High-intensity ultrasound processing to improve the quality of semitendinosus steak from Bos indicus cattle†
Received
17th October 2024
, Accepted 28th December 2024
First published on 15th January 2025
Abstract
High-intensity ultrasound (HIUS) has been extensively explored as an emerging technology to promote meat tenderization. However, appropriate processing conditions for maximum quality preservation have not been elucidated. Therefore, this study aimed to model the sonication of semitendinosus of Bos indicus by varying time and power. A central composite rotatable design was applied to evaluate the individual and combined effects of time (8–22 min) and power (150–675 W) on the physicochemical parameters of semitendinosus subjected to sonication. Drip loss (DL), metmyoglobin-reducing activity (MRA), lipid oxidation (MDA), hardness, and toughness were analyzed as the physicochemical variables of interest. DL increased (0.25–2.18%) as time and power increased. MRA and MDA were affected by linear and quadratic time. Hardness showed stability during storage at low powers. We hypothesized that ranges between 10 and 16 min and 150 and 262.5 W represent suitable values for texture maintenance and minimize discoloration and oxidative stress during storage. Our findings are promising for the industrial utilization of HIUS to obtain high-quality products.
Sustainability spotlight
Nowadays, the demand for environment-friendly technologies has attracted the attention of consumers and industries, aiming to reduce water and energy consumption during food processing. High-intensity ultrasound (HIUS) is an emerging non-thermal technology widely explored for its green nature and ability to lower energy and water requirements of food processing. However, the application of HIUS in meat and meat products is accountable for a series of undesirable physicochemical changes, including water losses, discoloration, and meat hardening, depending on the process conditions. The use of HIUS in specific ranges of time and power, as suggested by our study, could ensure low water losses and decrease discoloration and oxidative stress while improving texture attributes. At the same time, the mild processing conditions proposed are promising to lower the energy consumption of the food industry, collaborating for the development of sustainable food systems. This work is aligned with Sustainable Development Goals (SDGs) 7 (affordable and clean energy) and 9 (industry, innovation and infrastructure).
|
1 Introduction
High-intensity ultrasound (HIUS, 20–100 kHz, 10–1000 W cm−2) is an emerging non-thermal technology widely explored for its green nature and ability to lower electric energy and water requirements of food processing.1 Moreover, the ability of HIUS to promote tenderization in different food systems has been drawing attention.2 Ultrasonic waves are responsible for the cavitation mechanism, in which microbubble implosion generates mechanical (micro-jet releasing), thermal (hot spots), and chemical (free radical formation) effects on the target.3 Although HIUS is a promising technology, its application in meat is accountable for a series of undesirable physicochemical changes such as water losses,4 discoloration,5 oxidative stress,6 and meat hardening,7 depending on the treatment conditions.
Meat is a complex matrix presenting variable contents of water, protein, and lipids, which vary according to the cattle breed and fiber composition.8Bos indicus, which represents around three-quarters of Brazilian cattle, exhibits a lower muscular fat content than does Bos taurus, requiring muscle-specific strategies to improve the texture parameters of meat.8 Furthermore, although semitendinosus is considered a color-stable muscle owing to the predominance of type IIB fibers, its surface discoloration during display, resulting from metmyoglobin (MetMb) accumulation, could lead to consumer rejection and economic losses.8 Nevertheless, meat possesses a MetMb-reducing enzymatic system that limits myoglobin oxidation, which could be hypothetically enhanced by HIUS cavitation since it may improve mass transfer.9 However, as related in the literature, sonication could increase lipid oxidation rates due to the generation of free hydroxyl radicals.2 This phenomenon refers directly to color alterations and meat toughening by forming protein cross-links.10 In this context, sonication time and power are critical factors due to their intrinsic relationship with the HIUS intensity.11
Recent studies have evaluated the effects of HIUS on the physicochemical parameters of meat.6,12 However, Fallavena et al.6 did not evaluate the effects of sonication time and power. Moreover, Chang et al.12 did not apply any modeling approach to deeply understand the behavior of such variables. Therefore, no model has been developed for the impact of sonication time (min) and power (W). The hypothesis evaluated by this study is the possibility of establishing a range of time and power for the sonication process of meat, in which the preservation and improvement of texture and color occur with minimal oxidative stress. Therefore, this study aimed to model the sonication of semitendinosus steak from Bos indicus as a function of drip loss, metmyoglobin-reducing activity, lipid oxidation, hardness, and toughness to improve the quality of fresh steak. This is the first report in the literature about modeling the time and power of HIUS on the physicochemical properties of bovine meat by using a central composite rotatable design (CCRD) approach, aiming to obtain mathematical models and response surfaces describing their effects on key meat quality attributes.
2 Materials and methods
2.1 Experimental design
2.1.1 Central composite rotatable design.
A CCRD was used in a 3-factor experiment (Table 1), composed of four factorial points, four axial points, and two replicates in the central point. Ten treatments (Table 1) were executed randomly to model the effects of HIUS’ time and power in the physicochemical parameters of semitendinosus steak. After sonication, the samples were refrigerated for seven days, as the physicochemical changes were evident during storage. The time and power values were adopted considering the operational limits of the equipment and the limitations proposed by Bernardo et al.3
Table 1 Ultrasonic treatment factors (independent variables) and levels coded and not coded, according to the central composite rotatable designa
Treatment |
Time (min) |
Amplitude (power input) |
C: central point, [ ]: represent the coded variable.
|
1 |
10 [−1] |
30% (225 W) [−1] |
2 |
10 [−1] |
80% (600 W) [+1] |
3 |
20 [+1] |
30% (225 W) [−1] |
4 |
20 [+1] |
80% (600 W) [+1] |
5 |
8 [−1.41] |
55% (412.5 W) [0] |
6 |
22 [+1.41] |
55% (412.5 W) [0] |
7 |
15 [0] |
20% (150 W) [−1.41] |
8 |
15 [0] |
90% (675 W) [+1.41] |
9 (C) |
15 [0] |
55% (412.5 W) [0] |
10 (C) |
15 [0] |
55% (412.5 W) [0] |
2.1.2 Sample acquisition and preparation.
The semitendinosus muscles from Bos indicus cattle were bought from a local market (Rio de Janeiro, Brazil) 1 day after the animals' slaughter. The muscles were immediately transported to the laboratory at Universidade Federal do Rio de Janeiro (Rio de Janeiro, Brazil) in the isothermal recipient (0 ± 1 °C). External fat was excised, and the samples were sliced (12.1 ± 0.9 cm height, 7.8 ± 0.7 cm length, 1.0 ± 0.1 cm thickness, and 70.5 ± 2.5 g weight).
