DOI:
10.1039/D4CE01250J
(Paper)
CrystEngComm, 2025,
27, 1736-1741
Fine-tuning of gas uptake and selectivity in a hexafluorozirconate pillared coordination network that features two porous phases†
Received
11th December 2024
, Accepted 6th February 2025
First published on 7th February 2025
Abstract
Hybrid coordination networks, sustained by divalent transition metal ions and a combination of organic and inorganic linker ligands, are an emerging class of physisorbents for adsorptive gas and vapour capture, especially under trace (≤1%) concentrations. Herein, we report a Cu(II) hybrid coordination network using the anionic pillar hexafluorozirconate ZrF62−, [Cu(pypz)2ZrF6]n; ZRFSIX-21-Cu (21 = 4-(3,5-dimethyl-1H-pyrazol-4-yl)pyridine). ZRFSIX-21-Cu possesses two ultramicroporous phases, a two-dimensional square lattice phase (α), and a three-dimensional primitive cubic unit phase (β), a rarity among hybrid ultramicroporous materials. Interestingly, ZRFSIX-21-Cu-α revealed better selectivity for C2H2 over CO2, and ZRFSIX-21-Cu-β for C2H2 over C2H4.
Introduction
Hybrid ultramicroporous materials (HUMs),1 a subfamily of hybrid coordination networks (HCNs), have emerged as a class of porous physisorbents suitable for gas separations.1–3 By taking advantage of ultramicroporous channels (<7 Å) and highly fluorinated pillars (SiF62−, TiF62−, etc.), HUMs offer strong electrostatic-rich high density of sorbate binding sites. Such strong binding often enables HUMs to demonstrate i) highly selective trace gas capture; ii) record-high binary and multicomponent gas separations (CO2/N2, C2H2/CO2, C2H2/C2H4; C2H2/C2H4/CO2, C2H2/C2H4/CH4, among others).2,4 Owing to their modular nature and amenability to the crystal engineering design principles, new HUMs can be readily synthesized.5 Simply put, fine-tuning of components within the core blueprint of HUMs enables superior control of their pore environment. This is key to optimising the resulting performance parameters,2 such as gas uptake, binding energy, selectivity, and separation potential.6–8 To optimise these aspects, several iterations of HUMs have been synthesized, often by varying the inorganic pillaring linkers. These include the first and second generation HUMs, namely, MFSIX (SiF62−, TiF62−, GeF62−),8,9DICRO (Cr2O72−),10MOFFIVE (NbOF52−, TaOF52−),11MFFIVE (AlF52−, FeF52−),12 and the mmo nets (CrO42−, MoO42−, WO42−).13
Traditionally, the above-mentioned HUMs relied upon pyrazine or pyridyl linkers to dictate pore size and/or promote interpenetration.7 The latest generation of HUMs have advanced this area by utilizing other N-heterocyclic derivatives, namely pyrazole. A recent report demonstrated the crystal engineering of a series of HUMs incorporating 4-(3,5-dimethyl-1H-pyrazol-4-yl) pyridine (pypz).14 Inclusion of this ligand into the HUM platform afforded materials possessing both high selectivity and working capacity of C2H2 over CO2.15 Additionally, use of such ligands has been found to promote increased hydrolytic stability, which has been a prevalent issue in both HUMs and metal–organic materials (MOMs), in general.16,17 Previous studies have shown that exposure to water or water vapour in HUMs typically leads to a structural transformation of the 3D pcu network to a 2D square lattice sql or sql-c* network. In the latter case, the square grids are interlocked, and the pillaring anions displaced, resulting in non-porous structures (Fig. 1).17,18
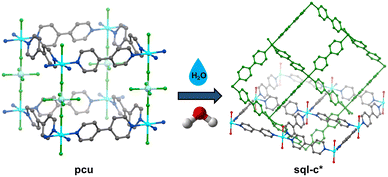 |
| Fig. 1 Illustration showing the phase transition of a prototypical pcu topology HUM to an sql-c* topology CN, in the presence of excess water. | |
To further explore the capabilities of the pypz ligand within a HUM platform, we report here the synthesis of [Cu(pypz)2ZrF6]n (ZRFSIX-21-Cu), a new HUM featuring the ZrF62− pillar. Unlike its parent HUM, SIFSIX-21-Cu,15ZRFSIX-21-Cu exists in two distinct ultramicroporous phases, a 2D square lattice (sql) α-phase and a conventional 3D pillared pcu β-phase. Strikingly, and different to other anion-pillared HUMs, ZRFSIX-21-Cu is shown also to be porous in its 2D phase, a first in this family of materials. The work here reveals the structural differences between the phases and the impact these have on the porosity and separation potential for different gas mixtures.
