DOI:
10.1039/D4CC05167J
(Feature Article)
Chem. Commun., 2025,
61, 3093-3108
Advances in magnetic nanoparticles for molecular medicine†
Received
1st October 2024
, Accepted 20th January 2025
First published on 20th January 2025
Abstract
Magnetic nanoparticles (MNPs) are highly versatile nanomaterials in nanomedicine, owing to their diverse magnetic properties, which can be tailored through variations in size, shape, composition, and exposure to inductive magnetic fields. Over four decades of research have led to the clinical approval or ongoing trials of several MNP formulations, fueling continued innovation. Beyond traditional applications in drug delivery, imaging, and cancer hyperthermia, MNPs have increasingly advanced into molecular medicine. Under external magnetic fields, MNPs can generate mechano- or thermal stimuli to modulate individual molecules or cells deep within tissue, offering precise, remote control of biological processes at cellular and molecular levels. These unique capabilities have opened new avenues in emerging fields such as genome editing, cell therapies, and neuroscience, underpinned by a growing understanding of nanomagnetism and the molecular mechanisms responding to mechanical and thermal cues. Research on MNPs as a versatile synthetic material capable of engineering control at the cellular and molecular levels holds great promise for advancing the frontiers of molecular medicine, including areas such as genome editing and synthetic biology. This review summarizes recent clinical studies showcasing the classical applications of MNPs and explores their integration into molecular medicine, with the goal of inspiring the development of next-generation MNP-based platforms for disease treatment.

Xiaoyue Yang
| Dr Xiaoyue Yang earned her PhD in pharmacological sciences from Zhejiang University in 2020. Following her graduation, she joined the Nanomedicine and Immunoengineering Lab in the Department of Biomedical Engineering at the University of Kentucky, where she currently serves as a postdoctoral scholar. |

Sarah E. Kubican
| Sarah Kubican obtained her BS in Engineering from Miami University of Ohio in 2023. She went on to join the Nanomedicine and Immunoengineering Lab in pursuit of her MS in Biomedical Engineering from University of Kentucky. She expects to complete her thesis research and graduate by May 2025. |

Zhongchao Yi
| Zhongchao Yi received his BS in Functional Materials from Chongqing University of Science and Technology in 2018 and went on to earn his MS in Bioengineering from Rice University in 2020. He is currently pursuing his PhD in the Department of Biomedical Engineering at the University of Kentucky, with plans to complete his thesis research and graduate by December 2024. |
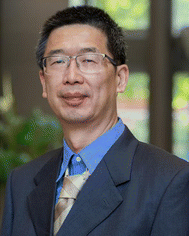
Sheng Tong
| Prof. Sheng Tong received his BS and MS degrees in Mechanical Engineering from the University of Science and Technology of China and Peking University, respectively. He completed his PhD in Biomedical Engineering from Duke University and subsequently pursued two years of postdoctoral training at the University of California, San Diego. Currently, he is an Associate Professor in the Department of Biomedical Engineering at the University of Kentucky. His research focuses on studying the interactions of nanomaterials with biological systems and developing therapeutic strategies centered on gene editing and immune engineering. |
Introduction
Magnetic nanoparticles (MNPs), composed of crystals of magnetic metal elements or metal oxides, possess unique nanoscale magnetic properties, such as superparamagnetism, high magnetization, and rapid relaxation in the presence of external magnetic fields. With dimensions similar to biomolecules, MNPs can translate magnetic signals into mechanical or thermal stimuli targeted at specific cell surface receptors, structural proteins, or subcellular organelles. This capability enables remote control of biological processes at cellular and molecular levels, making MNPs invaluable for both research and therapeutic applications.
The exploration of MNPs in biomedical research dates back to the 1960s, when magnetic attraction of iron carbonyl macroparticles was first demonstrated in vivo using experimental dogs.1 This capacity for directing MNPs to specific tissues sparked numerous studies on magnetic targeting for drug and gene delivery. In parallel, MNPs were also identified as effective contrast agents in magnetic resonance imaging (MRI). Furthermore, the heat generated by MNPs under an alternating magnetic field (AMF) led to the development of deep tissue heating technology. Over the past four decades, targeted drug delivery, imaging, and thermal therapy have become the three primary areas of clinical research on MNPs.2 Many studies have culminated in the clinical approval of MNPs for tumor imaging and thermal ablation therapy (Table 1).
Table 1 Representative clinical applications and clinical trials of MNPs
|
Agent |
Condition |
Identifier |
Start date |
Status |
Abbreviations: MTC-DOX – magnetic targeted carriers absorbed with doxorubicin hydrochloride; HCEC – human corneal endothelial cell; NP – nanoparticle; MNP – magnetic nanoparticle; SPIO – superparamagnetic iron oxide particle; GI – gastrointestinal; USPIO – ultrasmall superparamagnetic iron oxide nanoparticle. |
Magnetic targeting |
Ferrofluids |
Various cancers |
— |
1996 |
Phase I |
MTC-DOX |
Liver cancer |
NCT00034333 |
2002 |
Phase II/III, terminated |
Silica–gold iron-bearing NP3,4 |
Atherosclerotic lesions |
NCT01270139 |
2007 |
Completed |
CD34 antibody labeled MNP5 |
Leukemia |
NCT01411904 |
2010 |
Withdrawn |
MNP-labeled HCECs6 |
Corneal edema |
NCT04894110 |
2021 |
Phase I |
Molecular imaging/cell tracking |
Endorem®7 |
Liver tumor |
— |
— |
1996 FDA approval, currently discontinued |
SPIO8 |
Melanoma |
NCT00243594 |
1999 |
Phase I/II, completed |
Resovist® |
Hepatic cancer |
NCT00307866 |
2001 |
Phase III, completed |
Resovist® |
Liver tumor |
— |
2001 |
EMA approval, currently discontinued |
Lumirem® |
GI track imaging |
— |
— |
1996 FDA approval, 2001 EMA approval, currently discontinued |
Ferumoxtran-10 |
Prostate cancer |
NCT00185029 |
2002 |
Phase IV |
Ferumoxtran-10 |
Breast cancer |
NCT00107484 |
2005 |
Phase II, completed |
Feridex®9 |
Multiple sclerosis and amyotrophic lateral sclerosis |
NCT00781872 |
2006 |
Phase I/II, completed |
Sinerem®10,11 |
Prostate and bladder cancer |
NCT00622973 |
2007 |
Phase III, completed |
USPIO |
Infectious and degenerative diseases of the spine |
NCT00372281 |
2007 |
Phase III |
Feraheme® |
Pancreatic cancer |
NCT00920023 |
2008 |
Phase IV, completed |
USPIO12 |
Aortic aneurysm instability |
NCT00794092 |
2008 |
Phase II/III, completed |
Ferumoxytol |
Brain tumor |
NCT00978562 |
2009 |
Phase I, completed |
Feraheme® |
Anemia associated with chronic renal failure, iron deficiency anemia |
— |
— |
2009 FDA approval |
SPIO13 |
Healthy volunteer |
NCT00972946 |
2009 |
Completed |
SPIO13 |
Healthy volunteer with induced inflammation in the forearm |
NCT01169935 |
2010 |
Completed |
Ferahem®14 |
Myocardial Infarction |
NCT01323296 |
2010 |
Completed |
Ferahem®15 |
Healthy volunteer |
NCT01244282 |
2010 |
Phase I, completed |
SPIO |
Myocardial infarction |
NCT01127113 |
2010 |
Withdrawn |
Thermal therapy |
NanoTherm® |
Glioblastoma multiforme |
— |
— |
2010 EMA approval |
MNPs |
Prostate cancer |
NCT02033447 |
2013 |
Phase I, completed |
Iron nanoparticles |
Prostate cancer |
NCT05010759 |
2021 |
Terminated |
NanoTherm® |
Recurrent glioblastoma multiforme |
NCT06271421 |
2024 |
Recruiting |
During the same period, the synthesis of MNPs has seen major advancements (Fig. 1). The first generation synthesis relied on the rapid coprecipitation of iron salts in aqueous buffers, producing large quantities of iron oxide nanocrystals with varied shapes and broad size distributions.16,17 One notable product of this method is ferumoxytol, a formulation that was first approved for clinical use in 2009 and remains a prominent candidate in ongoing clinical trials (Fig. 1(A)). A breakthrough in the field was the development of the thermal decomposition method for nanocrystal synthesis.18,19 In this process, organic iron salts are heated to high temperatures in non-coordinating solvents, allowing their gradual decomposition into Fe–O precursors. This method allows for greater control over nanocrystal size, shape, and composition – critical factors influencing nanoscale magnetic responses (Fig. 1(B) through Fig. 1(L)).20–26 Compared to coprecipitation, which typically produces nanocrystals smaller than 10 nanometers, thermal decomposition can yield highly uniform nanocrystals ranging from a few nanometers to several hundred nanometers. Consequently, this enhances both the magnetic moment and heat generation, enabling novel approaches for manipulating molecular or cellular targets.25,27–31 A detailed review of nanosynthesis and magnetic properties of MNPs can be found elsewhere.2,32,33
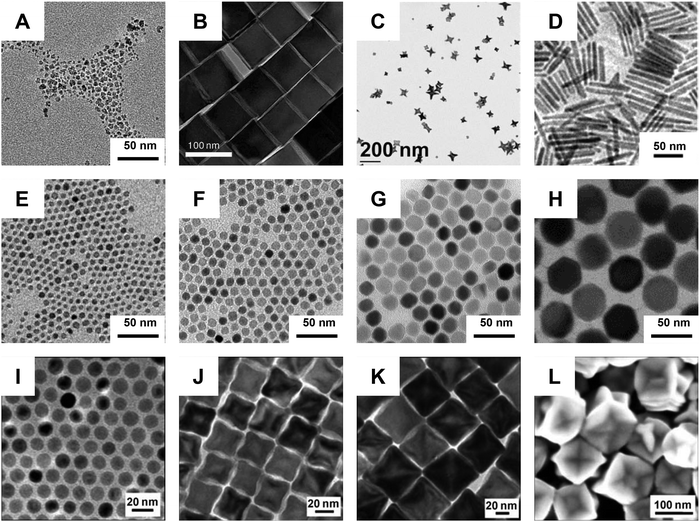 |
| Fig. 1 Synthesis of iron oxide nanoparticles. (A) Ferumoxytol synthesized via co-precipitation. (Reproduced with permission from ref. 34, ©2023 John Wiley and Sons). B through L are iron oxide nanoparticles synthesized via thermodecomposition. (B) Magnetite nanocubes. (Reproduced with permission from ref. 22, ©2009 American Chemical Society). (C) Magnetite nanostars. (Reproduced with permission from ref. 23, ©2011 American Chemical Society). (D) Magnetite nanorods. (Reproduced with permission from ref. 24, ©2012 American Chemical Society). (E)–(H). Magnetite nanoparticles from 6 to 40 nm. (Reproduced with permission from ref. 25, ©2011 American Chemical Society). (I)–(L). Wüstite nanoparticles from 14 to 100 nm. (Reproduced with permission from ref. 26, ©2007 Wiley-VCH Verlag GmbH & Co. KGaA, Weinheim). | |
In the last decade, a wide range of innovative applications leveraging MNP-mediated mechanical or thermal stimulation have emerged, with the potential to significantly transform future medical interventions. For instance, our study demonstrated that MNPs can enhance the cellular entry of an insect viral vector, offering a valuable platform for targeted in vivo gene editing.35 Other studies have shown that MNPs can mechanically stimulate cells, altering cytoskeleton, activating ion channels, and inducing cell polarization. Notably, Lee et al. used large clusters of MNPS (∼100 s nm) to modulate neuronal activity via the mechanosensitive ion channel, Piezo1.29,30 Interestingly, separate research have indicated that synthetic and biogenic MNPs tethered to thermosensitive ion channels, TRPs, can activate neurons through heating.34,36–39 Although the precise mechanisms are still not fully understood,40–42 these findings could lead to groundbreaking advances in brain modulation.