The steaks were individually packed in nylon-polyethylene bags (15 cm width, 22 cm height, 80 μm thickness) with barrier properties of 66.31 cc per m2 per days for O2 transmission rate (OTR) and 4.91 gm per m2 per day for water-vapor transmission rate (WVTR), according to the information from the manufacturer (Gabrilina, São Paulo, Brazil). Packages were then vacuum-sealed, and ultrasonic treatments were performed, as determined by the CCRD.
2.1.3 Ultrasonic processing.
The packed steaks were immersed in an acrylic holder containing 1 L of distilled water for each treatment. A VC-750 Ultrasonic Processor at 20 kHz, with a maximum input power of 750 W (Sonics & Materials Inc., Newtown, USA), was employed in this study. The effective HIUS power dissipated into the liquid was measured using eqn (1) by the calorimetric method.11 To obtain these data, the initial and final temperatures of the sonicated medium for each power were measured. The ultrasound intensity (UI) was obtained using eqn (2), as depicted in Table S1.† The treatment time (min) and amplitude (%) were adjusted in the equipment interface settings. During the HIUS application, the temperature was continuously measured using a digital thermometer maintained at 22 ± 1 °C by continuous water circulation using chiller equipment (Sonics & Materials Inc). The temperature (22 ± 1 °C) was chosen according to Fallavena, Marczak, & Mercali.6 The authors demonstrated that sonication temperatures between 16 and 28 °C improve the meat tenderness. After treatments, the samples were stored in a refrigerator (4 ± 1 °C) for seven days till the physicochemical analysis to better visualize and measure oxidative processes.12 For each treatment, three steaks were sonicated, and each of the steaks was analyzed in triplicate. | 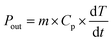 | (1) |
|  | (2) |
where Pout is the power output (W), m is the mass of water (g), Cp is the water-specific heat capacity (4.184 J g−1 °C), dT is the temperature increase (°C), dt is the sonication time (s), UI is the ultrasound intensity (W cm−2), and r is the probe radius (cm).
2.2 Physicochemical analyses
2.2.1 Drip loss on storage.
Steak beef samples were weight-recorded after storage (initial weight). The bags were opened, exudate released from the meat was discarded, and packed steaks were weighed again (final weight). Drip loss (DL) was calculated as expressed in eqn (3): |  | (3) |
2.2.2 Metmyoglobin-reducing activity (MRA).
Metmyoglobin-reducing activity (MRA) was determined according to the method developed by Sammel et al.13 Three blocks (2.5 × 2.0 × 1.0 cm3) were sliced from each steak beef and separately submerged in a sodium nitrite solution (0.3%) for 20 min to stimulate metmyoglobin formation. The blocks were prepared with their initially light-exposed side facing up to guarantee contact with the nitrite solution. Blocks were then paper-dried and vacuum-packed, and the reflectance values were measured using a portable spectrophotometer CM-600D (Konica Minolta Sensing Inc., Osaka, Japan). After incubation at 30 °C for two hours, allowing for metmyoglobin reduction, the reflectance values of each previously evaluated surface were re-recorded. Metmyoglobin formation on the surface was determined using the K/S ratios and formulas used by Canto et al.,8 and the MRA was calculated using eqn (4): |  | (4) |
2.2.3 Lipid oxidation.
Lipid oxidation was evaluated in triplicate by the thiobarbituric acid reactive substance (TBARS) method, according to Yin et al.,14 with slight modifications. The samples (5 g) were homogenized with 22.5 mL of 11% trichloroacetic acid (TCA) solution and centrifuged at 15
000×g for 15 min at 4 °C. The supernatant (1 mL) was mixed with 1 mL of the aqueous solution of 20 mM thiobarbituric acid (TBA) and incubated at 25 °C for 20 h without light. The absorbance values (532 nm) were measured using a UV-1900 spectrophotometer (Shimadzu Corporation, Kyoto, Japan). The results are expressed in mg malonaldehyde (MDA) per kg of sample. The concentration of malonaldehyde (MDA) was obtained from a standard curve developed using 1,1,3,3-tetramethoxypropane (CAS number 102-52-3).
2.2.4 Hardness.
Three blocks from each raw steak beef sample (2.5 × 2.0 × 1.0 cm3) were analyzed by texture profile analysis (TPA) at room temperature (20 °C) using a TA.XTplus Texture Analyser (Stable Micro Systems, Godalming, UK) equipped with a 50 kg load cell, using the following specifications: cylindrical P/36R probe, 5 g trigger force, 40% compression rate, 2 s holding time, and 5, 1, and 5 mm s−1 of pre-test, test, and post-test speed, respectively. The hardness, as defined by Bernardo et al.,15 was then determined from the resulting time–force curve plotted using the Texture Exponent 6.1.9.1© software (Stable Micro Systems, Surrey, UK).
2.2.5 Shear force.
The shear force was determined using the same apparatus and software as in TPA, as determined by AMSA.16 After CL measurement, cooked steak beef samples were chilled at room temperature (20 °C). Cores of 1.27 cm in diameter were obtained, followed by cut using a “V” form Warner–Bratzler knife, with a triangular aperture of 60° and a thickness of 1.17 mm, at a 200 mm min−1 crosshead speed. The maximum peak force (kg) required to shear each sample was recorded using three replicates to obtain toughness values.
2.3 Statistical analysis
The principal component analysis (PCA) was applied to understand the association of the physicochemical response variables (DL, MRA, MDA, hardness, and toughness), as well as the two independent factors (time and power). The components were built using a correlation matrix.
