Nutrient-rich puffed snacks developed using blended flours and lignin Pickering emulsions containing curcumin and vitamin D3
Received
17th December 2024
, Accepted 10th February 2025
First published on 11th February 2025
Abstract
This study explores the use of blended flours and fortification with health-promoting compounds to improve the nutritional profile of extruded puffed snacks (EPS). Lignin particles extracted from Ayurvedic spent materials were used to create lignin Pickering emulsions (LPEs) for incorporating the lipophilic compounds, curcumin and vitamin D3. A blended flour composed of pearl millet, spent coconut, and corn grits was used to replace 80% of the traditionally used corn grits. Fortification was achieved by incorporating LPE containing curcumin and vitamin D3. Protein content, dietary fiber, texture profiles, and structural integrity of the snacks were evaluated. Compared to a control made solely from corn grits, the EPS with blended flour showed higher protein (13.8%) and dietary fiber (19.2%) contents. However, the increase in protein and fiber content resulted in lower expansion ratios for EPS produced with the blended flour, with or without LPE. The EPS containing LPE had similar hardness, microstructure, fracturability, chewiness, and gumminess as those without LPE. Sensory analysis scores confirmed the acceptability of both EPS and EPS containing LPE. Importantly, the inclusion of LPE enhanced the stability of curcumin (69.0%) and vitamin D3 (65.7%), highlighting the protective encapsulation effect of lignin particles. This study underscores the potential of lignin-based Pickering emulsions loaded with lipophilic compounds, combined with blended flours, for producing nutrient-rich and health-promoting ready-to-eat snacks through extrusion.
Sustainability spotlight
The sustainability goals of this study align with SDG 2 (Zero Hunger), SDG 3 (Good Health and Well-being) and SDG 12 (Responsible Consumption and Production). By utilizing nutrient-dense blended flours and lignin particles derived from agricultural and Ayurvedic waste, the study promotes food waste valorization and a circular economy. Fortifying snacks with curcumin and vitamin D3 addresses nutritional deficiencies, while producing affordable, shelf-stable, and health-promoting ready-to-eat snacks enhances food security and supports sustainable food innovation.
|
1. Introduction
Extrusion cooking is a widely used industrial process that applies high pressure, heat, and mechanical force in a short time, resulting in significant transformations in food matrices. This method is effective for producing ready to eat (RTE) snacks with unique textures and flavors. The high-temperature short-time (HTST) nature of extrusion enables rapid cooking and facilitates the production of a wide variety of snack products.1 However, the intense conditions can also degrade heat-sensitive nutrients like vitamins, essential fatty acids, and bioactive compounds, which poses a challenge in the development of nutritionally fortified snacks.2
Fortifying extruded snacks with bioactive and nutritional compounds has become increasingly important because it offers health benefits beyond basic nutrition.3 Traditional fortification methods typically involve the direct addition of vitamins, minerals, or other bioactive compounds to the food matrix before extrusion. However, these methods often result in significant nutrient losses due to the harsh processing conditions, thereby reducing the effectiveness of fortification.4 When carried out as a preceding step, microencapsulation protects bioactive compounds like omega-3 fatty acids and probiotics from the detrimental effects of extrusion by creating a protective barrier, thereby enhancing the nutritional quality of the final product.5,6 Pickering emulsions that are stabilized by solid particles rather than surfactants have attracted increasing attention as delivery vehicles owing to their higher stability and better ability to protect encapsulated bioactive compounds.7 Studies have focused on improving the nutritional characteristics of extruded products by incorporating biopolymers, such as inulin and chitosan.8,9 Incorporating inulin, a prebiotic fiber, into extruded snacks has been shown to enhance dietary fiber content, improve gut health, and boost antioxidant activity, thereby making the snacks nutritionally superior.8 Another study highlighted the role of chitosan in enhancing the functional properties of proteins, reducing fat absorption during extrusion, and improving the textural attributes of snacks.9
Lignin, a natural biopolymer derived from plant cell walls, is gaining attention for its potential to create delivery systems that enhance the bioavailability of encapsulated bioactive compounds.10,11 A recent study showed that lignin, as a Pickering particle, effectively protects sensitive nutrients like vitamins (e.g., vitamin D3) and polyphenols (e.g., curcumin) from heat and mechanical degradation, while improving their stability and delivery.7 Curcumin, a polyphenol found in turmeric, is well-known for its anti-inflammatory, antioxidant, and antimicrobial properties, while vitamin D3 is essential for calcium absorption and bone health.12 The combination of these bioactives in lignin-based Pickering emulsions represents a novel approach for fortification, particularly in high temperature processes like extrusion.
Extruded snacks are commonly made using corn flour, which is favored for its high starch content that supports the puffing and expansion processes during extrusion. However, corn-based extruded snacks often lack significant nutritional value, as they are typically low in protein, fiber, and essential micronutrients.13,14 In developing nutritionally enhanced extruded snacks, the choice of raw materials is of prime importance, and blended flours offer a practical solution.13 These flour blends typically combine grains, tubers, legumes, and seeds, providing a balanced mix of proteins, carbohydrates, fibers, and micronutrients.14,15 Ahmed et al. demonstrated that twin-screw extrusion can produce granules containing iron and vitamin D3, using a blend of corn and lentil flours as the carrier matrix. These granules exhibited excellent chemical stability and bioactive effects when tested on human osteoblast cells, suggesting their potential applications for bone health.14 Grasso reviewed the use of industrial by-products in extruded snacks, emphasizing nutritional and sustainability benefits.15 These studies highlight the versatility of blended flours in developing nutrient-rich extruded snacks. Spent coconut flour, a by-product of virgin coconut oil production, is rich in dietary fiber, protein, and antioxidants, making it an excellent ingredient to enhance the health benefits of snacks, particularly for gluten-free and vegan consumers.16 Similarly, pearl millet flour, rich in essential minerals and other nutrients, is derived from a crop known for its drought resilience.17
This study aimed to develop fortified extruded puffed snacks (EPS) using blends of pearl millet, corn grits, and spent coconut flour, enriched with LPE-encapsulated curcumin and vitamin D3. The EPSs were compared to a corn-based control for their physicochemical, textural, and sensory properties. The stability of the encapsulated bioactives (curcumin and vitamin D3) was also compared to that of the non-encapsulated formulation. This research achieves improved stability of curcumin and vitamin D3 while creating healthier snacks that contribute to improved micronutrient intake and support better overall nutrition.
