Payback trade-offs from the electrolyte design between energy efficiency and lifespan in zinc-ion batteries
Received
5th August 2024
, Accepted 14th November 2024
First published on 15th November 2024
Abstract
Aqueous zinc ion batteries (AZIBs) present a transformative avenue in electrochemical energy storage technologies, leveraging zinc anodes and aqueous electrolytes for safety and cost-effectiveness. The primary challenge of mitigating zinc dendrite formation in these batteries is addressed through electrolyte strategies, focusing on reducing water activities. Despite advancements in extending cycle life, a trade-off emerges between enhanced cycling performances and increased polarization, impacting energy efficiency. This often-overlooked concern becomes crucial when considering the payback period in energy storage systems. Experimental data illustrate the intricate relationship among electrolyte modifications, polarization, cycle life, and energy efficiency. The economic implications are scrutinized, emphasizing the need for a balanced approach in the electrolyte development to optimize service life without compromising energy efficiency. Striking this balance is imperative for the economic viability and environmental efficacy of AZIBs in sustainable energy storage solutions.
Broader context
Aqueous zinc-ion batteries (AZIBs) are revolutionizing electrochemical energy storage with zinc anodes and aqueous electrolytes, which offer substantial safety and cost benefits. Recent advancements address the critical issue of zinc dendrite formation by employing electrolyte modifications that reduce water activities, thereby enhancing cycle life. However, this improvement often comes at the cost of increased polarization, which affects energy efficiency and the economic viability. This opinion underscores the importance of considering not only technological advancements but also their broader economic and policy implications. By providing a detailed analysis of how electrolyte composition impacts energy efficiency and payback periods, this work offers valuable insights for AZIBs developers in long-term and short-term storage scenario. An effective balance of energy efficiency and cycle life can incentivize the adoption of AZIBs by addressing financial barriers and promoting investments in sustainable energy technologies. Additionally, the economic analysis highlights the potential for AZIBs to compete with established energy storage technologies like lithium-ion and lead-acid batteries, particularly in applications requiring high safety standards. The findings advocate for a balanced approach in electrolyte development, ensuring that advancements in cycle life do not compromise energy efficiency, thereby supporting the economic and environmental sustainability of energy storage solutions.
|
I. Introduction
Aqueous zinc ion batteries (AZIBs) represent a promising frontier in the realm of electrochemical energy storage technologies.1–5 These batteries, which utilize zinc as the anode material and aqueous electrolytes, have garnered significant attention due to their potential to revolutionize the energy landscape.6,7 AZIBs have emerged as a formidable contender in the pursuit of efficient, cost-effective, and environmentally friendly solutions. Unlike lithium-ion batteries (LIBs), AZIBs operate using an aqueous electrolyte, offering inherent advantages in terms of safety, cost-effectiveness, and ease of manufacturing.8 They hold immense promise for various applications, including renewable energy conversion integration, grid-level energy storage, and portable electronics.9 The primary challenge associated with aqueous electrolytes in zinc ion batteries is indeed preventing the formation and accumulation of zinc dendrites, as well as extending the electrochemically stable potential window (ESPW) to increase the energy density by alleviating hydrogen evolution reactions (HER) and oxygen evolution reactions (OER) and to reduce the accumulation of by-products.10 These factors can lead to short-circuits, reduced battery efficiency, and diminished cycle life. To address these challenges in aqueous electrolytes, strategies like “water-in-salt”, pH buffer, redox mediators, molecular crowding, and ionic liquid, etc. have been employed.11–13 These strategies have made great progress, increasing capacity retention and extending the cycle life of AZIBs, and preventing the formation of zinc dendrites and the generation of side reactions. In fact, the core of the strategies is to reduce the activity of free water in the electrolyte, reduce the overall reaction kinetics and obtain a long cycle life.14
