DOI:
10.1039/D4BM01394H
(Review Article)
Biomater. Sci., 2025,
13, 1161-1178
Extracellular vesicles as drug and gene delivery vehicles in central nervous system diseases
Received
20th October 2024
, Accepted 8th January 2025
First published on 28th January 2025
Abstract
Extracellular vesicles (EVs) are secreted by almost all cell types and contain DNA, RNA, proteins, lipids and other metabolites. EVs were initially believed to be cellular waste but now recognized for their role in cell-to-cell communication. Later, EVs from immune cells were discovered to function similarly to their parent cells, paving the way for their use as gene and drug carriers. EVs from different cell types or biological fluids carry distinct cargo depending on their origin, and they perform diverse functions. For instance, EVs derived from stem cells possess pluripotent properties, reflecting the cargo from their parent cells. Over the past two decades, substantial preclinical and clinical research has explored EVs-mediated drug and gene delivery to various organs, including the brain. Natural or intrinsic EVs may be effective for certain applications, but as drug or gene carriers, they demonstrate broader and more efficient potential across various diseases. Here, we review research on using EVs to treat central nervous system (CNS) diseases, such as Alzheimer's Disease, Parkinson diseases, depression, anxiety, dementia, and acute ischemic strokes. We first reviewed the naïve EVs, especially mesenchymal stem cell (MSC) derived EVs in CNS diseases and summarized the clinical trials of EVs in treating CNS diseases and highlighted the reports of two complete trials. Then, we overviewed the preclinical research of EVs as drug and gene delivery vehicles in CNS disease models, including the most recent two years’ progress and discussed the mechanisms and new methods of engineered EVs for targeting CNS. Finally, we discussed challenges and future directions and of EVs as personalized medicine for CNS diseases.
1. Introduction
Extracellular vesicles were traditionally called exosomes. Exosomes are the smallest extracellular vesicles, ranging in size from 40 nm to 100 nm.1,2 Almost all cells secrete exosomes.3,4 Researchers first observed exosomes in plasma six decades ago but initially thought they were ‘platelet dust’. It wasn't until four decades ago that exosomes were rediscovered as intercellular communication vesicles for both short and long distances.5 For example, exosomes derived from immune cells, such as B lymphocytes or dendritic cells, showed antigen-presentation or tumor eradication abilities, respectively.6,7 In addition, exosomes have been implicated in various aspects of nervous system development and function, particularly their ability to cross the blood–brain barrier (BBB).8 Exosomes have been found to help communication between neurons, glia, and astrocytes within the brain. These brain-exosomes can be detected in the blood's plasma as CNS disease biomarkers.9 To study their ability to cross the BBB, exosomes from different cells or biological fluids were radioactively labeled and injected intravenously into mice, and were subsequently detected in the mice's brains.10 Compared to the limited cases of FDA approved antibodies (Aduhelm, Leqembi and Kisunla) or gene therapy (ZOLGENSMA) for treating Alzheimer's disease or pediatric spinal muscular atrophy (SMA), which can cross the BBB, exosomes naturally carry genetic or cellular materials from their host cells and deliver these materials to the brain cells. For example, exosomes derived from stem cells can be used as cell-free therapy for CNS diseases similar to their host cells, such as mesenchymal stem cell (MSC) exosomes.11 In addition, exosomes also have been used as natural lipid-bilayer nanoparticles to encapsulate drugs, proteins, vaccines, miRNA, mRNA, DNA aptamers, and plasmid DNA.9 For better CNS targeting, exosomes can also be engineered with RVG, antibodies, and other molecules for specific brain cell targeting, as recently reviewed by Neiland et al.12 In this review, we use the term “extracellular vesicles (EVs)” following the Minimal Information for Studies of Extracellular Vesicles 2018 (MISEV2018) guidelines.13 While the cited references may use “exosomes”, we consider EVs and exosomes interchangeable in this context.
Here, we reviewed the preclinical applications and clinical trials of naïve EVs in treating CNS disorders, especially stem cell-derived EVs. Then, we summarized EVs as gene or drug delivery vehicles which includes the brief history of EVs as delivery carriers, reviewed the most recent two years’ new research of EVs carried drug/gene in treating different CNS diseases, highlighted the engineered CNS-targeting EVs protocols, which opened a promising hope for CNS therapy. However, the limited EVs yields, various EVs’ resources, isolation and purification of EVs are challenging for commercial EVs translation. These have been recently reviewed by Fan et al.14 Therefore, we focus on the application of EVs in CNS. We also explore their potential as precision-targeting and personalized medication tools and discuss their integration with other nanotechnologies for advancing CNS disease treatment.
2. Applications and clinical trials of naïve EVs in treating CNS diseases
Naïve EVs are naturally produced by cells without engineering or loading of drugs. They have been found to be the underlying mechanisms of stem cell therapies. Since 1980s, stem cell therapies, including those using MSCs, have been used to treat neurodegenerative diseases.15,16 The primary mechanism of these cells is not their regeneration or differentiation abilities, but mainly the secretomes from the paracrine,11 and EVs being one of the important secretomes of the MSC cells.17 Unlike stem cells, EVs could not replicate but carry their host cells’ non-genetic contents to be functional which make EVs a safer cell therapy substitute. More importantly, EVs have emerged as a promising drug delivery system for central nervous system (CNS) disorders due to their ability to cross the blood BBB and deliver therapeutic cargo to the brain.18,19 MSCs derived EVs in treating CNS diseases has been studied by many researcher for more than ten years. We summarized the mechanisms of MSCs EVs in CNS and then surveyed the clinical trials of EVs in CNS diseases.
The first application was to systematically administer MCS EVs to stroke model rats. The rats recovered functionally by increasing neurite remodeling (Fig. 1A).20 MSC's EVs have also shown therapeutic results in other CNS disorders including brain tumors, stroke, traumatic brain injury, Alzheimer disease, Parkinson disease, Huntington disease, Amyotrophic lateral sclerosis (ALS), multiple sclerosis, and etc.11,16,21 The mechanisms of MSC EVs in treating these disorders have also been studied. In both general tumors and brain tumors, MSC EVs have dual roles in inhibiting or promoting tumor growth.22 For inhibition, MSC derived EVs’ microRNA, the microRNA-133b, a known tumor suppressor, was able to attenuate glioma development.23 The results of the in vitro test and in vivo tail vein injection of MSC EVs to glioma nude mice suggested that the MSC EVs carrying miR-133b could inhibit glioma growth via disruption of the Wnt/β-catenin signaling pathway by inhibiting EZH2.23 More research suggested that MSC-EVs overexpressed miRNAs, proteins and as chemotherapy drugs carriers in treating brain cancers, which have been thoroughly reviewed by Ghasempour,24 Sun,9 Hao,25 Mousavikia,26 and Yang.27
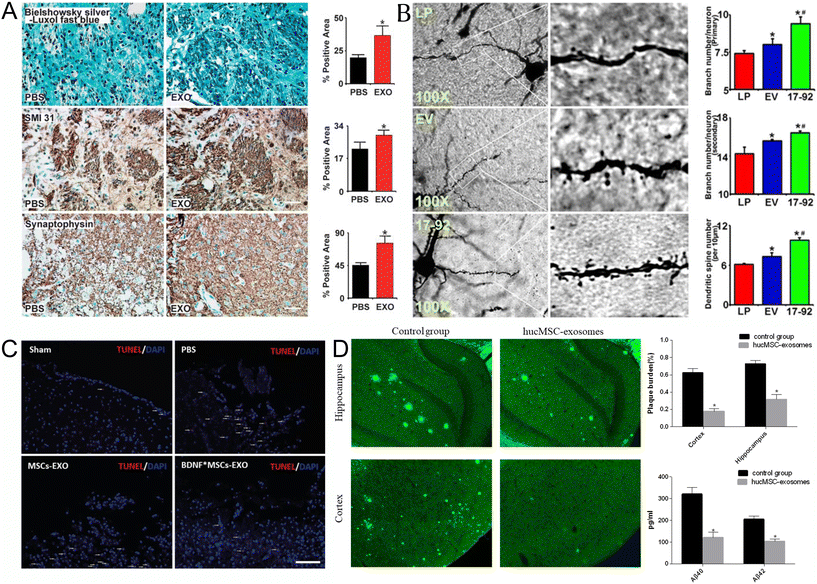 |
| Fig. 1 Naïve EVs in treating CNS diseases (A) Exosomes increase neurite remodeling in the ischemic boundary zone (IBZ). Bielschowsky silver and Luxol fast blue double staining of the neurofibrils (top row), SMI-31 immunostaining of the neurofilaments (middle row), and synaptophysin immunostaining of presynaptic vesicles in neurons (lower row) show that exosome treatment increased neurite remodeling and synaptic plasticity in the IBZ of ischemic rats compared with phosphate-buffered saline (PBS) treatment. *P < 0.05, respectively. Mean ± s.d., n = 6 per group. Scale bar 50 μm. Reproduced with permission from Xin et al.20 Copyright 2013 SAGE. (B) MiR-17-92 cluster-elevated MSC exosomes increase neuronal dendritic plasticity. Representative optical microscopy images (left) show the morphology of Golgi silver impregnation-stained neurons and their dendrites (individual row represents for LP, EV, and 17-92 treatment, respectively). The primary (right upper) and secondary (right middle) neurite branching as well as the spine density (right lower) were significantly increased after exosome treatment, and miR-17-92 cluster-enriched exosomes (Exo-miR-17-92+) further increased dendritic plasticity compared with the control MSC-derived exosomes (Exo-Con; P < 0.05, respectively). LP, MCAO rats treated with liposome; EV, MCAO rats treated with Exo-Con; 17-92, MCAO rats treated with Exo-miR-17-92+. MCAO indicates middle cerebral artery occlusion. *P < 0.05 compared with LP. #P < 0.05 compared with EV. Mean ± SE n = 3 per group. Reproduced with permission from Xin et al.28 Copyright 2017 LWW. (C) The apoptosis of brain cells (white dash in images) in the BDNF-induced MSCs-Exo group was significantly less than that in the MSC-Exo group, as detected by TUNEL staining after TBI (scale bar: 200 μm). Reproduced with permission from Xu et al.29 Copyright 2020 ISI. (D) Effects of HucMSC-exosomes injection on Aβ deposition and soluble Aβ quantities. Thioflavin S staining (left) was used to evaluate Aβ deposition. Images were captured with a camera system connected to a fluorescence microscope (Olympus 1 × 71S1F-3, Japan). Scale bar, 200 μm. Quantification of thioflavin S staining (right upper). The Aβ plaque burden was calculated as the percentage of the thioflavin S staining area over the total area. (n = 6 in each group). Image Pro Plus 6 (Media Cybernetics) was used to analyze the images. ELISA kits (Invitrogen) were performed to quantify soluble Aβ40 and Aβ42 levels in the mice (right lower). (n = 6 in each group). Data are presented as mean ± SEM; *P < 0.05; the hucMSC-exosomes transplantation group versus the control group. Reproduced with permission from Ding et al.30 Copyright 2018 Springer. | |
The mechanisms of MSC EVs in treating strokes have been reviewed by Otero-Ortega,31 Seyedaghamiri,32 and Yin.33 MSC derived EVs are involved in many restorative activities after stroke including neuroprotection, anti-inflammation, anti-apoptosis, angiogenesis and neurogenesis by targeting neurons, astrocytes, microglia, microvessels, etc.33,34 A good example is the EVs’ microRNAs in treating ischemic stroke.33 Chopp and his coworkers found that miR-133b in MSC derived EVs promotes neural plasticity and functional recovery after stroke by intravenously injecting these EVs to stroke rats.35 They also found that the secondary release of EVs from astrocytes treated with miR-133b EVs increased neurite outgrowth in stroke rat models.36 In addition, Xin and Chopp discovered miR-17-92 enriched MSC EVs exhibited significant improvement of neural plasticity and functional recovery (Fig. 1B).28 Later, they also found that the miR-17-82 enriched EVs can enhance axon-myelin remodeling and electrophysiological recovery after stroke, but lost function by adding PI3K/Akt/mTOR pathway inhibitors. This suggests that miR-17-92 targeted the phosphatase and tensin homolog to activate the PI3K/Akt/mTOR pathway.37 Furthermore, MSC derived EVs reduced miR-21-3p level, increased miR-150-5p level in middle cerebral artery occlusion (MCAO) rats, and/or increased miR25-3p level in stroke mice to inhibit neuronal apoptosis and autophagy.38–40 Additionally, a few microRNAs in MSC derived EVs were found to attenuate stroke through microglia: miR-146a-5p reduced microglial-mediated neuroinflammation by suppression of the IRAK1/TRAF6 signaling pathway,41 miR-26a-5p mediated suppression of CDK inhibited microglia apoptosis,42 miR-30d-5p inhibited autophagy-mediated microglial polarization to M1 to prevent cerebral injury in acute ischemic stroke rats,43 microRNA-223-3p attenuated rats’ cerebral ischemia through inhibiting microglial M1 polarization mediated inflammation,44 and miR-126 improved neurogenesis and suppressed microglia activation in stroke rats.45 Furthermore, microRNA-138-5p-overexpressing MSC derived EVs confer neuroprotection to astrocytes via inhibition of lipocalin 2 in stroke mice and cultured cells.46 What's more, EVs’ miR21-5p promoted angiogenesis in ischemic stroke mice to significantly reduce infarct volume and improve neurological functions; these results were confirmed by in vitro tests in human umbilical vein endothelial cells.47 Moreover, some in vitro results showed the mechanisms of MSC derived EVs: EVs’ miR-181b-5p promoted the angiogenesis of brain microvascular endothelial cells after oxygen–glucose deprivation.48 EVs’ miR-22-3p alleviated cerebral ischemic injury by inhibiting KDM6B mediated effects on the BMP2/BMF axis,49 and EVs’ miR-134 suppressed rat oligodendrocytes apoptosis by negatively regulating the caspase-8.50 Other mechanisms of MSC derived EVs in treating stroke include increasing interleukin-33 (IL-33) and suppressing tumorigenicity 2 receptor (ST2) in astrocytes, which released neurotrophic factors to improve neuronal survival under ischemia-condition in brain.51 EVs derived from neural stem cells also have been used in stroke mice,52 rats,53–55 and porcine56 models.