2. Materials and methods
2.1. Materials
Lignin was isolated from Ayurvedic spent materials and converted to nano lignin using a planetary mill (Pulverisette, Fritsch, Germany), as reported by Abraham.7 Spent coconut meal, a by-product of virgin coconut oil processing, was supplied by Apex Coco Solar Energy Pvt. Ltd (Tamil Nadu, India). The residual oil was removed to produce spent coconut flour (SCF). Pearl millet and corn were sourced from the local market in Thiruvananthapuram, cleaned, ground into flour, and sieved through a 200 mm mesh to achieve uniform particle size. Curcumin and cholecalciferol (vitamin D3) were procured from Sigma-Aldrich Chemicals Pvt. Ltd (Massachusetts, USA). All other chemicals and reagents used in this study were of chromatography grade and were sourced from reliable local suppliers.
2.2. Preparation of O/W Pickering emulsions
Oil-in-water (O/W) Pickering emulsions were formulated using lignin nanoparticles as the stabilizing agent. LNP solution was prepared at a concentration of 2000 ppm at a pH of 8.0. Sunflower oil (10% w/w), containing 50 ppm curcumin and 50 ppm vitamin D3 was used as the dispersed phase. This emulsion was prepared by sonicating with a VCX-750 Vibra Cell (Sonics & Materials, USA) for 45 min (using a 10 s on/10 s off cycle at 50% amplitude) according to the recent study.7 The resulting Pickering emulsion (LPE) was used for fortification of the extruded snacks.
2.3. Preparation of extruded snacks
The composition of the flour blend was determined through preliminary trials and contained 15% Spent Coconut Flour (SCF), 65% Pearl Millet Flour (PMF), and 20% Corn Grits (CG) on a w/w basis. This formulation yielded extrudates with sensory and physical properties comparable to the control made with only corn grits. The control, prepared using only corn grit, is referred to as C1, and the product with optimized flour blend is denoted as S1.
This optimised flour blend was subsequently infused with LPE. The resulting LPE infused (which contained curcumin and vitamin D3 as stated above) extrudates are referred to as S2.
2.4. Extrusion process
The blended flour (S1) was uniformly mixed using a laboratory-scale mixer (Basic Technology Private Ltd, Kolkata, India) and conditioned with 16 ± 1% water to achieve optimal expansion and texture during extrusion cooking. The hydrated flour was equilibrated for 1 h at ambient temperature (30 ± 2 °C) in an airtight container before extrusion. After conditioning, the flour mixtures were extruded. For all the EPSs, the time and temperature were kept the same.
Extrudates were prepared using a laboratory scale twin screw extruder employing hot extrusion technology (L-TSE, Basic Technology Private Ltd, Kolkata, India). The operating parameters of the extrusion process including screw speed, feed moisture content, and cooking temperature, were optimized through preliminary trials. The extrusion process is illustrated in Fig. 1. Before operation, the extruder was primed, and a 2 mm circular die was attached to the barrel end. The barrel was heated to 105 °C and 80 °C. The screw speed (350 rpm), feeder speed (10 rpm), and cutter speed (70 rpm) were adjusted via the main control panel. Once the desired barrel temperature was reached, the optimized flour blend was fed using a semi-automatic feeder, and the extruded product emerged from the die. The extrudates were cut uniformly using a cutter assembly, cooled to room temperature, and promptly packed into pouches. The extrudates were toasted in a conventional oven (Bajaj OTG oven, India) at 160 °C for 3 min. This temperature–time combination was determined through trials. The toasted extruded products were coated with refined sunflower oil and a seasoning agent (Symega Food Ingredients Limited, Cochin, Kerala, India) for sensory appeal.
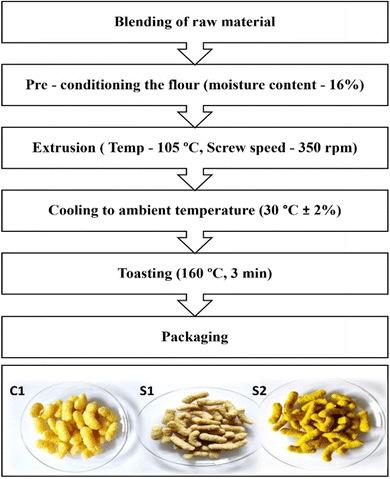 |
| Fig. 1 Flowchart of the extrusion process for producing EPS: C1 – control, S1 – EPS with blended flour, and S2 – EPS with lignin-based Pickering emulsion (LPE) fortification. | |
2.5. Analysis of the physical and functional attributes of extrudates
2.5.1. Proximate composition of flours and extrudates. Moisture, ash, fat, and protein contents were determined according to the procedures outlined by the AOAC.18 Samples were oven-dried at 105 °C, and then transferred to a desiccator and allowed to cool to room temperature (32.0 ± 2 °C). For determining ash content, the mass of the samples was recorded before and after the ashing step using a muffle furnace (550 °C for 6 h). Protein content was determined using the Micro Kjeldahl method with a nitrogen-to-protein conversion factor of 6.25. The fat content was determined via Soxhlet extraction. Total carbohydrate was determined by difference.