Another important discovery is the ability of MNPs to catalyze the generation of hydroxyl free radicals via a heterogeneous Fenton reaction.43–45 The free radicals are key intermediates in chemo- and radiation therapies. Compared to small-molecule chemotherapeutic reagents, MNPs have improved availability to diseased tissue due to a long circulation half-life and targeting capability. Therefore, MNPs can not only serve as MRI detectable, targeted drug carriers but also an effective adjuvant to other drug molecules, providing new treatment avenues. This finding also shed light on mitigating potential adverse effects of MNPs.
In this review, we will briefly summarize the clinical studies illustrating the classical applications of MNPs, followed by an exploration of cutting-edge research on their mechanical, thermal, and enzymatic effects in recent years. We will highlight the studies utilizing MNPs as a nanoscale force or heat generators for targeted modulation of biological processes, as these will pave the way for future precision medicine.
Current clinical investigations
MNPs are among the most extensively studied nanomaterials in clinical research and have received multiple approvals for medical use in both the US and Europe (Table 1, for a full list, see ESI,† Table S1). In clinical settings, only iron oxide nanoparticles, such as magnetite (Fe3O4) and maghemite (γ-Fe2O3), are used, owing to their well-established pharmacokinetic properties and biosafety profiles.46,47 Notably, ferumoxytol has been approved for the treatment of iron deficiency anemia, owing to its biocompatibility and biodegradability. While most clinical research has focused on cancer detection and treatment, recent studies have revealed the potential of MNPs in other disease conditions, including myocardial infarction (NCT01323296), atherosclerosis (NCT01270139), diabetes (NCT01521520), and retinal edema (NCT04894110). In this section, we summarize the key clinical applications of MNPs, emphasizing their magnetic force, imaging, and thermal properties.
Magnetic targeting
Magnetic nanoparticles (MNPs) were initially developed for magnetically targeting therapeutic agents to specific tissues. However, their clinical application has been complicated by the need to balance multiple factors essential for effective drug delivery, including drug payload, pharmacokinetics, targeting efficiency, and drug release. The efficiency of magnetic targeting depends on the relative magnitude of magnetic force versus fluid drag force exerted on MNPs in the blood circulation, which are body and surface forces, respectively. Larger MNPs are generally more favorable for magnetic targeting due to their smaller surface area-to-volume ratio.
Preclinical studies have demonstrated that magnetic targeting must be combined with intra-arterial (ia) infusion of drug-loaded MNPs via tumor-feeding vessels to achieve effective tumor inhibition.48 In this approach, ia infusion increases the availability of MNPs in tumor tissue, while magnetic targeting enhances their local retention. Along these lines, several clinical trials for magnetic targeting were performed with magnetic targeted carriers (MTC) infused through tumor-feeding arteries in patients with advanced hepatocarcinoma.49 MTCs are 0.5–5.0 μm iron particles bound to doxorubicin. While MRI confirmed enhanced accumulation of MTCs in liver tumors, the clinical trials were terminated due to a lack of therapeutic efficacy. It is likely that MTCs failed to penetrate the tumor tissue, as the dense extracellular matrix of solid tumors can form a barrier to the transport of macron-sized particles.50,51 The success of future magnetic drug delivery strategies will require a careful balance between particle size, drug payload, and magnetic traction.
An alternative approach utilizes MNPs to control cell movement in and out of the body. Clinical trials have explored this strategy for targeted delivery of endothelial cells to treat corneal edema in a minimally invasive way (NCT04894110), as well as for extracting circulating cancer cells or leukemia cells to reduce metastasis and detect minimal residual disease (NCT02025413 and NCT01411904).
Imaging applications
Since the 1980s, research has revealed that MNPs can alter the magnetic relaxation of nearby protons, generating imaging contrast detectable through T1- or T2-weighted MRI. Compared to small-molecule contrast agents, such as GdDTPA, MNPs offer enhanced sensitivity in detecting functional changes associated with malignancy, significantly improving MRI detection capability. For example, liver tumors are identified by the loss of phagocytic activity in tumor tissue compared to normal liver tissue.52 The mechanism is also used to detect lymph node metastasis in various cancers.53
Moreover, MNPs have been extensively investigated for detecting disease-associated inflammation, which increases vascular endothelial permeability or leads to the infiltration of phagocytes, both resulting in MNP accumulation in diseased tissues. Clinical studies have explored their use in monitoring diabetes progression, detecting atherosclerotic plaques, and assessing inflammation related to myocardial infarction and cerebral aneurysms (see ESI,† Table S1).
In cell therapy, evaluating the distribution of engineered cells in the body is critical to determining therapeutic efficacy. MNPs, with their low cytotoxicity, force-mediated endocytosis, and excellent detection sensitivity, provide a valuable tool for this purpose.54,55 Due to their entrapment in lysosomes and slow degradation, MNPs can label the cells for several days, enabling real-time and long-term tracking of mesenchymal stem cells, dendritic cells, and mononuclear cells.56 With the growing development of various cell therapies, the use of MNP-based cell tracking is expected to increase significantly in future clinical trials.
Thermal therapies
MNPs can convert electromagnetic energy into heat upon exposure to an AMF, a process commonly known as magnetic heating or magnetic hyperthermia in biomedical research. This ability enables heating of tissue deep within the body, as the magnetic field can penetrate the biological tissue with minimal adsorption and scattering. During magnetic heating, MNPs distributed within the tissue generate heat, which dissipates into the surrounding tissues. Since the biological tissue is a poor conductor of heat, regions containing MNPs can be heated to high temperatures, such as 50 °C, without significantly affecting nearby tissues.
A further advantage of magnetic heating is the distribution of MNPs in the body can be visualized with MRI. This enables precise thermal ablation of target tissue when combined with MRI-guided infusion. A key application of this technique is the treatment of brain tumors, which present therapeutic challenges due to their critical anatomical location and the presence of the blood brain barrier. Clinical studies have shown that MNP thermotherapy, in conjunction with reduced radiation doses, is a safe treatment for glioblastoma multiforme.57 This combination therapy has achieved a median survival rate of 23.2 months, compared to 14.6 months in the reference group.57,58 This treatment has been approved for clinical use by EMA and is currently undergoing clinical trials for treating prostate cancer.
In summary, clinical studies enhance conventional imaging, targeted drug delivery, and thermal therapy by leveraging the unique magnetic properties of MNPs to provide high imaging contrast, advanced targeting capabilities, and deep heating potential (Fig. 2). While much of the current research focuses on these areas, the following sections will highlight innovative applications that could shape the future of medicine.
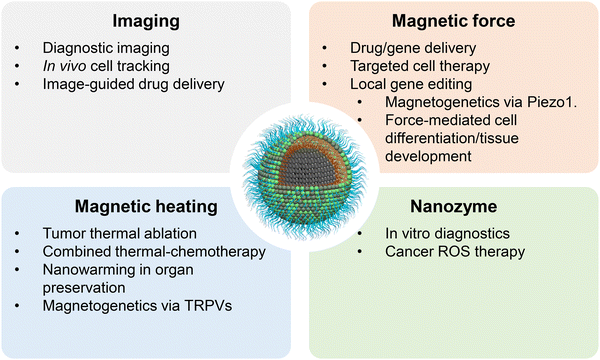 |
| Fig. 2 Applications of magnetic nanoparticles. Applications of MNPs can be broadly categorized into four groups based on the physical or chemical properties emphasized. | |
Applications of magneto-mechanical force
MNPs, as nanosized magnets, experience a force in a nonuniform magnetic field along its gradient. The magnetic force (F) is determined by both the magnetic moment (m) of MNPs and the field gradient (∇H) according to the following equation,where μ represents the magnetic permeability of the tissue. With a field gradient of 10 T m−1, the magnetic force ranges from approximately 2 atto-Newtons for a 10 nm magnetite nanocrystal to 2 femto-Newtons as the size increases to 100 nm. In comparison, the shear force exerted on endothelial cells in microcirculation is on the order of hundreds of pico-Newtons.59 Additionally, the engagement of T cell receptors and agonist peptide–MHC complexes involves forces of approximately 10 pico-Newtons.60 Achieving biologically relevant benchmarks for magneto-mechanical applications often necessitates the use of high-gradient magnetic fields and large clusters of magnetic nanoparticles.
Most force-related research are conducted using small permanent magnets in superficial tissues of small rodent models. A notable advancement in this field is the magnetic particle imaging (MPI) instrument, designed to produce images of MNPs.61 The MPI instrument can generate a field gradient on the order of 10 T m−1, facilitating magnetic navigation of MNPs within the body. A human brain-sized MPI instrument has been developed in the lab.62 However, the size and cost of the MPI instrument are comparable to an MRI machine, posing a considerable challenge to translational research.
Depending on its magnitude, the magnetic force can be used to direct circulating MNPs to specific tissues for targeted delivery, promote molecular interactions, or trigger biological responses by influencing cellular mechanosensors. These mechanisms have found impactful applications in the fields of drug/gene delivery, immunotherapy, regenerative medicine, gene editing, and neuroscience.
Magnetic force-mediated cellular/molecular interactions
The ability of MNPs to migrate or form aggregates in response to an external magnetic field can be utilized to control the distribution of biomolecules on the cell surface or within subcellular compartment. For instance, Etoc et al. created MNPs functionalized with canonical small Rho-GTPases, which regulate cell morphology through complex spatiotemporal activity patterns.63 After injecting MNPs into the cells, a magnetic tip was used to displace them, triggering local remodeling of the actin cytoskeleton and morphological changes in the cells. Similarly, Li et al. demonstrated that fluorescent MNPs bound to molecules on the cell surface could be laterally pulled using a magnetic needle, offering a versatile approach for probing membrane processes.64
T cell intratumoral infiltration, recognition of cancer cells, and activation are critical components of effective cancer immunotherapy. T cell activation depends not only on individual T cell receptor (TCR)-major histocompatibility complex (MHC) interactions but also on TCR clustering, which increases avidity and, consequently, sensitivity to cancer antigens. In this context, Perica et al. developed artificial antigen-presenting cells (nano-aAPCs) by conjugating MHC-Ig dimers and anti-CD28 antibodies to 50–100 nm iron-dextran nanoparticles.65 After binding to the TCR, these nano-aAPCs could aggregate under a magnetic field, leading to larger TCR clusters and an increased number of activated antigen-specific T cells in a mouse melanoma model. In another approach, similar MNPs were conjugated with an immune checkpoint inhibitor (anti-PD-L1) and a T cell activator (anti-CD3).66 The study showed that, following magnetic targeting, the concentration of MNPs in the tumor reached over 15% ID g−1 at 24 hours post-injection – three times higher than in the reference group. The two surface ligands could bind to cancer cells and T cells, respectively, acting as a linker to enhance cell-to-cell interactions. Importantly, magnetic targeting improved the tumor selectivity of T cells, leading to prolonged survival while reducing the incidence of adverse events.
