DOI:
10.1039/D5DT00249D
(Frontier)
Dalton Trans., 2025,
54, 6005-6014
Chirality in molecular conductors from enantiopure or racemic coordination complexes
Received
30th January 2025
, Accepted 1st March 2025
First published on 12th March 2025
Abstract
There has been growing interest in recent years in the synthesis of multifunctional materials that exhibit both chirality and electrical conductivity. These materials can exhibit electrical magnetochiral anisotropy (eMChA) or the chirality induced spin selectivity (CISS) effect. Several families of chiral tetrathiafulvalene (TTF)-based donor molecules have been successfully used with acceptors or simple anions to prepare chiral molecular conductors. Chiral materials have also been obtained by employing racemic or enantiopure counter anions with chiral or achiral TTF donors. Most recently, enantiopure molecular conductors of bis(ethylenedithio)tetrathiafulvalene (BEDT-TTF) and 2,5-bis(1,3-dithiolan-2-ylidene)-1,3,4,6-tetrathiapentalene (BDH-TTP) have been obtained through chiral induction from a racemic mixture of a coordination complex, which provides the anion. This Frontier article provides an overview of chiral molecular conductors and a summary of progress to date. It highlights future perspectives on how chirality can be introduced into molecular conductors by employing enantiopure or racemic coordination complexes, which also have the potential to introduce magnetism into the multifunctional material.
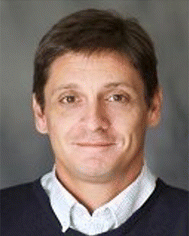 Lee Martin | Lee Martin received his PhD in chemistry in 1999 under the supervision of Prof. Peter Day at the Royal Institution of Great Britain. After working for the International Union of Crystallography and the Royal Society of Chemistry, he returned to the Davy-Faraday Research Laboratory at the Royal Institution of Great Britain as a postdoctoral research assistant in the group of Prof. Peter Day. Lee has been a lecturer at the University of Hertfordshire and Nottingham Trent University since 2007 and is currently an Associate Professor in Materials Chemistry at NTU. His research interests include the study of the structure and properties of chiral and 2D molecular conductors and quantum spin liquids in collaboration with researchers in Japan, the USA, and China. |
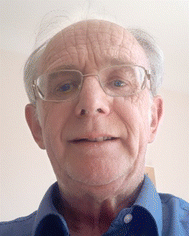 John D. Wallis | John Wallis was a Professor of Organic Chemistry at Nottingham Trent University for 20 years from 1999 and is now an Emeritus Professor. Prior to this, he was a Senior Lecturer in Chemistry at the University of Kent. His research interests combine organic synthesis and X-ray crystallography and include the determination of structures corresponding to partially complete organic reactions, the synthesis and the study of molecular organic conductors and molecular structures, and also the study of molecular structures in general. He has collaborated with groups in France, Canada and Japan and continues to do research. |
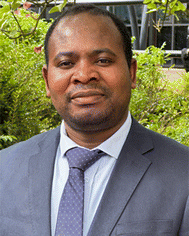 Joseph O. Ogar | Joseph Ogar became a member of the academic staff of the Department of Chemistry, University of Calabar, in 2013 after graduating from the University of Nigeria, Nsukka, in 2010. He completed his master's degree in inorganic chemistry at the University of Calabar in 2016 and his PhD in chemistry in the group of Neil Champness at the University of Nottingham in 2021. Most recently, he has worked as a post-doctoral fellow in the group of Dr Lee Martin and as a lecturer in chemistry at Nottingham Trent University, working on the synthesis of superconducting materials utilising various inorganic anions and bis(ethylenedithio)tetrathiafulvalene. |
Introduction
The effect of chirality on the electrical properties of a material has only recently been experimentally observed owing to the lack of enantiopure materials with there being no examples in nature. Of interest in chiral conductors are the physical phenomena of electrical magnetochiral anisotropy (eMChA)1 and the chirality induced spin selectivity (CISS) effect.2 Measuring the conducting behaviour of a material as a function of temperature in both enantiomeric forms as well as the racemate provides an understanding of the effects of chirality on the conductivity, or superconductivity, of a material.
eMChA occurs when a pair of isostructural enantiomeric materials, e.g. existing in enantiomorphic space groups, show different conductivities in an applied magnetic field depending upon their handedness and upon the direction of the electric current and the applied magnetic field. eMChA was first reported in 2001 in bismuth helices3 and 2002 in single-walled carbon nanotubes,4 and then in 2014 in the first molecular chiral conductor based on TTF,5 which will be the type of material that is the focus of this Frontier article (Fig. 1).
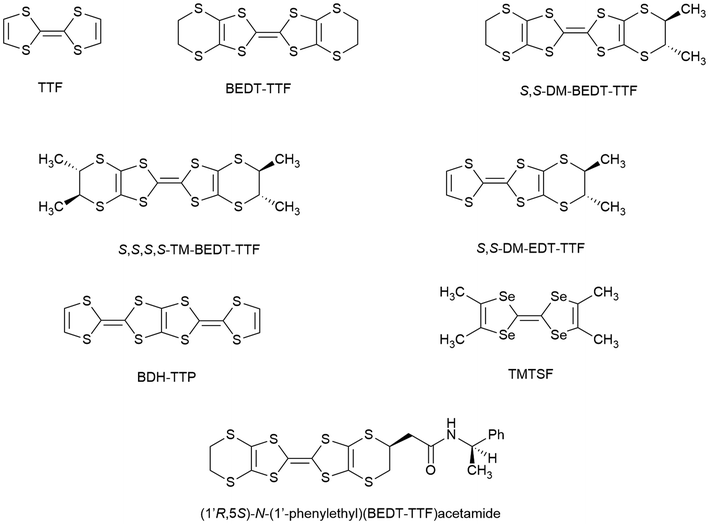 |
| Fig. 1 Donor molecules. | |
The effect of chirality on superconductivity was first reported in an individual nanotube of tungsten disulphide.6 In an achiral material, the current flows equally in both directions, but in WS2 nanotubes, the superconductivity has been shown to reflect the chiral structure where the forward and backward current flows are not equivalent in an applied magnetic field.6 Further reports of superconductivity in non-centrosymmetric materials7 provide an intriguing prospect for exploration of exotic superconducting properties, quantum phenomena and non-trivial Cooper pairing.