Second-order polynomial equations (eqn (5)), according to Baş & Boyacı,17 were used to describe the effects of time and power on the variables of interest, maintaining the significant terms (p < 0.05). The graphical representation of the models was assessed by applying response surface methodology (RSM). Mean square error (MSE), lack-of-fit (LOF), and the adjusted coefficient of determination (Radj2) were calculated to evaluate the goodness-of-fit of the model. The Shapiro–Wilk test was used to verify the normality of the residual data (p < 0.05).
| Variable response = B0 + B1X1 + B2X2 + B11X12 + B22X22 + B12X1X2 + ε | (5) |
where
X is the independent factor (time and power),
B is the regression coefficient, and
ε is the experimental error.
Five extra experiments using random conditions were performed to validate the models (Table S2†). The validation was assessed through the accuracy (Af) and the bias (Bf) factors (eqn (6) and (7)), measured as described by Baranyi, Pin, & Ross.18 In this context, Af represents the data spread around the predicted values, and Bf is the level of agreement between the predicted and observed responses.18
| 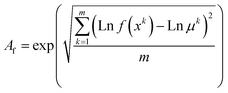 | (6) |
| 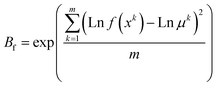 | (7) |
where
f(
x) is the value predicted by the model,
μ is the observed value, and
m is the number of additional experiments. The Statistica 10
© software package was used for all the statistical analyses.
3 Results and discussion
3.1 Principal component analysis
After collecting the responses of the dependent variables for each one of the treatments, as summarized in Table 2, PCA was performed to understand the correlation between the dependent and independent factors. As could be observed, the principal components (PC) in which time and power are better explained were PC1 (42.87%) and PC2 (33.12%). Fig. 1 describes the behavior of each variable by plotting PC1 by PC2. As expected, time and power show no correlation since they were independently designed before the analysis, meeting at 90° in the graph. MRA and MDA showed a contrary behavior, as MDA seems to increase parallel with sonication time, suggesting that MRA decreases as MDA increases. Furthermore, DL and hardness seem to be strongly influenced by the sonication power, indicating that these variables' values increase power dependently. Finally, toughness is strongly affected by time and power (Table S3†). After PCA, the behavior of the variables was explored and confirmed by the mathematical modeling and response surface.
Table 2 Variable responses (mean ± standard deviation of three independent replicates) for semitendinosus steak from Bos indicus after ultra-sound treatments and storage (seven days) at 4 ± 1 °Ca
Variables |
Cb |
1 |
2 |
3 |
4 |
5 |
6 |
7 |
8 |
9 |
10 |
C: control, DL: drip loss, MDA: malondialdehyde, MRA: metmyoglobin reducing activity, N: Newton.
Control refers to non-sonicated samples stored for seven days at 4 ± 1 °C.
|
DL (%) |
0.25 ± 0.03 |
1.25 ± 0.04 |
0.52 ± 0.02 |
0.35 ± 0.01 |
1.63 ± 0.05 |
1.11 ± 0.03 |
0.92 ± 0.03 |
0.12 ± 0.03 |
2.02 ± 0.06 |
2.09 ± 0.06 |
2.18 ± 0.06 |
MRA (%) |
23.96 ± 0.6 |
27.75 ± 0.64 |
30.28 ± 0.7 |
22.18 ± 0.51 |
22.48 ± 0.52 |
20.32 ± 0.47 |
19.06 ± 0.44 |
27.54 ± 0.64 |
30.41 ± 0.7 |
30.67 ± 0.7 |
29.68 ± 0.7 |
MDA (mg kg−1) |
0.0151 ± 0.000 |
0.0165 ± 0.000 |
0.0186 ± 0.000 |
0.0204 ± 0.000 |
0.0216 ± 0.000 |
0.0179 ± 0.000 |
0.0234 ± 0.000 |
0.0175 ± 0.000 |
0.0178 ± 0.000 |
0.0175 ± 0.000 |
0.0171 ± 0.000 |
Hardness (N) |
13.39 ± 0.42 |
6.18 ± 0.24 |
10.36 ± 0.41 |
11.22 ± 0.45 |
13.37 ± 0.53 |
8.01 ± 0.32 |
10.08 ± 0.4 |
7.99 ± 0.32 |
15.39 ± 0.61 |
11.17 ± 0.46 |
11.82 ± 0.46 |
Toughness (N mm−1 s−1) |
14.7 ± 0.49 |
15.7 ± 0.35 |
24.8 ± 0.55 |
24.2 ± 0.54 |
23.9 ± 0.53 |
24.4 ± 0.54 |
26.2 ± 0.58 |
16.8 ± 0.37 |
21.6 ± 0.48 |
22 ± 0.5 |
22.7 ± 0.5 |
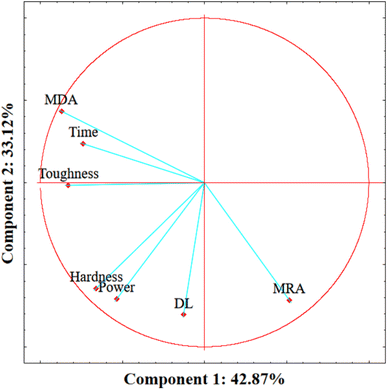 |
| Fig. 1 Principal component analysis of the physicochemical variables. DL: drip loss, MDA: malondialdehyde, and MRA: metmyoglobin reducing activity. | |
3.2 Models' performance and validation
The performance indices utilized to evaluate the models are displayed in Table 3. The Radj2 values were 0.78, 0.80, 0.91, 0.85, and 0.92 for DL, MRA, MDA, hardness, and toughness, respectively, characterizing a satisfactory explanation for the data variability since results close to 1.00 designate better-fitted models. The LOF was not significant (p > 0.1), demonstrating a good fit of the obtained models to the experimental data since this term was calculated by applying the error variance independently of the model predictions. Furthermore, the models presented low MSE, which characterizes the variability not accounted for by the model's LOF, including the experiment's natural variability and systematic errors.19 The Shapiro–Wilk test, summarized in Table 3, demonstrated that residual data were normally distributed (p > 0.05). The effects of time and power on the response variables (DL, MRA, MDA, hardness, and toughness) are described in eqn (8)–(12), respectively. The five equations were modeled by multiple regression analysis, maintaining only the significant terms. | Drip loss (%) = 1.0548 − (0.00976t2) + (0.00148P) − (0.000011P2) + (0.00069tP) | (8) |
| MRA(%) = −7.5882 + (5.3732t) − (0.1917t2) | (9) |
| MDA(mg kg−1) = 0.0266 − (0.00157t) + (0.0000647t2) | (10) |
| Hardness (N) = −8.6616 + (1.8093t) − (0.05117t2) + (0.0112P) | (11) |
| Toughness (N mm s−1) = 3.986 − (0.4431t) + (0.0577t2) + (0.0855P) − (0.000045P2) − (0.0025tP) | (12) |
where t is the time (min) of sonication and P is the power (W).