The past decade has seen rapid advancements in CRISPR gene editing. CRISPR employs Cas nucleases and guide RNAs (gRNAs) to modify specific genomic loci, rendering it a versatile tool for gene modification or regulation.12,13 Nonetheless, gRNAs can bind to DNA sequences with base mismatches, and Cas nucleases can tolerate base mismatches and DNA and RNA bulges, leading to off-target activities.67 Similar to cancer chemotherapy, in vivo therapeutic gene editing requires targeted delivery of the CRISPR system to enhance editing efficiency while minimizing genotoxicity due to off-target effects.68 To address this challenge, we developed a novel gene delivery platform by modifying a baculoviral vector (BV) with MNPs conjugated to TAT peptides (Fig. 3).35 BV, derived from an insect virus, offers good biocompatibility, and can effectively transduce a wide range of mammalian cells. However, its in vivo applications have been limited due to rapid inactivation by the complement system. Our study demonstrated that MNP-BV can be locally activated with a magnetic field, which enhances its entry into the cells and allows it to escape from complement inactivation through separation. The magnetic force also promotes actin filament polymerization, improving the localization of the viral genome to the cell nucleus. Meanwhile, MNP-BV outside the magnetic field is inactivated by the complement system. This dual mechanism of magnetic targeting and complement inactivation provides an on/off switch for MNP-BV, enabling spatially controlled gene editing.
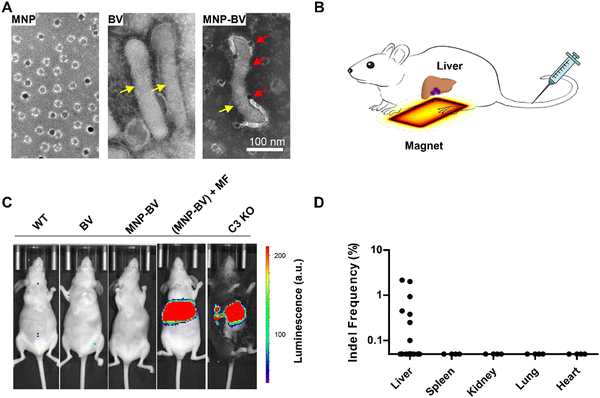 |
| Fig. 3 Spatial control of in vivo gene editing. Baculoviral vectors (BV) labeled with MNPs facilitate localized gene editing through the combined effects of magnetic activation and complement inhibition. (A) TEM images of MNPs, BV, and MNP-BV hybrids. (B) Schematic illustration of MNP-BV-mediated transgene delivery to the mouse liver. (C) Bioluminescence analysis of transgene expression: mice injected with MNP-BV-LUC and exposed to a magnetic field exhibited strong luminescence in the liver. In contrast, no luminescence was observed in mice treated with BV-LUC alone or with MNP-BV-LUC without magnetic field application. C3 knockout mice served as controls. (D) Assessment of gene editing in vital organs. (Reproduced with permission from ref. 35, ©2018 Springer Nature). | |
Magnetic force-induced mechanosensing
The ability to detect and respond to mechanical cues in the surrounding environment is a fundamental function of mammalian cells.69 Traditionally, research in this field has focused on cellular responses to fluid flow, mechanical stretching, and substrate stiffness. However, the versatility of conjugation chemistry enables MNPs to be functionalized for binding to cell surface proteins or accumulating in intracellular compartments, allowing for precise mechanical stimulation of specific mechanosensors or cytoskeletal components. Fernández-Sánchez et al. used an implanted magnet to exert magnetic force on MNP-loaded lysosomes in mesenchymal cells within the connective tissue surrounding colonic crypts.70 This magnetic force mimicked the endogenous stress of early tumor growth without altering tissue stiffness, revealing previously unexplored mechanisms of tumor propagation based on β-catenin signaling pathways. Similarly, Abdel Fattah et al. created human neural organoids with MNP-labeled human pluripotent stem cells, where spatially defined magnetic force provides short-term mechanical tissue perturbations and long-term cytoskeleton remodeling in the organoids.71 The study demonstrates improved patterning in human neural tube organoids through precise, spatially defined MNP-driven actuation.
In our own study, we demonstrated that MNPs accumulated in endothelial cells could induce the relocation of lysosomes and the formation of actin filaments along the field gradient.59,72 Notably, with nearly 5.8 × 105 MNPs accumulated within each endothelial cell, the intracellular magnetic force reached 200 pN per cell.59 The alteration of actin filaments further led to a reversible increase in vascular permeability by affecting the adherence junctions between endothelial cells, potentially providing a magnetic switch to enhance the extravasation of therapeutic agents. More recently, we also found that oscillating magnetic force applied to intracellular MNPs could stimulate several mechanosensing pathways associated with exosome biogenesis and release.73 The cells experiencing intracellular magnetic force released more exosomes and exhibited increased intercellular communication compared with untreated cells.
MNPs can also trigger mechanosensing by interacting with cell surface protein sensors. Optogenetics, a widely used method for investigating brain functions, controls neuronal activity through light.74 Central to this technology are light-sensitive ion channels, which can be activated by light to initiate action potentials in targeted neurons. However, the limited penetration of light in biological tissues necessitates the implantation of optical fibers through the skull to deliver light signals to cortical neurons. This invasive procedure can disrupt normal behavior and physiology. In contrast, a recent study introduced a non-contact neuron activation technique utilizing MNPs and mechanosensitive ion channels, such as Piezo1 (Fig. 4).29,30 By assembling 25 nm MNPs into 200 nm clusters, the researchers generated mechanical force of 2–10 pN force at a magnetic flux density of 20–50 mT. The study further employed a circular magnet array with an effective working range of approximately 70 cm, enabling consistent and reproducible neuromodulation in mice. This innovative approach holds potential for wireless, long-term studies of freely moving large animals.
 |
| Fig. 4 Magnetic force-induced neuron activation. (A) Design of the nanoscale magnetic torquer system. The m-Torquer system acts as a nanoscale magnetic compass to generate the Fτ needed for magnetomechanical signal transduction. (B) Schematic of genetic encoding of Piezo1 and its magnetomechanical gating with specifically targeted m-Torquer with Myc antibody. (C) Temporal repetitive stimulation of Piezo1 with m-Torquer-treated (red) neurons. WGA-m-Torquer-treated (blue) neurons were used as controls. (Reproduced with permission from ref. 30, ©2021 Springer Nature). | |
Applications of magnetic heating
Magnetic heating arises from the relaxation of MNPs within an AMF. In biological media, this involves both Néel and Brownian relaxation.75 Néel relaxation refers to the internal reorientation of the magnetic domain constrained by the magnetic anisotropy energy barrier, while Brownian relaxation involves the rotation of the particle in the surrounding medium. The heating efficiency of MNPs is quantified by the specific loss power (SLP), also known as specific absorption rate (SAR), which represents the thermal energy generated per unit mass of MNPs. The SLP is influenced not only by the magnetic properties of the MNPs but also by the frequency (f), field strength (H), and wave form of the AMF. An increase in the frequency and field strength of the AMF leads to higher SLP. However, a safety limit of H × f < 4.85 × 108 A m−1 s−1 for the whole body is imposed to prevent eddy currents in bodily fluids during clinical applications.76 This restriction may be somewhat relaxed in the treatment of local diseases or in preclinical and in vitro studies.77
Unlike the magneto-mechanical effect, there is still no analytical model that accurately predicts SLP during magnetic heating. One widely cited model of magnetic heating is the linear response theory (LRT).78 According to LRT, under the assumptions that superparamagnetic MNPs have a uniaxial crystalline structure, a single magnetic domain, and a magnetic susceptibility linearly proportional to the inductive field, the SLP is given by,
|  | (2) |
where
μ0 is the permeability of vacuum,
χ0 is the equilibrium susceptibility, and
τ is the relaxation time.
78 The LRT predicts that SLP reaches its maximum when the relaxation time matches the period of the AMF. Consequently, the optimal size for magnetic iron oxide nanoparticles is predicted to be approximately 14 nm when the frequency is 300 kHz. While some experimental results align with this prediction, our study, along with others, has found that SLR increases monotonically with particle size (
Fig. 5).
25,79,80 The discrepancy among experimental studies is likely due to variations in MNP dispersity and composition. It is important to note that many instruments used in these studies are not specifically designed for magnetic heating and are unable to produce a monochromatic AMF. Several new models of magnetic heating have been proposed that incorporate more complex relaxation processes and realistic anisotropy energy profile.
81–83 However, further experimental validation is needed to confirm these models’ predictions.
 |
| Fig. 5 Size-dependent heating of MNPs. SAR was measured for MNPs ranging from 6 to 40 nm dispersed in DI water, using an AMF at 325 kHz and 20.7 kA m−1. The solid curve represents the theoretical SAR predictions according to the LRT model. (Reproduced with permission from ref. 25, ©2017 American Chemical Society). | |
The heat generation of MNPs is also known to depend on particle shape and composition. Experimental studies have demonstrated that SLP of cubic MNPs is twice that of spherical MNPs.84,85 Nano-octopods and nanorods have similarly shown higher SLP compared to spherical particles.86,87 Our study has further revealed that simply oxidizing magnetite nanoparticles to maghemite can more than double the SLP, likely due to an increase in magnetic anisotropy.88 Additionally, tuning of MNP composition by incorporating metallic ions such as Mn2+ and Zn2+ has been shown to enhance the SLP as well.28,84
The efficiency of magnetic heating typically falls within the range of hundreds of W g−1 MNP, which is considerably lower than other clinical heating techniques.25 However, the use of radio frequency AMF enables deep tissue penetration with minimal absorption and scattering, making magnetic heating particularly valuable for thermal treatment of areas like the brain. Efficient heating traditionally requires a high concentration of MNPs, achievable only through local infusion. For instance, in the clinical application of NanoTherm, an MNP solution was infused into a brain tumor, achieving a final concentration of approximately 30 mg mL−1 within the tumor tissue.57 Due to the low heating efficiency of magnetic heating, a long-standing debate exists around nano-scale heating effects. Some argue that magnetic heating results in a relatively smooth temperature increase across the affected tissue, as predicted by classical Fourier heat transfer theory.42,89 In contrast, others propose that individual MNPs can heat nearby molecules without substantially increasing the surrounding medium's temperature, a concept linked to the emerging field of magnetogenetics.34,36–39
To date, most research on magnetic heating has focused on cancer treatment. Beyond thermal ablation, magnetic heating has been extensively studied for tumor hyperthermia, which involves raising tissue temperature to approximate 41–45 °C.90 Studies have shown that cells in the mitosis stage are more heat-sensitive, allowing hyperthermia to specifically target cancer cells due to their elevated proliferation rate.91,92 Consequently, magnetic hyperthermia has been combined with treatments such as chemotherapy and radiation. For example, in a study using MNPs loaded with doxorubicin, we observed a synergistic effect between mild hyperthermia at 43 °C and doxorubicin in reducing HeLa cell viability.93 More recently, the development of high heating MNPs has opened up new applications for magnetic heating, particular in two emerging fields: organ cryopreservation and magnetogenetics.