Another physical phenomenon of interest in chiral conducting materials is the CISS effect. First reported in 1999, the CISS effect has implications for many fields including enantioseparation, chiral spintronics, and electron-transfer processes.8 Electrons with a certain spin can traverse the material more easily depending on the handedness of the chiral material owing to the CISS effect. The CISS effect has recently been observed in a molecular superconductor derived from BEDT-TTF, which crystallises in space group P21, owing to the handedness of the relative arrangement of achiral anions and cations in κ-(BEDT-TTF)2Cu(NCS)2.9
Radical-cation salts
Molecular materials provide an advantage over traditional continuous-lattice solids because of their ability to achieve multifunctionality and enable observation of interplay between properties within the same material. Organic–inorganic hybrid salts consist of two molecular layers in the same single crystal, where each layer gives its own individual distinct properties to the material. Crystal engineering of TTF-based radical-cation salts has proved to be a valuable route to high-quality single crystals combining together chirality and conductivity in salts, which can be synthesised in both enantiomeric forms and as a racemate. These salts have produced a wide variety of electronic ground states and exhibit many interesting phenomena such as superconductivity, spin-Peierls transition and quantum spin-liquid behavior. The electrical conductivity arises from the inter-TTF sulphur–sulphur close contacts and the variety is due to the multitude of donor packing arrangements that are possible (Fig. 2). Radical-cation salts are built up of alternating stacks of conducting BEDT-TTF layers and insulating anion layers. Subtle changes in the packing of the anion layer can therefore have pronounced effects on the packing of the donor layer, e.g. through hydrogen-bonding interactions with the terminal ethylene groups of the BEDT-TTF molecules. The donor packing arrangement and sulphur–sulphur contacts and their electronic properties can be tuned by the size and shape of the anions and by their packing arrangement in the insulating layers of the salt.
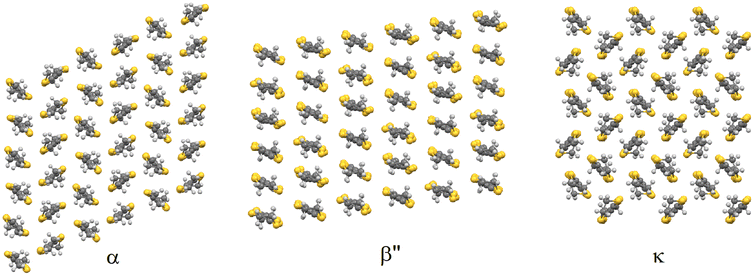 |
| Fig. 2 Alpha, beta′′, and kappa BEDT-TTF packing arrangements.10 | |
Since the discovery of the first metallic salt TTF-TCNQ (TCNQ = tetracyanoquinodimethane) in 1973
11 and the first molecular superconductor (TMTSF)2PF6 (TMTSF = tetramethyltetraselenafulvalene) in 1980,
12 a large number of organic donor molecules have been synthesised. The organic donor molecule BEDT-TTF has been the most extensively used in radical-cation salts because it has given a large number of superconductors with many simple and polyatomic anions as well as coordination complexes. The highest superconducting Tc values to date are 14.2 K under applied pressure for β′-(BEDT-TTF)2ICl213 and 11.6 K at ambient pressure for κ-(BEDT-TTF)2Cu[N(CN)2]Br.14
This Frontier article will present examples of four approaches that have been employed to combine chirality with conductivity in TTF-based radical-cation salts: using chiral TTF-based donors, chiral anions, and chiral guest solvent molecules or through chiral induction.
Chiral donor molecules
Using chiral donor molecules has proved to be the most promising route to introduce chirality into radical-cation salts.15 The first chiral TTF-type donor tetramethyl-(S,S,S,S)-BEDT-TTF (TM-BEDT-TTF)16 (Fig. 1) has produced a number of radical-cation salts and many other enantiopure TTF derivatives have been synthesised with stereogenic carbon or sulphur atoms,15 axial chirality, e.g. TTF-BINOLs,17 TTF-helicenes,18 or spiro-TTFs.19
The first, and to date only, observation of eMChA in a bulk molecular conductor was reported in 2014 in a pair of enantiopure salts, R,R and S,S, of DM-EDT-TTF (dimethylethylenedithiotetrathiafulvalene).4 The enantiomers of DM-EDT-TTF crystallised with the anion ClO4 in enantiomorphic space groups P6222 and P6422 and both were metals down to 40 K.