While the modification of the electrolyte is introduced, the polarization during battery cycling is usually unavoidably increased, which causes some concerns about energy efficiency, but this has not attracted the attention of researchers.15 Energy efficiency refers to the ratio of energy output (measured in watt-hours, W h) during discharge to energy input (also measured in watt-hours, W h) during charge, and it is a critical factor in evaluating the overall performance and economic feasibility of energy storage systems.16,17 Energy efficiency is pivotal as it directly influences the payback period—the time needed for an energy storage system to recover its initial investment.18 This period is influenced not only by the technology itself but also by policies, complicating the adoption of less efficient solutions in cost-sensitive scenarios like peak-shaving. To assess the economic feasibility of energy storage systems, three primary indicators are commonly utilized: levelized cost of storage (LCOS), internal rate of return (IRR), and net present value (NPV).19 Given the differences in properties of energy storage solutions, it is impossible to consider energy storage for specific scenarios (such as those with high safety requirements) from solely an economic perspective. As a typical representative of emerging technology, AZIBs has the potential to adapt to high-safety energy storage scenarios. However, for new energy storage technologies, the payback period has an expected range. This is because among the commercialized technologies, LIBs, lead-acid batteries (LABs) and flow batteries have already made a distinction between short-term and long-term energy storage.20–22 New energy storage technologies need to gain advantages in payback period and economic cost in more segmented fields before they can be marketed and promoted. Usually, energy and power density, capacity retention, energy efficiency, financing conditions and policy incentives are comprehensively considered.18 As AZIBs are approaching commercialization, it is necessary for researchers to consider the actual market requirements and increase the consideration of energy efficiency while optimizing energy density, capacity retention and lifespan, especially in the electrolyte modification strategy.
In this perspective, we presented extensive experimental data examining the interplay between polarization, cycle life, and energy efficiency in popular electrolyte strategies. We also explore how energy efficiency impacts the payback period, with the aim of encouraging the development of more economically viable electrolyte solutions. By comparing the payback periods of current commercial energy storage technologies with AZIBs, we provide valuable insights for the future application scenarios of AZIBs. The issue of payback period considering energy efficiency is also discussed here to provide inspiration and design principles to researchers and engineers to develop more economically feasible electrolyte solutions.
II. Trade-offs of cycle life and energy efficiency in electrolyte strategies
Finely tuning the electrolyte composition stands as a practical approach, affording dynamic control over the electrode interface. In neutral or slightly acidic environments, zinc anode corrosion is exacerbated, attributed to the limited passivation capabilities of amphoteric zinc oxide (ZnO).23 To address this, strategies for aqueous electrolyte regulation encompass reduction of water content via high-concentration electrolytes, ionic liquids, and gel polymer electrolytes.24 An alternative avenue involves the integration of functional additives into existing aqueous electrolytes, aiming at displacing or occupying the solvation shell of zinc cations, thereby impeding water-induced corrosion on the zinc anode surface.25 This additive introduction expedites the de-solvation process of zinc-ions, averting direct contact with active water, rendering it an economically viable means of control. Presently, commercially employed electrolytes in aqueous zinc-ion batteries maintain a mildly acidic nature, exemplified by zinc sulfate (ZnSO4) and zinc trifluoromethanesulfonate [Zn(OTf)2], boosting ionic conductivities. Optimizing electrolyte compositions involves enhancing ionic conductivity while minimizing water reactivity and polarization. High-concentration electrolytes, ionic liquids, and functional additives can stabilize the zinc anode, reducing dendrite formation and corrosion. The electrolyte also plays a significant role in the overall system cost, especially considering the electrolyte-to-capacity (E/C) ratio, which directly affects energy density and efficiency. Since polarization directly affects both battery performance and the payback period, balancing cycle life with energy efficiency becomes essential. One strategy is to set acceptable thresholds for polarization and efficiency losses based on application requirements—short-term systems may prioritize higher energy efficiency, while long-term storage can accommodate some efficiency loss to extend cycle life. This framework helps us tailor electrolyte strategies to meet specific performance and economic goals, ensuring an optimized balance between these factors.