2.5.2. Estimation of dietary fiber. The dietary fiber content of EPSs – S1, S2 and C1 – was determined according to the Bureau of Indian Standards Method with a slight modification.19 Fat- and moisture-free samples were autoclaved with water, the pH was adjusted, and pepsin, pancreatin, and glucoamylase were added. The mixture was then incubated for enzymatic digestion. After incubation, the pretreated extract was centrifuged at 1
118
000 × g for 10 min to separate the residue and supernatant. The residue, containing insoluble fiber, was collected, washed three times with acetone and diethyl ether, and lyophilized to obtain a stable weight of insoluble dietary fiber. The supernatant, containing soluble fiber, was precipitated using ethanol and refrigerated overnight. It was then centrifuged (Model 7780; KUBOTA, Tokyo, Japan) again at 1
118
000 × g for 10 min, and the resulting residue was collected. This residue was washed three times with alcohol, acetone, and diethyl ether, and then lyophilized in a pilot scale freeze dryer (SP Scientific VirTis Genesis 35L Pilot Lyophilizer, Warminster, USA) to achieve a constant weight, yielding the soluble dietary fiber.20
2.5.3. Expansion ratio. The expansion ratio of an EPS was measured by comparing the diameter of the extruded product to that of the die.21 A digital vernier calliper (0.1 accuracy) was used to measure the diameter of ten randomly selected extrudates, and the mean value was recorded. The expansion ratio of the extrudates was calculated using eqn (1). |
 | (1) |
2.5.4. Bulk density. The length and diameter of ten EPS samples were measured using a digital vernier caliper. The mass of these 10 EPS samples was also measured. The bulk density was measured using eqn (2) considering they were cylindrical in shape.22 |
Bulk density (g cm−3) = 4m/(πd2L).
| (2) |
where, L is the length (cm), m is the mass (g) and d is the diameter (cm) of the extruded snacks, respectively.
2.5.5. Colour analysis. The colour parameters of the EPS were measured using a colorimeter (Hunter lab, Colour Flex EZ, Virginia, USA). The empty cuvette was calibrated initially using standard white and black plates. The hue of the samples was assessed on the L* (lightness), a* (red-green), and b* (yellow-blue) colour systems. To measure the color values, the extruded products were pulverized and transferred into cylindrical glass sample cuvettes, which were then placed in the sample port. The color values were recorded after 10 rotations of each sample, with measurements taken in triplicate.23
2.5.6. Texture analysis. The textural properties of extrudates were analyzed using a texture analyzer (TA. XT texture analyzer, Stable Microsystems, Surrey, England). Texture profile analysis (Stable Microsystems) was employed to measure properties such as hardness, gumminess, chewiness, fracturability. A 2 mm cylindrical probe was used in these measurements. The tests were carried out in compression mode using the following parameters: pre-test speed: 1 mm s−1, post-test speed: 5 mm s−1, test speed: 1 mm s−1, trigger force: 5 g, and distance (compression): 10 mm. The texture of EPSs was analyzed at three different positions of the sample, and each sample was tested in triplicate.
2.5.7. Sensory analysis. Sensory analysis was conducted to evaluate the acceptability of extrudate snacks. A semi trained panel of 25 members, aged 25 to 45, assessed the samples based on appearance, color, aroma, taste, and overall acceptance. Panelists were instructed to focus on one attribute at a time and cleanse their palate with water between samples to prevent cross-sensory contamination. Participants were encouraged to provide candid feedback based on their overall sensory experience. A 9-point hedonic scale was used for the evaluation, with scores ranging from 1 (‘dislike extremely’), 5 (‘neither like nor dislike’), and 9 (‘like extremely’), following the guidelines of ISO 4121:2003. The average score across all criteria was calculated to determine the sensory attributes.24
2.5.8. Cross-sectional microscopic images. The microstructure of the EPS was observed using an Olympus CX41 microscope (Olympus, Shinjuku, Tokyo, Japan). Images of the surface morphology of the extrudates were captured at 4× magnification using a camera attached to the microscope and equipped with QCapture software (QImaging).
2.5.9. Observing microscopic morphology. The EPSs were sliced and mounted onto an aluminum specimen holder using carbon tape. Prior to microscopic examination, a thin layer of gold was applied using a gold/palladium sputter coater (SC7620, Emitech, Quorum Technologies Ltd, Kent, UK). Scanning electron microscopy (ZEISS; EVO 18, Germany) was used to acquire and analyse the microscopic surface morphology of the extruded samples. The instrument operated at an accelerating voltage of 15 kV. Micrographs were captured at 500× magnification.
2.5.10. Stability of curcumin and vitamin D3. The bioactive stability, representing the percentage retention of curcumin and vitamin D3 in the extruded product, was determined using high-performance liquid chromatography with a diode-array detector (HPLC-DAD). For the analysis, 1 g of powdered EPS was mixed with 5 mL of methanol and magnetically stirred for 3 h. The methanolic extract was centrifuged at 1
118
000 × g for 10 min, and the supernatant was collected and filtered through a 0.22 μm PTFE syringe filter. The HPLC analysis was performed as previously described by the authors.7 Briefly, a Prominence UFLC system (Shimadzu, Japan) was used, equipped with a Phenomenex Gemini C18 column (250 × 4.6 mm, 5 μm). The system operated with an isocratic flow of 100% methanol at a rate of 1 mL min−1, with detection wavelengths of 420 nm for curcumin and 261 nm for vitamin D3. Retention times for curcumin and vitamin D3 were 3.2 min and 7.6 min, respectively. The column temperature was maintained at 40 °C throughout the analysis.
2.6. Statistical analysis
Experiments were carried out in triplicate, except where otherwise mentioned. All analyses were conducted with three technical replicates, and the results are presented as mean ± standard deviation (SD). The data were subjected to analysis of variance (ANOVA) using GraphPad Prism 5.0 statistical software to determine if there were statistically significant differences between any two mean values or samples (p < 0.05).