MSC derived EVs showed promising neuroprotection and neurorestoration in TBI, while all previous TBI clinical trials failed.57 The mechanisms of MSC derived EVs in treating TBI have been recently reviewed by Xiong, Mot, Beylerli and Zhang.57–60 For example, three microRNAs have been found in MSC derived EVs associated with TBI recovery. MiR-124 promoted microglia's M2 polarization by inhibiting TLR4 pathway to help anti-inflammation and enhance hippocampus neurogenesis after TBI.61 In addition, EVs derived from MSCs treated with brain-derived neurotrophic factors better promote neurogenesis and inhibit apoptosis than MSCs-derived EVs in rats after TBI (Fig. 1C). This mechanism may be related to the high expression of miR-216a-5p after determining the mRNA of BDNF MSCs-derived EVs treated rats.29 Also, the known miR-17-92 in stroke improved tissue miR-17-92 cluster-enriched MSC derived EVs.62
Alzheimer diseases (AD) is a neurodegenerative disease characterized by the progressive loss of neurons in the brain, and there is no effective drug used as treatment.63,64 Recently, MSC derived EVs demonstrated significant neuroprotective effects in preclinical research which was reviewed by Chang.64 It has been shown that human MSC derived EVs can reduce β-amyloid and hyperphosphorylated Tau in AD mice and rats’ brains (Fig. 1D).30,65,66
The clinical applications of EVs for CNS drug delivery have progressed significantly over the past decade. Here are the current clinical trials registered in the U.S. (ClinicalTrials.gov) by using EVs to treat CNS diseases in Table 1. The CNS diseases include acute ischemic stroke, craniofacial neuralgia, depression, anxiety and dementias, Alzheimer's disease, Parkinson's disease, autism, epilepsy, extreme low birth weight, etc.
Table 1 Clinical trials of EVs for CNS
ClinicalTrial.gov ID |
Diseases |
EVs sources |
Injection methods |
Phase |
Study year |
NCT03384433 |
Acute ischemic stroke |
Allogenic MSC |
Stereotaxis/intraparenchymal |
1 & 2 |
2017–2021 |
NCT04202783 |
Craniofacial neuralgia |
Neonatal stem cells |
FUS and Intravenous |
Pending due to covid-19 |
2019–2024 |
NCT04202770 |
Depression, anxiety, and dementias |
MSC |
FUS and Intravenous |
Pending due to covid-19 |
2019–2024 |
NCT04388982 |
Alzheimer's disease |
Allogenic adipose MSC |
Intranasal |
1 & 2 |
2020–2022 |
NCT05490173 |
Extremely low birth weight |
MSC |
Intranasal |
N/A |
2022–2026 |
NCT05886205 |
Refractory focal epilepsy |
iPSC |
Intranasal |
1 |
2023–2025 |
NCT06138210 |
Acute ischemic stroke |
Human iPSC |
Intravenous |
1 |
2024–2025 |
NCT06612710 |
Ischemic stroke |
Human induced neural stem cells |
Intravenous |
1 |
2024–2027 |
NCT06607900 |
Alzheimer's disease, Parkinson's disease, multiple system atrophy, Lewy body dementia, and frontotemporal dementia |
Human umbilical cord MSC |
Intranasal |
1 |
2024–2028 |
NCT06600529 |
Autism spectrum disorder |
Umbilical cord |
Intravenous |
N/A |
2024–2025 |
There are two completed phase I/II clinical trials of EVs in CNS diseases (Table 1). One is the allogenic placenta mesenchymal stem cell derived exosome in patients with acute ischemic stroke clinical results (NTC03384433). After decompressive craniectomy treatment of malignant middle cerebral artery infarct, within 48 h, intraparenchymal injection of placenta MSC derived EVs to five patients (male) has been reported to have no post-interventional adverse effects (Fig. 2A and B). This indicated that EVs have no toxicity with a 3-months follow-up of safety and disability indexes.67 The function of MSC derived EVs to help recovery of acute ischemic stroke patients needs to be tested in future clinical trials after this safety pilot research. The other completed clinical trial research is Clinical safety and efficacy of allogeneic human adipose mesenchymal stromal cells-derived exosomes in patients with mild to moderate Alzheimer's disease (AD): a phase I/II clinical trial (NTC04388982).68 The results showed that intranasal administration of allogeneic human adipose MSCs-EVs (ahaMSCs-Exos) in nine mild to moderate AD patients (5 males and 4 females) was safe and well tolerated, and a dose of at least 4 × 108 particles could be selected for further clinical trials. The results also suggested that ahaMSCs-Exos may have therapy effect equivalent or better than drugs for AD on the current market drugs like GV-971 or donepezil (Fig. 2C and D). There is a new registered clinical trial planning to test the effect of intravenous injection of human induced ploripotent stem cell (iPSC) derived EVs to treat acute ischemic stroke's patients. EVs from these stem cells have demonstrated high regenerative properties such as angiogenesis, cell proliferation, anti-apoptosis69 and supporting the immune system.24
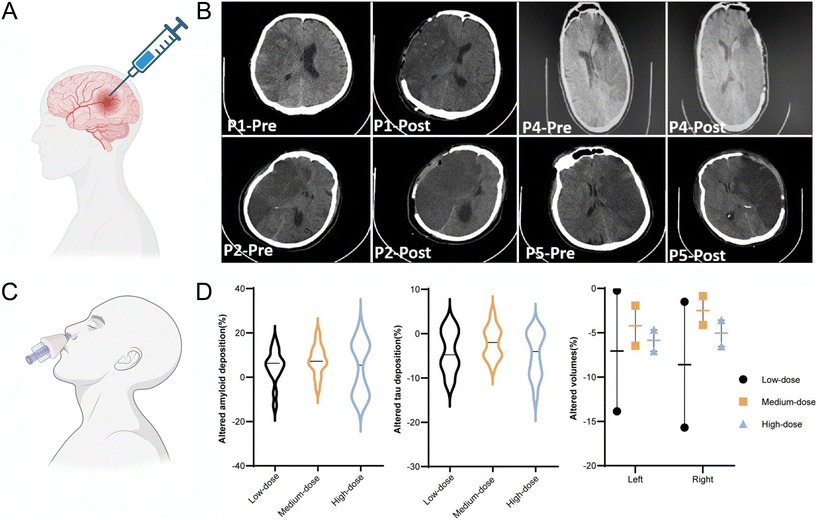 |
| Fig. 2 Clinical trials report of EVs treatment and safety evaluation in acute ischemic stroke and AD patients. (A) Schematic of intraparenchymal injection of allogeneic MSC exosomes to acute ischemic stroke patients after decompressive craniectomy. Created with BioRender.com (B) Computed tomography (CT) scans of patient pre and post operation from, P1 to P5 after malignant middle cerebral artery infarct patients underwent decompressive craniectomy, and all received a dose of exosome in infarct penumbra region by frameless stereotactic navigation cannula in one minute. Reproduced with permission from Dehghani et al.67 Copyright 2022 Medknow. (C) Intranasal administration of exosomes to AD patients. Created with BioRender.com (D) Changes of hallmarks of AD pathology (amyloid, tau, neurodegeneration) in the follow-up period compared with baseline after mild to moderate AD patient intranasally administered human MSC exosomes. The violin plot of the amyloid deposition (left) and tau deposition (middle) alterations after 1 year detected by PET-MRI, compared with baseline. The selected regions of interest included the frontal cortex, parietal cortex, lateral temporal, precuneus, anterior cingulate cortex and posterior cingulate cortex for amyloid, whereas lateral parietal, lateral temporal, medial temporal, frontal, occipital cortex, precuneus and posterior cingulate cortex for tau. Altered proportions of bilateral hippocampal volumes indicated less atrophy in the subjects who accepted medium-dose administration (right). AD, Alzheimer's disease; MRI, magnetic resonance imaging; PET, positron emission tomography. Reproduced with permission from Xie et al.68 Copyright 2023 BMJ. | |
The current clinical trials only use stem cell derived EVs, especially from MSCs and iPSCs without any engineering or modification. EVs from non-stem cells like blood, plant, amniotic fluid, tumor cells, bacteria, cardiovascular progenitor cells, dendritic cells and T cells have been used in clinical trials non-CNS diseases which has been recently reviewed by Mizenko et al.70 Also, the methods of encapsulating drugs/genes to EVs has been reviewed by Fan et al., including incubation freeze–thaw, sonication, saponin, electroporation, extrusion, covalent and non-covalent modification.14
3. Applications of EVs as drugs/genes delivery vehicles in CNS diseases
EVs have been applied to be drug/gene delivery vehicles for more than a decade. Herein, we first summarized the EVs drug delivery history and highlighted some of the typical applications in CNS. Next, we reviewed the recent two years’ research of EVs carrying drugs or genes to treat different CNS diseases, and some of them applied EVs fusing with other nanoparticles. Then, we discussed EVs that deliver mRNA to the brain. Finally, we reviewed new methods of engineering EVs for CNS targeting.
3.1 EVs/drug delivery history and for CNS applications
EVs have been used as drug delivery vehicles since 2010. In Fig. 3, I highlighted some studies and organized them in the historical timeline. Sun et al. incorporated curcumin into EL-4-derived (mouse lymphoma cell line) EVs and then i.p injected to lipopolysaccharide (LPS)-induced septic shock mice and it showed better therapeutic effects compared to free curcumin.71 In 2011, Alvarez-Erviti et al. showed that EVs derived from dendritic cells expressed rabies virus glycoprotein (RVG), a neuron-specific peptide, could deliver siRNA to the mouse brain after intravenous injection, resulting in a knockdown of the targeted BACE1 gene.72 They also showed that EVs can be delivered to the brain without engineering or modification. They used EVs from self-derived dendritic cells to reduce immunogenicity and engineered EVs with RVG for better neuron targeting.72 In the same year, Zhuang et al. demonstrated that EVs could deliver anti-inflammatory drugs across the BBB to treat brain inflammation. In a mouse model of lipopolysaccharide-induced brain inflammation, EVs were loaded with curcumin or a Stat3 inhibitor and showed significant anti-inflammatory effects after intranasal administration.73 In 2013, Xin et al. used MSC derived EVs to treat stroke by systemic administration in a rat model, and the EVs promoted functional recovery and increased neurite remodeling through microRNA-133b.20,35 In 2014, Cooper et al. applied RVG engineered EVs to encapsulate alpha-synuclein siRNA to human phospho-mimic S129D alpha synuclein and overexpressed transgenic mice by systemic injection, and found that the alpha synuclein mRNA and protein level decreased in the treated mice's dopaminergic neurons in the substantia nigra.74 In 2015, Haney et al. showed that EVs could deliver catalase across the BBB to treat Parkinson's disease in a mouse model.75 The EVs were isolated from macrophages, loaded with catalase, and administered intranasally. This experiment demonstrated significant neuroprotective effects and attenuation of neuroinflammation. In 2016, siRNAs were conjugated with TEG-cholesterol to make them more hydrophobic to fuse with EVs.76 In 2018, dopamine was encapsulated in EVs and successfully delivered to hippocampus neurons in PD mice models.77 This might help PD patients by taking dopamine instead of its precursor, levodopa, to which patients can develop resistance, leading to symptoms not improving.78 A 2020 study by Izadpanah et al. utilized EVs to deliver neprilysin, an amyloid beta-degrading enzyme, to the brains of transgenic mice for the treatment of Alzheimer's Disease.79 Another study utilized EVs for brain disease through injection of plasmids-siRNA modified with RVG-Lamb2 to mice's liver. Then, the liver cells synthesize RVG-EVs-siRNA and extracellular transported to targeted neurons in the brain.80 This could make gene delivery by EVs to be simplified from EVs isolation, purification, and encapsulation, which are the main challenges for EVs manufacturing and marketing. The engineered EVs crossed the BBB to the brain after intravenous injection and reduced amyloid beta levels and plaque deposition in the brain. Additionally, researchers are exploring novel sources of EVs with innate brain-targeting abilities. A 2017 study by Yuan et al. made use of naïve macrophage (Mϕ) EVs to deliver BDNF, which also showed better efficiency to the mice brain.81 Another study in 2023 by Zhu et al. additionally demonstrated that EVs from neural stem cells could deliver therapeutic proteins (BDNF) to the brain more efficiently than EVs from other cell types.55 Other than mice or rats models, other animal models such us zebrafishes and monkeys have also been tested using EVs as drug delivery after systemic injection.82,83
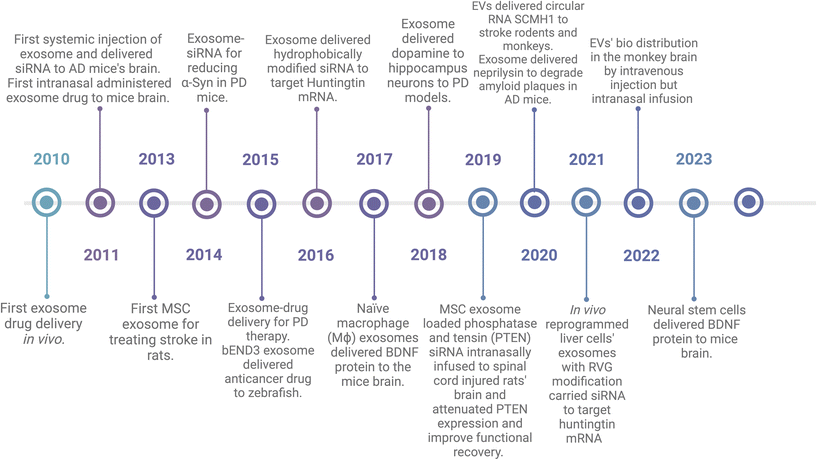 |
| Fig. 3 History of EVs encapsulated drugs to CNS. Created with BioRender.com. | |
There were a few publications of EVs as drug/gene delivery in CNS every year until 2019 and the publications after 2020 boomed. These findings have been thoroughly reviewed by Shahjin, Shaimardanova, Sun, Khan and Zhang.9,21,84–86 In Table 2 and the following subchapters, we summarized new publications from the past two years (till 2024 June) of preclinical applications of EVs in treating CNS diseases, such as AD, PD, depression, glioma, glioblastoma, MS, neural inflammation, drug substitute, cancer brain metastases, spinocerebellar ataxia type 3, and demyelinating disease.