Results and discussion
Synthesis and structural characterization
Single crystals of ZRFSIX-21-Cu-α were isolated following the evaporation of an acetonitrile solution containing a mixture of pypz, Cu2+ and ZrF62−, whereas single crystals of ZRFSIX-21-Cu-β were obtained through layering the reagents atop each other in methanol at room temperature. Bulk samples of the α-phase were synthesized directly by mixing the reagents in acetonitrile, whereas methanol solvent afforded the β-phase (see synthesis section of ESI† for further details). Single-crystal X-ray diffraction (SC-XRD) revealed that ZRFSIX-21-Cu-β is isostructural to the previously reported pypz-based HUMs (SIFSIX-21-Cu, SIFSIX-21-Ni, etc.), crystallising as a pcu topology framework in the orthorhombic space group Pnna.15 Whereas ZRFSIX-21-Cu-α crystallises as an sql network in the orthorhombic space group Pna21, it can be characterised as an incomplete or intermediate phase in the formation of ZRFSIX-21-Cu-β. The key difference between the two phases is that ZRFSIX-21-Cu-α contains only one axially bound ZrF62− anion per Cu2+ node, rather than two, resulting in a square pyramidal {N4F} coordination chromophore around the Cu(II) centre (Fig. S1†). The absence of a second axial ZrF62− anion in ZRFSIX-21-Cu-α leads to sequential sql layers exhibiting AB stacking, instead of being locked into a pcu network (Fig. S2†).
Ultramicroporosity is present in both phases due to one-dimensional channels aligned along the crystallographic c-axis for α and b-axis for β, respectively. ZRFSIX-21-Cu-α was found to feature a minimum pore window of ca. 2.5 Å, and a maximum pore window of ca. 6.0 Å (Fig. 2a and b). In ZRFSIX-21-Cu-β, the pore window was determined at ca. 4.8 Å, comparable to the isostructural HUM, SIFSIX-21-Cu (4.5 Å), and TIFSIX-4-Cu (4.7 Å) (Fig. 2c).15 Interestingly, the pore chemistry of ZRFSIX-21-Cu-α resembles that of the previously reported sql HUM, sql-16-Cu-NO3-α (16 = 4,4′-(2,5-dimethyl-1,4-phenylene)dipyridine).19 In both cases, the pore window is partially occupied by the coordinating anion, causing the axial anions to interdigitate between the undulating 2D layers. The presence of the NO3− anion in sql-16-Cu-NO3-α contributed to strong sorbent–sorbate interactions observed for C2H2 compared with CO2,19 suggesting a similar effect might be observed with the ZrF62− anion in ZRFSIX-21-Cu-α.
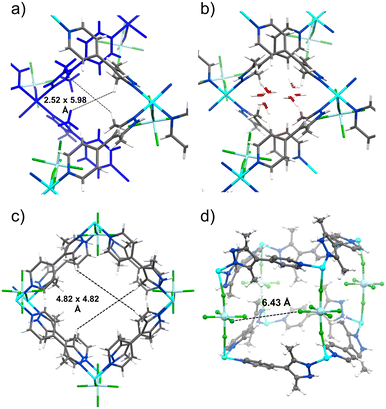 |
| Fig. 2 Crystal structures of ZRFSIX-21-Cu (α and β). (a) Ultramicropores of ZRFSIX-21-Cu-α generated through AB stacking of 2D layers. (b) Packing of the guest solvent molecules within the pores of ZRFSIX-21-Cu-α. (c) Ultramicropores of 3D ZRFSIX-21-Cu-β. (d) ZrF62− pillars of ZRFSIX-21-Cu-β used to generate the 3D structure and to potentially facilitate strong sorbate binding sites (due to short F–F distances between the neighbouring pillars). Colour code: Cu (cyan), Zr (pale blue), F (green), N (blue), C (grey) and H (white). | |
Powder X-ray diffraction (PXRD) was employed to confirm the bulk phase purity of ZRFSIX-21-Cu-α and β, as well as to verify the structural distinction between the two materials (sqlvs.pcu). The PXRD diffractogram recorded after air drying revealed that ZRFSIX-21-Cu-α exists in a desolvated α′ phase, as indicated by subtle differences between the simulated and experimental diffractograms. Thermogravimetric analysis (TGA) showed thermal decomposition occurring at 473 K and 538 K for ZRFSIX-21-Cu-α and ZRFSIX-21-Cu-β, respectively (Fig. S5†).
Phase transition studies
To establish the relationship between and interconversion of ZRFSIX-21-Cu-α and ZRFSIX-21-Cu-β phases, we conducted a series of experiments to track the transition between crystals of the α and β phases.