3. Results and discussion
3.1. Nutritional profile of extruded snacks
The nutritional profiles of EPS are shown in Table 1. The fat content showed a slight increase in S2 (1.64%), attributed to the inclusion of sunflower oil in the emulsion, while S1 (0.10%) and C1 (0.08%) showed comparatively lower fat levels. Variations in carbohydrate content were observed, likely due to differences in ingredient composition, such as dietary fiber. Other parameters, including moisture and ash content, showed no significant differences among C1, S1, and S2. EPS produced using the blended flour showed significantly higher protein content as compared to C1. Typically, extruded snacks are made with corn flour to achieve better expansion and texture. However, it is low in protein and other essential nutrients. Over-reliance on corn flour results in nutritionally deficient snacks with a high glycemic index. The use of blended flour in this study produced snacks with increased protein content (13.5%) in S1 against 9.8% in the corn-based control (C1). The increased protein content resulted from the spent coconut flour (SCF), a known protein-rich ingredient. SCF contains 25.5% protein; thus, it significantly contributed the protein content in the formulation. Additionally, pearl millet, with a reported protein content of 12.5%,25 further enhanced the protein profile of the blend. A similar increase in protein content was reported in enriched extrudates; for example, Gojiya et al. observed an increase in protein content from 14.0% to 26.7% when 10% to 40% defatted sesame flour was added into the formulation.26
Table 1 Nutritional profile of EPSs made from corn grit (C1), blended flours (S1), and blended flours infused with curcumin and vitamin D3 (S2)a
Composition (Wb%) |
C1 |
S1 |
S2 |
Different superscripts within the same row denote significant differences between the samples and control (p > 0.05; n = 3). |
Protein |
9.8 ± 0.0a |
13.5 ± 0.1b |
13.8 ± 0.2b |
Moisture |
2.9 ± 0.5a |
2.8 ± 0.1b |
3.3 ± 0.0c |
Fat |
0.1 ± 0.2a |
0.1 ± 0.2a |
1.6 ± 0.1c |
Ash |
1.4 ± 0.2a |
2.0 ± 0.1b |
1.74 ± 0.0b |
Carbohydrate |
85.8 ± 0.2a |
81.6 ± 0.5b |
79.5 ± 0.1c |
Total dietary fiber |
13.0 ± 0.2 a |
18.9 ± 0.1b |
19.2 ± 0.1c |
Insoluble dietary fiber |
9.6 ± 0.1a |
14.2 ± 0.1b |
14.6 ± 0.2b |
Soluble dietary fiber |
3.4 ± 0.2a |
4.6 ± 0.1b |
4.6 ± 0.2b |
The addition of PMF and SCF to the blended flour significantly enhanced the dietary fiber content in the EPS. SCF contains 54.0% total dietary fiber, including 44.5% insoluble fiber and 9.5% soluble fiber. Similarly, pearl millet contributes to the fiber profile of S1, as it contains 11.5% total dietary fiber, comprising 9.1% insoluble fiber and 2.3% soluble fiber.17 S1 contained significantly higher levels of total dietary fiber (18.9%), insoluble dietary fiber (14.2%), and soluble dietary fiber (4.6%) compared to C1, which contained 13.0%, 9.6%, and 3.4%, respectively (Table 1). This increase in fiber content can be attributed to the contributions of pearl millet and SCF. A recent study reported a 5% increase in protein content in extrudates with the addition of green gram and cowpea flour, along with a 3% reduction in total carbohydrate content, making it a healthier snack option.27 Similarly, another study reported significant increases in protein (7.6% to 27.2%) and fiber content (0.1% to 2.6%) in extrudates with the incorporation of cowpea (10% to 30%) and whey protein concentrate (WPC) (5% to 20%) compared to the control. The formulation containing 15% cowpea and 5% WPC achieved the highest acceptance, with a protein content of 14.6% and a total dietary fiber content of 1.6%.3 These findings highlight that the use of blended flour containing fiber- and protein-rich ingredients can significantly improve the nutritional profile of extruded snacks.13,28 The snacks developed in this study were significantly dense in protein and dietary fiber and can be considered a healthier option.
3.2. Expansion ratio and bulk density
The expansion ratio and bulk density are important indicators of texture, mouthfeel, and overall consumer acceptance of extruded snacks. Bulk density reflects the degree of puffing in extruded products and the blended flour and LPE are expected to alter the bulk density of the snack samples. As shown in Fig. 2a, the bulk density of S1 (0.70 g cm−3) and S2 (0.72 g cm−3) were higher than that of the control C1 (0.50 g cm−3). The similar bulk density values of S1 and S2 indicated that the incorporation of LPE did not significantly alter the physical or internal structure of the snacks. Bulk density is influenced by the interactions among starch, protein, and fiber components.29 SCF is rich in dietary fiber, which enhances the compactness of extruded snacks and increases their bulk density. The observed increase in bulk density with the addition of dietary fiber suggests that higher fiber content contributes to a denser and more compact extrudate structure. Previous studies have shown that increased fiber content in extruded products correlates with higher bulk density.