Table 3 Effect estimates, significance, residual data, and performance of the variables of interesta
Model |
DL |
MRA |
MDA |
Hardness |
Toughness |
EE |
SE |
p-Value |
EE |
SE |
p-Value |
EE |
SE |
p-Value |
EE |
SE |
p-Value |
EE |
SE |
p-Value |
A
f: accuracy factor, Bf: bias factor, DL: drip loss, EE: effect estimates, LOF: lack-of-fit, MDA: malondialdehyde, MRA: metmyoglobin reducing activity, MSE: mean square error, NS: non-significant (p > 0.05), Radj2: adjusted coefficient of determination, SE: standard error (pure).
|
Intercept |
2.14 |
0.044 |
— |
29.871 |
0.324 |
— |
0.017 |
>0.001 |
— |
11.585 |
0.213 |
— |
22.355 |
0.345 |
— |
Time |
NS |
— |
— |
−3.785 |
0.495 |
0.083 |
0.004 |
>0.001 |
0.034 |
2.742 |
0.326 |
0.075 |
2.531 |
0.345 |
0.086 |
Time2 |
−1.177 |
0.058 |
0.032 |
−9.586 |
0.592 |
0.039 |
0.003 |
>0.001 |
0.047 |
−2.558 |
0.39 |
0.096 |
2.887 |
0.456 |
0.099 |
Power |
0.811 |
0.044 |
0.035 |
NS |
— |
— |
NS |
— |
— |
4.2 |
0.326 |
0.049 |
3.906 |
0.345 |
0.056 |
Power2 |
−1.12 |
0.058 |
0.033 |
NS |
— |
— |
NS |
— |
— |
NS |
— |
— |
−3.192 |
0.456 |
0.09 |
Time × power |
1.00 |
0.063 |
0.04 |
NS |
— |
— |
NS |
— |
— |
NS |
— |
— |
−4.71 |
0.488 |
0.066 |
Normality |
Normal (0.108) |
Normal (0.871) |
Normal (0.627) |
Normal (0.075) |
Normal (0.484) |
R
adj
2
|
0.78 |
0.80 |
0.90 |
0.85 |
0.92 |
MSE |
0.004 |
0.491 |
>0.001 |
0.213 |
0.238 |
LOF |
0.118 |
0.243 |
0.28 |
0.298 |
0.311 |
A
f
|
1.106 |
1.039 |
1.023 |
1.061 |
1.046 |
B
f
|
1.031 |
1.013 |
1.004 |
1.031 |
0.984 |
The accuracy factor (Af) and bias factor (Bf) were measured through the five random additional treatments (Table 3). Mostly, Af ranges from 1.03 to 1.45, while Bf ranges from 1.00 to 1.32.20Af found in our study (1.023–1.106) suggests that predicted and observed values are in good concurrence since the closer to the perfect fit (1.00), the less information is spread around the fitted values.21 Moreover, the Bf values lower than 0.7 or higher than 1.15 are unsuitable.22 Therefore, Bf in our study (0.984–1.031) represents suitable results. Furthermore, Bf between 0.90 and 1.05 is considered good since this index indicates the degree of over- or under-prediction depicted by the model.23 Therefore, considering the performance evaluation indices achieved, i.e., Radj2 and LOF, the proposed models showed satisfactory performance. The validation regarding Af and Bf showed that the models could be reliably applied for different conditions (outside the model).
3.3 Drip loss
Drip loss (DL) could be defined as an aqueous protein solution that emerges above the muscle surface after hours or days.4 In our study, time and power presented significant quadratic negative effects on this variable. Nonetheless, the positive interaction between these factors, combined with the linear positive impact of the power, allows us to state that the gradual increase of time and power results in high values for DL. In this context, a combination of 16–20 min and 150–300 W could be suitable for obtaining low DL responses (Fig. 2A).
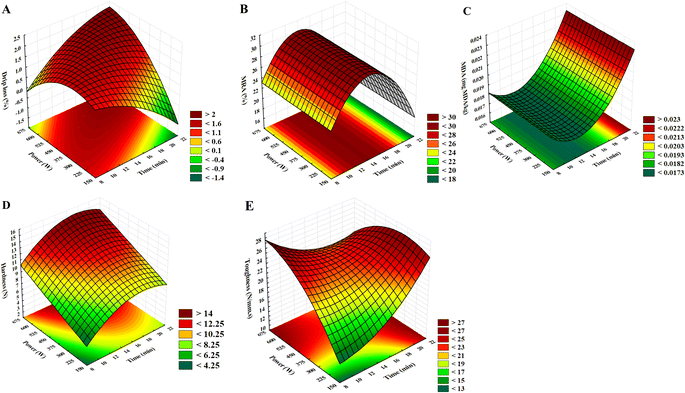 |
| Fig. 2 Response surface graphs of HIUS (time and power) effects on (A) drip loss, (B) metmyoglobin reducing activity (MRA), (C) malondialdehyde (MDA), (D) hardness, and (E) toughness of semitendinosus steak stored for one week at 4 ± 1 °C. | |
Our results are supported by Jayasooriya et al.,24 which evaluated HIUS application (24 kHz, 12 W cm−2) in vacuum-packaged meat from semitendinosus muscles at different sonication times (up to 240 s). Our study verified that linear sonication time increases led to a slight reduction in DL values. These findings could be justified due to the constant temperature control established in the experiments, limiting heating and thermal denaturation of proteins, preventing the formation of gaps between muscle fibers, and reducing the water-holding capacity (WHC) since DL and WHC present an inverse relationship.25
Furthermore, Carrillo-Lopez et al.26 determined that WHC in sonicated rabbit meat (24 kHz, 20 min, 9.6 W cm−2) tends to increase within 20 minutes of treatment and decrease later (40 min), corroborating our findings. As described by the authors, short ultrasonic periods seem responsible for increasing the water–protein interaction, resulting in a reduction in the size of myofibrillar proteins and an increase in the contact surface, which would reduce DL. Furthermore, Chang et al.27 found an increase in water loss when the sonication (40 kHz, 1500 W) time increased from 10 to 20 min due to microstructural changes in the meat. As Bernardo et al.3 pointed out, meat sonication can induce muscular cell rupture, increasing the extracellular space and weakening the connective tissue around the fibers, which explains improved water loss.