There are currently no chiral superconductors with chiral donor molecules – in 1992, κ-[(S,S)-DM-BEDT-TTF]2ClO4 was reported to show superconductivity under applied pressure, but this has recently been revisited and shown not to be superconductivity, but rather current induced charge order melting.20
The CISS effect has very recently been observed with enantiopure donor molecules DM-BEDT-TTF and TM-BEDT-TTF via electrochemistry.21 These enantiopure donor molecules have been chemisorbed onto gold and nickel surfaces to act as working electrodes in spin-dependent electrochemistry experiments. Spin-filtering ability is observed as a result of the CISS effect.22
There are also many examples of TTF derivatives with chiral side chains attached to one or both ends of the molecule, which can induce a chiral packing arrangement in the molecular material, such as helical crystal packing arrangements.15 The enantiopure donor (1′R,5S)-N-(1′-phenylethyl)(BEDT-TTF)acetamide (Fig. 1) produces a 4
:
1 salt with TCNQ, which is a metal down to at least 4.2 K, the lowest temperature observed for a chiral molecular metal. When this salt is warmed up above 283 K, it undergoes a transition from a metal to an insulator, granting it room-temperature switching capabilities.22
Chiral anions
Several racemic coordination complexes have been used as the anion in BEDT-TTF radical-cation salts. Racemic anions Fe(croconate)3,23 Cr(2,2′-bipy)(oxalate)2,24 Fe(chloranilate)3,25 Fe(chlorocyananilate)3,26 and TRISPHAT27,28 (Fig. 3) have produced racemic BEDT-TTF salts, which show semiconducting or insulating behaviour. The only example of an enantiopure coordination complex being employed is the antimony L-tartrate dianion (Fig. 3), which crystallises with BEDT-TTF as the semiconducting α-[ET]3[Sb2(L-tartrate)2]·CH3CN in the chiral space group P212121.29 The opposing enantiomer yielded α-[ET]3[Sb2(D-tartrate)2]·CH3CN, which was confirmed by circular dichroism to produce an identical, but opposite, spectrum.29 Both a chiral and a racemic BEDT-TTF salt have been obtained from the organic magnetic anion PROXYL-CONHCH2SO3 (PROXYL = 2,2,5,5-tetramethyl-pyrrolidin-1-oxyl).30 β′′-(BEDT-TTF)2(S- and rac-PROXYL-CONHCH2SO3) are metallic at room temperature and have similar broad metal–insulator transitions. The chiral salt shows resistivity three orders of magnitude higher than the racemic salt at 30 K, differing only in a deviation in the C–H bond direction at the chiral centre.
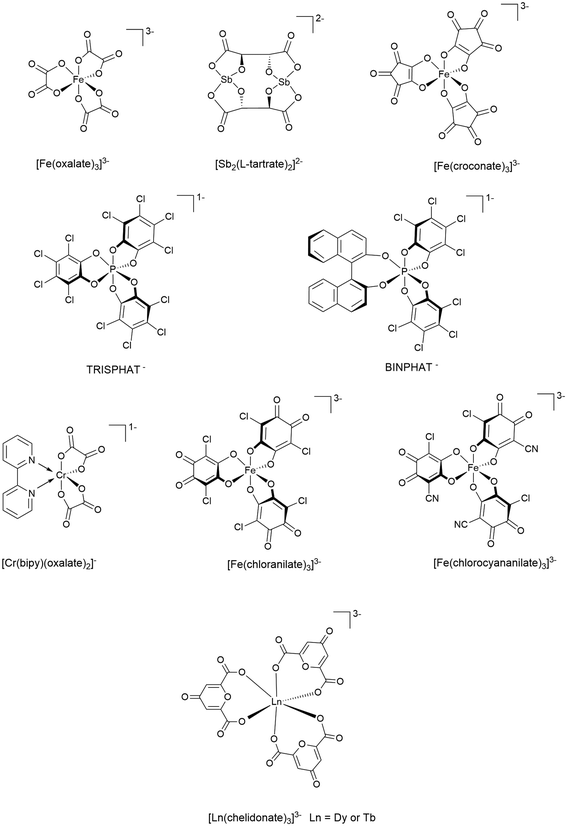 |
| Fig. 3 Chiral and racemic coordination complex anions. | |
Fe(chloranilate)3
31 and TRISPHAT28 have also crystallised with the enantiopure donor molecule TM-BEDT-TTF to produce chiral semiconductors, though in both cases the anion is a racemate in the radical-cation salt. Isostructural β-[TM-BEDT-TTF]3PPh4[KFe(chloranilate)3]·3H2O radical-cation salts have been synthesised with the TM-BEDT-TTF donor in enantiopure S,S,S,S- and R,R,R,R-forms and also as a racemic mixture, crystallising in the P1, P1 and P
space groups, respectively.31 The radical-cation salts S,S,S,S- and R,R,R,R-[TM-BEDT-TTF](rac-TRISPHAT)·2CH3CN crystallising in the space group P1 were obtained from racemic TRISPHAT. When starting from enantiopure Δ-TRISPHAT, the anion racemised under the conditions of electrochemical growth and both Δ and Λ TRISPHAT enantiomers were present to give the same structure as that obtained from the racemate.