Table 2 Preclinical research of EVs delivered drug/gene to CNS (2023–2024)
Disease |
Drug/gene |
Donor cells |
EV's modification |
Drug/gene loading |
Administration route |
Animal |
EVs’ targeted cells |
Ref. |
Alzheimer diseases |
Ber/Pal |
Microglia |
— |
Sonication |
Intravenous |
Mice |
Microglia |
87
|
Cory-B |
Hippocampus neurons |
Fe65 |
Sonication |
Intravenous |
Mice |
Neurons |
88
|
T-ALZ01 |
Microglia |
— |
Sonication |
Intranasal |
Rats |
Microglia |
89
|
miR29b-2 |
Dendritic cell |
SST |
Sonication |
Intravenous |
Mice |
Neurons |
90
|
Parkinson diseases |
Si-FTO |
MSC |
— |
Sonication |
Intravenous |
Mice |
Neurons |
91
|
Bai/Ole |
hUC MSC |
— |
Freeze/thaw |
— |
— |
Neurons |
92
|
miR-133b |
MSC |
RVG29 |
Sonication |
Intravenous |
Mice |
Neurons |
93
|
Major depressive disorder |
SIRT2 |
Oligodendrocyte |
— |
Transfection |
Intravenous |
Mice |
Hippocampus neurons |
94
|
Drug substitute |
CBD |
HEK 293T |
RVG |
Sonication |
Intraperitoneal |
Mice |
Neurons |
95
|
Brain cancer |
TMZ |
Astrocyte |
Folic acid |
Electroporation |
Intravenous |
Mice |
Glioma cells |
96
|
SAB/CPT |
Rats’ serum |
TfR |
Electroporation |
Intravenous |
Mice |
Glioma cells |
97
|
DOX |
A549, LLC, BV2, U87 cells |
Pep2 |
Incubation |
Intravenous |
Mice |
Glioblastoma cells |
98
|
siRNA/TMZ |
Blood |
— |
Electroporation |
Intravenous |
mice |
Glioblastoma cells |
99
|
si-LPCAT1 |
HEK 293T |
EGFR ScFV |
Electroporation |
Intravenous |
Mice |
Cancer cells |
100
|
RSL3 |
NK-92 cells |
CART/T7 |
Co-extrusion |
Intravenous |
Mice |
Cancer cells |
101
|
Multiple sclerosis |
T3 |
Neural stem cell |
PDGFP-ligand |
Transfection |
Intravenous |
Mice |
Oligodendrocyte |
102
|
Spinocereb-ellar ataxia 3 |
miR-25 miR-181a |
HEK 293 |
RVG |
Electroporation |
Intravenous |
Mice |
Neurons |
103
|
3.2 Recent applications of EVs in different CNS diseases
3.2.1 Alzheimer disease (AD).
Alzheimer disease (AD) is one of the most prevalent neurodegenerative diseases in both the U.S. and worldwide and is the most common cause of dementia.104 AD is biologically characterized by β-amyloid (Aβ)-containing extracellular plaques and tau-containing intracellular neurofibrillary tangles.104 Targeting to remove the disease mutated Aβ plaques or their precursors is the focus of most preclinical and clinical studies. Recently, there have been three FDA approved antibodies that can remove Aβ plaques for early or median AD patients. However, they are expensive and have not been approved to be very effective in the AD community. EVs, especially the stem cell derivative EVs, have emerged as a promising hope for treating AD.
PSEN1 is one of the genetic factors for the early-onset AD patient (familia AD). Lin et al. found that microRNA-29b-2 (miR29b-2) could reduce presenilin 1 (PSEN1) gene expression and decreasing the β-amyloid accumulation for Alzheimer's disease (AD) in vitro and in vivo. They created new engineered dendritic cells’ EVs with the CD47-somatostatin (SST), and encapsulated miR29b-2, then intravenously injected to 3xTg-AD mice. As a result, it targeted the mice's brains and significantly reduced Aβ 1-42 oligomer protein in the hippocampus region (Fig. 4A).90
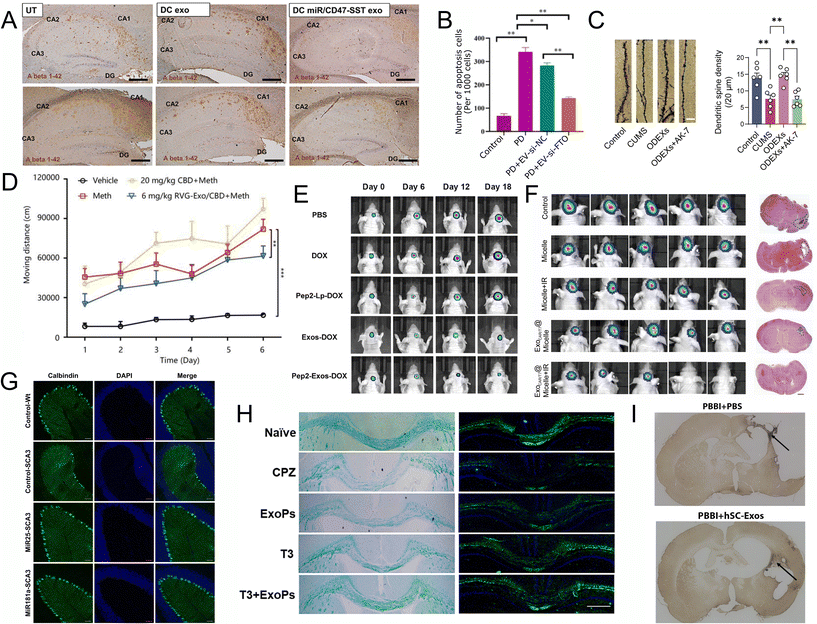 |
| Fig. 4 Recent applications of EVs for CNS disease. (A) The Aβ 1–42 oligomers protein content of the 3xTg-AD mouse hippocampus was examined with IHC (scale bar represents 200 μm). UT, untreated. DC exo, dendritic cell exosomes treated. DC miR/CD47-SST exo, engineered dendritic cell exosome with SST linked exosomal CD47 and overexpressed miR29b-2 in exosomes. Reproduced with permission from Lin et al.90 Copyright 2024 Dove. (B) Immunofluorescence staining analysis of the apoptosis of dopaminergic neurons in the striatum of mice PD model injected with MSC-exo-si-NC or MSC-exo-si-FTO (n = 3). Data are represented as mean ± SD, *represents P < 0.05, **represents P < 0.01. Reproduced with permission from Geng et al.91 Copyright 2023 BMC Springer. (C) Golgi-Cox-stained images of dendritic spines (scale bar, 5μm) and the quantification of dendritic spine density (n = 6). CUMS, mice with chronic unpredictable mild stress (CUMS)-induced depression via the tail vein. ODEXs oligodendrocytes exosomes. AK-7, selective SIRT2 inhibitor. Four groups are control group (Control), CUMS group (CUMS), CUMS + ODEX group (ODEXs), and CUMS + ODEX + AK-7 group (ODEXs + AK-7). Reproduced with permission from Zhang et al.94 Copyright 2024 WILEY. (D) The effect of CBD and RVG-Exo/CBD on Meth-induced behavioral sensitization. Reproduced with permission from Li et al.95 Copyright 2024 Elsevier. (E) Tumor volumes of tumor-bearing mice treated with PBS, DOX, Pep2-Lp-DOX, Exos-DOX and Pep2-Exos-DOX at different time points with IVIS Spectrum. Reproduced with permission from Wang et al.98 Copyright 2024 Elsevier. (F) Luminescence images of HER2+ BCBM lesion tumor-bearing nude mice after different treatments and representative H&E images (scale bars = 1 mm) of tumor area after different treatments, n = 5. Reproduced with permission from Tao et al.101 Copyright 2024 Elsevier. (G) Exosomal miRNA treatment reduced the apoptosis of Calbindin positive cells. Histological analysis of Calbindin positive cells in the sagittal cerebellar section. 4 weeks after initial injection. Scale bar = 50 μm. Reproduced with permission from Tang et al.103 Copyright 2023 Springer. (H) T3 + ExoPs improved remyelination in the CPZ-induced animal model. LFB and FluoroMyelin stains of the corpus callosum area. Reproduced with permission from Wang et al.102 Copyright 2024 Elsevier. (I) hSC-Exos treatment reduces the number of Iba-1 positive activated and amoeboid microglia 21 days after penetrating ballistic-like brain injury (PBBI). Representative images (10×) in the injured cortex. Reproduced with permission from Nishimura et al.105 Copyright 2024 MAL. | |
Jiang et al. made hybrid EVs-lipid nanoparticles that co-delivered the β-site amyloid precursor protein (APP) cleaving enzyme-1 (BACE1) siRNA, and TREM2 plasmid (pTREM2) gene drug with angiopep-2 to APP/PS1 mice.106 The expressed TREM2 transformed the microglia from pro-inflammatory to an active anti-inflammatory state, which could improve phagocytosis Aβ plaques, curb neuroinflammation, and guard neurons. Together with the siBACE1 to inhibit the BACE1 activities, it results in less amyloid protein generated in APP/PS1 mouse model and improved their cognitive function.
Iyaswamy et al. engineered EVs derived from hippocampus neurons with overexpressed Fe65 protein and encapsulated a natural autophagy inducer, Cory-B, to treat the AD mouse.88 The Fe65 hijacked the APP over-expressed neurons in the treated mice, delivered Cory-B to these neurons, and induced the autophagy to clear the APPs. The treated AD mice demonstrated improved cognitive behavior in the rotarod and Morris’ water maze tests.
There are two other studies made using neuroinflammation inhibitors encapsulated in EVs to treat AD. Richards et al. used the T-ALZ01, an inhibitor of complement component C1r. The EVs-T-ALZ01 demonstrated significant reduction in degenerating neurons and also the activation of resident microglia, astrocytes, and inflammatory markers in vivo in the lipopolysaccharide (LPS)-induced AD rat model.89 Zhao et al. used the Berberine and Palmatine (Ber/Pal), and EVs-Ber/Pal, which showed improvement in cognitive impairment and nerve injury in APP/PS1 Mice.87
3.2.2 Parkinson disease (PD).
Parkinson disease (PD) is the second most common neurodegenerative disease worldwide and has been characterized by dopaminergic neuronal loss in the substantia nigra and widespread intracellular protein (α-synuclein) accumulation.107 The current treatment for PD focuses on improving motor symptoms by medication, like levodopa (dopamine precursor) and deep brain stimulation.108 However, these treatments cannot slow the PD progress or cure it. Targeting α-synuclein can be a new hope for PD. PD might have diverse pathological underlying causes, including tau, polyglutamine, and Alzheimer's disease pathology, as well as nigral cell loss without hallmark pathological features of α-synuclein.109 EVs have been used to deliver drugs or siRNA to target α-synuclein, Tau, and other genes for PD treatment.