A series of experiments were conducted involving both adding Zn(OTf)2 and ZnSO4 into the electrolyte in varying proportions, while maintaining an overall zinc concentration of 2 M. For comparative purposes, cathode testing was performed using VO2 with an active material loading of 5 mg cm−2 to assess battery cycling performance as the incrementally augmented proportion of Zn(OTf)2 (Fig. 1a). While MnO2 is commonly used in AZIBs, it typically requires the addition of Mn2+ (like MnSO4) to stabilize cycling. This would interfere with our benchmark research model, so VO2 was chosen as the cathode material instead. The progressive increase in Zn(OTf)2 proportion correlated with an enhancement in full cell cycling performance. At a current density of 0.5 A g−1, 2 M Zn(OTf)2 yielded a 72% retention after 1000 cycles. When the Zn(OTf)2 proportion in the electrolyte stood at 75%, 50%, 25%, and 0%, the corresponding retention rates after 1000 cycles were 60%, 49%, 19%, and 18%, respectively, with the Coulombic efficiency are all close to 100% (Fig. 1b). This intriguing observation aligns with prior research, affirming Zn(OTf)2's efficacy in curbing vanadium oxide dissolution in AZIBs circulation by creating a water deficiency and Zn2+ rich Inner Helmholtz layer.26 Despite the notable retention improvement, a discernible inverse relationship was observed between energy efficiency during steady cycling and Zn(OTf)2 concentration. Notably, the electrolyte with 2 M ZnSO4 demonstrated the highest energy efficiency, surpassing 82% (Fig. 1b). This phenomenon is attributed to the escalating polarization evident in Fig. 1c. Given that the energy efficiency calculation hinges on the median voltage disparity between charge and discharge, heightened Zn(OTf)2 involvement leads to increased polarization and subsequently reduced energy efficiency. This trend is conspicuous in Zn‖Zn symmetric cells, where amplified Zn(OTf)2 concentration induces augmented polarization while significantly enhanced cycle life, as depicted in Fig. 1d. When performing charge and discharge tests at a current density of 1 mA cm−2 and a specific capacity of 1 mA h cm−2, the average overpotentials were approximately 0.0647 V, 0.0798 V, 0.1199 V, 0.1433 V, and 0.1484 V, respectively. In the Zn‖Zn symmetric cells employing 2 M ZnSO4 electrolyte, a short circuit was initially observed, yet it exhibits a minimal overpotential. Attributed to the irreversible deposition of zinc on the electrodes, the overpotentials of Zn‖Zn symmetric cells experience a gradual reduction over successive charge and discharge cycles. Conversely, the half-cell utilizing 2 M Zn(OTf)2 electrolyte demonstrates stable cycling for more than 1000 hours, accompanied by the highest observed polarization.
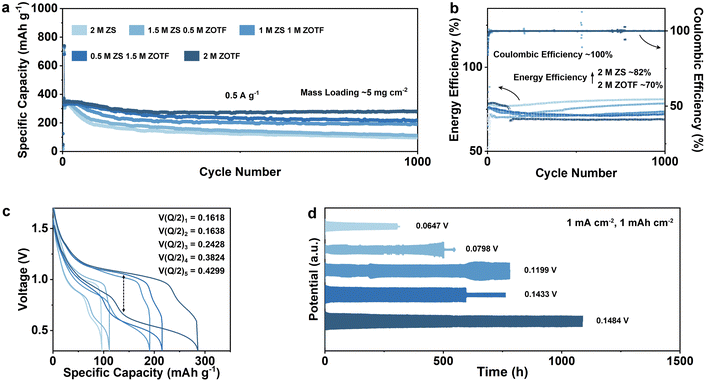 |
| Fig. 1 (a) Galvanostatic cycling tests on full cells with the VO2 cathode in different electrolytes, (b) corresponding energy efficiency and Coulombic efficiency in the stable cycles between 1st to 1000th cycles, (c) corresponding electrochemical profiles at the 1000th cycle. The mass loading of the cathode material in full cells is ∼5 mg cm−2. (d) Cycling performance of Zn‖Zn symmetric cells, marked with average overpotentials, at 1 mA cm−2/1 mA h cm−2 of 2 M ZnSO4, 1.5 M ZnSO4 + 0.5 M Zn(OTf)2, 1 M ZnSO4 + 1 M Zn(OTf)2, 0.5 M ZnSO4 + 1.5 M Zn(OTf)2, and 2 M Zn(OTf)2, respectively. | |
However, the cost of raw materials for Zn(OTf)2 (USD 6.09 g−1vs. USD 0.06 g−1 for ZnSO4 from Sigma–Aldrich) has been a persistent concern. As an alternative, additives are employed to facilitate the smooth deposition of Zn2+ during cycling, ensuring sustained long-term stability. Here, selected organic additive solutions are also comparatively introduced to attenuate overall reaction kinetics, aiming to improve long-term stability. In this comparative study, a 2 M ZnSO4 solution was utilized as a reference electrolyte, supplemented with 0.1 M of each electrolyte additive, polypropylene glycol (PPG), tetraethylammonium bromide (TEAB), disodium glycerophosphate (DG), and tetrabutylammonium bromide (TBAB). Fig. 2a illustrates the full cell tests employing a VO2 cathode with a loading of ∼5 mg cm−2, followed by extended cycling under a low current density condition of 0.1 mA g−1. Among them, PPG, TEAB, and TBAB were more effective than the bare one in maintaining reversible cycling of full cells. Fig. 2b, however, reveals that all additives significantly diminish energy efficiency in the period of stable cycles.