When soaked in a solution of MeOH, violet crystals of ZRFSIX-21-Cu-α were observed to slowly undergo a gradual phase transition to yield characteristic light-blue crystals of the ZRFSIX-21-Cu-β phase, over a period of 48 hours (Fig. S42†). PXRD measurements showed that in 24 hours, the sample comprised of a mixture of α and β phases, with complete conversion to ZRFSIX-21-Cu-β observed at 48 hours (Fig. 3). TGA measurements also confirmed successful phase transition as the decomposition profile complements that of the directly synthesized ZRFSIX-21-Cu-β (Fig. S5†). Attempts to reverse the phase change through heating crystals of ZRFSIX-21-Cu-β in acetonitrile or water to regenerate ZRFSIX-21-Cu-α were unsuccessful, suggesting thermodynamic stability of the β phase over the α phase.
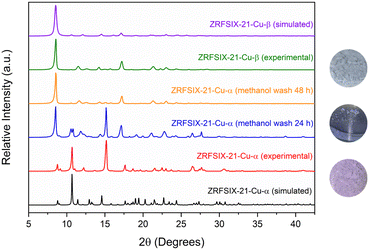 |
| Fig. 3 PXRD patterns of ZRFSIX-21-Cu. ZRFSIX-21-Cu-α (red) undergoes a phase transition to ZRFSIX-21-Cu-β (green) upon treatment with methanol, over a 48 hour period (blue and yellow). Optical images on the right illustrate colour change observed during the α to β phase transition. | |
Attempts were also made to induce a phase transition through direct heating of dried ZRFSIX-21-Cu-α to remove any residual solvent and potentially enable the ZrF62− anions to lock the 2D layers together, forming ZRFSIX-21-Cu-β. These efforts were inspired by our previous work on the HUM SIFSIX-3-Ni, where the 3D material was realised by heating the sql precursor at 80 °C under vacuum. Heating ZRFSIX-21-Cu-α at 120 °C under vacuum resulted in the formation of an additional poly-crystalline phase, ZRFSIX-21-Cu-γ (Fig. S6 and S8†), distinguishable by its green colour (Fig. 4). No phase transition was observed below 120 °C, with ZRFSIX-21-Cu-α remaining unchanged.
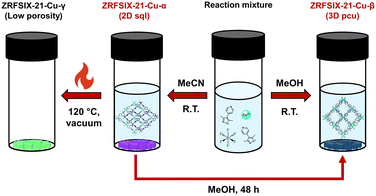 |
| Fig. 4 Reaction pathways for the synthesis of ZRFSIX-21-Cu-α, ZRFSIX-21-Cu-β and ZRFSIX-21-Cu-γ. | |
Gas adsorption studies
Single-component gas adsorption studies were conducted on all three phases to evaluate their porosity. Activation of ZRFSIX-21-Cu-α at 50 °C and 120 °C under vacuum generated the phases ZRFSIX-21-Cu-α′ and ZRFSIX-21-Cu-γ (Fig. S6†), while ZRFSIX-21-Cu-β was activated at room temperature under vacuum. N2 adsorption isotherms at 77 K revealed that both ZRFSIX-21-Cu-α′ and ZRFSIX-21-Cu-β displayed typical type I isotherms, with BET surface areas calculated at 367 m2 g−1 and 404 m2 g−1, respectively, whereas ZRFSIX-21-Cu-γ showed no N2 uptake (Fig. S9, S18 and S25†). Since ZRFSIX-21-Cu-γ was subjected to harsh activation at 120 °C under high vacuum, quality of the single crystals was no longer suitable for SC-XRD studies. Notably, the surface area of ZRFSIX-21-Cu-β was found to be markedly reduced compared to the other pypz-based HUMs, which typically report surface areas ranging from 747 m2 g−1 to 931 m2 g−1.14 CO2 isotherms at 195 K showed similar uptake levels and pore size distributions in ZRFSIX-21-Cu-α′ and ZRFSIX-21-Cu-β (Fig. S9 and S18†), whereas ZRFSIX-21-Cu-γ exhibited lower CO2 uptake at 195 K, resulting in a BET surface area of 343 m2 g−1 (Fig. S25†). In ZRFSIX-21-Cu-γ, the lack of N2 uptake and minimal CO2 uptake at 195 K were further supported by no uptake observed across several other gas isotherms at 298 K (Fig. S26–S29†). The conspicuously low BET surface areas of ZRFSIX-21-Cu-α′ and ZRFSIX-21-Cu-β, relative to other pypz-based HUMs, prompted us to explore their ability to adsorb CO2 and C2 gases under ambient conditions. C2H2, C2H4, and CO2 adsorption isotherms were measured at 273, 283, and 298 K for ZRFSIX-21-Cu-α′ and at 273 and 298 K for ZRFSIX-21-Cu-β. All measurements recorded at 273 K and above displayed type I isotherms.