Magnetic heating in organ cryopreservation
Organ transplantation is the last therapeutic option for patients with otherwise incurable diseases. However, a major public health challenge is the shortage of organs, primarily due to the lack of long-term organ preservation methods.94,95 Current organ preservation techniques can only maintain most organs for 4–12 hours, creating significant stress for transportation, immune crossmatching, and surgical planning. For example, approximately 70% of donated hearts are discarded during processing.94 Recently, cryopreservation via vitrification has emerged as a promising technology for long-term organ storage. This method preserves organs in a glass-like state to prevent structural damage and loss of cell viability caused by ice crystal formation.96–98 However, when these preserved organs are warmed to body temperature for transplantation, recrystallization in the cryoprotectant can occur if the warming rate is slower than the critical warming rate.99,100 Therefore, convective heating, which warms the organ from the surface, faces a critical size limitation of 1–3 mL due to slow heat conduction in the tissue, making it unsuitable for cryopreserving human organs.
Magnetic heating has emerged as a viable option for tissue warming, as it can deliver heat uniformly throughout the tissue. The nanowarming process involves perfusing the donor organ with a cryoprotectant containing MNPs, rapidly freezing it and storing at an ultralow temperature (<−140 °C), warming the organ to body temperature, and replacing the cryoprotectant with a blood-compatible solution (Fig. 6).101 Typically, the cryoprotectant contains high concentrations of organic and inorganic antifreeze compounds. For example, the common cryoprotectant, VS55, is composed of 3.1 M dimethyl sulfoxide, 2.2 M propylene glycol, 3.1 M formamide, and 2.4 g L−1 HEPES in EuroCollins solution. Ensuring MNP stability in the cryoprotectant during extreme temperature shifts is critical, as MNP aggregation can alter magnetic relaxation and decrease heating efficiency.102 Additionally, both the magnetic properties of MNPs and the medium viscosity vary considerably with temperature.103
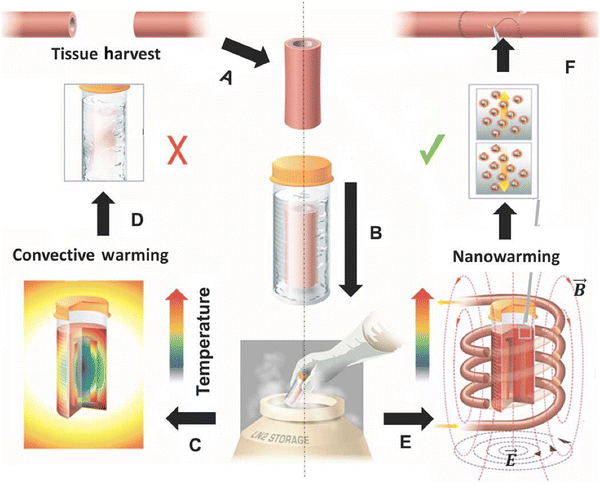 |
| Fig. 6 Schematic illustrating tissue vitrification, convective warming, and nanowarming. (A) Tissues are harvested from a donor. (B) Tissues are loaded in a vial with CPA (VS55) and MNPs in a stepwise protocol, vitrified by standard convection, and stored at cryogenic temperatures. Warming by standard convection (C) leads to failure in larger 50-ml systems (D). Nanowarming in an alternating magnetic field, an inductive RF coil that stimulates nanoparticle heating (E), avoids warming failure and renders the tissue suitable for further testing or use (F). (Reproduced with permission from ref. 101, ©2017 The American Association for the Advancement of Science). | |
Several studies have demonstrated that the colloidal and thermal stability of MNPs in VS55 can be maintained though surface coating with resorcinol–formaldehyde resin or silica.104–107 Additionally, surface modification with poly(ethylene glycol) has been shown to reduce cellular interactions and thus cytotoxicity.106,107 Manuchehrabadi achieved nanowarming of porcine arteries and porcine aortic heart valve leaflet tissues using magnetic heating.101 The preserved tissues showed excellent viability with no change in biomechanical properties. Notably, uniform and rapid rewarming at a rate of >130 °C per minute was achieved in volumes up to 80 mL. A subsequent study demonstrated that vitrified rat kidneys recovered through nanowarming were suitable for transplantation and restored full renal function in nephrectomized recipients.108 Although nanowarming technology is still in its infancy, these studies show great promise to transform the landscape of organ transplantation.
Magnetogenetics
Inspired by optogenetics, magnetogenetics utilizes MNPs under an AMF as magnetic switches to activate cellular functions. This emerging field holds great potential for remotely controlling individual neurons in the brain. In a pioneering study, Huang et al. demonstrated that synthetic ferrite nanoparticles, when bound to a temperature-sensitive ion channel (TRPV1), could activate this channel under a radio-frequency AMF.39 Subsequently, similar effects were achieved using transient receptor potential (TRP) channels coupled with biogenic ferritin particles containing 5–12 nm iron oxide cores.109 So far, studies using either synthetic or biogenic magnetic nanoparticles (MNPs) have shown the ability to remotely control brain activities in live mice (Fig. 7).36,38,110–112 Additionally, magnetic heating of MNPs near thermosensitive TRPA1-A channels, which regulate the “fruitless” driver in Drosophila melanogaster, allows for controlled wing extension with sub-second response times.34 When combined with synthetic genetic circuits, magnetogenetics has enabled applications in other areas, such as the remote regulation of glucose homeostasis and the targeted activation of engineered bacteria for cancer immunotherapy.109,113
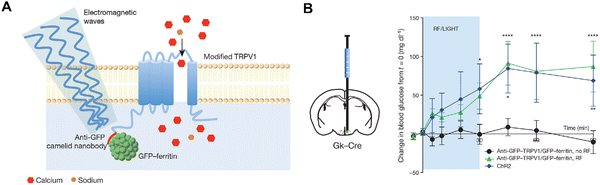 |
| Fig. 7 Remote neural activation in vivo using radio waves. (A) Schematic of activation system. Ferritin-TRPV1 complexes were delivered to hypothalamic glucose-sensing neurons via viral transfection. (B) Treatment with a radio frequency AMF (465 kHz) elevated blood glucose levels in mice. (Reproduced with permission from ref. 38, ©2016 Springer Nature). | |
While experimental results support the feasibility of magnetogenetics, the underlying biophysical mechanisms remain unclear. It is generally assumed that the MNP-induced molecular activation is due to nanoscale heat confinement during magnetic nanoparticle heating, implying that the temperature on the surface of MNPs is significantly higher than that of the surrounding medium. As a result, the heat generated by MNPs under an AMF activates nearby TRP channels, leading to downstream cellular responses. However, analyses based on classical Fourier heat conduction theory and the properties of MNPs indicate that the temperature rise in MNPs under given conditions should be much lower than that required to activate TRPVs.40,42,89 Experimental attempts to measure the surface temperature of MNPs have yielded conflicting results, with both supporting and contradictory evidence.114–118 Moreover, recent publications have raised concerns that biogenic MNP-induced molecular activation may be artifactual.119–121
In addition to magnetic heating, alternative hypotheses have been proposed to explain the mechanism of magnetogenetics, including the magnetic force/torque effect, magneto-caloric effects, and reactive oxygen species (ROS)-mediated molecular activation.41,122 It is important to note that the MNP-mediated cellular functions, such as the activation of TRPVs in neurons, can arise from sporadic and transient actions of individual MNPs.34,38,39,111 In contrast, magnetic nanoparticle heating is usually modeled as a classical equilibrium process. Additional evidence and theoretical analysis at the nanoscale are needed to provide a compelling explanation. Furthermore, it remains unknown what the working range of the nanoscale effect is. Since MNP-induced neural responses typically occur on the order of seconds, it is essential to explore the possibility of shortening the response time to milliseconds, which would be more meaningful for investigating neuronal functions. Despite ongoing debates and unclear mechanisms, magnetogenetics opens an exciting new frontier in neuroscience research. It offers potential for brain–computer interfaces and introduces a novel class of therapeutic interventions by linking magnetic control to a range of physiological functions.
Functioning as nanozymes
Hydroxyl free radicals (˙OH) are highly reactive molecules capable of interacting with a wide range of biomolecules. Elevated levels of hydroxyl free radicals can damage cellular components such as DNA, proteins, and lipids, ultimately leading to cell death.123,124 Gao et al.'s pioneering work demonstrated that magnetite nanoparticles can catalyze the decomposition of hydrogen peroxide (H2O2) to hydroxyl free radicals through a heterogeneous Fenton reaction.43 Since this discovery, MNPs have been extensively studied for their ability to induce cell polarization, apoptosis, and ferroptosis. Their functionalization potential for targeted and combination therapies gives them advantages over to small-molecule drugs.
The MNP-catalyzed Fenton reaction occurs on the surface of iron oxide nanocrystals. Thus, the reaction rate is influenced by the interface between the nanocrystal and its surrounding medium.125,126 Interestingly, our study revealed that the catalytic activity of MNPs is highly dependent on their composition, despite the tendency of the nanocrystals to oxidize and exhibit similar surface oxidative states (Fig. 8).45 With comparable size, coating, and surface oxidation states, wüstite (FeO) nanoparticles demonstrated a 4.6 folds increase in catalytic activity compared to maghemite. Further analysis suggests that this increased catalytic activity is due to the presence of internal low-valence iron (Fe0 and Fe2+), which accelerates the recycling of surface Fe3+ back to Fe2+via intraparticle electron transport.45,127
 |
| Fig. 8 MNP-mediated heterogeneous Fenton reaction. (A) Schematic of enhancing surface Fenton reaction via intraparticle electron transport. In a composite nanoparticle of magnetite (Fe3O4) shell and a core of low-valence iron (Fe0 & Fe2+), the low-valence iron can accelerate the catalysis by reducing the octahedral Fe3+ in the magnetite shell to Fe2+ through intraparticle electron transport. (B) Catalytic activities of MNPs of various compositions. (Reproduced with permission from ref. 45, ©2023 John Wiley and Sons). | |
Notably, the catalytic activity of MNPs is also pH-dependent. MNPs catalyze the production of hydroxyl free radicals only in acidic environments, such as endosomes or lysosomes, but act as catalase, generating oxygen molecules instead of hydroxyl free radicals at neural pH.128,129 This unique pH sensitivity enables targeting of specific cell populations based on cellular uptake and iron metabolism. For instance, Trujillo-Alonso et al. found that ferumoxytol exhibited cytotoxic effects on leukemia cells with low levels of ferroportin, a cellular iron exporter.130 Moreover, Zanganeh et al. demonstrated that ferumoxytol could polarize macrophages toward a tumor-suppressing phenotype, inhibiting breast cancer growth and lung metastasis.44
Research into the catalytic properties of MNPs also offers valuable insight into mitigating potential adverse effects in therapeutic applications. Wu et al. found that ultrasmall magnetite nanoparticles (2.3 nm) were highly toxic due to increased free radical production, whereas 9.3 nm magnetite nanoparticles showed no apparent toxicity in a mouse model.131 The size of MNPs can also influence the nature of cytotoxicity. Xie et al. discovered that 9 nm magnetite nanoparticles induced cellular mitochondrial dysfunction, while 14 nm magnetite nanoparticles primarily damaged cell membrane integrity and promoted lactate dehydrogenase leakage.132 Consequently, the catalytic activity of MNPs is a critical factor in designing MNP-based applications.