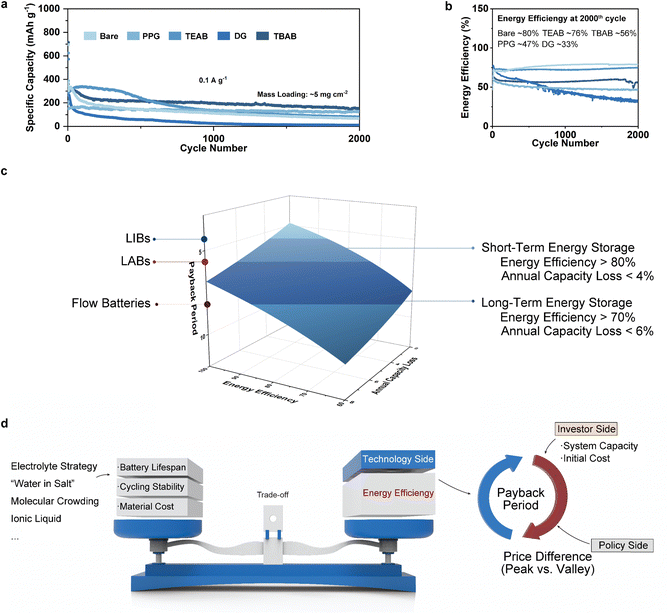 |
| Fig. 2 (a) Galvanostatic cycling tests on full cells with VO2 cathodes, and (b) corresponding energy efficiency in stable cycles between 1st and 1500th cycle of 2 M ZnSO4 with PPG, TEAB, DG, and TBAB. (c) Payback period requirements for AZIB development compared to commercial energy storage solutions. (d) Scheme of how the trade-off between electrolyte strategy and energy efficiency affects the payback period of energy system with a comprehensive consideration among technology, capital cost, and policy. | |
III. Considering the payback period
Commencing with a forward-looking perspective, it is imperative to assess the economic implications of the electrolyte solution, given its pivotal influence on the ultimate market viability of this technology. Prevailing energy storage system operation predominantly follows an investment-first, return-later model, leveraging disparities in peak and off-peak electricity pricing for profitability.27 Prior to committing to an energy storage system, the initial capital outlay and capacity of the system delineate the approximate payback period. Regional policy dynamics further accentuate the peak-to-trough price differential, thereby shaping the expeditiousness of capital recuperation.28 Returning to the technical domain, factors intrinsic to the system such as energy efficiency, annual capacity loss, lifespan, discharge depth, and operational expenses collectively underpin the pace of cost recovery. In instances where energy efficiency is notably deficient, there arises a legitimate query regarding the feasibility of cost recuperation within the system's operational lifespan. The payback period can be simplified and calculated as eqn (1), | 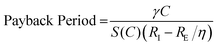 | (1) |
where γC is the initial cost of energy storage technologies, S(C) is the energy stored and retrieved from the energy storage with a capacity of C, RI is the cost of imported energy per KWh, and RE is the price paid for exported electricity per KWh, and η is the energy efficiency of the energy storage system. Considering a case of 1 MW h (initial cost of USD 224
320) energy storage system as a case study and adopting the prevailing two-charge and two-discharge policy along with the current electricity prices in May 2024 in Zhejiang, China, the peak electricity price stands at USD 0.150 per W h. Additionally, the normal electricity price is USD 0.094 per W h, and the off-peak price is USD 0.042 per W h. Employing an 8% discount rate and assuming a 2% depreciation rate annually, the calculations, based on a system efficiency of 80%, yield an IRR of 20.68% and a payback period of 6.13 years. However, variations in system efficiency have a notable impact on economic viability. When the efficiency decreases to 75%, 70%, 65%, and 60%, the payback periods increase significantly to 6.56, 7.06, 7.62, and 8.28 years, respectively. Despite the claim of a 15-year lifespan for common energy storage systems, practical issues such as poor maintenance and substantial real attenuation often lead to decommissioning after around 10 years. There is a general agreement that the energy efficiency of AZIBs may not surpass that of LIBs. AZIBs are likely to penetrate the LIBs market only if their production costs can match those of LIBs in the future. Currently, the market expectation is that the payback period for LIBs is ∼4 years. AZIBs, with their aqueous electrolyte, offer enhanced safety, a significant advantage over LIBs. This safety feature also makes them a competitor to LABs, which, despite being a traditional choice for safety-critical applications, have not fully met market expectations due to their lower performance. Although LABs are inexpensive, their need for frequent maintenance and the risk of cell failure pose significant