Geng et al. found that the obesity-related protein (FTO) is overexpressed in PD patients. They utilized MSC-EVs delivering FTO-targeted siRNA (si-FTO) in the PD mice and successfully delivered si-FTO to the striatum of animal brain, which synergistically alleviates dopaminergic neuronal death and significantly suppressed α-Syn expression (Fig. 4B). In addition, their results suggest that FTO upregulates ATM (ataxia telangiectasia mutated) gene expression through increasing its mRNA stability in dopaminergic neurons.91
Aliakbari used oleuropein (Ole) to inhibit primary and secondary αSN fibrillation and neurotoxicity in SHSY5Y cells. They found that these nanosystems took advantage of the high drug loading with lipid-drug in the nanoliposomes, but also better cell internalization, less cytotoxicity, and successful BBB crossing of EVs.92
Jiang et al. delivered miR-133b encapsulated in RVG29 peptide-modified EVs to PD mice, which improved their motor function, reduced depression, enhanced dopaminergic neuronal function, and attenuated 6-OHDA-induced nerve damage. They also found that the mechanism of RVG29-EVs-133b in PD treatment decreases the phosphorylation level of the Tau protein by targeting the RhoA-ROCK signaling pathway.93
3.2.3 Major depressive disorder (MDD).
Major depressive disorder (MDD), clinical depression, is a common mental disorder that affects millions of people globally; it is characterized by a prolonged low mood and is associated with high suicide rates in children, adolescents and adults.110 The neuron pathways of MDD include neurogenesis dysfunction and lower synaptic density in both patients and animal models.111,112 Zhang et al. found that the mechanism of sirtuin 2 (SIRT2) within the oligodendrocyte derived EVs in depressed mice is through AKT/GSK-3 pathway due to a loss of function confirmed by SIRT2 selected inhibitor AK7.94 They overexpressed SIRT2 in oligodendrocytes and isolated these EVs (ODEXs) and intravenously injected them to CUMS induced depressed mice. The results showed ODEXs treatment significantly ameliorated depressive-like behaviors, and restored neurogenesis and synaptic plasticity in CUMS mice. For example, the spines of dendrites were restored with ODEXs treatment, but AK-7 eliminated the beneficial impact of ODEXs on synaptic plasticity (Fig. 4C).
3.2.4 Drug substitute.
Cannabidiol (CBD) is an abundant non-psychoactive phytocannabinoids in cannabis extracts, which has been used in epilepsy, mental health, pain relief, and etc.113 With the increasing demand of CBD, the adverse effects and toxicity of CBD problems cannot be ignored and lowering the dose of CBD is a promising hope.114 Li et al. invented a new nanodelivery system with RVG-modified EVs encapsulated CBD (RVG-EVs-CBD), enhancing brain-targeting and efficacy. While suppressing the movement of the methamphetamine-induced (Meth) hyperactivity in mice, the RVG-EVs-CBD treatment used less than ⅓ of drug than the free CBD, but showed better suppression function (Fig. 4D).95
3.2.5 Brain cancer.
Here, I discussed three types of brain cancers which can apply EVs to their treatment: glioma, glioblastoma, brain metastases.
Glioma is a type of primary brain tumor (originates from the brain) that is believed to have developed from neural stem cells or progenitor cells.115 The current treatment for glioma includes chemotherapy, radiation, and tumor-treating fields, but also involves non-specific targeting of the proliferating cells.116 Temozolomide (TMZ) is the first chemotherapy drug for glioma, but it has side effects and is non-specific to glioma cells.117 Liu et al. used the astrocyte-derived EVs decorated with folic acid to deliver TMZ. They showed enhanced effects against glioma in mice models. Also, there was significantly less cytotoxicity of TMZ@Astro-EVs-FA than free TMZ. This research demonstrates the potential for EVs to be utilized for targeted drug delivery and drug carriers in the treatment of glioma.96
A traditional Chinese herb, Danshen, can promote blood circulation and remove blood stasis which is used as an anti-tumour medication clinically.118 The hybridized liposome with EVs decorated with transferrin receptors were used to co-deliver two Danshen affective molecules, cryptotanshinone (CPT), and salvianolic acid B (SAB) to treat glioma in mice models. The results showed increased BBB transcytosis into brain parenchyma and the targeting effect of CPT and SAB in the glioma.97
Glioblastoma, otherwise known as a grade 4 glioma, is the most common and aggressive primary brain tumor in adults.119 The current standard treatment for glioblastoma utilizes radiotherapy, alkylating chemotherapy, and surgery.119 However, treatment for glioblastoma using chemical drugs is extremely limited due to the presence of the blood brain barrier. Due to the current limitations, EVs have been identified as a potential method of delivering drugs to the brain and tumors.119 Wang et al. developed an EVs-based drug delivery system to target glioblastoma. They loaded the functional oligopeptide 2 (Pep2) decorated EVs with doxorubicin (DOX), which has been demonstrated to have significant anti-cancer activity. Their results show that the Pep2-EVs-DOX displayed favorable brain targeting ability as well as significant anti-cancer activity associated with DOX98 (Fig. 4E). Similarly, Zhao et al. demonstrated how blood EVs delivered specific TMZ targeted siRNA to treat glioblastoma. The results of their study showed that encapsulating both the siRNA and drugs used for treatment generated a significant therapeutic effect against TMZ resistant glioblastoma in vivo.99
Brain metastases are cancerous growths that have spread to the brain as a result of cancer being present in other areas of the body. Brain metastases often occur with breast, lung, colorectal cancers, renal cell carcinoma, or melanoma, which has resulted in roughly 20% of all patients with cancer-developing brain metastases.120
Current treatment for brain metastases is often limited due to the blood brain barrier. Jiang et al. demonstrated the EVs’ ability to assist in the treatment of brain metastases. In their study, anti-EGFR ScFv engineered EVs were loaded with siRNA targeting lysophosphatidylcholine acyltransferase 1 (LPCAT1) and were able to cross the blood brain barrier and efficiently arrest tumor growth.100
Similarly, Tao et al. investigated whether EVs could be used to disrupt ferroptosis defense mechanisms. Disrupting ferroptosis, an intracellular iron-dependent form of cell death, would enhance the effectiveness of antitumor treatment. Their study showed that administration of chimeric antigen receptor-natural killer cell derived EVs combined with a nanobomb led to significant anti-tumor response in vivo. Combined with the plus laser irradiation (IR) treatment, the tumors were significantly reduced or eliminated (Fig. 4F).101
3.2.6 Spinocerebellar ataxia type 3 (SCA3).
Spinaocerebeller ataxia type 3 (SCA3) is the most common autosomal dominant hereditary ataxia with the ATXN3 mutation. Tang et al. codelivered the miR-25 and miR-181a by RVG engineered EVs to treat the SAC3 mice, which resulted in inhibiting ATXN3 aggression, reducing neuron apoptosis (Fig. 4G), and inducing motor improvement.103
3.2.7 Multiple sclerosis (MS).
Multiple sclerosis (MS) is an autoimmune demyelinating disease and considered to be the most common cause of non-traumatic disability.121,122 The current treatments of MS cannot fully cure the patients. Wang et al. encapsulated the T3 into the EVs derived from neural stem cells (NSCs) engineered with platelet-derived growth factors A (PDGFA)-ligand to treat CPZ-induced demyelinating mice.102 The results showed a lower dose of T3-EVs-Ps enhanced remyelination compared with free T3 (Fig. 4H).
3.2.8 Traumatic brain injury (TBI).
Traumatic brain injury (TBI) is a common cause of disability and death globally. Penetrating TBI (pTBI) is caused by bullets and shrapnel. Nishimura et al. used EVs derived from human Schwann cells in treating penetrating ballistic-like brain injuries in mice models. The results showed significantly reduced overall contusion volume and decreased frequency of Iba-1 positive activated and amoeboid microglia (Fig. 4I).105
3.2.9 Neuroinflammation.
Neuroinflammation is associated with the development of various CNS disorders including AD, PD, and MDD. Ishida et al. made use of the EVs-like nanoparticles (ELNs) from Allium Tuberosum to alleviate Lipopolysaccharide (LPS)-induced inflammation in two microglial cell lines, BV-2 and MG-6, and showed significantly decreased levels of the LPS-induced nitric oxide and inflammatory cytokines.123 The plant-derived EVs can be good drug carriers for neuroinflammation.
3.3 Delivery mRNA to CNS with EVs
Research has also been conducted using EVs to deliver genes, especially mRNA. In 2020, Yang et al. showed that RVG engineered EVs derived from the overexpressed nerve growth factor mRNA host cells can carry and deliver mRNA, deliver to the ischemic cortex, and improve function after systemic administration. These EVs were found in cerebral ischemic mice.124 Furthermore, Rufino-Ramos et al. designed RVG-EVs in the host cells, which constantly overexpressed Cre mRNA to form Cre EVs for tracing extracellular communication between brain cells.125 After intracranially injecting Cre EV to the striata of Ai9 transgenic mice, the in vivo transfer of functional events mediated by physiological levels of endogenous brain-derived EVs throughout the brain have been detected. Another method of EVs-mRNA delivery to the brain is the leukocyte EVs with retrovirus-like capsids; the capsid-forming activity regulated cytoskeleton-associated (Arc) to better package overexpressed mRNA. These Cre mRNA EVs inherit endothelial adhesion molecules from donor cells, which makes it easier for them to cross the BBB and enter the neurons in neuro-inflammatory sites.126
3.4 Mechanisms and new methods of engineered EVs for targeting CNS applications
EVs without modification have limited ability to reach the brain after systemic administration. To address this, researchers have developed various strategies to engineer the surface of EVs, either endogenously by manipulating donor cells or exogenously by modifying isolated EVs. These modifications aim to enhance the targeting capabilities and functionality of EVs. RVG is one of the most common and effective neurons targeting molecules, which are both endogenously expressed on EVs with Lamp2 adapter and exogenously modified on EVs. We mentioned the engineering of EVs in our contents but here we demonstrated the mechanisms of engineering EVs and exemplified some typical engineering methods published recently. Also, Nieland et al. have thoroughly reviewed the engineered EVs to target CNS.12
Endogenous modifications involve genetic or chemical manipulation of donor cells to incorporate specific cargos and express surface markers on the EVs.127 For example, glioma cells and their derived EVs were labeled with a palmitoylated fluorescent tag, allowing visualization of EV uptake by microglia in the CNS in mouse models.128 Another approach used a sensitive EV reporter system to track the fate of engineered EVs using a palmitoylated luciferase.129
Meanwhile, exogenous modifications involve the isolation of EVs from biofluids or conditioned mediums, followed by additional cargo or marker loading to improve their targeting capabilities. For instance, EVs were conjugated with peptides using protein ligating enzymes, such as Sortase A and OaAEP1 ligase, to create covalent bonds between EV surface proteins and peptides.130 Another method involved the use of the C1C2 domain of human lactadherin to conjugate EVs with an epidermal growth factor receptor (EGFR)-targeting peptide, facilitating the uptake of these EVs by EGFR-positive cells.131
Tao et al. designed EVs with T7 peptide, which can bind to the transferrin receptor on cerebral vascular endothelial cells to enhance BBB penetration.101 They also added CAR decoration to the T7-EVs for better HER2+ tumor cells in the brain metastasis (Fig. 5A). Fe65, a brain-enriched phospho-adapter protein that interacts with APP, has also been incorporated in EVs for targeting amyloid-β precursor protein and encapsulated Corynoxine-B for inducing autophagy in AD mice model (Fig. 5B).88 EVs were also armed with platelet-derived growth factors, such as (PDGFA)-ligand to guide EVs to target the PDGFRα+ oligodendrocyte progenitor cells, which increased in the demyelination lesion area and encapsulated a myelination drug, 3,5,30-L-triiodothyronine (T3) and injected to demyelination mice model, which showed significantly promotes remyelination.102 Additionally, a redox-response oligopeptide was designed for incorporation into the membranes of EVs to lock the drug during circulation.98 The oligopeptides contain cysteine residues, which can form crosslinks with each other via disulfide bonds to lock the drug during blood circulation. This would release the drug by breaking the disulfide bonds when encountering the glioblastoma cells, which have high GSH concentration (Fig. 5C).