Transition-metal coordination complexes, including the aforementioned iron- and chromium-based anions, have been used to introduce magnetism into (super)conducting radical-cation salts of BEDT-TTF. Tris(oxalato)metallate anions, M(C2O4)33− (Fe(C2O4)33− shown in Fig. 3), are the most commonly used in radical-cation salts of BEDT-TTF and have produced paramagnetic superconductors,32 ferromagnetic metals,33 and antiferromagnetic metals.34
M(C2O4)33− with a counter cation has the ability to form a hexagonal 2D insulating anion layer with the hexagons accommodating guest molecules (Fig. 4).32 It is possible to make small changes to the packing of the insulating anion layers to tune the desired magnetic and conducting properties.35 Changing the metal of M(C2O4)33− can change the magnetic properties of the material,35 and the bridging ability of the oxalato ligand can allow long-range magnetic order through the introduction of a second metal centre into the material.33 The two faces of a 2D insulating M(C2O4)33− anion layer are adjacent to conducting BEDT-TTF layers so that small changes in the structure of the M(C2O4)33− anion layer will lead to changes in the BEDT-TTF packing and affect the conducting properties (Fig. 5). Changing the metal of M(C2O4)33−, the counter cation (H3O+, K+, NH4+, and Na+), and in particular, the guest molecule in the hexagonal cavity (Fig. 5), has a marked effect on the packing and thus the conducting behaviour of the neighbouring BEDT-TTF layers (Fig. 6).35
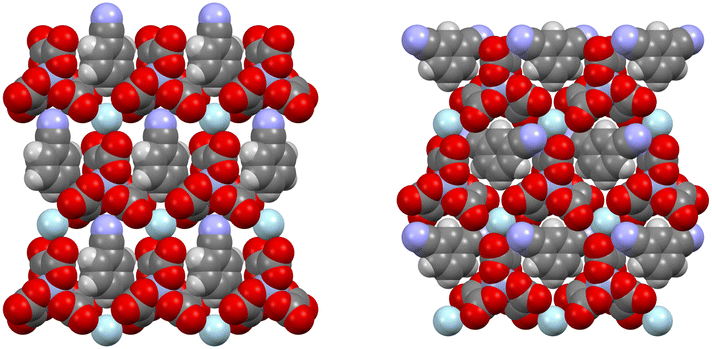 |
| Fig. 4 Hexagonal anion layer in the C2/c (left) and Pbcn (right) (BEDT-TTF)4[(A)M(C2O4)3]·GUEST salts. | |
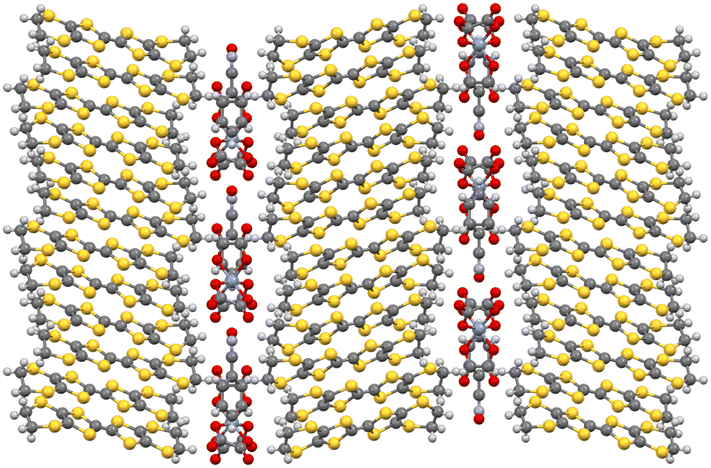 |
| Fig. 5 Layered structure of (BEDT-TTF)4[(A)M(C2O4)3]·GUEST salts. | |
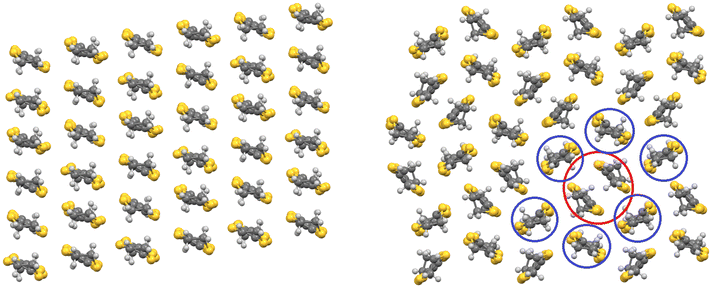 |
| Fig. 6 Different packing arrangements in the BEDT-TTF donor layer in the C2/c (left) and Pbcn (right) (BEDT-TTF)4[(A)M(C2O4)3]·GUEST salts. | |
In the superconducting series β′′-(BEDT-TTF)4[(A)M(C2O4)3]·GUEST (A = cation H3O+, NH4+, K+; M = Fe3+, Cr3+, Ga3+, Ru3+, Mn3+, Rh3+, Co3+, Al3+),35 crystallising in the monoclinic space group C2/c, the BEDT-TTF molecules pack in a β′′ motif (Fig. 6 left). The guest solvent molecule within the hexagonal cavity of the anion layer (e.g. benzonitrile in Fig. 4 left) has its –C
N bond aligned along the b axis directed towards the M(C2O4)33− metal centre. Including longer guest molecules increases the length of the b axis and this affects the neighbouring BEDT-TTF layers by increasing the superconducting Tc.36
Each anion layer in these superconducting salts consists of only a single enantiomer of M(C2O4)33− with the next layer consisting of only the opposite enantiomer to make the salt a racemate (Fig. 4 left and 5).32 Attempts to grow crystals of this salt starting from enantiopure Cr(C2O4)33− produced no crystals initially, but once racemisation occurred in solution, racemic crystals began to grow.37 However, these crystals were semiconducting polymorphs, crystallising in the space group Pbcn, owing to a different distribution of the enantiomers of M(C2O4)33− (Fig. 4 right). In the Pbcn polymorph, a single anion layer does not contain a single enantiomer of M(C2O4)33−, but instead, each layer consists of alternating rows of Δ and Λ enantiomers, and the lattice is racemic (Fig. 4 right). This subtle change in the distribution of the enantiomers in the anion layer affects the packing of the guest molecule with the –C
N group of the benzonitrile now disordered and directed towards the countercation instead of the M(C2O4)33− metal centre (Fig. 4 right). Despite both C2/c and Pbcn salts being racemates, the distribution of Δ and Λ enantiomers leads to markedly different BEDT-TTF packing motifs, which gives rise to superconducting or semiconducting behaviour. In the C2/c salt, the BEDT-TTF0.5+ donors pack in a β′′ motif, leading to metallic behaviour and superconductivity at low temperatures (Fig. 6 left), whilst in the Pbcn salt, BEDT-TTF1+ dimers (red circle, Fig. 6 right) are surrounded by BEDT-TTF0 monomers (blue circles, Fig. 6 right), resulting in semiconducting behaviour.36
A recent report presented the first radical-cation salt of BEDT-TTF with a racemic nine-coordinate propeller-shaped lanthanide-based anion, which offers the possibility of including not only chirality but also magnetism or luminescence into these multifunctional materials. The labile [Ln(chelidonato)3]3− anions (Ln = Dy or Tb) are present at a 50
:
50 ratio in the salt with the enantiomers segregated into rows within each anion layer (Fig. 7).38 The salt α-(BEDT-TTF)5Dy(chelidonato)3·EtOH·2H2O is a semiconductor, whilst the isomorphous Tb salt is a semimetal.