drawbacks, leading to an expected payback period of more than 5.7 years. In the case of AZIBs, being an aqueous battery, they must demonstrate competitiveness at least against traditional LABs, especially in terms of economic viability. In the realm of long-term energy storage, the substantial manufacturing and maintenance expenses associated with flow batteries lead to an anticipated payback period of ∼8.7 years. Assuming the system cost of AZIBs aligns with that of LIBs ($0.2 per W h), Fig. 2c shows the projected payback periods for AZIBs based on their energy efficiency and annual capacity loss. To meet payback period requirements, AZIBs should maintain at least 80% energy density with less than 4% annual capacity loss for short-term storage applications. For long-term storage, they should achieve at least 70% energy density with less than 6% annual capacity loss. These criteria will be key for AZIBs to become a viable option in both short-term and long-term energy storage markets.
As demonstrated in Fig. 2d, in the endeavor towards electrolyte advancement, a meticulous evaluation of its influence on energy efficiency is imperative, given its substantial bearing on the payback period—a pivotal metric in the economic assessment of energy storage technologies. Historically, researchers around the electrolyte design have predominantly concentrated on augmenting the operational lifespan of energy storage systems, recognizing that an extended service life facilitates a more protracted utilization cycle, thereby amortizing the initial capital outlay over an elongated temporal horizon (i.e., reducing LCOS). Nevertheless, some electrolyte modification methods, albeit geared towards enhancing performance, have been observed to instigate heightened polarization, consequently diminishing energy efficiency, and perturbing the payback period—an aspect that has hitherto received comparatively less attention from the research community. Ideally, an optimal equilibrium can be sought in the trade-off between prolonging service life and mitigating energy efficiency losses within a tolerable threshold. An electrolyte characterized by elevated ionic conductivity, diminished internal resistance, and the capacity to stabilize free water content is envisaged, ensuring an optimum cadence of charge and discharge cycles. This strategic selection serves to curtail energy dissipation while optimizing the overall efficiency of the system.
IV. Conclusion
In conclusion, it is paramount for researchers in the field of aqueous electrolyte development to conscientiously consider the trade-off between extending service life and preserving energy efficiency. While efforts to enhance longevity and capacity are commendable, modifications must be approached judiciously, as they can inadvertently amplify polarization, impinging on energy efficiency and investment return periods. Striking an optimal balance is essential. By prioritizing energy efficiency, researchers can advance sustainable energy storage solutions with greater economic viability and environmental efficacy. In short-term energy storage, AZIBs have clear economic advantages over LIBs, owing to lower safety costs and simpler system requirements. Although LIBs currently offer quicker payback periods, enhancing AZIB energy efficiency could significantly improve their competitiveness in targeted applications, reinforcing their role in the energy storage market. Additionally, addressing potential energy losses during rest periods caused by side reactions will further enhance the long-term performance of AZIBs.
Data availability
The data that support the findings of this study are available on request from the corresponding author upon reasonable request.
Conflicts of interest
The authors declare no conflicting interests regarding the content of this article.