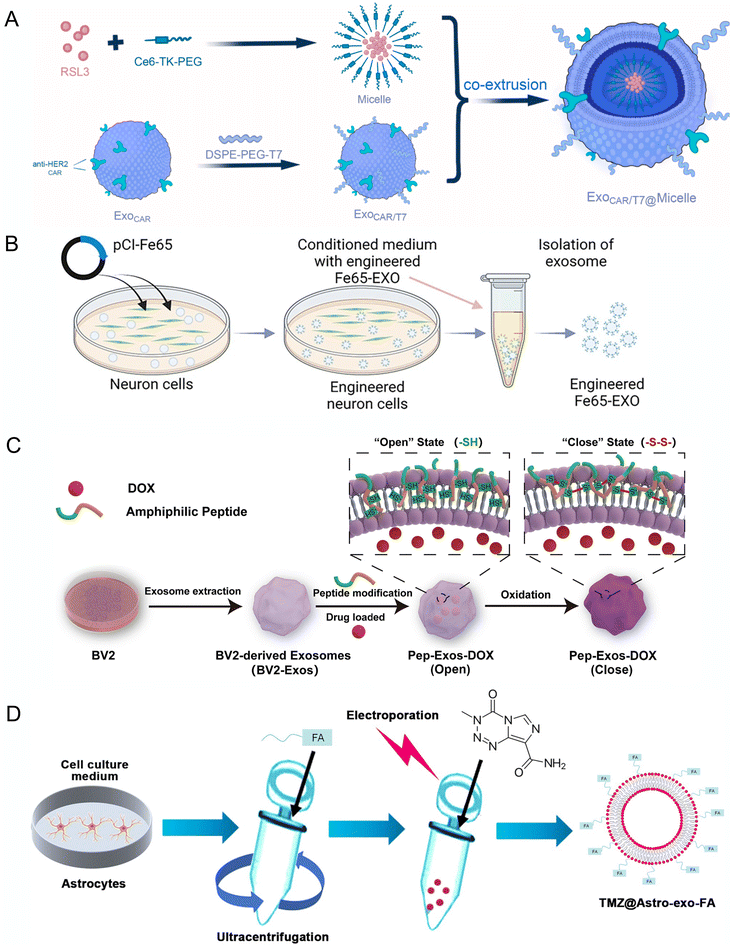 |
| Fig. 5 Engineering of EVs for targeting CNS applications. (A) Schematic diagram of endogenous anti-HER2 CAR and exogenous modification of T7 in exosomes and load with RSL3 in lipid micelle. Reproduced with permission from Tao et al.101 Copyright 2023 Elsevier. (B) Schematic diagram showing the steps involved in the production of engineered Fe65-EXO. Reproduced with permission from Iyaswamy et al.88 Copyright 2023 Springer. (C) Technical route flowchart for engineered pep exosomes preparation and mechanism of drug release. Reproduced with permission from Wang et al.98 Copyright 2024 Elsevier. (D) Schematic diagram representing the generation of engineered exosomes TMZ@Astro-exo-FA. Reproduced with permission from Liu et al.96 Copyright 2024 Wiley. | |
EVs have been exogenously modified with folic acid (FA) to prepare anti-cancer drug delivery systems targeting tumor cells, which highly express FA receptors in a glioblastoma mice model (Fig. 5D).96 Ligands specific to hippocampus neuron receptors, like cholecystokinin (CCT) and somatostatin, have also been used to engineer the EVs for targeting hippocampus neurons in AD mice model.90
4. Challenges in EVs research
4.1 Advancements in methods for isolating and purifying EVs
Innovative approaches are being developed to optimize the extraction and purification of EVs. Traditional methods, such as ultracentrifugation, although commonly utilized, are not only time-consuming but also potentially detrimental to the integrity of EVs’ membranes.132 In response to these challenges, researchers have introduced several novel and less invasive methodologies that promise both cost-effectiveness and efficiency. For instance, the method developed by Bathini et al. utilizes Vn96-bound magnetic particles integrated within a liquid biopsy chip to streamline the isolation of EVs, thereby enhancing the process efficiency.133 Chen et al. have introduced the EXODUS system, which employs negative pressure oscillation and dual resonators to agitate membranes, that has significantly accelerated and improved the EVs purification process.134 These technological advancements are tailored to meet specific requirements related to the source, desired purity, and end-use of the EVs. Their adoption is crucial for pushing the boundaries of EVs research forward, particularly in areas of disease diagnosis and therapeutic applications.
4.2 Expanding EVs sources for therapeutic applications
As the role of EVs in cellular communication is increasingly uncovered, their potential in therapeutic applications is also being recognized. Traditionally, EVs are extracted from bodily fluids and cell culture supernatants, but these sources are limited in yield and controllability, which are insufficient for clinical applications. Therefore, scientists are exploring new sources of EVs, such as stem cells, immune cells, and transformed cells, that can produce EVs with specific biological activities, thereby expanding their application in disease treatment.135
However, expanding the sources of EVs presents multiple challenges. First, efficiently extracting and purifying EVs from these new cellular sources remains a technical challenge. Additionally, ensuring the functional consistency and long-term stability of these EVs is a critical issue that must be resolved for clinical application. Researchers are developing new bioengineering methods and purification techniques to improve EVs yield and quality, aiming for more effective use in disease treatment and regenerative medicine.13
Plant-derived cells are recognized as a cost-effective and sustainable option for producing EVs due to their straightforward cultivation and plentiful resource availability. EVs isolated from plants contain rich bioactive components, including secondary metabolites, proteins, and lipids, that have demonstrated efficacy in anti-inflammatory, anti-cancer, and antioxidative treatments. In a notable development, Pan et al. created an innovative hydrogel-based system employing EVs-like nanovesicles collected from the external skin of aloe vera. This system, combined with a hydrogel from the plant's inner gel, has proven effective in mouse models for treating conditions, such as atopic dermatitis and diabetic wounds.136 Their compatibility and low toxicity primarily stem from their evolutionary distance from human cellular mechanisms, which substantially reduces immune reactions.137
5. Future directions
The rapid growth of EV-based drug delivery in non-CNS diseases has inspired the development of personalized and regenerative medicine for treating CNS diseases. In the manuscript, we summarize the precision targeting and personalized medicine approaches using EVs for various organs and diseases. We also discuss the fusion of EVs with other nanoparticles to target different organs and highlight their role in regenerative medicine for various tissues. These advancements provide a foundation for future research on the application of EVs in treating CNS diseases.
5.1 Precision targeting and personalized medicine
Advancements in bioengineering are expected to enhance the precision with which EVs can be directed to specific tissues or cell types.138 This involves functionalizing EVs with specific ligands or modifying their membrane compositions to improve their ability to target and merge with cells.139,140 Such precision will be crucial for developing personalized medicine approaches where treatments are tailored based on individual patient profiles and specific cellular targets.141 For example, a significant study conducted by Tian Y et al. demonstrates how EVs can be engineered to express ligands that specifically target receptors overexpressed in tumor cells.142 This approach facilitates the direct delivery of therapeutic agents to the tumor, thereby increasing treatment efficacy and reducing adverse effects on healthy tissues. The use of ligands like the iRGD peptide, which enhances the penetration of EVs into tumor tissues through the enhanced permeability and retention (EPR) effect, exemplifies this strategy. These ligands bind specifically to integrins on tumor cells, effectively guiding the EVs to their target and ensuring the delivery of their cargo directly into the tumor microenvironment.
5.2 Integration with other nanotechnologies
The integration of EVs with other nanotechnologies, especially magnetic nanoparticles, marks a considerable advancement in targeted therapy.143 Utilizing magnetic EV carriers that respond to external stimuli presents a potential solution for enhancing precision in targeting and delivery. This approach allows for the controlled manipulation of EVs biodistribution, enabling precise and on-demand therapeutic action.144 By attaching these nanoparticles, it becomes navigable via external magnetic fields, allowing for precise direction to specific body tissues.143 This method significantly improves the delivery of therapeutic agents directly to targeted areas, thus reducing unintended effects on healthy tissues and enhancing overall treatment efficacy. In practical applications, such as treating muscular dystrophy, this technology has been successfully employed to guide therapeutic molecules specifically to dystrophic muscles in mouse models. The results include notably improved muscle repair and reduced inflammation. This example underscores the broader potential of using magnetic nanoparticle–EVs systems in medical treatments, offering advantages, such as pinpoint targeting, controlled release of therapeutic agents, and minimized side effects, which collectively promise to transform the landscape of treatment for various diseases. This approach not only improves the effectiveness of treatments but also opens new avenues for the application of nanotechnology in medicine, particularly in the management and treatment of complex diseases.
5.3 EVs in regenerative medicine
Research on EVs in regenerative medicine is exploring their potential to promote tissue repair and regeneration across various contexts.145–147 For instance, studies have highlighted the use of mesenchymal stem cell (MSC)-derived EVs in enhancing soft tissue repair and regeneration, particularly in tendons, skeletal muscles, and peripheral nerves.148,149 These EVs have been shown to promote myogenesis, increase tenocyte differentiation, and enhance neurite outgrowth and Schwann cell proliferation. These are crucial for the healing of composite soft tissue defects.150 Another promising area is the application of EVs in cardiovascular regeneration. They have been used to protect cardiomyocytes from ischemic injury and promote cardiac regeneration through mechanisms, like neovascularization and anti-vascular remodeling.151 Additionally, EVs have been found effective in promoting angiogenesis in bone regeneration, enhancing the repair processes in various bone-related injuries.152 These findings suggest that EVs could play a vital role in advancing regenerative medicine by providing a platform for the efficient delivery of therapeutic molecules to specific tissues, thereby enhancing the natural repair processes.
The ongoing advancements in EVs research are setting the stage for transformative applications in personalized medicine, targeted therapy, and regenerative medicine. The integration of novel isolation techniques and the exploration of diverse EVs sources are not only enhancing the therapeutic potential of these vesicles but also paving the way for their broader application in clinical settings. With each innovation, particularly in harnessing plant-derived EVs for medical treatments, researchers are moving closer to realizing the full potential of EVs in improving patient outcomes and treating a wide range of diseases more effectively and sustainably.
6. Conclusions
Taken together, we have highlighted the applications of EVs in treating CNS diseases, covering both clinical and preclinical research. The clinical survey in this manuscript specifically focuses on EVs used in the treatment of CNS diseases, including a discussion of two completed clinical trials. We have also addressed key challenges related to the clinical application of EVs, such as low yields and difficulties in isolation. Finally, we have proposed future directions for EV-based therapies in CNS diseases, emphasizing the potential for achieving precise and personalized medicine.
Author contributions
Xi Shi: writing original draft, conceptualization, writing review & editing. Weilong He: writing original draft, conceptualization, writing review & editing. Ashwin Gupta, Daniel Wang and Leonardo Clark: writing original draft, writing review & editing. Thomas Wynn, Kyran To, Nitya Mirle, Akash Ganesh and Helena M Zeng: writing review & editing. Huiliang Wang: conceptualization, funding acquisition, supervision, and writing review & editing.
Data availability
No primary research results, software or code have been included and no new data were generated or analysed as part of this review.
Conflicts of interest
There are no conflicts to declare.
Acknowledgements
Prof. Huiliang Wang acknowledges funding support from the NIH Maximizing Investigators’ Research Award (National Institute of General Medical Sciences 1R35GM147408), Robert A. Welsh Foundation Grant (No. F-2084-20210327), Craig H. Neilsen Foundation Pilot Research Grant, National Science Foundation (NSF) CAREER award (2340964), the University of Texas at Austin Startup Fund and American Parkinson Disease Association (APDA) grant. We acknowledge BioRender.com for the figure drawing.
References
- S. El Andaloussi, I. Mäger, X. O. Breakefield and M. J. A. Wood, Nat. Rev. Drug Discovery, 2013, 12, 347–357 CrossRef PubMed.
- C. Aslan, S. H. Kiaie, N. M. Zolbanin, P. Lotfinejad, R. Ramezani, F. Kashanchi and R. Jafari, BMC Biotechnol., 2021, 21, 20 CrossRef PubMed.
- S. Lykke-Andersen, D. E. Brodersen and T. H. Jensen, J. Cell Sci., 2009, 122, 1487–1494 CrossRef PubMed.
- E. Evguenieva-Hackenberg, L. Hou, S. Glaeser and G. Klug, Wiley Interdiscip. Rev. RNA, 2014, 5, 623–635 Search PubMed.
- N. P. Hessvik and A. Llorente, Cell. Mol. Life Sci., 2018, 75, 193–208 CrossRef PubMed.
- G. Raposo, H. W. Nijman, W. Stoorvogel, R. Liejendekker, C. V. Harding, C. J. Melief and H. J. Geuze, J. Exp. Med., 1996, 183, 1161–1172 CrossRef CAS PubMed.
- L. Zitvogel, A. Regnault, A. Lozier, J. Wolfers, C. Flament, D. Tenza, P. Ricciardi-Castagnoli, G. Raposo and S. Amigorena, Nat. Med., 1998, 4, 594–600 CrossRef CAS PubMed.
- V. Zappulli, K. P. Friis, Z. Fitzpatrick, C. A. Maguire and X. O. Breakefield, J. Clin. Invest., 2016, 126, 1198–1207 CrossRef PubMed.
- K. Sun, X. Zheng, H. Jin, F. Yu and W. Zhao, Pharmaceutics, 2022, 14, 2252 CrossRef CAS PubMed.
- W. A. Banks, P. Sharma, K. M. Bullock, K. M. Hansen, N. Ludwig and T. L. Whiteside, Int. J. Mol. Sci., 2020, 21, 4407 CrossRef CAS PubMed.
- H. Yari, M. V. Mikhailova, M. Mardasi, M. Jafarzadehgharehziaaddin, S. Shahrokh, L. Thangavelu, H. Ahmadi, N. Shomali, Y. Yaghoubi, M. Zamani, M. Akbari and S. Alesaeidi, Stem Cell Res. Ther., 2022, 13, 423 CrossRef CAS PubMed.
- L. Nieland, S. Mahjoum, E. Grandell, K. Breyne and X. O. Breakefield, J. Controlled Release, 2023, 356, 493–506 CrossRef CAS PubMed.