ZRFSIX-21-Cu-α′ at ambient temperatures
At 1 bar and 298 K, the C2H2 uptake was 57.0 cm3 g−1, while the CO2 uptake was less than half this value, at 24.2 cm3 g−1 (Fig. 5a).
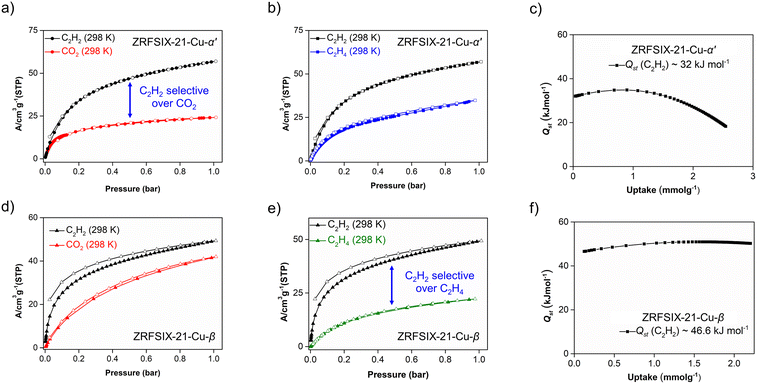 |
| Fig. 5 Adsorption isotherms of ZRFSIX-21-Cu-α′ for (a) C2H2 and CO2 at 298 K and (b) C2H2 and C2H4 at 298 K. Closed and open symbols represent adsorption and desorption, respectively. (c) C2H2 adsorption enthalpy for ZRFSIX-21-Cu-α′. Adsorption isotherms of ZRFSIX-21-Cu-β for (d) C2H2 and CO2 at 298 K and (e) C2H2 and C2H4 at 298 K respectively. (f) C2H2 adsorption enthalpy for ZRFSIX-21-Cu-β. | |
The C2H2/CO2 uptake ratio for ZRFSIX-21-Cu-α′ was calculated to be 2.4, comparable to previously reported sql networks UTSA-83a (3.1)20 and sql-16-Cu-NO3-α′ (2.1).19 Although it has a lower uptake ratio, ZRFSIX-21-Cu-α′ exhibits a much higher C2H2 uptake capacity, nearly five times that of UTSA-83a (57.0 cm3 g−1vs. 11.9 cm3 g−1).20 The isosteric enthalpies of adsorption (Qst) for C2H2 and CO2 were determined from the isotherms at 273, 283, and 298 K. Low-loading Qst values for C2H2 (32.0 kJ mol−1) and CO2 (22.8 kJ mol−1) were consistent with the significant difference in pure gas isotherm uptakes (Fig. 5c and S30†).
The selectivity of C2H2/CO2 was determined using IAST by fitting the pure gas isotherms to the dual-site Langmuir–Freundlich equation. For a binary mixture of C2H2/CO2 (1
:
1, v/v) at 1 bar and 298 K, the selectivity of C2H2/CO2 was 4.8 (Fig. S34†), which is lower than that of sql-16-Cu-NO3-α′ (27.8)19 and UTSA-83a (6.2),20 yet still higher than several 3D C2H2 adsorbents such as UTSA-220 (4.4),21FJU-89a (4.3),22ZNU-8 (3.7),23JNU-1 (3.0),24 and Zn-MOF-74 (2.0) (Fig. 6a).25 Notably, at 1 bar and 283 K, the selectivity of ZRFSIX-21-Cu-α′ increases significantly to 15.2, suggesting more favourable conditions for C2H2/CO2 separation. With its relatively low binding energy and high C2H2 capacity, ZRFSIX-21-Cu-α′ demonstrates potential as a versatile, C2H2-selective sorbent, with implications for mild regeneration conditions. Adsorption isotherms for C2H4 were also collected for ZRFSIX-21-Cu-α′, showing an uptake of 34.8 cm3 g−1 at 1 bar and 298 K and a C2H2/C2H4 uptake ratio of 1.6 (Fig. 5b). However, similar Qst values for C2H2 (32.0 kJ mol−1) and C2H4 (30.7 kJ mol−1) (Fig. 5c and S31†), along with a low selectivity of 1.7 (1
:
1, v/v), indicated that ZRFSIX-21-Cu-α′ is not well-suited for C2H2/C2H4 separation (Fig. S34†).