This difference can also be observed in our study and may be related to the power and the interaction between these factors. At low powers (<300 W), the increase in sonication time is responsible for the reduction of DL, while at high powers (>300 W), the opposite occurs. This behavior establishes that the migratory movements of water in the sonicated meat depend on time and power. Moreover, considering low power, short treatments (15–20 min) are preferable.
Contreras-Lopez et al.28 assessed the WHC of ultrasound-treated cured pork (37 kHz, 22 W cm−2). The WHC of the samples did not vary compared to the control and sonicated samples. However, the authors stated that the WHC of treated samples differed between the thick and thin steaks, whereas the thicker ones showed higher WHC. Thus, the beef thickness seems to be a critical factor concerning the DL of sonicated meat. In this study, the thickness of the samples was standardized (1.0 ± 0.1 cm), so that this factor did not represent a bias in our results. Moreover, as reported by Siró et al.,25 water loss in pork is not affected by ultrasonic treatment (20 kHz) at different times (10–180 min) and intensities (2–4 W cm−2). However, the power input by these authors ranged from 40 to 80 W, being inferior to those used herein.
In our study, most of the HIUS treatments resulted in higher DL than control. These findings are supported by Valenzuela et al.,4 who evaluated the DL of sonicated (40 kHz, 11 W cm−2) semitendinosus muscle and verified that treated samples exhibit higher DL than control samples through storage. Therefore, it is possible to state that DL is affected by time, power, and the interaction between these factors, increasing water losses. In this way, DL can be considered a limiting factor in establishing the desirable conditions for meat sonication.
3.4 MRA
Metmyoglobin (MetMb), an oxidative ferric form of myoglobin (Mb-Fe3+), is an undesirable brownish compound responsible for meat surface discoloration.29 Therefore, the activity of natural MetMb-reducing systems is one of the most crucial mechanisms for preserving the color of fresh meat. Despite this fact, only two studies have evaluated the effect of HIUS on the MRA of meat to date.5,30 In our research, the MRA values (Table 3) were linear and quadratically affected by the sonication time, and long treatments (>18 min) gradually reduced MRA, independently of power (Fig. 2B). Furthermore, due to the negative influence of the quadratic term, MRA presented low levels in short treatments (<10 min). Our findings agree with Stadnik & Dolatowski,5 who stated that MetMb (%) values vary between control and sonicated beef, which may be higher or lower depending on storage time. In our study, 60% of the treatments presented higher MRA than the control after seven days of refrigeration, demonstrating an exciting finding for minimizing meat discoloration. Moreover, De Lima et al.30 stated that applying different acoustic densities (276.36–747.79 W cm−2) have no significant effect on the MRA of meat through storage (7 days), supporting our results.
One possible explanation for this phenomenon is the transient cavitation mechanism (>1 W cm−2) originated by HIUS. The physical damage caused by the microbubble implosion and formation of micro-jets could justify the translocation of nicotinamide adenine dinucleotide (NADH) from the inner meat portion to the outer ones. This occurs due to an improved mass transfer generated by cavitation that increases the yield of enzymatic reactions.9 The NADH concentration is naturally higher in the inner portion of the muscle.13 Its translocation to the external portion should favor the NADH-cytochrome b5 reductase system, in which NADH serves as an electron donor, to reduce MetMb to Mb.31 Our findings suggest that, regarding MRA, sonication times between 12 and 16 min are indicated, promoting an elevated escape of NADH from the inner meat portion and increasing beef color preservation during storage under refrigeration. Furthermore, when applying long treatments (>16 min), the reduction in MRA could be explained due to its interaction with lipid oxidation products. As reported by the literature, there is an association between lipid and Mb oxidative processes, where lipid oxidation products such as hydroxynonenal (HNE) and MDA bind directly to the myoglobin molecule, exposing it to an oxidizing medium and inducing oxidation.32 Moreover, HNE could reduce NADH formation due to covalent binding to lactate dehydrogenase, decreasing Mb reduction.32 These results may be reinforced by our mathematical models concerning MRA and MDA and by the strongest negative association found between these two dependent variables. Therefore, MRA seems to be strictly associated with treatment time and lipid oxidation.