- C. Théry, K. W. Witwer, E. Aikawa, M. J. Alcaraz, J. D. Anderson, R. Andriantsitohaina, A. Antoniou, T. Arab, F. Archer, G. K. Atkin-Smith, D. Craig Ayre, J.-M. Bach, D. Bachurski, H. Baharvand, L. Balaj, S. Baldacchino, N. N. Bauer, A. A. Baxter, M. Bebawy, C. Beckham, A. B. Zavec, A. Benmoussa, A. C. Berardi, P. Bergese, E. Bielska, C. Blenkiron, S. Bobis-Wozowicz, E. Boilard, W. Boireau, A. Bongiovanni, F. E. Borràs, S. Bosch, C. M. Boulanger, X. Breakefield, A. M. Breglio, M. Á. Brennan, D. R. Brigstock, A. Brisson, M. L. D. Broekman, J. F. Bromberg, P. Bryl-Górecka, S. Buch, A. H. Buck, D. Burger, S. Busatto, D. Buschmann, B. Bussolati, E. I. Buzás, J. B. Byrd, G. Camussi, D. R. F. Carter, S. Caruso, L. W. Chamley, Y.-T. Chang, C. Chen, S. Chen, L. Cheng, A. R. Chin, A. Clayton, S. P. Clerici, A. Cocks, E. Cocucci, R. J. Coffey, A. Cordeiro-da-Silva, Y. Couch, F. A. W. Coumans, B. Coyle, R. Crescitelli, M. F. Criado, C. D'Souza-Schorey, S. Das, A. D. Chaudhuri, P. de Candia, E. F. De Santana, O. De Wever, H. A. del Portillo, T. Demaret, S. Deville, A. Devitt, B. Dhondt, D. Di Vizio, L. C. Dieterich, V. Dolo, A. P. D. Rubio, M. Dominici, M. R. Dourado, T. A. P. Driedonks, F. V. Duarte, H. M. Duncan, R. M. Eichenberger, K. Ekström, S. E. L. Andaloussi, C. Elie-Caille, U. Erdbrügger, J. M. Falcón-Pérez, F. Fatima, J. E. Fish, M. Flores-Bellver, A. Försönits, A. Frelet-Barrand, F. Fricke, G. Fuhrmann, S. Gabrielsson, A. Gámez-Valero, C. Gardiner, K. Gärtner, R. Gaudin, Y. S. Gho, B. Giebel, C. Gilbert, M. Gimona, I. Giusti, D. C. I. Goberdhan, A. Görgens, S. M. Gorski, D. W. Greening, J. C. Gross, A. Gualerzi, G. N. Gupta, D. Gustafson, A. Handberg, R. A. Haraszti, P. Harrison, H. Hegyesi, A. Hendrix, A. F. Hill, F. H. Hochberg, K. F. Hoffmann, B. Holder, H. Holthofer, B. Hosseinkhani, G. Hu, Y. Huang, V. Huber, S. Hunt, A. G.-E. Ibrahim, T. Ikezu, J. M. Inal, M. Isin, A. Ivanova, H. K. Jackson, S. Jacobsen, S. M. Jay, M. Jayachandran, G. Jenster, L. Jiang, S. M. Johnson, J. C. Jones, A. Jong, T. Jovanovic-Talisman, S. Jung, R. Kalluri, S.-I. Kano, S. Kaur, Y. Kawamura, E. T. Keller, D. Khamari, E. Khomyakova, A. Khvorova, P. Kierulf, K. P. Kim, T. Kislinger, M. Klingeborn, D. J. Klinke, M. Kornek, M. M. Kosanović, Á. F. Kovács, E.-M. Krämer-Albers, S. Krasemann, M. Krause, I. V. Kurochkin, G. D. Kusuma, S. Kuypers, S. Laitinen, S. M. Langevin, L. R. Languino, J. Lannigan, C. Lässer, L. C. Laurent, G. Lavieu, E. Lázaro-Ibáñez, S. Le Lay, M.-S. Lee, Y. X. F. Lee, D. S. Lemos, M. Lenassi, A. Leszczynska, I. T. S. Li, K. Liao, S. F. Libregts, E. Ligeti, R. Lim, S. K. Lim, A. Linē, K. Linnemannstöns, A. Llorente, C. A. Lombard, M. J. Lorenowicz, Á. M. Lörincz, J. Lötvall, J. Lovett, M. C. Lowry, X. Loyer, Q. Lu, B. Lukomska, T. R. Lunavat, S. L. N. Maas, H. Malhi, A. Marcilla, J. Mariani, J. Mariscal, E. S. Martens-Uzunova, L. Martin-Jaular, M. Carmen Martinez, V. R. Martins, M. Mathieu, S. Mathivanan, M. Maugeri, L. K. McGinnis, M. J. McVey, D. G. Meckes, K. L. Meehan, I. Mertens, V. R. Minciacchi, A. Möller, M. M. Jørgensen, A. Morales-Kastresana, J. Morhayim, F. Mullier, M. Muraca, L. Musante, V. Mussack, D. C. Muth, K. H. Myburgh, T. Najrana, M. Nawaz, I. Nazarenko, P. Nejsum, C. Neri, T. Neri, R. Nieuwland, L. Nimrichter, J. P. Nolan, E. N. M. N.-'t Hoen, N. N. Hooten, L. O'Driscoll, T. O'Grady, A. O'Loghlen, T. Ochiya, M. Olivier, A. Ortiz, L. A. Ortiz, X. Osteikoetxea, O. Østergaard, M. Ostrowski, J. Park, D. Michiel Pegtel, H. Peinado, F. Perut, M. W. Pfaffl, D. G. Phinney, B. C. H. Pieters, R. C. Pink, D. S. Pisetsky, E. P. von Strandmann, I. Polakovicova, I. K. H. Poon, B. H. Powell, I. Prada, L. Pulliam, P. Quesenberry, A. Radeghieri, R. L. Raffai, S. Raimondo, J. Rak, M. I. Ramirez, G. Raposo, M. S. Rayyan, N. Regev-Rudzki, F. L. Ricklefs, P. D. Robbins, D. D. Roberts, S. C. Rodrigues, E. Rohde, S. Rome, K. M. A. Rouschop, A. Rughetti, A. E. Russell, P. Saá, S. Sahoo, E. Salas-Huenuleo, C. Sánchez, J. A. Saugstad, M. J. Saul, R. M. Schiffelers, R. Schneider, T. H. Schøyen, A. Scott, E. Shahaj, S. Sharma, O. Shatnyeva, F. Shekari, G. V. Shelke, A. K. Shetty, K. Shiba, P. R.-M. Siljander, A. M. Silva, A. Skowronek, O. L. Snyder, R. P. Soares, B. W. Sódar, C. Soekmadji, J. Sotillo, P. D. Stahl, W. Stoorvogel, S. L. Stott, E. F. Strasser, S. Swift, H. Tahara, M. Tewari, K. Timms, S. Tiwari, R. Tixeira, M. Tkach, W. S. Toh, R. Tomasini, A. C. Torrecilhas, J. P. Tosar, V. Toxavidis, L. Urbanelli, P. Vader, B. W. M. van Balkom, S. G. van der Grein, J. Van Deun, M. J. C. van Herwijnen, K. Van Keuren-Jensen, G. van Niel, M. E. van Royen, A. J. van Wijnen, M. Helena Vasconcelos, I. J. Vechetti, T. D. Veit, L. J. Vella, É. Velot, F. J. Verweij, B. Vestad, J. L. Viñas, T. Visnovitz, K. V. Vukman, J. Wahlgren, D. C. Watson, M. H. M. Wauben, A. Weaver, J. P. Webber, V. Weber, A. M. Wehman, D. J. Weiss, J. A. Welsh, S. Wendt, A. M. Wheelock, Z. Wiener, L. Witte, J. Wolfram, A. Xagorari, P. Xander, J. Xu, X. Yan, M. Yáñez-Mó, H. Yin, Y. Yuana, V. Zappulli, J. Zarubova, V. Žėkas, J.-Y. Zhang, Z. Zhao, L. Zheng, A. R. Zheutlin, A. M. Zickler, P. Zimmermann, A. M. Zivkovic, D. Zocco and E. K. Zuba-Surma, J. Extracell. Vesicles, 2018, 7, 1535750 CrossRef PubMed.
- X. Fan, Y. Zhang, W. Liu, M. Shao, Y. Gong, T. Wang, S. Xue and R. Nian, Biomater. Sci., 2024, 12, 3500–3521 RSC.
- R. Sakthiswary and A. A. Raymond, Neural Regen. Res., 2012, 7, 1822–1831 Search PubMed.
- N. Fayazi, M. Sheykhhasan, S. Soleimani Asl and R. Najafi, Mol. Neurobiol., 2021, 58, 3494–3514 CrossRef CAS PubMed.
- C. M. Trigo, J. S. Rodrigues, S. P. Camões, S. Solá and J. P. Miranda, J. Adv. Res., 2024 DOI:10.1016/j.jare.2024.05.004.
- A. G. Thompson, E. Gray, S. M. Heman-Ackah, I. Mäger, K. Talbot, S. E. Andaloussi, M. J. Wood and M. R. Turner, Nat. Rev. Neurol., 2016, 12, 346–357 CrossRef CAS PubMed.
- F. U. Rehman, Y. Liu, M. Zheng and B. Shi, Biomaterials, 2023, 293, 121949 CrossRef CAS PubMed.
- H. Xin, Y. Li, Y. Cui, J. J. Yang, Z. G. Zhang and M. Chopp, J. Cereb. Blood Flow Metab., 2013, 33, 1711–1715 CrossRef CAS PubMed.
- X.-M. Zhang, J. Huang, X.-Y. Ni, H.-R. Zhu, Z.-X. Huang, S. Ding, X.-Y. Yang, Y.-D. Tan, J.-F. Chen and J.-H. Cai, Eur. J. Med. Res., 2024, 29, 15 CrossRef PubMed.
- R. Zhao, X. Chen, H. Song, Q. Bie and B. Zhang, Stem Cells Int., 2020, 2020, 8844730 Search PubMed.
- H. Xu, G. Zhao, Y. Zhang, H. Jiang, W. Wang, D. Zhao, J. Hong, H. Yu and L. Qi, Stem Cell Res. Ther., 2019, 10, 381 CrossRef CAS PubMed.
- E. Ghasempour, S. Hesami, E. Movahed, S. H. Keshel and M. Doroudian, Stem Cell Res. Ther., 2022, 13, 527 CrossRef CAS PubMed.
- X. Hao, S. Wang, L. Wang, J. Li, Y. Li and J. Liu, J. Nanobiotechnol., 2024, 22, 340 CrossRef PubMed.
- S. N. Mousavikia, L. Darvish, M. T. Bahreyni Toossi and H. Azimian, Life Sci., 2024, 350, 122743 CrossRef CAS PubMed.
- S. Yang, Y. Sun, W. Liu, Y. Zhang, G. Sun, B. Xiang and J. Yang, Cancers (Basel), 2024, 16, 823 CrossRef CAS PubMed.
- H. Xin, M. Katakowski, F. Wang, J.-Y. Qian, X. S. Liu, M. M. Ali, B. Buller, Z. G. Zhang and M. Chopp, Stroke, 2017, 48, 747–753 CrossRef CAS PubMed.
- H. Xu, Z. Jia, K. Ma, J. Zhang, C. Dai, Z. Yao, W. Deng, J. Su, R. Wang and X. Chen, Med. Sci. Monit., 2020, 26, e920855 CAS.
- M. Ding, Y. Shen, P. Wang, Z. Xie, S. Xu, Z. Zhu, Y. Wang, Y. Lyu, D. Wang, L. Xu, J. Bi and H. Yang, Neurochem. Res., 2018, 43, 2165–2177 CrossRef CAS PubMed.
- L. Otero-Ortega, F. Laso-García, M. Gómez-de Frutos, B. Fuentes, L. Diekhorst, E. Díez-Tejedor and M. Gutiérrez-Fernández, Transl. Stroke Res., 2019, 10, 241–249 CrossRef CAS PubMed.
- F. Seyedaghamiri, L. Salimi, D. Ghaznavi, E. Sokullu and R. Rahbarghazi, Cell Commun. Signal., 2022, 20, 110 CrossRef PubMed.
- W. Yin, H. Ma, Y. Qu, J. Ren, Y. Sun, Z.-N. Guo and Y. Yang, Neural Regen. Res., 2024, 20, 1221–1235 CrossRef PubMed.
- O. Y. Bang and E. H. Kim, Front. Neurol., 2019, 10, 211 CrossRef PubMed.
- H. Xin, Y. Li, Z. Liu, X. Wang, X. Shang, Y. Cui, Z. G. Zhang and M. Chopp, Stem Cells, 2013, 31, 2737–2746 CrossRef PubMed.
- H. Xin, F. Wang, Y. Li, Q.-E. Lu, W. L. Cheung, Y. Zhang, Z. G. Zhang and M. Chopp, Cell Transplant., 2017, 26, 243–257 Search PubMed.
- H. Xin, Z. Liu, B. Buller, Y. Li, W. Golembieski, X. Gan, F. Wang, M. Lu, M. M. Ali, Z. G. Zhang and M. Chopp, J. Cereb. Blood Flow Metab., 2021, 41, 1131–1144 CrossRef PubMed.
- C. Li, K. Fei, F. Tian, C. Gao and S. Yang, Croat. Med. J., 2019, 60, 439–448 CrossRef PubMed.
- Y. Kuang, X. Zheng, L. Zhang, X. Ai, V. Venkataramani, E. Kilic, D. M. Hermann, A. Majid, M. Bähr and T. R. Doeppner, J. Extracell. Vesicles, 2020, 10, e12024 CrossRef PubMed.
- X. Li, T. Bi and S. Yang, Bioengineered, 2022, 13, 3030–3043 Search PubMed.
- Z. Zhang, X. Zou, R. Zhang, Y. Xie, Z. Feng, F. Li, J. Han, H. Sun, Q. Ouyang, S. Hua, B. Lv, T. Hua, Z. Liu, Y. Cai, Y. Zou, Y. Tang and X. Jiang, Aging, 2021, 13, 3060–3079 CrossRef PubMed.
- C. Cheng, X. Chen, Y. Wang, W. Cheng, X. Zuo, W. Tang and W. Huang, Mol. Med., 2021, 27, 67 Search PubMed.
- M. Jiang, H. Wang, M. Jin, X. Yang, H. Ji, Y. Jiang, H. Zhang, F. Wu, G. Wu, X. Lai, L. Cai, R. Hu, L. Xu and L. Li, Cell. Physiol. Biochem., 2018, 47, 864–878 CrossRef PubMed.