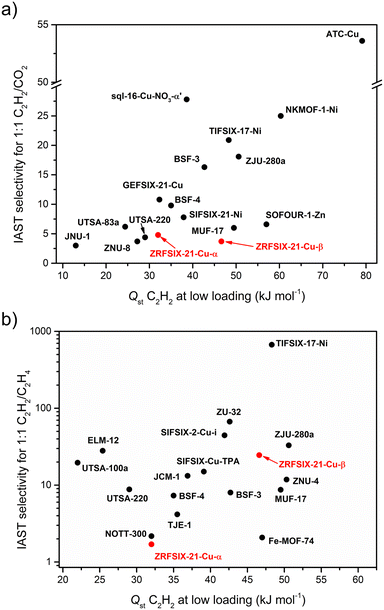 |
| Fig. 6 Comparison scatter plot of C2H2 heat of adsorption and IAST selectivity for a) C2H2/CO2 (1 : 1) and b) C2H2/C2H4 (1 : 1) of ZRFSIX-21-Cu-α and ZRFSIX-21-Cu-β with other top performing sorbents at 298 K. Molecular sieving materials have been omitted for clarity. | |
ZRFSIX-21-Cu-β at ambient temperatures
As observed in isostructural analogues, ZRFSIX-21-Cu-β also demonstrated a higher affinity for C2H2 over CO2, with uptakes of 49.4 cm3 g−1 and 42.0 cm3 g−1 at 1 bar and 298 K, respectively (Fig. 5d), and a significantly lower C2H4 uptake of 22.2 cm3 g−1 (Fig. 5e). Although the C2H2/CO2 uptake ratio for ZRFSIX-21-Cu-β is lower than that of ZRFSIX-21-Cu-α′ (1.2 vs. 2.4), ZRFSIX-21-Cu-β exhibited superior uptake kinetics for C2H2 relative to CO2. Calculated Qst values revealed binding energies of 46.6 and 28.3 kJ mol−1 for C2H2 and CO2 at zero loading, respectively (Fig. 5f and S32†). Compared to ZRFSIX-21-Cu-α′, ZRFSIX-21-Cu-β exhibits a much greater affinity for C2H2, with a difference of 14.6 kJ mol−1 in their C2H2 binding energies, while the CO2 values remain relatively similar. This change in Qst is likely due to differences in the pore environment between the α and β phases. Considering Qst values at half-loading, an important metric for assessing 1
:
1 C2H2/CO2 separations, the binding energy difference (ΔQst)C2H2/CO2 for ZRFSIX-21-Cu-β increases substantially to 28.5 kJ mol−1. This (ΔQst)C2H2/CO2 value exceeds those of many high-performing C2H2/CO2 selective materials, such as the TCuX series (X: Br, Cl, I; 5.5–8.2 kJ mol−1),26UTSA-74a (6.0 kJ mol−1),27NKMOF-1-Ni (9.5 kJ mol−1),28 and sql-16-Cu-NO3-α′ (11.4 kJ mol−1).19 Only benchmark materials such as ATC-Cu exhibit a greater (ΔQst)C2H2/CO2 at half-loading, a value heavily influenced by its exceptionally high binding energy for C2H2 (Qst = 79.1 kJ mol−1).29
As shown in previous studies of isostructural HUMs (e.g., SIFSIX-21-Ni, SIFSIX-21-Cu),15ZRFSIX-21-Cu-β benefits from multiple ZrF62− pillars and methyl groups that line the walls of the material. These features create strong electrostatic binding sites within the HUM, effectively trapping acetylene. ZRFSIX-21-Cu-α′ can be considered an “incomplete” phase of ZRFSIX-21-Cu-β. SC-XRD analysis reveals that the ZrF6 anions bound in ZRFSIX-21-Cu-α′ are isolated from neighbouring ZrF62− anions due to the interdigitation of sql layers, which could significantly reduce the effectiveness of the binding sites, limiting C2H2 interactions to a single ZrF62− pillar and weaker interactions with the ligand. Furthermore, the larger ZrF62− anion in ZRFSIX-21-Cu-β results in a greater protrusion of F atoms into the pore windows due to longer Zr–F bonds (2.015 Å) and short F–F distances (6.43 Å) between adjacent ZrF62− pillars (Fig. 2d), leading to a contracted pore volume and enhanced binding between sorbent and sorbate. IAST selectivity calculations for 1
:
1 binary mixture confirmed SC2H2/CO2 and SC2H2/C2H4 values of 3.7 and 24.6 at 1 bar and 298 K, respectively (Fig. S35†), with C2H2/CO2 selectivity comparable to ZRFSIX-21-Cu-α′ (SC2H2/CO2 (1
:
1) = 4.8). With a selectivity of 24.6, ZRFSIX-21-Cu-β is well-suited for C2H2/C2H4 separation. However, these values still lag a few HUM physisorbents and other materials (Fig. 6b), such as the benchmark material ZJU-300a.30 Additionally, SIFSIX-14-Cu-i and UTSA-300a exploit molecular sieving to achieve record-high selectivities.31,32
Conclusions
Ultramicroporous materials ZRFSIX-21-Cu-α, with a sql topology, and ZRFSIX-21-Cu-β, with a pcu topology, are additions to the MFSIX series of sorbents. Although they share an identical chemical formula, they form distinct structures due to variations in the Cu(II) coordination geometry and can be synthesised separately depending on the reaction conditions. Gas adsorption analysis revealed that both α and β phases are selective for C2H2, though unexpectedly, each favours a different gas pair. The α phase is more selective for C2H2 over CO2, while the β phase shows higher selectivity for C2H2 over C2H4. Notably, IAST calculations indicate that ZRFSIX-21-Cu-β exhibits excellent C2H2/C2H4 selectivity (24.6). These newly discovered materials as physisorbents exemplify the nuances of crystal engineering and underscore the value of tuneable phases for specific gas separations.