3.5 Lipid oxidation
The results obtained for MDA in this study were significantly affected by the sonication time, linearly and quadratically, like MRA. However, contrary to MRA, the quadratic factor was positive. Thus, MDA levels tend to gradually increase when applying elevated times (>14 min), with a range of 10–14 min suggested for meat sonication (Fig. 2C). As previously stated, this behavior could justify the reduction of MRA in treatments above 16 min. Lipid and hemeprotein (e.g., hemoglobin and myoglobin) oxidative processes seem to occur concomitantly, so that each one enhances the other. Moreover, they are both correlated with the Fenton reaction mechanism, as elegantly reviewed by Domínguez et al.33 The oxidation of Mb to its ferric form (MetMb) could yield alkyl and hydroxyl radicals, which initiate lipid oxidation. Furthermore, Mb oxidation processes release free iron, catalyzing lipid oxidation. In the same way, lipid oxidation products, such as aldehydes, could increase the susceptibility of Mb to oxidation and change heme-protein's redox stability, leading to a decrease in MRA and an increase in prooxidant activity.33 In addition, both processes were possibly enhanced by the generation of free radicals by sonication, which is intensity-related.6
Moreover, the results for MDA achieved in our study are supported by recent literature on sonicated meat and meat products. Cichoski et al.34 reported that sonicated mortadella (25 kHz, 462 W) presented reduced oxidative stability compared to control. This behavior was attributed to breaking fat globules into tiny particles, enhancing the interaction between fatty acids and free radicals. Moreover, Zhang et al.35 described high lipid oxidation levels when increasing HIUS treatment times (25 kHz, 240 W, 15–35 min) on Frankfurt-type sausages. Moreover, the storage period contributes to an elevated oxidative stress environment.35 However, the MDA values in the sonicated meat samples did not exceed 0.5 mg kg−1 during storage, which is promising since the flavor originating from results below this limit is not detected by a sensory panel.36 Thus, as well as DL, MDA represents an undesirable limiting parameter to establish the optimal conditions for meat sonication.
3.6 Hardness and toughness
Hardness was significantly influenced by time (linearly and quadratically) and power (linearly); however, this influence was marginally significant (0.05 < p < 0.1). The hardness property gradually increases as high powers are applied (Fig. 2D). Hardness could be defined as the maximum peak force required to compress the sample at the first cycle in TPA.15 In our study, 9 out of 10 treatments reached hardness values below the control, except the 675 W (90% amplitude) treatment (Table 2). Thus, it can be stated that HIUS is an efficient technology to promote hardness stability, depending on the power. In this context, treatments presenting low time (8–12 min) and powers (150–300 W) were the most satisfactory in this accomplishment (Fig. 2D).
Cavitation is responsible for physical damage in the meat, leading to structural weakening due to the rupture of cellular membranes, improving endogenous proteolysis, and reducing the hardness.3 Moreover, HIUS can improve meat tenderization by fragmentation and denaturation of collagen macromolecules.26 However, at the highest tested power, meat hardening was observed. This tendency was reported previously in the literature. Garcia-Galicia et al.7 reported that treated beef longissimus lumborum exhibited higher hardness than the control when applying the most intense ultrasound treatment (37 kHz, 90 W cm−2, 40 min). A reasonable explanation for this phenomenon is the improved formation of free hydroxyl radicals that occurs during sonication, responsible for increasing the formation of protein cross-links (covalent intermolecular or intramolecular bonds between myofibrillar proteins), contributing to meat hardening.37 Moreover, as stated previously, at elevated times and powers, the DL presented an increase, which can be responsible for preventing meat tenderization.
Different from hardness, the toughness was marginally affected by time and power (0.05 < p < 0.1), linearly, quadratically, and by the interaction between these factors. As depicted in Fig. 2E, the lower values obtained for toughness were achieved at times and powers ranging from 8 to 16 min and 150 to 262.5 W, respectively, supporting the results reached for hardness. However, contrary to hardness, all treatments performed lead to meat toughening. Toughness could be defined as the energy dissipated by a material before it fails; in the case of cooked meat, it can be physically described as the difficulty of separating the sample into two different portions by applying shear forces. Cooked meat toughness directly depends on intramuscular connective tissue (IMCT) contributions.38 It seems that HIUS, due to the generation of free radicals, could enhance the insolubility of collagen in IMCT.
Besides the cavitation mechanism described herein, the Fenton reaction could be correlated with meat toughening. The released hydroxyl free radicals subject meat to an oxidative stress condition, as protein oxidation rates tend to increase in a Fenton reaction system.33 Protein oxidation could result in the formation of cross-links, affecting its structure and spatial arrangement and promoting the toughening effect.10 Thus, the evaluation of protein oxidation and collagen content, as well as the visualization of meat microstructure, is a vital parameter to understand the effects of HIUS on cooked meat in further studies.
Concerning the physicochemical changes and the models developed, our study suggests that meat sonication aiming for tenderization should be performed at low levels of time (12–16 min) and power (150–262.5 W). The application of HIUS at this range ensures low water losses and decreases discoloration and oxidative stress while maintaining or improving texture attributes. At the same time, these mild processing conditions are promising to reduce energy requirements by the food industry, collaborating for the development of sustainable food systems.
4 Conclusions
An optimal sonication range was achieved to tenderize and minimize the discoloration of semitendinosus from Bos indicus cattle during one week under refrigeration, considering the time and power, in which undesirable changes are close to a minimum, ensuring meat quality maintenance. Drip loss, metmyoglobin-reducing activity, lipid oxidation, hardness, and toughness were influenced by HIUS power and time, although not necessarily in an intensity-dependent manner. Oxidative stress seems to be the critical factor in understanding the influence of sonication on meat characteristics due to its intrinsic relationship with other physicochemical parameters such as metmyoglobin reducing activity and raw or cooked texture. Thus, the HIUS technology can be applied to improve the meat quality with minimal undesirable physicochemical changes.
Data availability
The data supporting this article were included in the main document or as part of the ESI.†
Conflicts of interest
The authors declare no competing financial interest.
Acknowledgements
This research was funded by the Fundação de Amparo à Pesquisa do Estado do Rio de Janeiro (FAPERJ) Brazil – grant numbers [E-26/200.891/2021, E-26/202.272/2021, E-26/200.060/2024], the Conselho Nacional de Desenvolvimento Científico e Tecnológico (CNPq) – grant number [313119/2020-1 and 152241/2022-1], and the Coordenação de Aperfeiçoamento de Pessoal de Nível Superior (CAPES) Brazil – Finance Code 001.
References
- L. P. Marques, Y. A. A. Bernardo and C. A. Conte-Junior, Applications of High-intensity Ultrasound on Shrimp: Potential, Constraints, and Prospects in the Extraction and Retrieval of Bioactive Compounds, Safety, and Quality, J. Food Sci., 2024, 1750–3841, DOI:10.1111/1750-3841.17093.