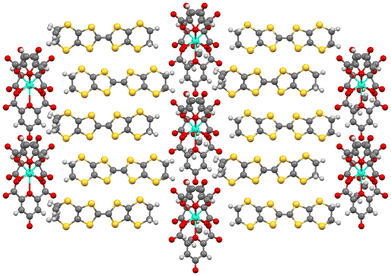 |
| Fig. 7 Layered structure of the α-(BEDT-TTF)5M(clo)3·EtOH·2H2O (M = Tb or Dy) salts. | |
Chiral guest molecules
A guest molecule is often included in these salts, which is the solvent used in the electrocrystallisation method of crystal growth. This limits the number of guest molecules that are possible to only those that can be used for electrocrystallisation. A chiral guest has been introduced using sec-phenethyl alcohol as both enantiopure (S)- and racemic (R/S)-forms.39sec-Phenethyl alcohol is asymmetrical and also a larger guest compared to those used in the aforementioned β′′-(BEDT-TTF)4[(A)M(C2O4)3]·GUEST superconducting salts (e.g. benzonitrile, bromobenzene, and nitrobenzene). The asymmetrical sec-phenethyl alcohol molecules do not sit centrally within the hexagonal cavities of the anion layer and protrude on just one side of the anion layer. This leads to different BEDT-TTF donor packing motifs on either side of each anion layer, one α and the other β′′. The enantiopure (S)- and racemic (R/S)-sec-phenethyl alcohol BEDT-TTF salts are isostructural but crystallise in P1 and P
space groups, respectively. In the enantiopure salt, only the (S)-sec-phenethyl alcohol is present in the hexagonal cavity, whilst in the racemic salt, both R and S enantiomers of sec-phenethyl alcohol are present but disordered at a ratio of 58.5
:
41.5. Both enantiopure and racemic salts exhibit metal–insulator behavior, but a more pronounced metal–insulator transition is observed in the racemic salt as a result of the structural disorder.39
Chiral induction
A series of BEDT-TTF/M(C2O4)33− salts has been obtained when using enantiopure (R)-(−)-carvone as a chiral guest molecule. Using (R)-(−)-carvone alone produced no crystals, possibly due to the large size of the guest not fitting within the hexagonal cavities in the 2D M(C2O4)33− layer. However, using (R)-(−)-carvone mixed with a second solvent leads to this second solvent entering the radical-cation salt as the guest molecule, and the presence of (R)-(−)-carvone leads to chiral induction in tris(oxalato)metallates.40 This series of salts contain either an enantiomeric excess of the complex anion or just the single enantiomer, Δ-M(C2O4)33−, despite starting from a racemic mixture. Crystallising in space groups P21, P212121, or P1, these chiral salts are semiconductors having a formula of (BEDT-TTF)3[(A)M(C2O4)3]·GUEST (M = Al3+, Cr3+; A = Li+, Na+, NH4+) (Fig. 8) with a 3
:
1 ratio of donor
:
anion, compared to the 4
:
1 ratio in superconducting salts.40
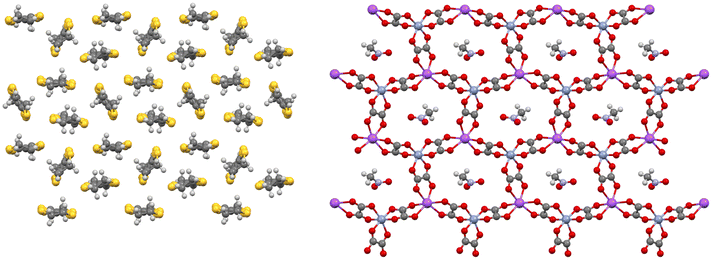 |
| Fig. 8 Donor (left) and anion (right) layers in the 3 : 1 salt (BEDT-TTF)3[NaCr(C2O4)3]·nitromethane. | |
Similarly, chiral induction has been observed when adding a chiral solid additive during the electrocrystallisation of radical-cation salts starting from a racemic mixture of Fe(C2O4)33−. The addition of enantiopure L-(+)-tartaric acid affords a semiconducting radical-cation salt α-(BEDT-TTF)5[D-Fe(C2O4)3]·[L-(+)-tartaric acid]2, which contains only the single enantiomer Δ-Fe(C2O4)3, crystallising in the space group C2.40 Using the same method with enantiopure (R)-(−)-3-hydroxytetrahydrofuran as the solid additive did not lead to resolution of the Fe(C2O4)3 anion but the additive was included in the lattice to afford semiconducting α-(BEDT-TTF)12[Fe(oxalate)3]2·(H2O)16·(ethanol)·(R)-(−)-3-hydroxytetrahydrofuran, crystallising in the space group P21.41
Spiroborate anions offer the possibility of more than one chiral centre: at the boron centre (if the bidentate ligand is asymmetrical) and on the ligand itself (if the ligand has a chiral centre). The huge pool of asymmetrical or chiral bidentate ligands available can lead to a great variety of sizes, shapes and packing modes, which has the potential to produce radical-cation salts with a wide variety of electrical properties and phenomena. Despite being labile at the boron centre, chiral crystallisation has been observed in radical-cation salts, which include only a single spiroborate enantiomer or a single pair of diastereomers.