Data availability
The data supporting this article have been included as part of the ESI.† Crystallographic data for ZRFSIX-21-Cu-α and ZRFSIX-21-Cu-β can be accessed from the Cambridge Crystallographic Data Centre (CCDC) as deposition numbers 2408456 and 2408457, respectively.
Author contributions
Conceptualization, N. C. H. R., S. M., M. J. Z., and P. E. K.; methodology, N. C. H. R., H. S. S. and K. M. P.; investigation, N. C. H. R., H. S. S., K. M. P., N. K. and S. M.; formal analysis, N. C. H. R., K. M. P., N. K., C. H., S. M. and P. E. K.; data curation, N. C. H. R., K. M. P., N. K., C. H., S. M. and P. E. K.; writing – original draft, N. C. H. R., S. M. and P. E. K.; writing – review & editing, all authors; funding acquisition, S. M., M. J. Z., and P. E. K.; supervision, S. M. and P. E. K.
Conflicts of interest
There are no conflicts to declare.
Acknowledgements
This publication arises from research supported by Research Ireland (formerly known as the Science Foundation Ireland) awarded to S. M., under grant number 21/PATH-S/9454. For open access, the authors have applied a CC BY public copyright licence to any author-accepted manuscript version of this submission. M. J. Z. and S. M. thank the SSPC Reward funding, AzAds. P. E. K. gratefully acknowledges the MacDiarmid Institute for Advanced Materials and Nanotechnology, and the School of Physical and Chemical Science at the University of Canterbury for financial and instrumental support.
Notes and references
- S. Mukherjee and M. J. Zaworotko, Trends Chem., 2020, 2, 506–518 CrossRef CAS.
-
(a) S. Mukherjee, D. Sensharma, K.-J. Chen and M. J. Zaworotko, Chem. Commun., 2020, 56, 10419–10441 RSC;
(b) X. Liu, H. Wang, C. Liu, J. Chen, Z. Zhou, S. Deng and J. Wang, Chem Bio Eng., 2024, 1(6), 469–487 CrossRef CAS.
-
(a) Z. Ajoyan, P. Marino and A. J. Howarth, CrystEngComm, 2018, 20, 5899–5912 RSC;
(b) T. Wang, E. Lin, Y.-L. Peng, Y. Chen, P. Cheng and Z. Zhang, Coord. Chem. Rev., 2020, 423, 213485 CrossRef CAS;
(c) J. Dechnik, J. Gascon, C. J. Doonan, C. Janiak and C. J. Sumby, Angew. Chem., Int. Ed., 2017, 56, 9292–9310 CrossRef CAS PubMed;
(d) S. J. Datta, A. Mayoral, N. M. S. Bettahalli, P. M. Bhatt, M. Karunakaran, I. D. Carja, D. Fan, M. M. P. Graziane, R. Semino, G. Maurin, O. Terasaki and M. Eddaoudi, Science, 2022, 376, 1080–1087 CrossRef CAS PubMed.
-
(a) X. Li, H. Bian, W. Huang, B. Yan, X. Wang and B. Zhu, Coord. Chem. Rev., 2022, 470, 214714 CrossRef CAS;
(b) Y. Jiang, W. Yang, Y. Zhang, L. Wang and B. Chen, J. Mater. Chem. A, 2024, 12, 5563–5580 RSC.