- A. D. Alarcon-Rojo, L. M. Carrillo-Lopez, R. Reyes-Villagrana, M. Huerta-Jiménez and I. A. Garcia-Galicia, Ultrasound and Meat Quality: A Review, Ultrason. Sonochem., 2019, 55, 369–382, DOI:10.1016/j.ultsonch.2018.09.016.
- Y. A. A. Bernardo, D. K. A. Do Rosario and C. A. Conte-Junior, Principles, Application, and Gaps of High-Intensity Ultrasound and High-Pressure Processing to Improve Meat Texture, Foods, 2023, 12(3), 476, DOI:10.3390/foods12030476.
- C. Valenzuela, I. A. Garcia-Galicia, L. Paniwnyk and A. D. Alarcon-Rojo, Physicochemical Characteristics and Shelf Life of Beef Treated with High-intensity Ultrasound, J. Food Process. Preserv., 2021, 45(4), e15350, DOI:10.1111/jfpp.15350.
- J. Stadnik and Z. J. Dolatowski, Influence of Sonication on Warner-Bratzler Shear Force, Colour and Myoglobin of Beef (m. Semimembranosus), Eur. Food Res. Technol., 2011, 233(4), 553–559, DOI:10.1007/s00217-011-1550-5.
- L. P. Fallavena, L. D. Ferreira Marczak and G. D. Mercali, Ultrasound Application for Quality Improvement of Beef Biceps Femoris Physicochemical Characteristics, LWT, 2020, 118, 108817, DOI:10.1016/j.lwt.2019.108817.
- I. A. Garcia-Galicia, V. G. Gonzalez-Vacame, M. Huerta-Jimenez, L. M. Carrillo-Lopez, J. M. Tirado-Gallegos, R. A. Reyes-Villagrana and A. D. Alarcon-Rojo, Ultrasound Versus Traditional Ageing: Physicochemical Properties in Beef Longissimus Lumborum, CyTA–J. Food, 2020, 18(1), 675–682, DOI:10.1080/19476337.2020.1834458.
- A. C. V. C. S. Canto, B. R. C. Costa-Lima, S. P. Suman, M. L. G. Monteiro, F. M. Viana, A. P. A. A. Salim, M. N. Nair, T. J. P. Silva and C. A. Conte-Junior, Color Attributes and Oxidative Stability of Longissimus Lumborum and Psoas Major Muscles from Nellore Bulls, Meat Sci., 2016, 121, 19–26, DOI:10.1016/j.meatsci.2016.05.015.
- J. Su and A. Cavaco-Paulo, Effect of Ultrasound on Protein Functionality, Ultrason. Sonochem., 2021, 76, 105653, DOI:10.1016/j.ultsonch.2021.105653.
- Y. Bao and P. Ertbjerg, Effects of Protein Oxidation on the Texture and Water-Holding of Meat: A Review, Crit. Rev. Food Sci. Nutr., 2019, 59(22), 3564–3578, DOI:10.1080/10408398.2018.1498444.
- D. Kang, Y. Zou, Y. Cheng, L. Xing, G. Zhou and W. Zhang, Effects of Power Ultrasound on Oxidation and Structure of Beef Proteins during Curing Processing, Ultrason. Sonochem., 2016, 33, 47–53, DOI:10.1016/j.ultsonch.2016.04.024.
- H.-J. Chang, X.-L. Xu, G.-H. Zhou, C.-B. Li and M. Huang, Effects of Characteristics Changes of Collagen on Meat Physicochemical Properties of Beef Semitendinosus Muscle during Ultrasonic Processing, Food Bioprocess Technol., 2012, 5(1), 285–297, DOI:10.1007/s11947-009-0269-9.
- L. M. Sammel, M. C. Hunt, D. H. Kropf, K. A. Hachmeister and D. E. Johnson, Comparison of Assays for Metmyoglobin Reducing Ability in Beef Inside and Outside Semimembranosus Muscle, J. Food Sci., 2002, 67(3), 978–984, DOI:10.1111/j.1365-2621.2002.tb09439.x.
- M. C. Yin, C. Faustman, J. W. Riesen and S. N. Williams, α-Tocopherol and Ascorbate Delay Oxymyoglobin and Phospholipid Oxidation In Vitro, J. Food Sci., 1993, 58(6), 1273–1276, DOI:10.1111/j.1365-2621.1993.tb06164.x.
- Y. A. de A. Bernardo, D. K. A. do Rosario, M. L. G. Monteiro, S. B. Mano, I. F. Delgado and C. A. Conte-Junior, Texture Profile Analysis: How Parameter Settings Affect the Instrumental Texture Characteristics of Fish Fillets Stored Under Refrigeration?, Food Anal. Methods, 2022, 15(1), 144–156, DOI:10.1007/s12161-021-02095-0.
-
American Meat Science Association, Research Guidelines for Cookery, Sensory Evaluation, and Instrumental Tenderness Measurements
of Meat, 2016 Search PubMed.
- D. Baş and İ. H. Boyacı, Modeling and Optimization I: Usability of Response Surface Methodology, J. Food Eng., 2007, 78(3), 836–845, DOI:10.1016/j.jfoodeng.2005.11.024.
- J. Baranyi, C. Pin and T. Ross, Validating and Comparing Predictive Models, Int. J. Food Microbiol., 1999, 48(3), 159–166, DOI:10.1016/S0168-1605(99)00035-5.
- M. C. te Giffel and M. H. Zwietering, Validation of Predictive Models Describing the Growth of Listeria Monocytogenes, Int. J. Food Microbiol., 1999, 46(2), 135–149, DOI:10.1016/S0168-1605(98)00189-5.
- D. K. A. Do Rosario, Y. A. A. Bernardo, Y. S. Mutz, B. Tiwari, A. Rajkovic, P. C. Bernardes and C. A. Conte-Junior, Modelling Inactivation of Staphylococcus Spp. on Sliced Brazilian Dry-Cured Loin with Thermosonication and Peracetic Acid Combined Treatment, Int. J. Food Microbiol., 2019, 309, 108328, DOI:10.1016/j.ijfoodmicro.2019.108328.