Acknowledgements
X. G., H. D., C. S., Y. D. contributed equally to this work. Xuan Gao thanked the industrial support from Vastech-Energy and funding support from China Scholarship Council/University College London for the joint PhD scholarship. The authors would like to acknowledge Engineering and Physical Sciences Research Council (EP/V027433/3) and UK Research and Innovation (UKRI) under the UK government's Horizon Europe funding guarantee (101077226; EP/Y008707/1) for funding support.
References
- B. Tang, L. Shan, S. Liang and J. Zhou, Issues and opportunities facing aqueous zinc-ion batteries, Energy Environ. Sci., 2019, 12, 3288–3304 RSC.
- T. Bashir, S. Zhou, S. Yang, S. A. Ismail, T. Ali, H. Wang, J. Zhao and L. Gao, Progress in 3D-MXene Electrodes for Lithium/Sodium/Potassium/Magnesium/Zinc/Aluminum-Ion Batteries, Electrochem. Energy Rev., 2023, 6, 5 CrossRef CAS.
- J. Zhang, W. Li, J. Wang, X. Pu, G. Zhang, S. Wang, N. Wang and X. Li, Engineering p-Band Center of Oxygen Boosting H+ Intercalation in δ-MnO2 for Aqueous Zinc Ion Batteries, Angew. Chem., Int. Ed., 2023, 62, e202215654 CrossRef CAS PubMed.
- Y. Xu, G. Zhang, J. Liu, J. Zhang, X. Wang, X. Pu, J. Wang, C. Yan, Y. Cao, H. Yang, W. Li and X. Li, Recent Advances on Challenges and Strategies of Manganese Dioxide Cathodes for Aqueous Zinc-Ion Batteries, Energy Environ. Mater., 2023, 6, e12575 CrossRef CAS.
- X. Chen, W. Li, D. Reed, X. Li and X. Liu, On Energy Storage Chemistry of Aqueous Zn-Ion Batteries: From Cathode to Anode, Electrochem. Energy Rev, 2023, 6, 33 CrossRef CAS.
- R. Chen, C. Zhang, J. Li, Z. Du, F. Guo, W. Zhang, Y. Dai, W. Zong, X. Gao and J. Zhu, Hydrated Deep Eutectic Electrolyte with Finely-Tuned Solvation Chemistry for High-Performance Zinc-Ion Batteries, Energy Environ. Sci., 2023, 16, 2540–2549 RSC.
- Y. Dai, R. Lu, C. Zhang, J. Li, Y. Yuan, Y. Mao, C. Ye, Z. Cai, J. Zhu, J. Li, R. Yu, L. Cui, S. Zhao, Q. An, G. He, G. I. N. Waterhouse, P. R. Shearing, Y. Ren, J. Lu, K. Amine, Z. Wang and L. Mai, Zn2+-mediated catalysis for fast-charging aqueous Zn-ion batteries, Nat. Catal., 2024, 7, 776–784 CrossRef CAS.
- C. Li, S. Jin, L. A. Archer and L. F. Nazar, Toward practical aqueous zinc-ion batteries for electrochemical energy storage, Joule, 2022, 6, 1733–1738 CrossRef.
- D. Chao, W. Zhou, F. Xie, C. Ye, H. Li, M. Jaroniec and S.-Z. Qiao, Roadmap for advanced aqueous batteries: From design of materials to applications, Sci. Adv., 2020, 6, eaba4098 CrossRef CAS.
- X. Gao, Y. Dai, C. Zhang, Y. Zhang, W. Zong, W. Zhang, R. Chen, J. Zhu, X. Hu and M. Wang, When It's Heavier: Interfacial and Solvation Chemistry of Isotopes in Aqueous Electrolytes for Zn–ion Batteries, Angew. Chem., Int. Ed., 2023, 62, e202300608 CrossRef CAS.
- L. Wang, S. Yan, C. D. Quilty, J. Kuang, M. R. Dunkin, S. N. Ehrlich, L. Ma, K. J. Takeuchi, E. S. Takeuchi and A. C. Marschilok, Achieving stable molybdenum oxide cathodes for aqueous zinc-ion batteries in water-in-salt electrolyte, Adv. Mater. Interfaces, 2021, 8, 2002080 CrossRef CAS.