The higher expansion ratios typically signify lighter, crispier textures. The control sample (C1) had the highest expansion ratio of 4.01, while the expansion ratio of both S1 and S2 was only 37.2% of that of the control (Fig. 2b). The inclusion of soluble and insoluble fibers in extruded snacks significantly influences their physicochemical characteristics, particularly the expansion ratio and bulk density. The observed decrease in the expansion ratio can be attributed to the increased protein and fiber content, resulting in a denser, less expanded structure. An increase in protein content also affects the extent and rate of starch gelatinization, which could lead to a decrease in the expansion ratio.29 Studies have shown that incorporating dietary fibers from various sources, such as polydextrose, gum acacia, inulin, xanthan gum, and resistant starch, into corn-based feed reduces the expansion of extruded snacks.30 The addition of fiber increases the viscosity of the starchy dough during extrusion, reducing the water available for gelatinization and thereby limiting starch expansion at the die.31
 |
| Fig. 2 (a) Bulk density and (b) expansion ratio content in the extrudates. Different letters denote significant differences between the samples (p < 0.05). | |
Fiber typically functions as an inert filler within the expanded starch matrix. At low concentrations, the fiber is insufficient to be evenly distributed throughout the extrudate matrix. However, as its content increases, the starch matrix may become overwhelmed, causing it to collapse as fiber particles penetrate. This process ultimately reduces the expansion ratio.32 A previous study reported reduced expansion index values in extruded products containing okara and mung bean powder. This was attributed to the high fiber content (60.8% in okara; 15.2% in mung bean) and notable protein levels (26.5% in okara; 20.2% in mung bean).32 Similarly, extruded snacks made with 80–90% broken rice and 10–20% lupin flour showed that higher rice content increased the expansion index, whereas higher lupin flour content reduced it.33
During snack extrusion, dietary fiber significantly impacts starch gelatinization by competing with starch for available water. This competition reduces amylose leaching and limits the full gelatinization of amylose and amylopectin, potentially affecting amylose retrogradation and disrupting the structure of amylopectin. Additionally, fiber increases dough viscosity, disrupting the starch matrix and hindering expansion. These structural disruptions weaken the matrix, reducing the expansion ratio and producing denser snacks with higher bulk density and less puffiness. As a result, the texture of fiber-enriched snacks differs markedly from fiber-free products.29,34
The extruded product formulated with 15% spent coconut flour (SCF) and 65% pearl millet flour successfully replaced 80% of corn flour, marking a significant step toward replacing traditional corn-based snacks. Similarly, an extruded snack developed using 80% pearl millet flour, 10% African walnut flour, and 10% corn starch achieved moderate sensory acceptance.35
3.3. Texture profile analysis
Texture attributes like hardness, fracturability, gumminess, and chewiness are essential for evaluating the quality and appeal of extruded snacks. Hardness affects the perception of freshness, while fracturability can be correlated with the crispiness. Gumminess indicates the effort required to chew, and chewiness influences the overall sensory experience. All of these attributes are vital for creating products that satisfy the consumers. The texture qualities of the extruded snacks are shown in Fig. 3. The increase in hardness observed in S1 and S2 compared to C1 is primarily due to the higher protein and fiber content from SCF and PMF, which disrupt the starch matrix, leading to reduced expansion and a denser texture. The strong relationship between hardness and expansion suggests that higher protein and fiber levels enable the formation of a compact structure that affects the overall texture. This type of relationship was also noted by Kumar et al.,9 who found that fortification of snacks with chitosan led to the increase in dietary fibre content. Similarly, the incorporation of 5 to 20% tomato pomace powder in extruded snacks was found to increase hardness.36 A protein–starch interaction that inhibited expansion might be attributed to an increase in hardness in sorghum-based extruded snacks when protein-rich soy meal flour was added up to 20% of the formulation.37 The control sample (C1) had the highest chewiness compared to S1 and S2. Earlier research also showed that chewiness was significantly reduced from 1.63 N to 0.42 N when corn flour was supplemented with soy and chickpea flour about 20–40%.38 The gumminess of EPS increased with the addition of SCF and PMF compared to the control. Fiber acts as both a structure-forming and water-absorbing component, creating a denser matrix that resists deformation and thereby enhances gumminess. This aligns with previous studies showing that incorporating soy and chickpea flour into corn flour significantly increases gumminess, demonstrating a positive correlation between dietary fiber content and gumminess in extruded products. It has also been reported that gumminess increased significantly from 0.33 N in the control to 1.99 N when 20% soy and 20% chickpea flour were substituted for corn flour.38 Fracturability is an important texture attribute that reflects the brittleness or crispness of a snack. A higher fracturability value indicates that the snack can break with less force, indicating its crunchy and crisp texture. In extruded snacks, it is affected by several factors, including the composition of the ingredients (e.g., starch, fiber, moisture, fat, or emulsifiers), the extrusion process parameters (e.g., temperature and pressure), and the extent of product expansion. It was observed that the addition of fiber rich SCF and PMF resulted in a slight reduction in fracturability compared to the control. Fiber-rich ingredients, such as SCF and PMF, disrupt the continuous starch network, reducing product expansion and creating a denser texture. This compact structure absorbs more force before breaking, thereby reducing the product's brittleness. The lower expansion caused by high fiber content also decreases porosity, making the product less brittle and slightly tougher to fracture. These findings are consistent with studies that reported similar effects when high-fiber ingredients, such as tomato pomace, were incorporated into extruded snacks, resulting in reduced fracturability due to the compact and dense structure.36,39
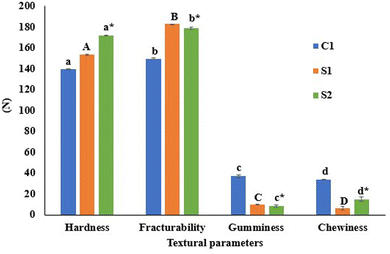 |
| Fig. 3 Texture profile of extruded puffed snacks. All the parameters expressed in Newton (N). Different letters denote significant differences in the values between the samples (p < 0.05). | |
Notably, the similar texture profiles of S1 and S2 suggest that incorporating lignin-based Pickering emulsions (containing curcumin and vitamin D3) does not significantly alter the core textural properties of the snacks. This finding indicates that these emulsions can be used to fortify extruded snacks without compromising their overall texture profile, making them a promising option for enhancing nutritional value.
3.4. Color analysis
The color properties of the extruded snacks, as indicated by the L* (lightness), a* (red-green), b* (yellow-blue), and yellowness index (YI) values, showed significant variation among C1, S1, and S2 in terms of colour (Table 2). Sample C1 exhibited the highest lightness value suggesting a brighter appearance compared to the other samples. The a* and b* values of C1 indicated a more pronounced reddish and yellowish hue, contributing to its high yellowness index (YI = 80.86). This is due to the presence of corn grits, whose yellow colour primarily results from natural carotenoid pigments, particularly lutein and zeaxanthin.