- L. Chen, Q. Liu, X. Zhao, H. Zhang, X. Pang and H. Yang, Inactivation Efficacies of Lactic Acid and Mild Heat Treatments against Escherichia Coli Strains in Organic Broccoli Sprouts, Food Control, 2022, 133, 108577, DOI:10.1016/j.foodcont.2021.108577.
- X. Zhao, L. Chen, L. Zhao, Y. He and H. Yang, Antimicrobial Kinetics of Nisin and Grape Seed Extract against Inoculated Listeria Monocytogenes on Cooked Shrimps: Survival and Residual Effects, Food Control, 2020, 115, 107278, DOI:10.1016/j.foodcont.2020.107278.
- T. Ross, Predictive Modelling of the Growth and Survival of Listeria in Fishery Products, Int. J. Food Microbiol., 2000, 62(3), 231–245, DOI:10.1016/S0168-1605(00)00340-8.
- S. D. Jayasooriya, B. R. Bhandari, P. Torley and B. R. D'Arcy, Effect of High Power Ultrasound Waves on Properties of Meat: A Review, Int. J. Food Prop., 2004, 7(2), 301–319, DOI:10.1081/JFP-120030039.
- I. Siró, Cs. Vén, Cs. Balla, G. Jónás, I. Zeke and L. Friedrich, Application of an Ultrasonic Assisted Curing Technique for Improving the Diffusion of Sodium Chloride in Porcine Meat, J. Food Eng., 2009, 91(2), 353–362, DOI:10.1016/j.jfoodeng.2008.09.015.
- L. M. Carrillo-Lopez, D. Robledo, V. Martínez, M. Huerta-Jimenez, M. Titulaer, A. D. Alarcon-Rojo, A. Chavez-Martinez, L. Luna-Rodriguez and L. R. Garcia-Flores, Post-Mortem Ultrasound and Freezing of Rabbit Meat: Effects on the Physicochemical Quality and Weight Loss, Ultrason. Sonochem., 2021, 79, 105766, DOI:10.1016/j.ultsonch.2021.105766.
- H.-J. Chang, Q. Wang, C.-H. Tang and G.-H. Zhou, Effects of Ultrasound Treatment on Connective Tissue Collagen and Meat Quality of Beef Semitendinosus Muscle: Ultrasound on Collagen and Meat Quality of Beef, J. Food Qual., 2015, 38(4), 256–267, DOI:10.1111/jfq.12141.
- G. Contreras-Lopez, A. Carnero-Hernandez, M. Huerta-Jimenez, A. D. Alarcon-Rojo, I. Garcia-Galicia and L. M. Carrillo-López, High-intensity Ultrasound Applied on Cured Pork: Sensory and Physicochemical Characteristics, Food Sci. Nutr., 2020, 8(2), 786–795, DOI:10.1002/fsn3.1321.
- A. E. D. Bekhit and C. Faustman, Metmyoglobin Reducing Activity, Meat Sci., 2005, 71(3), 407–439, DOI:10.1016/j.meatsci.2005.04.032.
- L. C. S. De Lima, A. P. A. D. A. Salim, I. Trezze, M. D. S. Ferreira, M. L. G. Monteiro, S. B. Mano and C. A. Conte-Junior, Influence of ultrasound intensities on colour, oxidative stability, and texture of Longissimus lumborum steaks from Nellore cattle, Int. J. Food Sci. & Technol., 2024, 59(3), 1990–2002, DOI:10.1111/ijfs.16376.
- C. L. Van Bibber-Krueger, A. M. Collins, K. J. Phelps, T. G. O'Quinn, T. A. Houser, K. K. Turner and J. M. Gonzalez, Effects of Quality Grade and Intramuscular Location on Beef Semitendinosus Muscle Fiber Characteristics, NADH Content, and Color Stability, J. Anim. Sci., 2020, 98(4), skaa078, DOI:10.1093/jas/skaa078.
- C. Faustman, Q. Sun, R. Mancini and S. P. Suman, Myoglobin and Lipid Oxidation Interactions: Mechanistic Bases and Control, Meat Sci., 2010, 86(1), 86–94, DOI:10.1016/j.meatsci.2010.04.025.
- R. Domínguez, M. Pateiro, M. Gagaoua, F. J. Barba, W. Zhang and J. M. Lorenzo, A Comprehensive Review on Lipid Oxidation in Meat and Meat Products, Antioxidants, 2019, 8(10), 429, DOI:10.3390/antiox8100429.
- A. J. Cichoski, J. S. da Silva, Y. S. V. Leães, S. S. Robalo, B. A. dos Santos, S. R. Reis, P. Nehring, S. P. Santos, R. Wagner and C. R. de Menezes,
et al., Effects of Ultrasonic-Assisted Cooking on the Volatile Compounds, Oxidative Stability, and Sensory Quality of Mortadella, Ultrason. Sonochem., 2021, 72, 105443, DOI:10.1016/j.ultsonch.2020.105443.
- F. Zhang, H. Zhao, C. Cao, B. Kong, X. Xia and Q. Liu, Application of Temperature-Controlled Ultrasound Treatment and Its Potential to Reduce Phosphate Content in Frankfurter-Type Sausages by 50, Ultrason. Sonochem., 2021, 71, 105379, DOI:10.1016/j.ultsonch.2020.105379.
- Y. Lan, Y. Shang, Y. Song and Q. Dong, Changes in the Quality of Superchilled Rabbit Meat Stored at Different Temperatures, Meat Sci., 2016, 117, 173–181, DOI:10.1016/j.meatsci.2016.02.017.
- M. Estévez, Protein Carbonyls in Meat Systems: A Review, Meat Sci., 2011, 89(3), 259–279, DOI:10.1016/j.meatsci.2011.04.025.
- P. P. Purslow, Intramuscular Connective Tissue and Its Role in Meat Quality, Meat Sci., 2005, 70(3), 435–447, DOI:10.1016/j.meatsci.2004.06.028.
|
This journal is © The Royal Society of Chemistry 2025 |
Click here to see how this site uses Cookies. View our privacy policy here.