Outlook and future perspectives
Since the emergence of nanomedicine four decades ago, MNPs have become one of the most active and successful areas of research, driven by their exceptional biocompatibility and unique nanoscale magnetic properties. Clinical studies have primarily focused on imaging, thermal therapy, and magnetic targeting. While MNPs were initially approved for cancer medicine in the US and Europe, recent clinical research has expanded globally, with notable efforts in countries like China exploring their applications in treating a broader range of diseases.
A new frontier in MNP research is the controlled mechanical and thermal modulation of cells and tissues. Advances in synthesis techniques have enabled precise control over MNP size and composition, leading to the development of particles optimized for specific mechanical and thermal properties. In recent years, the use of MNPs to stimulate mechanosensitive receptors, ion channels, and other cellular components has opened novel avenues for manipulating cellular behavior. These research efforts are supported by a better understanding of mechano- and thermo-sensitive ion channels, such as the TRP and Piezo protein families, which play crucial roles in various aspects of cell biology. The significance of these proteins was recognized with the 2021 Nobel Prize.
The ability to remotely control cellular processes using MNPs offers exciting applications in regenerative medicine, tissue engineering, and neuromodulation. For instance, mechanical stimulation of stem cells via MNPs can influence differentiation pathways, while thermal activation of neurons through MNPs conjugated to thermosensitive ion channels has opened possibilities for non-invasive brain stimulation techniques. It is conceivable that MNP-derived nanotechnology will soon intersect with synthetic biology, forging new directions in biomedical applications. Remarkably, all these functions can be achieved using a single MNP, with its size, composition, and functionalization determining its specific properties. The inherent multifunctionality of MNPs thus provides a highly versatile platform for biomedical innovation.
Despite their promise, several challenges remain for the clinical translation of MNP applications in emerging fields. Over the past two decades, significant progress has been made in understanding the biocompatibility and safety of MNPs, particularly in cancer treatment clinical studies. However, as the MNP applications extend to vital organs in the context of non-terminal diseases, there is an urgent need to assess their biodistribution, clearance, and long-term toxicity. This is particularly important given the association between abnormal iron metabolism and diseases like Alzheimer's.133 Additionally, regulatory considerations are crucial for the development of MNP functionalized with novel components such as targeting moieties and signaling molecules, as manufacturing practices, quality control, and reproducibility must meet stringent standards. The interdisciplinary nature of MNP-related research also presents challenges, especially since the magnetic properties of MNPs are heavily dependent on the applied magnetic field. Clinical translations will require the development of specialized electromagnetic instruments for human use. The widespread clinical use of MNPs in imaging is largely due to the availability of MRI machines in universities and major hospitals. Lastly, while the effects of magnetic fields on the human body have been extensively investigated, the impact of high-gradient, high-frequency magnetic fields on target organs needs to be carefully examined in new settings. The FDA has issued guidance for industry that provides a comprehensive summary of the major concerns associated with nanomaterials in clinical drug applications. Given the novelty of these research directions, it is likely that additional safety and ethical considerations, beyond those encountered in conventional imaging and drug delivery studies, will emerge as the field continues to develop.
Looking forward, the continued convergence of nanotechnology, materials science, engineering, and biomedicine is likely to drive further innovations in MNP design and application. By harnessing their unique nanoscale magnetic properties and improving our understanding of their interactions with biological systems, MNPs are poised to revolutionize the landscape of nanomedicine and enable new approaches for disease treatment and management.
Data availability
No primary research results, software or code have been included and no new data were generated or analyzed as part of this review.
Conflicts of interest
There are no conflicts of interest to declare.
Acknowledgements
This work was supported by the NIH/NHLBI funding (R21HL166178 to ST), the NIH/NIAMS funding (R21AR078447 to ST), the NSF funding (2342391 to ST).
References
- P. H. Meyers, C. M. Nice Jr., G. R. Meckstroth, H. C. Becker, P. J. Moser and M. Goldstein, Pathologic studies following magnetic control of metallic iron particles in the lymphatic and vascular system of dogs as a contrast and isotopic agent, Am. J. Roentgenol., Radium Ther. Nucl. Med., 1966, 96, 913–921 CrossRef CAS.
- S. Tong, H. Zhu and G. Bao, Magnetic Iron Oxide Nanoparticles for Disease Detection and Therapy, Mater. Today, 2019, 31, 86–99 CrossRef CAS PubMed.
- A. N. Kharlamov, A. E. Tyurnina, V. S. Veselova, O. P. Kovtun, V. Y. Shur and J. L. Gabinsky, Silica-gold nanoparticles for atheroprotective management of plaques: results of the NANOM-FIM trial, Nanoscale, 2015, 7, 8003–8015 RSC.
- A. N. Kharlamov, J. A. Feinstein, J. A. Cramer, J. A. Boothroyd, E. V. Shishkina and V. Shur, Plasmonic photothermal therapy of atherosclerosis with nanoparticles: long-term outcomes and safety in NANOM-FIM trial, Future Cardiol., 2017, 13, 345–363 CrossRef CAS PubMed.
- J. E. Jaetao, K. S. Butler, N. L. Adolphi, D. M. Lovato, H. C. Bryant, I. Rabinowitz, S. S. Winter, T. E. Tessier, H. J. Hathaway and C. Bergemann,
et al., Enhanced leukemia cell detection using a novel magnetic needle and nanoparticles, Cancer Res., 2009, 69, 8310–8316 CrossRef CAS PubMed.
- L. Charters, Magnetic cell therapy for corneal endothelial dysfunction, Ophthalmol. Times Eur., 2024, 20a, 34 Search PubMed.
- E. Seneterre, P. Taourel, Y. Bouvier, J. Pradel, B. Van Beers, J. P. Daures, J. Pringot, D. Mathieu and J. M. Bruel, Detection of hepatic metastases: ferumoxides-enhanced MR imaging versus unenhanced MR imaging and CT during arterial portography, Radiology, 1996, 200, 785–792 CrossRef CAS.
- E. H. Aarntzen, M. Srinivas, F. Bonetto, L. J. Cruz, P. Verdijk, G. Schreibelt, M. van de Rakt, W. J. Lesterhuis, M. van Riel and C. J. Punt,
et al., Targeting of 111In-labeled dendritic cell human vaccines improved by reducing number of cells, Clin. Cancer Res., 2013, 19, 1525–1533 CrossRef CAS PubMed.
- D. Karussis, C. Karageorgiou, A. Vaknin-Dembinsky, B. Gowda-Kurkalli, J. M. Gomori, I. Kassis, J. W. Bulte, P. Petrou, T. Ben-Hur and O. Abramsky,
et al., Safety and immunological effects of mesenchymal stem cell transplantation in patients with multiple sclerosis and amyotrophic lateral sclerosis, Arch. Neurol., 2010, 67, 1187–1194 CrossRef PubMed.
- F. D. Birkhäuser, U. E. Studer, J. M. Froehlich, M. Triantafyllou, L. J. Bains, G. Petralia, P. Vermathen, A. Fleischmann and H. C. Thoeny, Combined ultrasmall superparamagnetic particles of iron oxide-enhanced and diffusion-weighted magnetic resonance imaging facilitates detection of metastases in normal-sized pelvic lymph nodes of patients with bladder and prostate cancer, Eur. Urol., 2013, 64, 953–960 CrossRef.
- M. Triantafyllou, U. E. Studer, F. D. Birkhäuser, A. Fleischmann, L. J. Bains, G. Petralia, A. Christe, J. M. Froehlich and H. C. Thoeny, Ultrasmall superparamagnetic particles of iron oxide allow for the detection of metastases in normal sized pelvic lymph nodes of patients with bladder and/or prostate cancer, Eur. J. Cancer, 2013, 49, 616–624 CrossRef CAS.
- J. M. Richards, S. I. Semple, T. J. MacGillivray, C. Gray, J. P. Langrish, M. Williams, M. Dweck, W. Wallace, G. McKillop and R. T. Chalmers,
et al., Abdominal aortic aneurysm growth predicted by uptake of ultrasmall superparamagnetic particles of iron oxide: a pilot study, Circ. Cardiovasc. Imaging, 2011, 4, 274–281 CrossRef.
- J. M. Richards, C. A. Shaw, N. N. Lang, M. C. Williams, S. I. Semple, T. J. MacGillivray, C. Gray, J. H. Crawford, S. R. Alam and A. P. Atkinson,
et al., In vivo mononuclear cell tracking using superparamagnetic particles of iron oxide: feasibility and safety in humans, Circ. Cardiovasc. Imaging, 2012, 5, 509–517 CrossRef.
- S. R. Alam, A. S. Shah, J. Richards, N. N. Lang, G. Barnes, N. Joshi, T. MacGillivray, G. McKillop, S. Mirsadraee and J. Payne,
et al., Ultrasmall superparamagnetic particles of iron oxide in patients with acute myocardial infarction: early clinical experience, Circ. Cardiovasc. Imaging, 2012, 5, 559–565 CrossRef.
- R. Baumgartner, W. Cho, A. Coimbra, C. Chen, Z. Wang, A. Struyk, N. Venketasubramanian, M. Low, C. Gargano and F. Zhao,
et al., Evaluation of an fMRI USPIO-based assay in healthy human volunteers, J. Magn. Reson. Imaging, 2017, 46, 124–133 CrossRef.
- R. Weissleder, A. Bogdanov, E. A. Neuwelt and M. Papisov, Long-circulating iron oxides for MR imaging, Adv. Drug Delivery Rev., 1995, 16, 321–334 CrossRef CAS.
- R. Weissleder, G. Elizondo, J. Wittenberg, C. A. Rabito, H. H. Bengele and L. Josephson, Ultrasmall superparamagnetic iron oxide: characterization of a new class of contrast agents for MR imaging, Radiology, 1990, 175, 489–493 CrossRef CAS PubMed.
- S. Sun, H. Zeng, D. B. Robinson, S. Raoux, P. M. Rice, S. X. Wang and G. Li, Monodisperse MFe2O4 (M = Fe, Co, Mn) nanoparticles, J. Am. Chem. Soc., 2004, 126, 273–279 CrossRef CAS.
- J. Park, K. An, Y. Hwang, J. G. Park, H. J. Noh, J. Y. Kim, J. H. Park, N. M. Hwang and T. Hyeon, Ultra-large-scale syntheses of monodisperse nanocrystals, Nat. Mater., 2004, 3, 891–895 CrossRef CAS PubMed.
- A. Demortiere, P. Panissod, B. P. Pichon, G. Pourroy, D. Guillon, B. Donnio and S. Begin-Colin, Size-dependent properties of magnetic iron oxide nanocrystals, Nanoscale, 2011, 3, 225–232 RSC.
- J. H. Lee, Y. M. Huh, Y. W. Jun, J. W. Seo, J. T. Jang, H. T. Song, S. Kim, E. J. Cho, H. G. Yoon and J. S. Suh,
et al., Artificially engineered magnetic nanoparticles for ultra-sensitive molecular imaging, Nat. Med., 2007, 13, 95–99 CrossRef CAS PubMed.