- W. Zhang, Y. Dai, R. Chen, Z. Xu, J. Li, W. Zong, H. Li, Z. Li, Z. Zhang and J. Zhu, Highly Reversible Zinc Metal Anode in a Dilute Aqueous Electrolyte Enabled by a pH Buffer Additive, Angew. Chem., Int. Ed., 2023, 62, e202212695 CrossRef CAS PubMed.
- Z. Liu, R. Wang, Q. Ma, J. Wan, S. Zhang, L. Zhang, H. Li, Q. Luo, J. Wu and T. Zhou, A dual-functional organic electrolyte additive with regulating suitable overpotential for building highly reversible aqueous zinc ion batteries, Adv. Funct. Mater., 2024, 34, 2214538 CrossRef CAS.
- K. Wu, J. Huang, J. Yi, X. Liu, Y. Liu, Y. Wang, J. Zhang and Y. Xia, Recent advances in polymer electrolytes for zinc ion batteries: mechanisms, properties, and perspectives, Adv. Energy Mater., 2020, 10, 1903977 CrossRef CAS.
- Y. Lv, Y. Xiao, L. Ma, C. Zhi and S. Chen, Recent advances in electrolytes for “beyond aqueous” zinc-ion batteries, Adv. Mater., 2022, 34, 2106409 CAS.
- B. Dunn, H. Kamath and J.-M. Tarascon, Electrical energy storage for the grid: A battery of choices, Science, 2011, 334, 928–935 CrossRef CAS PubMed.
- S. Chu and A. Majumdar, Opportunities and challenges for a sustainable energy future, Nature, 2012, 488(7411), 294–303 CrossRef CAS PubMed.
- M. M. Rahman, A. O. Oni, E. Gemechu and A. Kumar, Assessment of energy storage technologies: A review, Energy Convers. Manage., 2020, 223, 113295 CrossRef CAS.
- A. Berrada, Financial and economic modeling of large-scale gravity energy storage system, Renew. Energy, 2022, 192, 405–419 CrossRef.
- B. Li and J. Liu, Progress and directions in low-cost redox-flow batteries for large-scale energy storage, Natl. Sci. Rev., 2017, 4, 91–105 CrossRef CAS.
- J. Cho, S. Jeong and Y. Kim, Commercial and research battery technologies for electrical energy storage applications, Prog. Energy Combust. Sci., 2015, 48, 84–101 CrossRef.
- Z. Zhu, T. Jiang, M. Ali, Y. Meng, Y. Jin, Y. Cui and W. Chen, Rechargeable batteries for grid scale energy storage, Chem. Rev., 2022, 122, 16610–16751 CrossRef CAS.
- T. Zhang, Y. Tang, S. Guo, X. Cao, A. Pan, G. Fang, J. Zhou and S. Liang, Fundamentals and perspectives in developing zinc-ion battery electrolytes: a comprehensive review, Energy Environ. Sci., 2020, 13, 4625–4665 RSC.
- Y. Wang, Z. Wang, F. Yang, S. Liu, S. Zhang, J. Mao and Z. Guo, Electrolyte engineering enables high performance zinc-ion batteries, Small, 2022, 18, 2107033 CrossRef CAS.
- S. Guo, L. Qin, T. Zhang, M. Zhou, J. Zhou, G. Fang and S. Liang, Fundamentals and perspectives of electrolyte additives for aqueous zinc-ion batteries, Energy Storage Mater., 2021, 34, 545–562 CrossRef.
- Z. Khan, D. Kumar and X. Crispin, Does Water-in-Salt Electrolyte Subdue Issues of Zn Batteries?, Adv. Mater., 2023, 35, 2300369 CrossRef CAS.
- P. Rotella Junior, L. C. S. Rocha, S. N. Morioka, I. Bolis, G. Chicco, A. Mazza and K. Janda, Economic analysis of the investments in battery energy storage systems: Review and current perspectives, Energies, 2021, 14, 2503 CrossRef.
- B. D. Olaszi and J. Ladanyi, Comparison of different discharge strategies of grid-connected residential PV systems with energy storage in perspective of optimal battery energy storage system sizing, Renewable Sustainable Energy Rev., 2017, 75, 710–718 CrossRef.
|
This journal is © The Royal Society of Chemistry 2025 |
Click here to see how this site uses Cookies. View our privacy policy here.