Table 2 Colour analysis of EPSs formulated with different combinations of blended floursa
Extruded puffed snack |
L* |
a* |
b* |
Yellowness index (YI) |
Entries within the same column with different superscripts denote significant differences between the sample and control (p > 0.05; n = 3). |
C1 |
67.9 ± 0.1a |
3.9 ± 0.0a |
38.5 ± 0.2a |
80.9 ± 0.0a |
S1 |
61.5 ± 0.0b |
3.0 ± 0.0b |
24.9 ± 0.1b |
57.8 ± 0.1b |
S2 |
67.8 ± 0.3a |
−0.4 ± 0.0c |
62.2 ± 0.2c |
131.3 ± 0.5c |
In contrast, S1 displayed the lowest lightness value and reduced a* and b* values, resulting in the lowest yellowness index (YI = 57.78). The lower colour intensity of S1 can be attributed to the incorporation of SCF and PMF, with the latter likely responsible for the darker colour. Pearl millet contributes to the dark color of extrudates due to its high phenolic content, including tannins and flavonoids, which act as natural pigments. During extrusion, the high temperatures and pressures trigger the Maillard reaction, forming brown melanoidins that further enhance the dark color. Pigments such as anthocyanins present in the bran layer of pearl millet further intensify the darkening effect during processing. It has been reported that reactions such as Maillard browning, caramelization, pigment degradation, and lipid oxidation contribute to the reduction in lightness of extruded snacks.40 S2, however, exhibited a markedly different colour profile. While its lightness was comparable to that of C1, the a* value shifted toward the green spectrum, and its b* value was significantly higher than that of other samples, resulting in the highest yellowness index (YI = 131.26). This indicates that S2 had a strong yellow hue due to the presence of curcumin and lignin, which carry yellow and brown pigments.7 The colour profile of the extruded snacks formulated in this work aligns with research showing that the addition of vegetable ingredients and other agricultural by-products to rice-, barley-, and corn-based extruded snacks improves their colour desirability.15,41,42 Furthermore, the inclusion of lignin in extruded snacks not only supports the stability of bioactives like curcumin and vitamin D3 but also enhances the product's visual appeal.
3.5. Sensory profile of snacks
The sensory profile of the EPSs was evaluated to determine their potential acceptance by the consumers. Attributes such as color, texture, flavor, and overall acceptability were evaluated (Fig. 4). All samples received mean scores above 8.0 out of 9 for each attribute, indicating a high level of acceptance among the panellists. The panellists noted that the sample fortified with LPE (S2) had a distinct darker yellow hue compared to both the control and the one produced using blended flour without the emulsion fortification, yet it received similar sensory scores for texture, flavor, and crispiness.
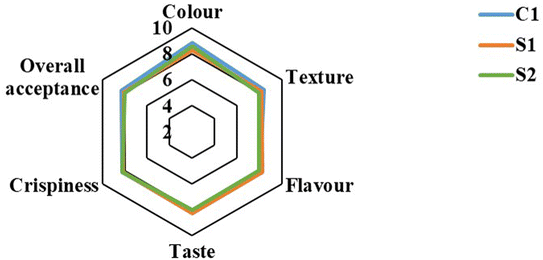 |
| Fig. 4 Sensory profiles of extruded puffed snacks. Corn grit (C1) and blended flours (S1), blended flours infused with curcumin and vitamin D3 (S2). | |
The high sensory scores across all samples suggest that the inclusion of SCF and PMF did not negatively impact consumer acceptability. The positive sensory attributes of S1 and S2 snacks, including taste, texture, and crispness could be attributed to the unique combination of pearl millet and coconut flour, which created a distinct flavor profile compared to commercial snacks. The crispness resulted from the extrusion process and the interaction of the ingredients.
The distinct darker yellow colour of the product fortified with LPE (S2) enhanced its visual appeal without compromising texture and flavour. A similar result was observed with the addition of tomato pomace powder to ready-to-eat (RTE) snacks. When included at levels of 5–10%, the snacks achieved the highest sensory scores. However, acceptability decreased at inclusion levels above 10%.36 Likewise, Hoglund43 incorporated bilberry press cake into extruded snack formulations. Substitutions of up to 25% were tested, but products with 10% inclusion were the most preferred by panellists. In another study, oat flour was added at levels of 5–20% to extruded snacks. Formulations containing 10% oat flour achieved the highest overall sensory scores.44 These studies highlighted that moderate inclusion levels of natural fiber-rich ingredients enhanced snack sensory appeal, whereas excessive amounts reduced acceptability. The findings confirm that the blend of SCF, PMF, and corn grits—fortified with LPE—improves the nutritional profile of extruded snacks while maintaining strong sensory appeal. Notably, the blended flour in this study contained 15% SCF and 65% PMF, replacing 80% of corn grits in extruded puffed snacks while still delivering desirable sensory properties.
3.6. Microstructural analysis
The microstructural analysis of EPS samples using an optical microscope (Fig. 5a–c) revealed notable differences among C1, S1, and S2. The control sample (C1) displayed a uniform pore distribution with large, homogeneous edges, indicating greater expansion and aeration during extrusion. In contrast, S1 had smaller pores and a denser, less porous structure due to the higher fiber content in SCF and PMF, which promoted a more compact matrix. S2 showed a similar structure to S1 but with a distinct yellow hue caused by the presence of curcumin.