- Y. Zhao, Y. Gan, G. Xu, K. Hua and D. Liu, Life Sci., 2020, 260, 118403 CrossRef PubMed.
- W. Geng, H. Tang, S. Luo, Y. Lv, D. Liang, X. Kang and W. Hong, Am. J. Transl. Res., 2019, 11, 780–792 Search PubMed.
- Y. Deng, D. Chen, F. Gao, H. Lv, G. Zhang, X. Sun, L. Liu, D. Mo, N. Ma, L. Song, X. Huo, T. Yan, J. Zhang and Z. Miao, J. Biol. Eng., 2019, 13, 1–18 CrossRef PubMed.
- H. Hu, X. Hu, L. Li, Y. Fang, Y. Yang, J. Gu, J. Xu and L. Chu, Biomolecules, 2022, 12, 883 CrossRef PubMed.
- L.-X. Xue, L.-Y. Shu, H.-M. Wang, K.-L. Lu, L.-G. Huang, J.-Y. Xiang, Z. Geng, Y.-W. Zhao and H. Chen, Neural Regener. Res., 2023, 18, 1983–1989 Search PubMed.
- Y. Zhang, J. Liu, M. Su, X. Wang and C. Xie, Stem Cell Res. Ther., 2021, 12, 111 CrossRef PubMed.
- Y. Xiao, F. Geng, G. Wang, X. Li, J. Zhu and W. Zhu, J. Cell. Biochem., 2019, 120, 2109–2118 CrossRef PubMed.
- C. Liu, T.-H. Yang, H.-D. Li, G.-Z. Li, J. Liang and P. Wang, Neural Regen. Res., 2023, 18, 2246–2251 CrossRef PubMed.
- C. Gu, Y. Li, J. Liu, S. Liu, J. Long, Q. Zhang, W. Duan, T. Feng, J. Huang, Y. Qiu, W. Ahmed, H. Cai, Y. Hu, Y. Wu and L. Chen, Exp. Neurol., 2023, 370, 114547 CrossRef PubMed.
- Z. Li, Z. Chen and J. Peng, J. Neurogenet., 2023, 37, 103–114 CrossRef PubMed.
- H. Luo, G. Ye, Y. Liu, D. Huang, Q. Luo, W. Chen and Z. Qi, Neurosci. Lett., 2022, 779, 136635 CrossRef PubMed.
- Z.-H. Zhu, F. Jia, W. Ahmed, G.-L. Zhang, H. Wang, C.-Q. Lin, W.-H. Chen and L.-K. Chen, Neural Regen. Res., 2023, 18, 404–409 Search PubMed.
- S. E. Spellicy, E. E. Kaiser, M. M. Bowler, B. J. Jurgielewicz, R. L. Webb, F. D. West and S. L. Stice, Transl. Stroke Res., 2020, 11, 776–788 Search PubMed.
- Y. Xiong, A. Mahmood and M. Chopp, Neural Regen. Res., 2024, 19, 49–54 Search PubMed.
- Y. Y. Mot, E. J. Moses, N. Mohd Yusoff, K.-H. Ling, Y. K. Yong and J. J. Tan, Cell. Mol. Neurobiol., 2023, 43, 469–489 Search PubMed.
- O. Beylerli, R. Tamrazov, I. Gareev, T. Ilyasova, A. Shumadalova, Y. Bai and B. Yang, Non-coding RNA Res., 2023, 8, 686–692 CrossRef PubMed.
- Y. Zhang, Z. Zheng, J. Sun, S. Xu, Y. Wei, X. Ding and G. Ding, Histol. Histopathol., 2024, 39, 1109–1131 CAS.
- Y. Yang, Y. Ye, C. Kong, X. Su, X. Zhang, W. Bai and X. He, Neurochem. Res., 2019, 44, 811–828 CrossRef CAS PubMed.
- Y. Zhang, Y. Zhang, M. Chopp, H. Pang, Z. G. Zhang, A. Mahmood and Y. Xiong, J. Neurotrauma, 2021, 38, 1535–1550 CrossRef PubMed.
- S. Shivani, IP Int. J. Compr. Adv. Pharmacol., 2021, 6, 108–116 CrossRef.
- J. Chang, Z. Feng, Y. Li, H. Lv, S. Liu, Y. Luo, N. Hao, L. Zhao and J. Liu, Biochem. Pharmacol., 2024, 222, 116064 CrossRef CAS PubMed.
- S. Liu, M. Fan, J.-X. Xu, L.-J. Yang, C.-C. Qi, Q.-R. Xia and J.-F. Ge, J. Neuroinflammation, 2022, 19, 35 CrossRef CAS PubMed.
- S. Sha, X. Shen, Y. Cao and L. Qu, Aging, 2021, 13, 15285–15306 CrossRef CAS PubMed.
- L. Dehghani, A. Khojasteh, M. Soleimani, S. Oraee-Yazdani, S. H. Keshel, M. Saadatnia, M. Saboori, A. Zali, S. M. Hashemi and R. Soleimani, Int. J. Prev. Med., 2022, 13, 7 CrossRef PubMed.
- X. Xie, Q. Song, C. Dai, S. Cui, R. Tang, S. Li, J. Chang, P. Li, J. Wang, J. Li, C. Gao, H. Chen, S. Chen, R. Ren, X. Gao and G. Wang, Gen. Psychiatry, 2023, 36, e101143 CrossRef PubMed.
- A. Y. L. Wang, Int. J. Mol. Sci., 2021, 22, 1769 CrossRef CAS PubMed.
- R. R. Mizenko, M. Feaver, B. T. Bozkurt, N. Lowe, B. Nguyen, K.-W. Huang, A. Wang and R. P. Carney, J. Extracell. Vesicles, 2024, 13, e12510 CrossRef PubMed.
- D. Sun, X. Zhuang, X. Xiang, Y. Liu, S. Zhang, C. Liu, S. Barnes, W. Grizzle, D. Miller and H.-G. Zhang, Mol. Ther., 2010, 18, 1606–1614 CrossRef CAS PubMed.
- L. Alvarez-Erviti, Y. Seow, H. Yin, C. Betts, S. Lakhal and M. J. A. Wood, Nat. Biotechnol., 2011, 29, 341–345 CrossRef CAS PubMed.
- X. Zhuang, X. Xiang, W. Grizzle, D. Sun, S. Zhang, R. C. Axtell, S. Ju, J. Mu, L. Zhang, L. Steinman, D. Miller and H.-G. Zhang, Mol. Ther., 2011, 19, 1769–1779 CrossRef CAS PubMed.
- J. M. Cooper, P. B. O. Wiklander, J. Z. Nordin, R. Al-Shawi, M. J. Wood, M. Vithlani, A. H. V. Schapira, J. P. Simons, S. El-Andaloussi and L. Alvarez-Erviti, Mov. Disord., 2014, 29, 1476–1485 CrossRef CAS PubMed.
- M. J. Haney, N. L. Klyachko, Y. Zhao, R. Gupta, E. G. Plotnikova, Z. He, T. Patel, A. Piroyan, M. Sokolsky, A. V. Kabanov and E. V. Batrakova, J. Controlled Release, 2015, 207, 18–30 CrossRef CAS PubMed.
- M.-C. Didiot, L. M. Hall, A. H. Coles, R. A. Haraszti, B. M. Godinho, K. Chase, E. Sapp, S. Ly, J. F. Alterman, M. R. Hassler, D. Echeverria, L. Raj, D. V. Morrissey, M. DiFiglia, N. Aronin and A. Khvorova, Mol. Ther., 2016, 24, 1836–1847 CrossRef CAS PubMed.
- M. Qu, Q. Lin, L. Huang, Y. Fu, L. Wang, S. He, Y. Fu, S. Yang, Z. Zhang, L. Zhang and X. Sun, J. Controlled Release, 2018, 287, 156–166 CrossRef CAS PubMed.
- J. Nonnekes, M. H. M. Timmer, N. M. de Vries, O. Rascol, R. C. Helmich and B. R. Bloem, Mov. Disord., 2016, 31, 1602–1609 CrossRef CAS PubMed.
- M. Izadpanah, L. Dargahi, J. Ai, A. Asgari Taei, S. Ebrahimi Barough, S. J. Mowla, G. TavoosiDana and M. Farahmandfar, Iran. J. Pharm. Res., 2020, 19, 45–60 CAS.
- L. Zhang, T. Wu, Y. Shan, G. Li, X. Ni, X. Chen, X. Hu, L. Lin, Y. Li, Y. Guan, J. Gao, D. Chen, Y. Zhang, Z. Pei and X. Chen, Brain, 2021, 144, 3421–3435 CrossRef PubMed.
- D. Yuan, Y. Zhao, W. A. Banks, K. M. Bullock, M. Haney, E. Batrakova and A. V. Kabanov, Biomaterials, 2017, 142, 1–12 CrossRef CAS PubMed.
- L. Yang, B. Han, Z. Zhang, S. Wang, Y. Bai, Y. Zhang, Y. Tang, L. Du, L. Xu, F. Wu, L. Zuo, X. Chen, Y. Lin, K. Liu, Q. Ye, B. Chen, B. Li, T. Tang, Y. Wang, L. Shen, G. Wang, M. Ju, M. Yuan, W. Jiang, J. H. Zhang, G. Hu, J. Wang and H. Yao, Circulation, 2020, 142, 556–574 CrossRef CAS PubMed.
- T. Driedonks, L. Jiang, B. Carlson, Z. Han, G. Liu, S. E. Queen, E. N. Shirk, O. Gololobova, Z. Liao, L. H. Nyberg, G. Lima, L. Paniushkina, M. Garcia-Contreras, K. Schonvisky, N. Castell, M. Stover, S. Guerrero-Martin, R. Richardson, B. Smith, V. Machairaki, C. P. Lai, J. M. Izzi, E. K. Hutchinson, K. A. M. Pate and K. W. Witwer, J. Extracell. Biol., 2022, 1, e59 CrossRef CAS PubMed.
- F. Shahjin, S. Chand and S. V. Yelamanchili, J. Neuroimmune Pharmacol., 2020, 15, 443–458 CrossRef PubMed.
- A. A. Shaimardanova, V. V. Solovyeva, D. S. Chulpanova, V. James, K. V. Kitaeva and A. A. Rizvanov, Neural Regen. Res., 2020, 15, 586–596 CrossRef CAS PubMed.
- S. U. Khan, M. I. Khan, M. U. Khan, N. M. Khan, S. Bungau and S. S. U. Hassan, Bioengineering (Basel), 2022, 10, 51 CrossRef PubMed.
- X. Zhao, P. Ge, S. Lei, S. Guo, P. Zhou, L. Zhao, Y. Qi, X. Wei, W. Wu, N. Wang, R. Guo, N. Yang, Q. Xiao, Q. Zhang and H. Zhu, Drug Des., Dev. Ther., 2023, 17, 2401–2420 CrossRef CAS PubMed.
- A. Iyaswamy, A. Thakur, X.-J. Guan, S. Krishnamoorthi, T. Y. Fung, K. Lu, I. Gaurav, Z. Yang, C.-F. Su, K.-F. Lau, K. Zhang, R. C.-L. Ng, Q. Lian, K.-H. Cheung, K. Ye, H. J. Chen and M. Li, Signal Transduct. Target. Ther., 2023, 8, 404 CrossRef CAS PubMed.
- T. Richards, J. C. Perron, K. Patel, J. Wurpel, S. E. Reznik and F. Schanne, Res. Sq., 2023 DOI:10.21203/rs.3.rs-3399248/v1.
- E.-Y. Lin, S.-X. Hsu, B.-H. Wu, Y.-C. Deng, W. Wuli, Y.-S. Li, J.-H. Lee, S.-Z. Lin, H.-J. Harn and T.-W. Chiou, Int. J. Nanomed., 2024, 19, 4977–4994 CrossRef PubMed.
- Y. Geng, X. Long, Y. Zhang, Y. Wang, G. You, W. Guo, G. Zhuang, Y. Zhang, X. Cheng, Z. Yuan and J. Zan, J. Transl. Med., 2023, 21, 652 CrossRef CAS PubMed.
- F. Aliakbari, K. Marzookian, S. Parsafar, H. Hourfar, Z. Nayeri, A. Fattahi, M. Raeiji, N. N. Boroujeni, D. E. Otzen and D. Morshedi, Sci. Adv., 2024, 10, eadl3406 CrossRef CAS PubMed.
- P. Jiang, Y. Xiao, X. Hu, C. Wang, H. Gao, H. Huang, J. Lv, Z. Qi and Z. Wang, ACS Biomater. Sci. Eng., 2024, 10, 3069–3085 CrossRef CAS PubMed.
- H. Zhang, X.-H. Xie, S.-X. Xu, C. Wang, S. Sun, X. Song, R. Li, N. Li, Y. Feng, H. Duan, D. Li and Z. Liu, CNS Neurosci. Ther., 2024, 30, e14661 CrossRef CAS PubMed.
- Y. Li, Z. Chen, J. Guo, D. Meng, X. Pang, Z. Sun, L. Pu, S. Yang, M. Yang and Y. Peng, Biochem. Biophys. Res. Commun., 2024, 725, 150260 CrossRef CAS PubMed.
- H.-M. Liu and Y. Zhang, Kaohsiung J. Med. Sci., 2024, 40, 435–444 CrossRef CAS PubMed.