- D. Kim, N. Lee, M. Park, B. H. Kim, K. An and T. Hyeon, Synthesis of uniform ferrimagnetic magnetite nanocubes, J. Am. Chem. Soc., 2009, 131, 454–455 CrossRef CAS PubMed.
- L. M. Bronstein, J. E. Atkinson, A. G. Malyutin, F. Kidwai, B. D. Stein, D. G. Morgan, J. M. Perry and J. A. Karty, Nanoparticles by Decomposition of Long Chain Iron Carboxylates: From Spheres to Stars and Cubes, Langmuir, 2011, 27, 3044–3050 CrossRef CAS PubMed.
- H. Y. Sun, B. Chen, X. L. Jiao, Z. Jiang, Z. H. Qin and D. R. Chen, Solvothermal Synthesis of Tunable Electroactive Magnetite Nanorods by Controlling the Side Reaction, J. Phys. Chem. C, 2012, 116, 5476–5481 CrossRef CAS.
- S. Tong, C. A. Quinto, L. Zhang, P. Mohindra and G. Bao, Size-Dependent Heating of Magnetic Iron Oxide Nanoparticles, ACS Nano, 2017, 11, 6808–6816 CrossRef CAS PubMed.
- Y. Hou, Z. Xu and S. Sun, Controlled synthesis and chemical conversions of FeO nanoparticles, Angew. Chem., Int. Ed., 2007, 46, 6329–6332 CrossRef CAS PubMed.
- R. Chen, M. G. Christiansen and P. Anikeeva, Maximizing hysteretic losses in magnetic ferrite nanoparticles via model-driven synthesis and materials optimization, ACS Nano, 2013, 7, 8990–9000 Search PubMed.
- J. H. Lee, J. T. Jang, J. S. Choi, S. H. Moon, S. H. Noh, J. W. Kim, J. G. Kim, I. S. Kim, K. I. Park and J. Cheon, Exchange-coupled magnetic nanoparticles for efficient heat induction, Nat. Nanotechnol., 2011, 6, 418–422 CrossRef CAS.
- S. H. Choi, J. Shin, C. Park, J. U. Lee, J. Lee, Y. Ambo, W. Shin, R. Yu, J. Y. Kim and J. D. Lah,
et al., In vivo magnetogenetics for cell-type-specific targeting and modulation of brain circuits, Nat. Nanotechnol., 2024, 19, 1333–1343 CrossRef CAS PubMed.
- J. U. Lee, W. Shin, Y. Lim, J. Kim, W. R. Kim, H. Kim, J. H. Lee and J. Cheon, Non-contact long-range magnetic stimulation of mechanosensitive ion channels in freely moving animals, Nat. Mater., 2021, 20, 1029–1036 CrossRef CAS PubMed.
- R. J. Mannix, S. Kumar, F. Cassiola, M. Montoya-Zavala, E. Feinstein, M. Prentiss and D. E. Ingber, Nanomagnetic actuation of receptor-mediated signal transduction, Nat. Nanotechnol., 2008, 3, 36–40 CrossRef CAS PubMed.
- S. Laurent, D. Forge, M. Port, A. Roch, C. Robic, L. Vander Elst and R. N. Muller, Magnetic iron oxide nanoparticles: synthesis, stabilization, vectorization, physicochemical characterizations, and biological applications, Chem. Rev., 2008, 108, 2064–2110 CrossRef CAS PubMed.
- Y. W. Jun, J. W. Seo and J. Cheon, Nanoscaling laws of magnetic nanoparticles and their applicabilities in biomedical sciences, Acc. Chem. Res., 2008, 41, 179–189 CrossRef CAS.
- C. Sebesta, D. Torres Hinojosa, B. Wang, J. Asfouri, Z. Li, G. Duret, K. Jiang, Z. Xiao, L. Zhang and Q. Zhang,
et al., Subsecond multichannel magnetic control of select neural circuits in freely moving flies, Nat. Mater., 2022, 21, 951–958 CrossRef CAS PubMed.
- H. Zhu, L. Zhang, S. Tong, C. M. Lee, H. Deshmukh and G. Bao, Spatial control of in vivo CRISPR-Cas9 genome editing via nanomagnets, Nat. Biomed. Eng., 2019, 3, 126–136 CrossRef CAS.
- S. A. Stanley, J. E. Gagner, S. Damanpour, M. Yoshida, J. S. Dordick and J. M. Friedman, Radio-wave heating of iron oxide nanoparticles can regulate plasma glucose in mice, Science, 2012, 336, 604–608 CrossRef CAS.
- R. Chen, G. Romero, M. G. Christiansen, A. Mohr and P. Anikeeva, Wireless magnetothermal deep brain stimulation, Science, 2015, 347, 1477–1480 CrossRef CAS.
- S. A. Stanley, L. Kelly, K. N. Latcha, S. F. Schmidt, X. Yu, A. R. Nectow, J. Sauer, J. P. Dyke, J. S. Dordick and J. M. Friedman, Bidirectional electromagnetic control of the hypothalamus regulates feeding and metabolism, Nature, 2016, 531, 647–650 CrossRef CAS PubMed.
- H. Huang, S. Delikanli, H. Zeng, D. M. Ferkey and A. Pralle, Remote control of ion channels and neurons through magnetic-field heating of nanoparticles, Nat. Nanotechnol., 2010, 5, 602–606 CrossRef CAS.
- P. Anikeeva and A. Jasanoff, Problems on the back of an envelope, eLife, 2016, 5, e19569 CrossRef.
- M. Barbic, Possible magneto-mechanical and magneto-thermal mechanisms of ion channel activation in magnetogenetics, eLife, 2019, 8, e45807 CrossRef PubMed.
- M. Meister, Physical limits to magnetogenetics, eLife, 2016, 5, e17210 CrossRef.
- L. Gao, J. Zhuang, L. Nie, J. Zhang, Y. Zhang, N. Gu, T. Wang, J. Feng, D. Yang and S. Perrett,
et al., Intrinsic peroxidase-like activity of ferromagnetic nanoparticles, Nat. Nanotechnol., 2007, 2, 577–583 CrossRef CAS PubMed.
- S. Zanganeh, G. Hutter, R. Spitler, O. Lenkov, M. Mahmoudi, A. Shaw, J. S. Pajarinen, H. Nejadnik, S. Goodman and M. Moseley,
et al., Iron oxide nanoparticles inhibit tumour growth by inducing pro-inflammatory macrophage polarization in tumour tissues, Nat. Nanotechnol., 2016, 11, 986–994 CrossRef CAS PubMed.
- Z. Yi, X. Yang, Y. Liang, F. Chapelin and S. Tong, Enhancing ROS-Inducing Nanozyme through Intraparticle Electron Transport, Small, 2024, 20, e2305974 CrossRef.
- R. Weissleder, D. D. Stark, B. L. Engelstad, B. R. Bacon, C. C. Compton, D. L. White, P. Jacobs and J. Lewis, Superparamagnetic iron oxide: pharmacokinetics and toxicity, AJR, Am. J. Roentgenol., 1989, 152, 167–173 CrossRef CAS.
- A. Kunzmann, B. Andersson, T. Thurnherr, H. Krug, A. Scheynius and B. Fadeel, Toxicology of engineered nanomaterials: focus on biocompatibility, biodistribution and biodegradation, Biochim. Biophys. Acta, 2011, 1810, 361–373 CrossRef CAS.
- C. Alexiou, W. Arnold, R. J. Klein, F. G. Parak, P. Hulin, C. Bergemann, W. Erhardt, S. Wagenpfeil and A. S. Lubbe, Locoregional cancer treatment with magnetic drug targeting, Cancer Res., 2000, 60, 6641–6648 CAS.
- M. W. Wilson, R. K. Kerlan Jr., N. A. Fidelman, A. P. Venook, J. M. LaBerge, J. Koda and R. L. Gordon, Hepatocellular carcinoma: regional therapy with a magnetic targeted carrier bound to doxorubicin in a dual MR imaging/conventional angiography suite–initial experience with four patients, Radiology, 2004, 230, 287–293 CrossRef PubMed.
- F. Yuan, A. Krol and S. Tong, Available space and extracellular transport of macromolecules: effects of pore size and connectedness, Ann. Biomed. Eng., 2001, 29, 1150–1158 CrossRef CAS.
- M. R. Dreher, W. Liu, C. R. Michelich, M. W. Dewhirst, F. Yuan and A. Chilkoti, Tumor vascular permeability, accumulation, and penetration of macromolecular drug carriers, J. Natl. Cancer Inst., 2006, 98, 335–344 CrossRef CAS.
- E. Seneterre, P. Taourel, Y. Bouvier, J. Pradel, B. Van Beers, J. P. Daures, J. Pringot, D. Mathieu and J. M. Bruel, Detection of hepatic metastases: ferumoxides-enhanced MR imaging versus unenhanced MR imaging and CT during arterial portography, Radiology, 1996, 200, 785–792 CrossRef CAS PubMed.
- M. G. Harisinghani, J. Barentsz, P. F. Hahn, W. M. Deserno, S. Tabatabaei, C. H. van de Kaa, J. de la Rosette and R. Weissleder, Noninvasive detection of clinically occult lymph-node metastases in prostate cancer, N. Engl. J. Med., 2003, 348, 2491–2499 CrossRef.
- L. Zhang, S. Hajebrahimi, S. Tong, X. Gao, H. Cheng, Q. Zhang, D. T. Hinojosa, K. Jiang, L. Hong and J. Huard,
et al., Force-Mediated Endocytosis of Iron Oxide Nanoparticles for Magnetic Targeting of Stem Cells, ACS Appl. Mater. Interfaces, 2023, 15, 50574–50585 CrossRef CAS PubMed.
- N. Landazuri, S. Tong, J. Suo, G. Joseph, D. Weiss, D. J. Sutcliffe, D. P. Giddens, G. Bao and W. R. Taylor, Magnetic
targeting of human mesenchymal stem cells with internalized superparamagnetic iron oxide nanoparticles, Small, 2013, 9, 4017–4026 CrossRef CAS.
- I. J. de Vries, W. J. Lesterhuis, J. O. Barentsz, P. Verdijk, J. H. van Krieken, O. C. Boerman, W. J. Oyen, J. J. Bonenkamp, J. B. Boezeman and G. J. Adema,
et al., Magnetic resonance tracking of dendritic cells in melanoma patients for monitoring of cellular therapy, Nat. Biotechnol., 2005, 23, 1407–1413 CrossRef CAS.
- K. Maier-Hauff, F. Ulrich, D. Nestler, H. Niehoff, P. Wust, B. Thiesen, H. Orawa, V. Budach and A. Jordan, Efficacy and safety of intratumoral thermotherapy using magnetic iron-oxide nanoparticles combined with external beam radiotherapy on patients with recurrent glioblastoma multiforme, J. Neurooncol., 2011, 103, 317–324 CrossRef PubMed.
- R. Stupp, W. P. Mason, M. J. van den Bent, M. Weller, B. Fisher, M. J. Taphoorn, K. Belanger, A. A. Brandes, C. Marosi and U. Bogdahn,
et al., Radiotherapy plus concomitant and adjuvant temozolomide for glioblastoma, N. Engl. J. Med., 2005, 352, 987–996 CrossRef CAS PubMed.