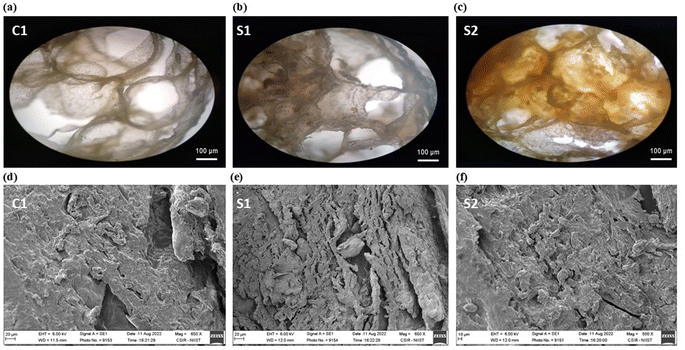 |
| Fig. 5 Microscopic images, (a–c) optical microscopy images, and (d–f) SEM images of extruded puffed snacks. | |
The microstructural characteristics of EPS samples—C1, S1, and S2—were examined through SEM (Fig. 5d–f). Fig. 5d reveals a rough, fractured surface with air pockets formed during the extrusion process. This porous structure results from the expansion of starch and protein components at the end of the extrusion process. The visible cracks may be due to moisture evaporation and rapid expansion. In contrast, Fig. 5e shows a more fibrous, layered structure with elongated strands, suggesting partial melting of starch or proteins that solidify into fibrous formations, enhancing the snack's crunchiness. Fig. 5f displays a microstructure similar to that of the control (C1), indicating that LPE did not affect the EPS microstructure. Similar findings were reported in extruded snacks made from rice starch and pea protein blends, which showed an even distribution of proteins and carbohydrates throughout the porous structure.45
3.7. Stability of bioactives in lignin-encapsulated extrudates
Emulsions stabilized by lignin show promise in encapsulating health-promoting compounds like vitamin D3 and curcumin for fortifying snack products.11 Incorporating these bioactive compounds into extruded snacks can significantly enhance their nutritional profile. Preserving the stability of curcumin and vitamin D3 within the emulsion is crucial for maintaining the health benefits of fortified snacks over time. To evaluate LPE's ability to protect curcumin and vitamin D3 during extrusion, a control EPS (C2) was prepared by adding these compounds directly to the blended flour without emulsifying with lignin particles. The stability of curcumin and vitamin D3 in C2 was then compared to that in the S2 sample. Fig. 6 shows the chromatograms of the extrudates S2 and C2, showing the stability of curcumin and vitamin D3 in the products. The bioactive stability of curcumin and vitamin D3 in S2 was 69.0 and 65.7%, respectively. In contrast, the stability of curcumin and vitamin D3 significantly decreased to 7.4 and 8.2%, respectively in C2. These results indicate that lignin stabilised emulsions effectively protect curcumin and vitamin D3 during the extrusion process.
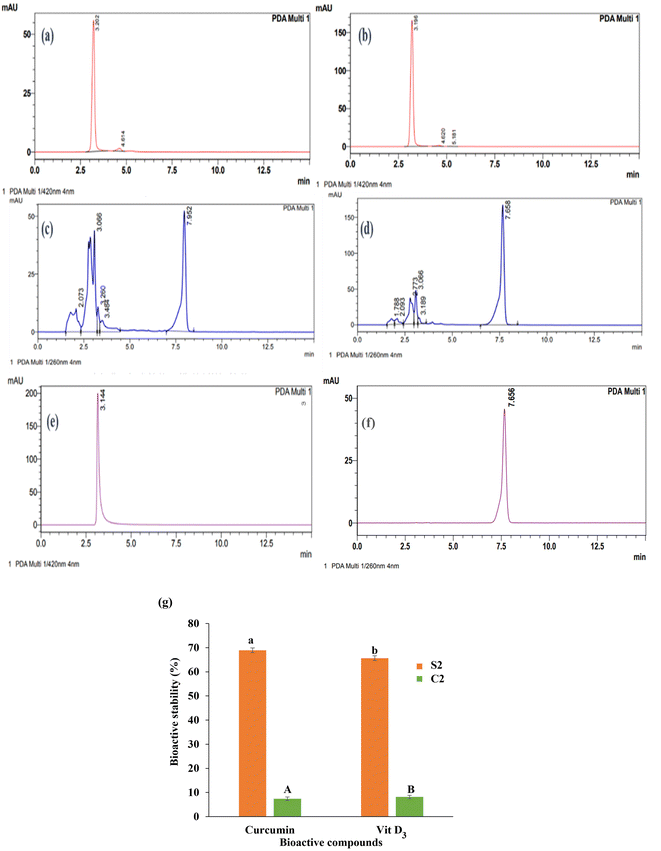 |
| Fig. 6 HPLC chromatograms of extruded snacks showing bioactive stability: (a) curcumin content in C2, (b) curcumin content in S2, (c) vitamin D3 content in C2, (d) vitamin D3 content in S2 (e) standard curcumin, and (f) standard vitamin D3. (g) Bioactive stability of curcumin and vitamin D3 in extruded snacks; different letters denote significant differences in values between C2 and S2. | |
The significant difference in bioactive stability between S2 and C2 underscores the vital role of lignin as a protective agent in extrusion processes. Lignin acts as a stabilizer, shielding curcumin and vitamin D3 from the harsh thermal conditions, thereby maintaining their integrity and activity. Without lignin, as observed in C2, curcumin and vitamin D3 are highly susceptible to thermal degradation their stability is compromised. These findings are in accordance with those of Bertolo,46 which demonstrated that lignin particles protect bioactive compounds (such as curcumin) in food systems. The notably higher stability of curcumin and vitamin D3 in S2 underscores lignin's potential as a protective agent, demonstrating its suitability for the production of fortified extruded snacks. Lignin has also been shown to possess antioxidative properties, with the ability to protect oxygen-sensitive compounds.47 In this study, lignin's role as a Pickering particle was vital for successfully encapsulating curcumin and vitamin D3, ensuring their stability during high-temperature extrusion (110 ± 2 °C). These findings align with the existing literature, where lignin is consistently highlighted as an encapsulating and protective agent for bioactive compounds.47,48
4. Conclusion
This study successfully developed and characterized nutritionally and functionally superior extruded puffed snacks (EPSs). The EPSs were formulated using a blend of spent coconut, pearl millet flour, corn grits, and lignin Pickering emulsions (LPE) in which curcumin and vitamin D3 were encapsulated. Notably, 80% of the corn flour typically used in traditional snacks was replaced with the blended flour, resulting in increased protein and dietary fiber content. Although the expansion ratio, bulk density, and textural properties of the blended flour snacks were lower than those of traditional corn flour snacks, the inclusion of LPE enhanced their nutritional profile. The overall sensory acceptance of these snacks was comparable to that of conventional snacks, indicating their suitability as nutritionally enriched alternatives. The study also confirmed that curcumin and vitamin D3 were effectively encapsulated within the snacks' matrix, with their structural integrity and bioactivity preserved during the extrusion process. Lignin played a vital role in maintaining the stability and bioavailability of curcumin and vitamin D3 in the snacks. These findings highlight the potential of lignin-stabilized emulsions for incorporating bioactive compounds into healthier, ready-to-eat snacks.
