Cu Doped ZnO-Nanoparticles: A Green Approach and Microbial Activity, Open Chem., 2019, 17(1), 246–253, DOI:10.1515/chem-2019-0018.
- L. Bonetti, A. Fiorati, A. D'Agostino, C. M. Pelacani, R. Chiesa, S. Fare and L. De Nardo, Smart methylcellulose hydrogels for pH-triggered delivery of silver nanoparticles, Gels, 2022, 8, 298, DOI:10.3390/gels8050298.
- N. Roy, N. Saha, T. Kitano and P. Saha, Biodegradation of PVP–CMC hydrogel film: A useful food packaging material, Carbohydr. Polym., 2012, 89(2), 346–353, DOI:10.1016/j.carbpol.2012.03.008.
- M. I. H. Mondal and M. S. Yeasmin, Toxicity study of food-grade carboxymethyl cellulose synthesized from maize husk in Swiss albino mice, Int. J. Biol. Macromol., 2016, 92, 965–971, DOI:10.1016/j.ijbiomac.2016.08.012.
- C. Xin, L. Nie, H. Chen, J. Li and B. Li, Effect of degree of substitution of carboxymethyl cellulose sodium on the state of water, rheological and baking performance of frozen bread dough, Food Hydrocolloids, 2018, 80, 8–14, DOI:10.1016/j.foodhyd.2018.01.030.
-
ASTM, Tentative methods of testing sodium carboxymethyl cellulose, ASTM: D1439-61T, 1961, pp. 1164–1173 Search PubMed.
- B. K. Barai, R. S. Singhal and P. R. Kulkarni, Optimization of a process for preparing carboxymethyl cellulose from water hyacinth (Eichornia crassipes), Carbohydr. Polym., 1999, 32(3–4), 229–231, DOI:10.1016/s0144-8617(96)00166-x.
- M. T. Khorasani, A. Joorabloo, A. Moghaddam, H. Shamsi and Z. MansooriMoghadam, Incorporation of ZnO nanoparticles into heparinised polyvinyl alcohol/chitosan hydrogels for wound dressing application, Int. J. Biol. Macromol., 2018, 114, 1203–1215, DOI:10.1016/j.ijbiomac.2018.04.010.
- F. Altaf, M. B. K. Niazi, Z. Jahan, T. Ahmad, M. A. Akram, A. Safdar, M. S. Butt, T. Noor and F. Sher, Synthesis and characterization of PVA/starch hydrogel membranes incorporating essential oils aimed to be used in wound dressing applications, J. Polym. Environ., 2021, 29, 156–174, DOI:10.1007/s10924-020-01866-w.
- H. S. El-Sayed, S. El-Sayed and A. M. Youssef, Novel approach for biosynthesizing of zinc oxide nanoparticles using Lactobacillus gasseri and their influence on microbiological, chemical, sensory properties of integrated yogurt, Food Chem., 2021, 365, 130513, DOI:10.1016/j.foodchem.2021.130513.
- S. Roy and J. W. Rhim, Carboxymethyl cellulose-based antioxidant and antimicrobial active packaging film incorporated with curcumin and zinc oxide, Int. J. Biol. Macromol., 2020, 148, 666–676, DOI:10.1016/j.ijbiomac.2020.01.204.
- R. R. Vildanova, N. N. Sigaeva, O. S. Kukovinets and S. V. Kolesov, Preparation and rheological properties of hydrogels based on N-succinyl chitosan and hyaluronic acid dialdehyde, Polym. Test., 2021, 96, 107120, DOI:10.1016/j.polymertesting.2021.107120.
- J. A. A. Abdullah, M. Jiménez-Rosado, A. Guerrero and A. Romero, Biopolymer-based films reinforced with green synthesized zinc oxide nanoparticles, Polymers, 2022, 14(23), 5202, DOI:10.3390/polym14235202.
- J. Saha, M. I. H. Mondal, F. Ahmed and M. Rahman, Extraction, characterization and functionality assessment of Aloe vera, chitosan and silk sericin, Arab, J. Chem., 2023, 16(9), 105087, DOI:10.1016/j.arabjc.2023.105087.
- S. N. Hosseini, S. Pirsa and J. Farzi, Biodegradable nano composite film based on modified starch-albumin/MgO; antibacterial, antioxidant and structural properties, Polym. Test., 2021, 97, 107182, DOI:10.1016/j.polymertesting.2021.107182.
- ARRIVE guidelines, https://nc3rs.org.uk/arrive-guidelines, 2023. Accessed on 16 September 2023.
- S. Taokaew, S. Seetabhawang, P. Siripong and M. Phisalaphong, Biosynthesis and Characterization of Nanocellulose-Gelatin Films, Materials, 2013, 6(3), 782–794, DOI:10.3390/ma6030782.
- Z. Lu, J. Gao, Q. He, J. Wu, D. Liang, H. Yang and R. Chen, Enhanced antibacterial and wound healing activities of microporous chitosan-Ag/ZnO composite dressing, Carbohydr. Polym., 2017, 156, 460–469, DOI:10.1016/j.carbpol.2016.09.051.
- I. Gholamali, M. Asnaashariisfahani and E. Alipour, Silver nanoparticles incorporated in pH-sensitive nanocomposite hydrogels based on carboxymethyl chitosan-poly (vinyl alcohol) for use in a drug delivery system, Regener. Eng. Transl. Med., 2020, 6, 138–153, DOI:10.1007/s40883-019-00120-7.
- A. Pourjavadi, S. Barzegar and G. R. Mahdavinia, MBAcrosslinked Na-Alg/CMC as a smart full-polysaccharide superabsorbent hydrogels, Carbohydr. Polym., 2006, 66(3), 386–395, DOI:10.1016/j.carbpol.2006.03.013.