-
(a) S. Subramanian and M. J. Zaworotko, Angew. Chem., Int. Ed. Engl., 1995, 34, 2127–2129 CrossRef CAS;
(b) W. Lu, Z. Wei, Z.-Y. Gu, T.-F. Liu, J. Park, J. Park, J. Tian, M. Zhang, Q. Zhang, T. Gentle, M. Bosch and H.-C. Zhou, Chem. Soc. Rev., 2014, 43, 5561–5593 RSC.
-
(a) K. Uemura, A. Maeda, T. K. Maji, P. Kanoo and H. Kita, Eur. J. Inorg. Chem., 2009, 16, 2329–2333 CrossRef;
(b) P. Nugent, Y. Belmabkhout, S. D. Burd, A. J. Cairns, R. Luebke, K. Forrest, T. Pham, S. Ma, B. Space, L. Wojtas, M. Eddaoudi and M. J. Zaworotko, Nature, 2013, 495, 80 CrossRef CAS PubMed;
(c) S. K. Elsaidi, M. H. Mohamed, H. T. Schaef, A. Kumar, M. Lusi, T. Pham, K. A. Forrest, B. Space, W. Xu, G. J. Halder, J. Liu, M. J. Zaworotko and P. K. Thallapally, Chem. Commun., 2015, 51, 15530–15533 RSC.
- A. Ebadi Amooghin, H. Sanaeepur, R. Luque, H. Garcia and B. Chen, Chem. Soc. Rev., 2022, 51, 7427–7508 RSC.
- X. Cui, K. Chen, H. Xing, Q. Yang, R. Krishna, Z. Bao, H. Wu, W. Zhou, X. Dong, Y. Han, B. Li, Q. Ren, M. J. Zaworotko and B. Chen, Science, 2016, 353, 141–144 CrossRef CAS PubMed.
- S.-i. Noro, S. Kitagawa, M. Kondo and K. Seki, Angew. Chem., Int. Ed., 2000, 39, 2081–2084 CrossRef PubMed.
-
(a) H. S. Scott, N. Ogiwara, K.-J. Chen, D. G. Madden, T. Pham, K. Forrest, B. Space, S. Horike, J. J. Perry IV, S. Kitagawa and M. J. Zaworotko, Chem. Sci., 2016, 7, 5470–5476 RSC;
(b) H. S. Scott, S. Mukherjee, D. R. Turner, M. I. J. Polson, M. J. Zaworotko and P. E. Kruger, CrystEngComm, 2018, 20, 1193–1197 RSC.
-
(a) A. Cadiau, K. Adil, P. M. Bhatt, Y. Belmabkhout and M. Eddaoudi, Science, 2016, 353, 137–140 CrossRef CAS PubMed;
(b) B. Gao, Z. Zhang, J. Hu, L. Yang, Y. Li, L. Chen, X. Cui and H. Xing, Chin. J. Chem. Eng., 2022, 42, 49–54 CrossRef CAS.
- A. Cadiau, Y. Belmabkhout, K. Adil, P. M. Bhatt, R. S. Pillai, A. Shkurenko, C. Martineau-Corcos, G. Maurin and M. Eddaoudi, Science, 2017, 356, 731–735 CrossRef CAS PubMed.
- M. H. Mohamed, S. K. Elsaidi, T. Pham, K. A. Forrest, H. T. Schaef, A. Hogan, L. Wojtas, W. Xu, B. Space, M. J. Zaworotko and P. K. Thallapally, Angew. Chem., Int. Ed., 2016, 55, 8285–8289 CrossRef CAS PubMed.
- N. Kumar, S. Mukherjee, N. C. Harvey-Reid, A. A. Bezrukov, K. Tan, V. Martins, M. Vandichel, T. Pham, L. M. van Wyk, K. Oyekan, A. Kumar, K. A. Forrest, K. M. Patil, L. J. Barbour, B. Space, Y. Huang, P. E. Kruger and M. J. Zaworotko, Chem, 2021, 7, 3085–3098 CAS.
- N. C. Harvey-Reid, D. Sensharma, S. Mukherjee, K. M. Patil, N. Kumar, S. J. Nikkhah, M. Vandichel, M. J. Zaworotko and P. E. Kruger, ACS Appl. Mater. Interfaces, 2024, 16, 4803–4810 CrossRef CAS PubMed.
- A. J. Howarth, Y. Liu, P. Li, Z. Li, T. C. Wang, J. T. Hupp and O. K. Farha, Nat. Rev. Mater., 2016, 1, 15018 CrossRef CAS.
- D. O'Nolan, A. Kumar and M. J. Zaworotko, J. Am. Chem. Soc., 2017, 139, 8508–8513 CrossRef PubMed.