The spiroborate anion B(malate)2− prepared from (R)-(−)-malic acid retains the (R) chirality on the malate ligands but is racemic at the boron centre, yielding the BSRR and BRRR enantiomers (Fig. 9). Upon electrocrystallisation with BEDT-TTF, the radical-cation salt contains only the BSRR enantiomer, crystallising in the space group P212121.42 When using racemic malic acid to synthesise the spiroborate anion, there are six enantiomers present: BRRR and BSRR with R-malate ligands; BRSS and BSSS with S-malate ligands; and BRRS and BSRS with a mixture of both R- and S-malate ligands. Electrocrystallisation of a mixture of these six enantiomers with BEDT-TTF produces a radical-cation salt, which contains only the diastereomeric pair BSRS and BRRS.42
 |
| Fig. 9 The two spiroborate anions BSRR (left) and BRRR (right) obtained using R-malic acid. | |
This preference for certain enantiomers or diastereomers over others during electrocrystallisation has also been observed in radical-cation salts of spiroborates with mandelate ligands.43 The first chiral radical-cation salt with the donor molecule BDH-TTP (Fig. 1) was grown from a racemic mixture of BS(S-chloromandelate)2 [BRSS and BSSS] to afford the radical-cation salt κ-BDH-TTP2[BS(S-chloromandelate)2], which includes only the BSSS enantiomer, crystallising in the space group P21.44 This salt is a metal down to at least 4.2 K, which is the lowest temperature that has been observed for a chiral molecular metal. Preference for certain enantiomers and diastereomers has also been observed in BEDT-TTF radical-cation salts of B(mandelate)2 and B(chloromandelate)2. When using enantiopure mandelate ligands, the crystals obtained via electrocrystallisation have a macromolecular helical morphology.43
Electrocrystallisation of enantiopure R,R- or S,S-B[1,1′-bis(BINOL)]2 with BEDT-TTF did not produce crystals, but starting from the racemic anion yielded crystals of the radical-cation salt (BEDT-TTF){B[1,1′-bis(BINOL)]2}·THF, which contains a 1
:
1 mixture of R,R- and S,S-B[1,1′-bis(BINOL)]2, but does not contain the meso-R,S-form.45
Recently, a new quantum spin-liquid candidate has been found in a BEDT-TTF salt with the spiroborate anion BR/S(salicylate)2.46 Despite first being proposed 50 years ago, the challenge of realizing a quantum spin liquid remains,47,48 which could be key to creating qubits for quantum computing or understanding the mechanism of high-temperature superconductivity.
Grown from racemic BR/S(salicylate)2, κ-(BEDT-TTF)2[BR/S(salicylate)2] (Fig. 10) is a 2D Mott insulator with a triangular lattice of BEDT-TTF molecules in the kappa packing arrangement (Fig. 2 right), crystallising in the space group P21 as an inversion twin. There have previously been a few 2D molecular quantum spin-liquid candidates with a triangular lattice and S = ½ spin system, but this is the first example to have no inversion symmetry centre, which may show novel behaviour due to the low symmetry. The 6 K anomaly in the specific heat capacity data is 2 or 3 times larger than those of previous molecular quantum spin-liquid candidates κ-(BEDT-TTF)2Cu2(CN)3 and EtMe3Sb[Pd(dmit)2]2.46 The anion layer in κ-(BEDT-TTF)2[BR/S(salicylate)2] consists of two rows of the spiroborate anion (Fig. 10), resulting in a 2D salt with widely separated conducting donor layers.
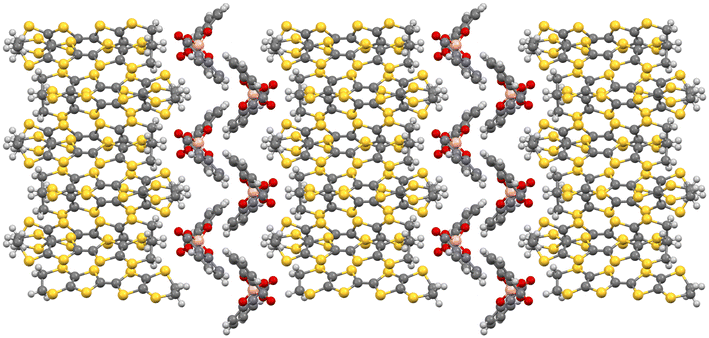 |
| Fig. 10 Double anion layer in κ-(BEDT-TTF)2[BR/S(salicylate)2]. | |
Conclusions and outlook
The question of whether chirality has an effect on the electrical properties of a material has only recently been experimentally investigated, owing to the lack of enantiopure conducting materials. Challenges with obtaining high-quality crystalline materials have been overcome to allow molecular conducting materials to be prepared from enantiopure donors, anions, or guest solvents, as well as through chiral induction. A chiral molecular superconductor has also crystallised in the space group P21 grown from an achiral donor and an achiral anion. Radical-cation salts offer the possibility of preparing families of materials in left-handed, right-handed, and racemic forms for direct comparison of their physical properties. Given the large number of radical-cation salts that have shown superconductivity over more than 40 years coupled with the multiple routes to introduce chirality into these systems highlighted in this Frontier article, there is a high likelihood that families of enantiopure metals, quantum spin-liquid candidates and superconductors can be discovered in this type of material.
Data availability
No new research data are presented in this Dalton Frontier article.
Conflicts of interest
There are no conflicts to declare.
Acknowledgements
LM and JOO would like to thank the Leverhulme Trust for financial support (RPG-2019-242).