- Y. Qiu, S. Tong, L. Zhang, Y. Sakurai, D. R. Myers, L. Hong, W. A. Lam and G. Bao, Magnetic forces enable controlled drug delivery by disrupting endothelial cell–cell junctions, Nat. Commun., 2017, 8, 15594 CrossRef CAS PubMed.
- B. Liu, W. Chen, B. D. Evavold, D. Brian and C. Zhu, Accumulation of Dynamic Catch Bonds between TCR and Agonist Peptide-MHC Triggers T Cell Signaling, Cell, 2014, 157, 357–368 CrossRef CAS.
- P. W. Goodwill, E. U. Saritas, L. R. Croft, T. N. Kim, K. M. Krishnan, D. V. Schaffer and S. M. Conolly, X-space MPI: magnetic nanoparticles for safe medical imaging, Adv. Mater., 2012, 24, 3870–3877 CrossRef CAS.
- M. Graeser, F. Thieben, P. Szwargulski, F. Werner, N. Gdaniec, M. Boberg, F. Griese, M. Moddel, P. Ludewig and D. van de Ven,
et al., Human-sized magnetic particle imaging for brain applications, Nat. Commun., 2019, 10, 1936 CrossRef CAS PubMed.
- F. Etoc, D. Lisse, Y. Bellaiche, J. Piehler, M. Coppey and M. Dahan, Subcellular control of Rac-GTPase signalling by magnetogenetic manipulation inside living cells, Nat. Nanotechnol., 2013, 8, 193–198 CrossRef CAS PubMed.
- J. H. Li, P. Santos-Otte, B. Au, J. Rentsch, S. Block and H. Ewers, Directed manipulation of membrane proteins by fluorescent magnetic nanoparticles, Nat. Commun., 2020, 11, 4259 CrossRef.
- K. Perica, A. Tu, A. Richter, J. G. Bieler, M. Edidin and J. P. Schneck, Magnetic Field-Induced T Cell Receptor Clustering by Nanoparticles Enhances T Cell Activation and Stimulates Antitumor Activity, ACS Nano, 2014, 8, 2252–2260 CrossRef CAS PubMed.
- C. S. Chiang, Y. J. Lin, R. Lee, Y. H. Lai, H. W. Cheng, C. H. Hsieh, W. C. Shyu and S. Y. Chen, Combination of fucoidan-based magnetic nanoparticles and immunomodulators enhances tumour-localized immunotherapy, Nat. Nanotechnol., 2018, 13, 746–754 CrossRef CAS.
- Y. N. Lin, T. J. Cradick, M. T. Brown, H. Deshmukh, P. Ranjan, N. Sarode, B. M. Wile, P. M. Vertino, F. J. Stewart and G. Bao, CRISPR/Cas9 systems have off-target activity with insertions or deletions between target DNA and guide RNA sequences, Nucleic Acids Res., 2014, 42, 7473–7485 CrossRef CAS PubMed.
- S. Tong, B. Moyo, C. M. Lee, K. Leong and G. Bao, Engineered materials for in vivo delivery of genome-editing machinery, Nat. Rev. Mater., 2019, 4, 726–737 CrossRef CAS PubMed.
- T. Iskratsch, H. Wolfenson and M. P. Sheetz, Appreciating force and shape-the rise of mechanotransduction in cell biology, Nat. Rev. Mol. Cell Biol., 2014, 15, 825–833 CrossRef CAS.
- M. E. Fernández-Sánchez, S. Barbier, J. Whitehead, G. Béalle, A. Michel, H. Latorre-Ossa, C. Rey, L. Fouassier, A. Claperon and L. Brullé,
et al., Mechanical induction of the tumorigenic β-catenin pathway by tumour growth pressure, Nature, 2015, 523, 92–95 CrossRef PubMed.
- A. R. Abdel Fattah, N. Kolaitis, K. Van Daele, B. Daza, A. G. Rustandi and A. Ranga, Targeted mechanical stimulation via magnetic nanoparticles guides in vitro tissue development, Nat. Commun., 2023, 14, 5281 CrossRef CAS PubMed.
- H. Shen, S. Tong, G. Bao and B. Wang, Structural responses of cells to intracellular magnetic force induced by superparamagnetic iron oxide nanoparticles, Phys. Chem. Chem. Phys., 2014, 16, 1914–1920 RSC.
- X. Yang, Z. Yi, Y. Liang and S. Tong, Magnetic Iron Oxide Nanoparticles Enhance Exosome Production by Upregulating Exosome Transport and Secretion Pathways, ACS Appl. Mater. Interfaces, 2024, 16, 67235–67245 CrossRef CAS PubMed.
- V. Emiliani, E. Entcheva, R. Hedrich, P. Hegemann, K. R. Konrad, C. Luscher, M. Mahn, Z. H. Pan, R. R. Sims and J. Vierock,
et al., Optogenetics for light control of biological systems, Nat. Rev. Methods Primers, 2022, 2, 55 CrossRef CAS PubMed.
- R. Kötitz, W. Weitschies, L. Trahms and W. Semmler, Investigation of Brownian and Néel relaxation in magnetic fluids, J. Magn. Magn. Mater., 1999, 201, 102–104 CrossRef.
- I. A. Brezovich and R. F. Meredith, Practical aspects of ferromagnetic thermoseed hyperthermia, Radiol. Clin. North Am., 1989, 27, 589–602 CrossRef CAS.
- R. Hergt and S. Dutz, Magnetic particle hyperthermia-biophysical limitations of a visionary tumour therapy, J. Magn. Magn. Mater., 2007, 311, 187–192 CrossRef CAS.
- R. E. Rosensweig, Heating magnetic fluid with alternating magnetic field, J. Magn. Magn. Mater., 2002, 252, 370–374 CrossRef CAS.
- P. Guardia, A. Riedinger, S. Nitti, G. Pugliese, S. Marras, A. Genovese, M. E. Materia, C. Lefevre, L. Manna and T. Pellegrino, One pot synthesis of monodisperse water soluble iron oxide nanocrystals with high values of the specific absorption rate, J. Mater. Chem. B, 2014, 2, 4426–4434 RSC.
- L. Lartigue, C. Innocenti, T. Kalaivani, A. Awwad, M. Sanchez Duque Mdel, Y. Guari, J. Larionova, C. Guerin, J. L. Montero and V. Barragan-Montero,
et al., Water-dispersible sugar-coated iron oxide nanoparticles. An evaluation of their relaxometric and magnetic hyperthermia properties, J. Am. Chem. Soc., 2011, 133, 10459–10472 CrossRef CAS PubMed.
- J. Carrey, B. Mehdaoui and M. Respaud, Simple models for dynamic hysteresis loop calculations of magnetic single-domain nanoparticles: Application to magnetic hyperthermia optimization, J. Appl. Phys., 2011, 109, 083921 CrossRef.
- E. M. Gubanova, R. A. Rytov and N. A. Usov, Dynamics of particles with cubic magnetic anisotropy in a viscous liquid, J. Magn. Magn. Mater., 2022, 541, 168494 CrossRef CAS.
- U. M. Engelmann, C. Shasha, E. Teeman, I. Slabu and K. M. Krishnan, Predicting size-dependent heating efficiency of magnetic nanoparticles from experiment and stochastic Néel-Brown Langevin simulation, J. Magn. Magn. Mater., 2019, 471, 450–456 CrossRef CAS.
- L. M. Bauer, S. F. Situ, M. A. Griswold and A. C. Samia, High-performance iron oxide nanoparticles for magnetic
particle imaging - guided hyperthermia (hMPI), Nanoscale, 2016, 8, 12162–12169 RSC.
- E. C. Abenojar, S. Wickramasinghe, J. Bas-Concepcion and A. C. S. Samia, Structural effects on the magnetic hyperthermia properties of iron oxide nanoparticles, Prog. Nat. Sci.: Mater. Int., 2016, 26, 440–448 CrossRef CAS.
- Z. Nemati, J. Alonso, L. M. Martinez, H. Khurshid, E. Garaio, J. A. Garcia, M. H. Phan and H. Srikanth, Enhanced Magnetic Hyperthermia in Iron Oxide Nano-Octopods: Size and Anisotropy Effects, J. Phys. Chem. C, 2016, 120, 8370–8379 CrossRef CAS.
- R. Das, J. Alonso, Z. N. Porshokouh, V. Kalappattil, D. Torres, M. H. Phan, E. Garaio, J. A. García, J. L. S. Llamazares and H. Srikanth, Tunable High Aspect Ratio Iron Oxide Nanorods for Enhanced Hyperthermia, J. Phys. Chem. C, 2016, 120, 10086–10093 CrossRef CAS.
- K. Y. Jiang, Q. B. Zhang, D. T. Hinojosa, L. L. Zhang, Z. Xiao, Y. Yin, S. Tong, V. L. Colvin and G. Bao, Controlled oxidation and surface modification increase heating capacity of magnetic iron oxide nanoparticles, Appl. Phys. Rev., 2021, 8, 031407 CAS.
- Y. Rabin, Is intracellular hyperthermia superior to extracellular hyperthermia in the thermal sense?, Int. J. Hyperthermia, 2002, 18, 194–202 CrossRef CAS PubMed.
- P. Moroz, S. K. Jones and B. N. Gray, Magnetically mediated hyperthermia: current status and future directions, Int. J. Hyperthermia, 2002, 18, 267–284 CrossRef CAS PubMed.
- J. R. Lepock, Cellular effects of hyperthermia: relevance to the minimum dose for thermal damage, Int. J. Hyperthermia, 2003, 19, 252–266 CrossRef CAS PubMed.
- B. Hildebrandt, P. Wust, O. Ahlers, A. Dieing, G. Sreenivasa, T. Kerner, R. Felix and H. Riess, The cellular and molecular basis of hyperthermia, Crit. Rev. Oncol. Hematol., 2002, 43, 33–56 CrossRef.
- C. A. Quinto, P. Mohindra, S. Tong and G. Bao, Multifunctional superparamagnetic iron oxide nanoparticles for combined chemotherapy and hyperthermia cancer treatment, Nanoscale, 2015, 7, 12728–12736 RSC.
- S. Giwa, J. K. Lewis, L. Alvarez, R. Langer, A. E. Roth, G. M. Church, J. F. Markmann, D. H. Sachs, A. Chandraker and J. A. Wertheim,
et al., The promise of organ and tissue preservation to transform medicine, Nat. Biotechnol., 2017, 35, 530–542 CrossRef CAS.
- J. K. Lewis, J. C. Bischof, I. Braslavsky, K. G. Brockbank, G. M. Fahy, B. J. Fuller, Y. Rabin, A. Tocchio, E. J. Woods and B. G. Wowk,
et al., The Grand Challenges of Organ Banking: Proceedings from the first global summit on complex tissue cryopreservation, Cryobiology, 2016, 72, 169–182 CrossRef.
- G. M. Fahy, B. Wowk, J. Wu, J. Phan, C. Rasch, A. Chang and E. Zendejas, Cryopreservation of organs by vitrification: perspectives and recent advances, Cryobiology, 2004, 48, 157–178 CrossRef CAS PubMed.
- H. Huang, X. He and M. L. Yarmush, Advanced technologies for the preservation of mammalian biospecimens, Nat. Biomed. Eng., 2021, 5, 793–804 CrossRef PubMed.