- R. Wang, X. Wang, H. Zhao, N. Li, J. Li, H. Zhang and L. Di, J. Controlled Release, 2024, 365, 331–347 CrossRef CAS PubMed.
- Y. Wang, Y. Huo, C. Zhao, H. Liu, Y. Shao, C. Zhu, L. An, X. Chen and Z. Chen, J. Controlled Release, 2024, 368, 170–183 CrossRef CAS PubMed.
- J. Zhao, X. Cui, Q. Zhan, K. Zhang, D. Su, S. Yang, B. Hong, Q. Wang, J. Ju, C. Cheng, C. Li, C. Wan, Y. Wang, J. Zhou and C. Kang, Theranostics, 2024, 14, 2835–2855 CrossRef CAS PubMed.
- J. Jiang, Y. Lu, J. Chu, X. Zhang, C. Xu, S. Liu, Z. Wan, J. Wang, L. Zhang, K. Liu, Z. Liu, A. Yang, X. Ren and R. Zhang, J. Nanobiotechnol., 2024, 22, 159 CrossRef CAS PubMed.
- B. Tao, R. Du, X. Zhang, B. Jia, Y. Gao, Y. Zhao and Y. Liu, J. Controlled Release, 2023, 363, 692–706 CrossRef CAS PubMed.
- L.-B. Wang, B.-Y. Liao, Y.-J. Li, Z.-H. Wang, Y. Yu, X. Li and Q.-H. Zhang, Exp. Neurol., 2024, 375, 114730 CrossRef CAS PubMed.
- Z. Tang, S. Hu, Z. Wu and M. He, Mol. Med., 2023, 29, 96 CAS.
- D. S. Knopman, H. Amieva, R. C. Petersen, G. Chételat, D. M. Holtzman, B. T. Hyman, R. A. Nixon and D. T. Jones, Nat. Rev. Dis. Primers, 2021, 7, 33 CrossRef PubMed.
- K. Nishimura, J. Sanchez-Molano, N. Kerr, Y. Pressman, R. Silvera, A. Khan, S. Gajavelli, H. M. Bramlett and W. D. Dietrich, J. Neurotrauma, 2024, 41, 2395–2412 CrossRef PubMed.
- S. Jiang, G. Cai, Z. Yang, H. Shi, H. Zeng, Q. Ye, Z. Hu and Z. Wang, ACS Nano, 2024, 18, 11753–11768 CrossRef CAS PubMed.
- W. Poewe, K. Seppi, C. M. Tanner, G. M. Halliday, P. Brundin, J. Volkmann, A.-E. Schrag and A. E. Lang, Nat. Rev. Dis. Primers, 2017, 3, 17013 CrossRef PubMed.
- M. J. Armstrong and M. S. Okun, J. Am. Med. Assoc., 2020, 323, 548–560 CrossRef PubMed.
- H. R. Morris, M. G. Spillantini, C. M. Sue and C. H. Williams-Gray, Lancet, 2024, 403, 293–304 CrossRef CAS PubMed.
- W. Marx, B. W. J. H. Penninx, M. Solmi, T. A. Furukawa, J. Firth, A. F. Carvalho and M. Berk, Nat. Rev. Dis. Primers, 2023, 9, 44 CrossRef PubMed.
- T. Toda, S. L. Parylak, S. B. Linker and F. H. Gage, Mol. Psychiatry, 2019, 24, 67–87 CrossRef CAS PubMed.
- S. E. Holmes, D. Scheinost, S. J. Finnema, M. Naganawa, M. T. Davis, N. DellaGioia, N. Nabulsi, D. Matuskey, G. A. Angarita, R. H. Pietrzak, R. S. Duman, G. Sanacora, J. H. Krystal, R. E. Carson and I. Esterlis, Nat. Commun., 2019, 10, 1529 CrossRef PubMed.
- J. Peng, M. Fan, C. An, F. Ni, W. Huang and J. Luo, Basic Clin. Pharmacol. Toxicol., 2022, 130, 439–456 CrossRef CAS PubMed.
- M. A. Huestis, R. Solimini, S. Pichini, R. Pacifici, J. Carlier and F. P. Busardò, Curr. Neuropharmacol., 2019, 17, 974–989 CrossRef CAS PubMed.
- M. Weller, P. Y. Wen, S. M. Chang, L. Dirven, M. Lim, M. Monje and G. Reifenberger, Nat. Rev. Dis. Primers, 2024, 10, 33 CrossRef PubMed.
- R. Chen, M. Smith-Cohn, A. L. Cohen and H. Colman, Neurotherapeutics, 2017, 14, 284–297 CrossRef PubMed.
- M. S. Tomar, A. Kumar, C. Srivastava and A. Shrivastava, Biochim. Biophys. Acta, Rev. Cancer, 2021, 1876, 188616 CrossRef CAS PubMed.
- X. Chen, J. Guo, J. Bao, J. Lu and Y. Wang, Med. Res. Rev., 2014, 34, 768–794 CrossRef CAS PubMed.
- H.-G. Wirsching, E. Galanis and M. Weller, Handb. Clin. Neurol., 2016, 134, 381–397 Search PubMed.
- A. S. Achrol, R. C. Rennert, C. Anders, R. Soffietti, M. S. Ahluwalia, L. Nayak, S. Peters, N. D. Arvold, G. R. Harsh, P. S. Steeg and S. D. Chang, Nat. Rev. Dis. Primers, 2019, 5, 5 CrossRef PubMed.
- R. Dobson and G. Giovannoni, Eur. J. Neurol., 2019, 26, 27–40 CrossRef CAS PubMed.
- S. L. Hauser and B. A. C. Cree, Am. J. Med., 2020, 133, 1380–1390 CrossRef CAS PubMed.
- T. Ishida, K. Kawada, K. Jobu, S. Morisawa, T. Kawazoe, S. Nishimura, K. Akagaki, S. Yoshioka and M. Miyamura, J. Pharm. Pharmacol., 2023, 75, 1322–1331 CrossRef PubMed.
- J. Yang, S. Wu, L. Hou, D. Zhu, S. Yin, G. Yang and Y. Wang, Mol. Ther. Nucleic Acids, 2020, 21, 512–522 CrossRef CAS PubMed.
- D. Rufino-Ramos, K. Leandro, P. R. L. Perdigão, K. O'Brien, M. M. Pinto, M. M. Santana, T. S. van Solinge, S. Mahjoum, X. O. Breakefield, K. Breyne and L. Pereira de Almeida, Mol. Ther., 2023, 31, 2220–2239 CrossRef CAS PubMed.
- W. Gu, S. Luozhong, S. Cai, K. Londhe, N. Elkasri, R. Hawkins, Z. Yuan, K. Su-Greene, Y. Yin, M. Cruz, Y.-W. Chang, P. McMullen, C. Wu, C. Seo, A. Guru, W. Gao, T. Sarmiento, C. Schaffer, N. Nishimura, R. Cerione, Q. Yu, M. Warden, R. Langer and S. Jiang, Nat. Biomed. Eng., 2024, 8, 415–426 CrossRef CAS PubMed.
- E. R. Abels, S. L. N. Maas, L. Nieland, Z. Wei, P. S. Cheah, E. Tai, C.-J. Kolsteeg, S. A. Dusoswa, D. T. Ting, S. Hickman, J. El Khoury, A. M. Krichevsky, M. L. D. Broekman and X. O. Breakefield, Cell Rep., 2019, 28, 3105–3119 CrossRef CAS PubMed.
- C. P. Lai, E. Y. Kim, C. E. Badr, R. Weissleder, T. R. Mempel, B. A. Tannous and X. O. Breakefield, Nat. Commun., 2015, 6, 7029 CrossRef CAS PubMed.
- C. P. Lai, O. Mardini, M. Ericsson, S. Prabhakar, C. Maguire, J. W. Chen, B. A. Tannous and X. O. Breakefield, ACS Nano, 2014, 8, 483–494 CrossRef PubMed.
- T. C. Pham, M. K. Jayasinghe, T. T. Pham, Y. Yang, L. Wei, W. M. Usman, H. Chen, M. Pirisinu, J. Gong, S. Kim, B. Peng, W. Wang, C. Chan, V. Ma, N. T. H. Nguyen, D. Kappei, X.-H. Nguyen, W. C. Cho, J. Shi and M. T. N. Le, J. Extracell. Vesicles, 2021, 10, e12057 CrossRef PubMed.
- S. A. A. Kooijmans, J. J. J. M. Gitz-Francois, R. M. Schiffelers and P. Vader, Nanoscale, 2018, 10, 2413–2426 RSC.
- B. J. Tauro, D. W. Greening, R. A. Mathias, H. Ji, S. Mathivanan, A. M. Scott and R. J. Simpson, Methods, 2012, 56, 293–304 CrossRef PubMed.
- S. Bathini, S. Pakkiriswami, R. J. Ouellette, A. Ghosh and M. Packirisamy, Biosens. Bioelectron., 2021, 194, 113585 CrossRef PubMed.
- Y. Chen, Q. Zhu, L. Cheng, Y. Wang, M. Li, Q. Yang, L. Hu, D. Lou, J. Li, X. Dong, L. P. Lee and F. Liu, Nat. Methods, 2021, 18, 212–218 CrossRef PubMed.
- R. Kalluri and V. S. LeBleu, Science, 2020, 367, eaau6977 CrossRef PubMed.
- Q. Pan, Z. Bao, Y. Wang and T. Wan, Chem. Eng. J., 2024, 480, 148282 CrossRef.
- B. Wang, X. Zhuang, Z.-B. Deng, H. Jiang, J. Mu, Q. Wang, X. Xiang, H. Guo, L. Zhang, G. Dryden, J. Yan, D. Miller and H.-G. Zhang, Mol. Ther., 2014, 22, 522–534 CrossRef PubMed.
- C. Marianecci and M. Carafa, Pharmaceutics, 2019, 11, 147 CrossRef PubMed.
- S. Salunkhe, Dheeraj, M. Basak, D. Chitkara and A. Mittal, J. Controlled Release, 2020, 326, 599–614 CrossRef PubMed.
- T. O. Pangburn, M. A. Petersen, B. Waybrant, M. M. Adil and E. Kokkoli, J. Biomech. Eng., 2009, 131, 074005 CrossRef PubMed.
- R. C. Ladner, A. K. Sato, J. Gorzelany and M. de Souza, Drug Discovery Today, 2004, 9, 525–529 CrossRef CAS PubMed.
- Y. Tian, S. Li, J. Song, T. Ji, M. Zhu, G. J. Anderson, J. Wei and G. Nie, Biomaterials, 2014, 35, 2383–2390 CrossRef PubMed.
- C. Villa, V. Secchi, M. Macchi, L. Tripodi, E. Trombetta, D. Zambroni, F. Padelli, M. Mauri, M. Molinaro, R. Oddone, A. Farini, A. De Palma, L. Varela Pinzon, F. Santarelli, R. Simonutti, P. Mauri, L. Porretti, M. Campione, D. Aquino, A. Monguzzi and Y. Torrente, Nat. Nanotechnol., 2024, 19, 1532–1543 CrossRef PubMed.
- S. Liu, X. Chen, L. Bao, T. Liu, P. Yuan, X. Yang, X. Qiu, J. J. Gooding, Y. Bai, J. Xiao, F. Pu and Y. Jin, Nat. Biomed. Eng., 2020, 4, 1063–1075 CrossRef PubMed.
- Z.-W. Luo, Y.-Y. Sun, J.-R. Lin, B.-J. Qi and J.-W. Chen, World J. Stem Cells, 2021, 13, 1762–1782 CrossRef PubMed.
- A. Forterre, A. Jalabert, K. Chikh, S. Pesenti, V. Euthine, A. Granjon, E. Errazuriz, E. Lefai, H. Vidal and S. Rome, Cell Cycle, 2014, 13, 78–89 CrossRef PubMed.
- A. Forterre, A. Jalabert, E. Berger, M. Baudet, K. Chikh, E. Errazuriz, J. De Larichaudy, S. Chanon, M. Weiss-Gayet, A.-M. Hesse, M. Record, A. Geloen, E. Lefai, H. Vidal, Y. Couté and S. Rome, PLoS One, 2014, 9, e84153 CrossRef PubMed.
- S. Jing, H. Li and H. Xu, Int. J. Nanomed., 2023, 18, 2707–2720 CrossRef PubMed.
- J. Li, Z.-P. Liu, C. Xu and A. Guo, Regen. Ther., 2020, 15, 70–76 CrossRef PubMed.
- R. Wan, A. Hussain, A. Behfar, S. L. Moran and C. Zhao, Int. J. Mol. Sci., 2022, 23, 3869 CrossRef PubMed.
- L. Shao, Y. Zhang, B. Lan, J. Wang, Z. Zhang, L. Zhang, P. Xiao, Q. Meng, Y.-J. Geng, X.-Y. Yu and Y. Li, Biomed Res. Int., 2017, 2017, 4150705 Search PubMed.
- L. Zhang, G. Jiao, S. Ren, X. Zhang, C. Li, W. Wu, H. Wang, H. Liu, H. Zhou and Y. Chen, Stem Cell Res. Ther., 2020, 11, 38 CrossRef PubMed.
Footnote |
† These authors contributed equally to this work. |
|
This journal is © The Royal Society of Chemistry 2025 |
Click here to see how this site uses Cookies. View our privacy policy here.