References
- G. L. J. A. Rikken and N. Avarvari, J. Phys. Chem. Lett., 2023, 14, 9727–9731 CrossRef CAS PubMed.
- A. Carella, S. Mishra, C. Ferrari, D. Vanossi, F. Rossella, F. Pop, N. Avarvari, H. Htoon, J. A. Hollingsworth, E. G. Bowes, S. Majumder, A. Crandall Jones and C. Fontanesi, Nanoscale, 2025, 17, 2599–2607 RSC.
- G. L. J. A. Rikken, J. Fölling and P. Wyder, Phys. Rev. Lett., 2001, 87, 236602 CrossRef CAS.
- V. Krstic, S. Roth, M. Burghard, K. Kern and G. L. J. A. Rikken, J. Chem. Phys., 2002, 117, 11315–11319 CrossRef CAS; V. Krstić and G. L. J. A. Rikken, Chem. Phys. Lett., 2002, 364, 51–56 CrossRef.
- F. Pop, P. Auban-Senzier, E. Canadell, G. L. J. A. Rikken and N. Avarvari, Nat. Commun., 2014, 5, 3757 CrossRef PubMed.
- F. Qin, W. Shi, T. Ideue, M. Yoshida, A. Zak, R. Tenne, T. Kikitsu, D. Inoue, D. Hashizume and Y. Iwasa, Nat. Commun., 2017, 8, 14465 CrossRef CAS PubMed.
- E. M. Carnicom, W. Xie, T. Klimczuk, J. Lin, K. Gornicka, Z. Sobczak, N. P. Ong and R. J. Cava, Sci. Adv., 2018, 4, aar7969 CrossRef; R. Wakatsuki, Y. Saito, S. Hoshino, Y. M. Itahashi, T. Ideue, M. Ezawa, Y. Iwasa and N. Nagaosa, Sci. Adv., 2017, 3, e1602390 CrossRef PubMed.
- R. Naaman, Y. Paltiel and D. H. Waldeck, Acc. Chem. Res., 2020, 53, 2659–2667 CrossRef CAS PubMed.
- R. Nakajima, D. Hirobe, G. Kawaguchi, Y. Nabei, T. Sato, T. Narushima, H. Okamoto and H. M. Yamamoto, Nature, 2023, 613, 479–484 CrossRef CAS.
- T. Mori, Bull. Chem. Soc. Jpn., 1998, 71, 2509–2526 CrossRef CAS; T. Mori, H. Mori and S. Tanaka, Bull. Chem. Soc. Jpn., 1999, 72, 179–197 CrossRef.
- J. P. Ferraris, D. O. Cowan, V. Walatka and J. H. Perlstein, J. Am. Chem. Soc., 1973, 95, 948–949 CrossRef CAS.
- D. Jérome, A. Mazaud, M. Ribault and K. Bechgaard, J. Phys. Lett., 1980, 41, 95–98 Search PubMed.
- H. Taniguchi, M. Miyashita, K. Uchiyama, K. Satoh, N. Mori, H. Okamoto, K. Miyagawa, K. Kanoda, M. Hedo and Y. Uwatoko, J. Phys. Soc. Jpn., 2003, 72, 468–471 CrossRef CAS.
- A. M. Kini, U. Geiser, H. H. Wang, K. D. Carlson, J. M. Williams, W. K. Kwok, K. G. Vandervoort, J. E. Thompson and D. L. Stupka, Inorg. Chem., 1990, 29, 2555–2557 CrossRef CAS.
- N. Avarvari and J. D. Wallis, J. Mater. Chem., 2009, 19, 4061–4076 RSC.
- J. D. Dunitz, A. Karrer and J. D. Wallis, Helv. Chim. Acta, 1986, 69, 69–70 CrossRef.
- A. Saad, F. Barriere, E. Levillain, N. Vanthuyne, O. Jeannin and M. Fourmigue, Chem. – Eur. J., 2010, 16, 8020–8028 CrossRef CAS.
- T. Biet, A. Fihey, T. Cauchy, N. Vanthuyne, C. Roussel, J. Crassous and N. Avarvari, Chem. – Eur. J., 2013, 19, 13160–13167 CrossRef CAS PubMed.
- A. Bogdan, I.-T. Moraru, N. Vanthuyne, P. Auban-Senzier, I. Grosu, N. Avarvari and F. Pop, Chem. – Eur. J., 2024, e202400564 CrossRef CAS PubMed.
- N. Mroweh, C. Meziere, F. Pop, P. Auban-Senzier, P. Alemany, E. Canadell and N. Avarvari, Adv. Mater., 2020, 32, 2002811 CrossRef CAS PubMed.
- A. Stefani, A. Bogdan, F. Pop, F. Tassinari, L. Pasquali, C. Fontanesi and N. Avarvari, J. Chem. Phys., 2023, 159, 204706 CrossRef CAS.
- J. Short, T. J. Blundell, S. Krivickas, S. Yang, J. D. Wallis, H. Akutsu, Y. Nakazawa and L. Martin, Chiral Molecular Conductor With An Insulator-Metal Transition Close To Room Temperature, Chem. Commun., 2020, 56, 9497–9500 RSC.
- C. J. Gómez-García, E. Coronado, S. Curreli, C. Giménez-Saiz, P. Deplano, M. L. Mercuri, L. Pilia, A. Serpe, C. Faulmann and E. Canadell, Chem. Commun., 2006, 4931–4933 RSC.
- A. M. Madalan, E. Canadell, P. Auban-Senzier, D. Brânzea, N. Avarvari and M. Andruh, New J. Chem., 2008, 32, 333–339 RSC.