- R. Frederickson, Cryopreservation by vitrification, Nat. Biotechnol., 2000, 18, 250 Search PubMed.
- J. F. Peyridieu, A. Baudot, P. Boutron, J. Mazuer, J. Odin, A. Ray, E. Chapelier, E. Payen and J. L. Descotes, Critical cooling and warming rates to avoid ice crystallization in small pieces of mammalian organs permeated with cryoprotective agents, Cryobiology, 1996, 33, 436–446 CrossRef CAS.
- J. B. Hopkins, R. Badeau, M. Warkentin and R. E. Thorne, Effect of common cryoprotectants on critical warming rates and ice formation in aqueous solutions, Cryobiology, 2012, 65, 169–178 CrossRef CAS.
- N. Manuchehrabadi, Z. Gao, J. Zhang, H. L. Ring, Q. Shao, F. Liu, M. McDermott, A. Fok, Y. Rabin and K. G. Brockbank,
et al., Improved tissue cryopreservation using inductive heating of magnetic nanoparticles, Sci. Transl. Med., 2017, 9, eaah4586 CrossRef PubMed.
- M. L. Etheridge, K. R. Hurley, J. Zhang, S. Jeon, H. L. Ring, C. Hogan, C. L. Haynes, M. Garwood and J. C. Bischof, Accounting for biological aggregation in heating and imaging of magnetic nanoparticles, Technology, 2014, 2, 214–228 CrossRef.
- M. L. Etheridge, Y. Xu, L. Rott, J. Choi, B. Glasmacher and J. C. Bischof, RF heating of magnetic nanoparticles improves the thawing of cryopreserved biomaterials, Technology, 2014, 2, 229–242 CrossRef.
- Z. Ye, Y. Tai, Z. Han, S. Liu, M. L. Etheridge, J. L. Pasek-Allen, C. Shastry, Y. Liu, Z. Li and C. Chen,
et al., Engineering Magnetic Nanoclusters for Highly Efficient Heating in Radio-Frequency Nanowarming, Nano Lett., 2024, 24, 4588–4594 CrossRef CAS PubMed.
- S. Liu, Z. Han, Z. Ye, M. Jiang, M. L. Etheridge, J. C. Bischof and Y. Yin, Magnetic-Nanorod-Mediated Nanowarming with Uniform and Rate-Regulated Heating, Nano Lett., 2024, 24, 11567–11572 CrossRef CAS PubMed.
- Z. Gao, H. L. Ring, A. Sharma, B. Namsrai, N. Tran, E. B. Finger, M. Garwood, C. L. Haynes and J. C. Bischof, Preparation of Scalable Silica-Coated Iron Oxide Nanoparticles for Nanowarming, Adv. Sci., 2020, 7, 1901624 CrossRef CAS.
- A. Chiu-Lam, E. Staples, C. J. Pepine and C. Rinaldi, Perfusion, cryopreservation, and nanowarming of whole hearts using colloidally stable magnetic cryopreservation agent solutions, Sci. Adv., 2021, 7, eabe3005 CrossRef PubMed.
- Z. Han, J. S. Rao, L. Gangwar, B. E. Namsrai, J. L. Pasek-Allen, M. L. Etheridge, S. M. Wolf, T. L. Pruett, J. C. Bischof and E. B. Finger, Vitrification and nanowarming enable long-term organ cryopreservation and life-sustaining kidney transplantation in a rat model, Nat. Commun., 2023, 14, 3407 CrossRef CAS.
- S. A. Stanley, J. Sauer, R. S. Kane, J. S. Dordick and J. M. Friedman, Remote regulation of glucose homeostasis in mice using genetically encoded nanoparticles, Nat. Med., 2015, 21, 92–98 CrossRef CAS PubMed.
- S. Rao, R. Chen, A. A. LaRocca, M. G. Christiansen, A. W. Senko, C. H. Shi, P. H. Chiang, G. Varnavides, J. Xue and Y. Zhou,
et al., Remotely controlled chemomagnetic modulation of targeted neural circuits, Nat. Nanotechnol., 2019, 14, 967–973 CrossRef CAS.
- S. A. Hescham, P. H. Chiang, D. Gregurec, J. Moon, M. G. Christiansen, A. Jahanshahi, H. Liu, D. Rosenfeld, A. Pralle and P. Anikeeva,
et al., Magnetothermal nanoparticle technology alleviates parkinsonian-like symptoms in mice, Nat. Commun., 2021, 12, 5569 CrossRef CAS PubMed.
- M. A. Wheeler, C. J. Smith, M. Ottolini, B. S. Barker, A. M. Purohit, R. M. Grippo, R. P. Gaykema, A. J. Spano, M. P. Beenhakker and S. Kucenas,
et al., Genetically targeted
magnetic control of the nervous system, Nat. Neurosci., 2016, 19, 756–761 CrossRef CAS PubMed.
- X. Ma, X. Liang, Y. Li, Q. Feng, K. Cheng, N. Ma, F. Zhu, X. Guo, Y. Yue and G. Liu,
et al., Modular-designed engineered bacteria for precision tumor immunotherapy via spatiotemporal manipulation by magnetic field, Nat. Commun., 2023, 14, 1606 CrossRef CAS.
- J. T. Dias, M. Moros, P. Del Pino, S. Rivera, V. Grazu and J. M. de la Fuente, DNA as a molecular local thermal probe for the analysis of magnetic hyperthermia, Angew. Chem., Int. Ed., 2013, 52, 11526–11529 CrossRef CAS.
- L. Polo-Corrales and C. Rinaldi, Monitoring iron oxide nanoparticle surface temperature in an alternating magnetic field using thermoresponsive fluorescent polymers, J. Appl. Phys., 2012, 111, 07B334 CrossRef.
- A. Riedinger, P. Guardia, A. Curcio, M. A. Garcia, R. Cingolani, L. Manna and T. Pellegrino, Subnanometer local temperature probing and remotely controlled drug release based on azo-functionalized iron oxide nanoparticles, Nano Lett., 2013, 13, 2399–2406 CrossRef CAS PubMed.
- H. C. Davis, S. Kang, J. H. Lee, T. H. Shin, H. Putterman, J. Cheon and M. G. Shapiro, Nanoscale Heat Transfer from Magnetic Nanoparticles and Ferritin in an Alternating Magnetic Field, Biophys. J., 2020, 118, 1502–1510 CrossRef CAS.
- S. Faure, N. Mille, S. S. Kale, J. M. Asensio, J. Marbaix, P. Farger, D. Stoian, W. van Beek, P. F. Fazzini and K. Soulantica,
et al., Internal Temperature Measurements by X-Ray Diffraction on Magnetic Nanoparticles Heated by a High-Frequency Magnetic Field, J. Phys. Chem. C, 2020, 124, 22259–22265 CrossRef CAS.
- G. Wang, P. Zhang, S. K. Mendu, Y. Wang, Y. Zhang, X. Kang, B. N. Desai and J. J. Zhu, Revaluation of magnetic properties of Magneto, Nat. Neurosci., 2020, 23, 1047–1050 CrossRef CAS.
- K. Kole, Y. Zhang, E. J. R. Jansen, T. Brouns, A. Bijlsma, N. Calcini, X. Yan, A. D. S. Lantyer and T. Celikel, Assessing the utility of Magneto to control neuronal excitability in the somatosensory cortex, Nat. Neurosci., 2020, 23, 1044–1046 CrossRef CAS PubMed.
- F. X. Xu, L. Zhou, X. T. Wang, F. Jia, K. Y. Ma, N. Wang, L. Lin, F. Q. Xu and Y. Shen, Magneto is ineffective in controlling electrical properties of cerebellar Purkinje cells, Nat. Neurosci., 2020, 23, 1041–1043 CrossRef CAS PubMed.
- M. I. Brier, J. W. Mundell, X. Yu, L. Su, A. Holmann, J. Squeri, B. Zhang, S. A. Stanley, J. M. Friedman and J. S. Dordick, Uncovering a possible role of reactive oxygen species in magnetogenetics, Sci. Rep., 2020, 10, 13096 CrossRef CAS PubMed.
- P. T. Schumacker, Reactive oxygen species in cancer cells: live by the sword, die by the sword, Cancer Cell, 2006, 10, 175–176 CrossRef CAS.
- L. Tong, C. C. Chuang, S. Wu and L. Zuo, Reactive oxygen species in redox cancer therapy, Cancer Lett., 2015, 367, 18–25 CrossRef CAS.
- T. Mai and J. Z. Hilt, Functionalization of iron oxide nanoparticles with small molecules and the impact on reactive oxygen species generation for potential cancer therapy, Colloids Surf., A, 2019, 576, 9–14 CrossRef CAS.
- S. Fu, S. Wang, X. Zhang, A. Qi, Z. Liu, X. Yu, C. Chen and L. Li, Structural effect of Fe(3)O(4) nanoparticles on peroxidase-like activity for cancer therapy, Colloids Surf., B, 2017, 154, 239–245 CrossRef CAS PubMed.
- R. C. C. Costa, F. C. C. Moura, J. D. Ardisson, J. D. Fabris and R. M. Lago, Highly active heterogeneous Fenton-like systems based on Fe0/Fe3O4 composites prepared by controlled reduction of iron oxides, Appl. Catal., B, 2008, 83, 131–139 CrossRef CAS.
- Z. Yi, X. Yang, Y. Liang and S. Tong, Iron oxide nanozymes enhanced by ascorbic acid for macrophage-based cancer therapy, Nanoscale, 2024, 16, 14330–14338 RSC.
- Z. Chen, J. J. Yin, Y. T. Zhou, Y. Zhang, L. Song, M. Song, S. Hu and N. Gu, Dual enzyme-like activities of iron oxide nanoparticles and their implication for diminishing cytotoxicity, ACS Nano, 2012, 6, 4001–4012 CrossRef CAS PubMed.
- V. Trujillo-Alonso, E. C. Pratt, H. Zong, A. Lara-Martinez, C. Kaittanis, M. O. Rabie, V. Longo, M. W. Becker, G. J. Roboz and J. Grimm,
et al., FDA-approved ferumoxytol displays anti-leukaemia efficacy against cells with low ferroportin levels, Nat. Nanotechnol., 2019, 14, 616–622 CrossRef CAS PubMed.
- L. Wu, W. Wen, X. Wang, D. Huang, J. Cao, X. Qi and S. Shen, Ultrasmall iron oxide nanoparticles cause significant toxicity by specifically inducing acute oxidative stress to multiple organs, Part. Fibre Toxicol., 2022, 19, 24 CrossRef CAS.
- Y. Xie, D. Liu, C. Cai, X. Chen, Y. Zhou, L. Wu, Y. Sun, H. Dai, X. Kong and P. Liu, Size-dependent cytotoxicity of Fe3O4 nanoparticles induced by biphasic regulation of oxidative stress in different human hepatoma cells, Int. J. Nanomed., 2016, 11, 3557–3570 CrossRef CAS.
- M. A. Smith, P. L. Harris, L. M. Sayre and G. Perry, Iron accumulation in Alzheimer disease is a source of redox-generated free radicals, Proc. Natl. Acad. Sci. U. S. A., 1997, 94, 9866–9868 CrossRef CAS.
|
This journal is © The Royal Society of Chemistry 2025 |
Click here to see how this site uses Cookies. View our privacy policy here.