- Y. Bao, J. Ma and N. Li, Synthesis and swelling behaviors of sodium carboxymethyl cellulose-g-poly(AA-co-AM-co-AMPS)/MMT superabsorbent hydrogel, Carbohydr. Polym., 2021, 84(1), 76–82, DOI:10.1016/j.carbpol.2010.10.061.
- H. Helmiyati, Z. S. Z. Hidayat, I. F. R. Sitanggang and D. Liftyawati, Antimicrobial packaging of ZnO–Nps infused into CMC–PVA nanocomposite films effectively enhances the physicochemical properties, Polym. Test., 2021, 104, 107412, DOI:10.1016/j.polymertesting.2021.107412.
- R. Jangde, S. Srivastava, M. R. Singh and D. Singh, In vitro and In vivo characterization of quercetin loaded multiphase hydrogel for wound healing application, Int. J. Biol. Macromol., 2018, 115, 1211–1217, DOI:10.1016/j.ijbiomac.2018.05.010.
- S. Chowdhury, Y. L. Teoh, K. M. Ong, N. S. R. Zaidi and S. K. Mah, Poly (vinyl) alcohol crosslinked composite packaging film containing gold nanoparticles on shelf life extension of banana, Food Packag, Shelf Life, 2020, 24, 100463, DOI:10.1016/j.fpsl.2020.100463.
- H. Kumar, A. K. Gehlaut, A. Gaur, J.-W. Park and S. Maken, Development of zinc-loaded nanoparticle hydrogel made from sugarcane bagasse for special medical application, J. Mater. Cycles Waste Manage., 2020, 22, 1723–1733, DOI:10.1007/s10163-020-01054-x.
- A. Tanwar, P. Date and D. Ottoor, ZnO NPs incorporated gelatin grafted polyacrylamide hydrogel nanocomposite for controlled release of ciprofloxacin, Colloid Interface Sci. Commun., 2021, 2, 100413, DOI:10.1016/j.colcom.2021.100413.
- A. I. Raafat, N. M. El-Sawy, N. A. Badawy, E. A. Mousa and A. M. Mohamed, Radiation fabrication of Xanthan-based wound dressing hydrogels embedded ZnO nanoparticles: In vitro evaluation, Int. J. Biol. Macromol., 2018, 118, 1892–1902, DOI:10.1016/j.ijbiomac.2018.07.031.
- M. I. H. Mondal, M. S. Yeasmin and M. S. Rahman, Preparation of food grade carboxymethyl cellulose from corn husk agrowaste, Int. J. Biol. Macromol., 2015, 79, 144–150, DOI:10.1016/j.ijbiomac.2015.04.061.
- P. Purkait, A. Bhattacharyya, S. Roy, S. Maitra, G. C. Das and M. G. Chaudhuri, Green synthesis of ZnO nanoparticle using Trema orientalis (L) leaf: an efficient photocatalyst for degradation of zoxamide fungicide in aqueous organic media under UV light irradiation, Int. J. Environ. Anal. Chem., 2023, 103(2), 1–19, DOI:10.1080/03067319.2020.1857750.
- M. S. Rahman, M. I. H. Mondal, M. S. Yeasmin, M. A. Sayeed, M. A. Hossain and M. B. Ahmed, Conversion of lignocellulosic corn agro-waste into cellulose derivative and its potential application as pharmaceutical excipient, Processes, 2020, 8(6), 711, DOI:10.3390/pr8060711.
- G. F. El Fawal, M. M. Abu-Serie, M. A. Hassan and M. S. Elnouby, Hydroxyethyl cellulose hydrogel for wound dressing: Fabrication, characterization and in vitro evaluation, Int. J. Biol. Macromol., 2018, 111, 649–659, DOI:10.1016/j.ijbiomac.2018.01.040.
- P. T. S. Kumar, V. K. Lakshmanan, T. V. Anilkumar, C. Ramya, P. Reshmi, A. G. Unnikrishnan, S. V. Nair and R. Jayakumar, Flexible and microporous chitosan hydrogel/nano ZnO composite bandages for wound dressing: in vitro and in vivo evaluation, ACS Appl. Mater. Interfaces, 2012, 4(5), 2618–2629, DOI:10.1021/am300292v.
- P. T. Sudheesh Kumar, V. K. Lakshmanan, T. V. Anilkumar, C. Ramya, P. Reshmi, A. G. Unnikrishnan, S. V. Nair and R. Jayakumar, Flexible and microporous chitosan hydrogel/nano ZnO composite bandages for wound dressing: in vitro and in vivo evaluation, ACS Appl. Mater. Interfaces, 2012, 4(5), 2618–2629, DOI:10.1021/am300292v.
- P. Basu, U. Narendrakumar, R. Arunachalam, S. Devi and I. Manjubala, Characterization and Evaluation of Carboxymethyl Cellulose-Based Films for Healing of Full-Thickness Wounds in Normal and Diabetic Rats, ACS Omega, 2018, 3(10), 12622–12632, DOI:10.1021/acsomega.8b02015.
- P. C. Nagajyothi, S. J. Cha, I. J. Yang, T. V. M. Sreekanth, K. J. Kim and H. M. Shin, Antioxidant and anti-inflammatory activities of zinc oxide nanoparticles synthesized using Polygala tenuifolia root extract, J. Photochem. Photobiol., B, 2015, 146, 10–17, DOI:10.1016/j.jphotobiol.2015.02.008.
|
This journal is © The Royal Society of Chemistry 2025 |
Click here to see how this site uses Cookies. View our privacy policy here.