- S. Benmansour, E. Coronado, C. Giménez-Saiz, C. J. Gómez-García and C. Rößer, Eur. J. Inorg. Chem., 2014, 24, 3949–3959 CrossRef CAS; M. Atzori, F. Pop, P. Auban-Senzier, C. J. Gómez-García, E. Canadell, F. Artizzu, A. Serpe, P. Deplano, N. Avarvari and M. L. Mercuri, Inorg. Chem., 2014, 53, 7028–7039 CrossRef PubMed.
- S. A. Sahadevan, A. Abhervé, N. Monni, P. Auban-Senzier, J. Cano, F. Lloret, M. Julve, H. Cui, R. Kato, E. Canadell, M. L. Mercuri and N. Avarvari, Inorg. Chem., 2019, 58, 15359–15370 CrossRef CAS PubMed.
- M. Clemente-León, E. Coronado, C. J. Gómez-García, A. Soriano-Portillo, S. Constant, R. Frantz and J. Lacour, Inorg. Chim. Acta, 2007, 360, 955–960 CrossRef.
- F. Riobé, F. Piron, C. Réthoré, A. M. Madalan, C. J. Gómez-García, J. Lacour, J. D. Wallis and N. Avarvari, New J. Chem., 2011, 35, 2279–2286 RSC.
- E. Coronado, J. R. Galán-Mascarós, C. J. Gómez-García, A. Murcia-Martine and E. Canadell, Inorg. Chem., 2004, 43, 8072–8077 CrossRef CAS.
- H. Akutsu, A. Kohno, S. S. Turner, S. Yamashita and Y. Nakazawa, Mater. Adv., 2020, 1, 3171–3175 RSC.
- M. Atzori, F. Pop, P. Auban-Senzier, R. Clérac, E. Canadell, M. L. Mercuri and N. Avarvari, Inorg. Chem., 2015, 54, 3643–3653 CrossRef CAS.
- A. W. Graham, M. Kurmoo and P. Day, J. Chem. Soc., Chem. Commun., 1995, 2061–2062 RSC.
- E. Coronado, J. R. Galán-Mascarós, C. J. Gómez-García and V. Laukhin, Nature, 2007, 408, 447–449 CrossRef CAS PubMed; A. Alberola, E. Coronado, J. R. Galán–Mascarós, C. Giménez–Saiz and C. J. Gómez–García, J. Am. Chem. Soc., 2003, 125, 10774–10775 CrossRef PubMed.
- B. Zhang, Y. Zhang and D. Zhu, Chem. Commun., 2012, 48, 197–199 RSC.
- L. Martin, Coord. Chem. Rev., 2018, 376, 277–291 CrossRef CAS; S. Benmansour and C. J. Gómez-García, Magnetochemistry, 2021, 7, 93 CrossRef.
- S. Imajo, H. Akutsu, A. Akutsu-Sato, A. L. Morritt, L. Martin and Y. Nakazawa, Phys. Rev. Res., 2019, 1, 033184 CrossRef CAS.
- L. Martin, S. S. Turner, P. Day, K. M. A. Malik, S. J. Coles and M. B. Hursthouse, J. Chem. Soc., Chem. Commun., 1999, 513–514 RSC.
- E. Howarth, J. Lopez, J. O. Ogar, T. J. Blundell, H. Akutsu, Y. Nakazawa, S. Imajo, Y. Ihara, S. J. Coles, P. N. Horton, J. Christensen and L. Martin, Dalton Trans., 2025, 54, 3207–3215 RSC.
- L. Martin, P. Day, H. Akutsu, J.-i. Yamada, S.-i. Nakatsuji, W. Clegg, R. W. Harrington, P. N. Horton, M. B. Hursthouse, P. McMillan and S. Firth, CrystEngComm, 2007, 10, 865–867 RSC.
- L. Martin, S.-i. Nakatsuji, J.-i. Yamada, H. Akutsu and P. Day, J. Mater. Chem., 2010, 20, 2738–2742 RSC.
- J. O. Ogar, J. D. Wallis, T. J. Blundell, E. K. Rusbridge, A. Mantle, H. Akutsu, Y. Nakazawa, S. Imajo and L. Martin, Inorg. Chem., 2025, 64(2), 1075–1084 CrossRef CAS PubMed.
- J. R. Lopez, L. Martin, J. D. Wallis, H. Akutsu, Y. Nakazawa, J.-i. Yamada, T. Kadoya, S. J. Coles and C. Wilson, Dalton Trans., 2016, 45, 9285–9293 RSC.
- T. J. Blundell, K. Sneade, J. R. Lopez, J. D. Wallis, H. Akutsu, Y. Nakazawa, S. J. Coles, C. Wilson and L. Martin, Dalton Trans., 2022, 51, 4843–4852 RSC.
- T. J. Blundell, M. Brannan, H. Nishimoto, T. Kadoya, J.-i. Yamada, H. Akutsu, Y. Nakazawa and L. Martin, Chem. Commun., 2021, 57, 5406–5409 RSC.
- J. O. Ogar, T. J. Blundell, R. Usman, M. Vavrovič and L. Martin, Polyhedron, 2024, 264, 117262 CrossRef CAS.
- T. J. Blundell, K. Sneade, J. O. Ogar, S. Yamashita, H. Akutsu, Y. Nakazawa, T. Yamamoto and L. Martin, J. Am. Chem. Soc., 2025, 147(7), 5658–5668 CrossRef CAS.
- L. Balents, Nature, 2010, 464, 199–208 CrossRef CAS.
- S. Tang and X. Wang, Angew. Chem., Int. Ed., 2024, 63, e202310147 CrossRef CAS PubMed.
|
This journal is © The Royal Society of Chemistry 2025 |
Click here to see how this site uses Cookies. View our privacy policy here.