- D. Sensharma, S. Vaesen, C. Healy, J. Hartmann, A. C. Kathalikkattil, P. Wix, F. Steuber, N. Zhu and W. Schmitt, Eur. J. Inorg. Chem., 2018, 2018, 1993–1997 CrossRef CAS.
- N. Kumar, S. Mukherjee, A. A. Bezrukov, M. Vandichel, M. Shivanna, D. Sensharma, A. Bajpai, V. Gascón, K. Otake and S. Kitagawa, SmartMat, 2020, 1, e1008 CrossRef.
- H. Cui, S. Chen, H. Arman, Y. Ye, A. Alsalme, R.-B. Lin and B. Chen, Inorg. Chim. Acta, 2019, 495, 118938 CrossRef CAS.
- H. Li, L. Li, R.-B. Lin, G. Ramirez, W. Zhou, R. Krishna, Z. Zhang, S. Xiang and B. Chen, ACS Sustainable Chem. Eng., 2019, 7, 4897–4902 CrossRef CAS PubMed.
- Y. Ye, S. Chen, L. Chen, J. Huang, Z. Ma, Z. Li, Z. Yao, J. Zhang, Z. Zhang and S. Xiang, ACS Appl. Mater. Interfaces, 2018, 10, 30912–30918 CrossRef CAS PubMed.
- Y. Zhang, W. Sun, B. Luan, J. Li, D. Luo, Y. Jiang, L. Wang and B. Chen, Angew. Chem., Int. Ed., 2023, 62, e202309925 CrossRef CAS PubMed.
- H. Zeng, M. Xie, Y. Huang, Y. Zhao, X. Xie, J. Bai, M. Wan, R. Krishna, W. Lu and D. Li, Angew. Chem., Int. Ed., 2019, 58, 8515–8519 CrossRef CAS PubMed.
- S. Xiang, W. Zhou, Z. Zhang, M. A. Green, Y. Liu and B. Chen, Angew. Chem., Int. Ed., 2010, 49, 4615–4618 CrossRef CAS PubMed.
- S. Mukherjee, Y. He, D. Franz, S. Wang, W. Xian, A. A. Bezrukov, B. Space, Z. Xu, J. He and M. J. Zaworotko, Chem. – Eur. J., 2020, 26, 4923–4929 CrossRef CAS PubMed.
- F. Luo, C. Yan, L. Dang, R. Krishna, W. Zhou, H. Wu, X. Dong, Y. Han, T.-L. Hu and M. O'Keeffe, J. Am. Chem. Soc., 2016, 138, 5678–5684 CrossRef CAS PubMed.
- Y.-L. Peng, T. Pham, P. Li, T. Wang, Y. Chen, K.-J. Chen, K. A. Forrest, B. Space, P. Cheng, M. J. Zaworotko and Z. Zhang, Angew. Chem., Int. Ed., 2018, 57, 10971–10975 CrossRef CAS PubMed.
- Z. Niu, X. Cui, T. Pham, G. Verma, P. C. Lan, C. Shan, H. Xing, K. A. Forrest, S. Suepaul, B. Space, A. Nafady, A. M. Al-Enizi and S. Ma, Angew. Chem., Int. Ed., 2021, 60, 5283–5288 CrossRef CAS PubMed.
- X. W. Gu, E. Wu, J. X. Wang, H. M. Wen, B. Chen, B. Li and G. Qian, Sci. Adv., 2023, 9, eadh0135 CrossRef CAS PubMed.
- B. Li, X. Cui, D. O'Nolan, H.-M. Wen, M. Jiang, R. Krishna, H. Wu, R.-B. Lin, Y.-S. Chen, D. Yuan, H. Xing, W. Zhou, Q. Ren, G. Qian, M. J. Zaworotko and B. Chen, Adv. Mater., 2017, 29, 1704210 CrossRef PubMed.
- R.-B. Lin, L. Li, H. Wu, H. Arman, B. Li, R.-G. Lin, W. Zhou and B. Chen, J. Am. Chem. Soc., 2017, 139, 8022–8028 CrossRef CAS PubMed.
Footnote |
† Electronic supplementary information (ESI) available: Experimental details, syntheses and characterisations: infrared spectra, thermogravimetric traces, powder X-ray diffractograms, gas sorption isotherms, etc. CCDC deposition entries 2408456 and 2408457. For ESI and crystallographic data in CIF or other electronic format see DOI: https://doi.org/10.1039/d4ce01250j |
|
This journal is © The Royal Society of Chemistry 2025 |
Click here to see how this site uses Cookies. View our privacy policy here.