Data availability
The data for the manuscript can be made available on request.
Author contributions
Billu Abraham: conceptualization, data curation, investigation, methodology, validation, writing – original draft preparation. Heeba Shakeela: data curation, writing – draft preparation. Pavithra P.: methodology, investigation. Charles Brennan: investigation, data analysis. Nitin Mantri: investigation, data interpretation. Benu Adhikari: supervision, validation, writing – review & editing. Nisha P.: conceptualization, project administration, resources, supervision, validation, visualization, writing – review & editing.
Conflicts of interest
The authors declare that there are no conflicts of interest regarding the publication of this paper.
Acknowledgements
The authors gratefully acknowledge CSIR-NIIST and RMIT for providing the necessary facilities and support for this research. The first author expresses gratitude for the CSIR-SRF direct fellowship. The partial financial support from the Department of Science and Technology, Government of India, is also acknowledged. Special thanks to Mr Pratheesh Kumar for assistance with extrusion processing and Mr Hareesh for conducting SEM analysis.
References
- Y. Abilmazhinov, G. Bekeshova, A. Nesterenko, Z. Dibrova, V. Ermolaev, E. Ponomarev and V. Vlasova, Food Sci. Technol., 2023, 43 DOI:10.5327/fst.80621.
- K. Prabha, P. Ghosh, S. Abdullah, R. M. Joseph, R. Krishnan, S. S. Rana and R. C. Pradhan, Future Foods, 2021, 3, 100019, DOI:10.1016/j.fufo.2021.100019.
- H. N. Dilrukshi, D. D. Torrico, M. A. Brennan and C. S. Brennan, Food Chem., 2022, 389, 133107, DOI:10.1016/j.foodchem.2022.133107.
- C. I. Onwulata, J. Food Process. Preserv., 2013, 37, 510–532, DOI:10.1111/j.1745-4549.2012.00680.x.
- E. Feizollahi, Z. Hadian and Z. Honarvar, Curr. Nutr. Food Sci., 2018, 14, 90–103, DOI:10.2174/1573401313666170728151350.
- M. Sultana, E. S. Chan, J. Pushpamalar and W. S. Choo, Trends Food Sci. Technol., 2022, 123, 69–86, DOI:10.1016/j.tifs.2022.03.006.
- B. Abraham, H. Shakeela, L. P. Devendra, K. B. Arun, K. V. Ragavan, C. Brennan, N. Mantri, B. Adhikari and P. Nisha, Food Chem., 2024, 458, 140284, DOI:10.1016/j.foodchem.2024.140284.
- K. C. Tsokolar-Tsikopoulos, I. D. Katsavou and M. K. Krokida, J. Food Sci. Technol., 2015, 52, 6170–6181, DOI:10.1007/s13197-015-1718-2.
- R. Kumar, K. M. Xavier, M. Lekshmi, A. Balange and V. Gudipati, Carbohydr. Polym., 2018, 194, 267–273, DOI:10.1016/j.carbpol.2018.04.050.
- K. Wang, M. Zhu, Z. Yang, L. Bai, S. Huan and C. Wang, ACS Sustainable Chem. Eng., 2023, 11, 9132–9142, DOI:10.1021/acssuschemeng.3c01972.
- B. Abraham, V. L. Syamnath, K. B. Arun, P. F. Zahra, P. Anjusha, A. Kothakotta, Y. H. Chen, V. K. Ponnusamy and P. Nisha, Sci. Total Environ., 2023, 881, 163316, DOI:10.1016/j.scitotenv.2023.163316.
- M. A. Al-Azzawi, A. J. Maftool, A. A. Al-Shimary and A. A. Mohammed, Int. J. Med. Sci. Dent. Health, 2023, 9(12), 37–46, DOI:10.55640/ijmsdh-09-12-08.
- C. A. Amadeu, S. M. Martelli and F. M. Vanin, Cereal Chem., 2024, 101(3), 450–467, DOI:10.1002/cche.10765.
- J. Ahmed, B. R. Giri, M. A. Reza, S. S. B. Qasim, L. Thomas, H. Al-Attar and M. Maniruzzaman, J. Food Sci., 2024, 89(1), 435–449, DOI:10.1111/1750-3841.16841.
- S. Grasso, Trends Food Sci. Technol., 2020, 99, 284–294, DOI:10.1016/j.tifs.2020.03.012.
- H. Shakeela, K. Mohan and P. Nisha, Sustainable Food Technol., 2024, 2(3), 497–505, 10.1039/D3FB00247K.
- T. Longvah, R. Ananthan, K. Bhaskarachary and K. Venkaiah, Indian Food Composition Tables 2017, National Institute of Nutrition, Indian Council of Medical Research, Hyderabad, Telangana, India, 2017, https://www.nin.res.in/ebooks/IFCT2017.pdf Search PubMed.
- AOAC, Official Methods of Analysis, Association of Official Analytical Chemists, 1990, vol. 62, pp. , pp. 2742–2744 Search PubMed.
- Bureau of Indian Standards, Method for Estimation of Total Dietary Fibre in Food Stuffs, Indian Standards Institution, New Delhi, India, 1984, p. , p. 11062 Search PubMed.
- K. B. Arun, S. Thomas, T. R. Reshmitha, G. C. Akhil and P. Nisha, J. Funct. Foods, 2017, 31, 198–207 CrossRef CAS.
- A. A. Tas and A. U. Shah, Trends Food Sci. Technol., 2021, 116, 701–711, DOI:10.1016/j.tifs.2021.08.016.
- S. Suri, A. Dutta, N. C. Shahi, R. S. Raghuvanshi, A. Singh and C. S. Chopra, LWT--Food Sci. Technol., 2020, 134, 110164, DOI:10.1016/j.lwt.2020.110164.
- H. Shakeela, N. M. Mini, B. Abraham, N. Natarajan and P. Nisha, Int. J. Food Eng., 2022, 18(5), 399–409, DOI:10.1515/ijfe-2021-0337.
- S. Wichchukit and M. O'Mahony, J. Sci. Food Agric., 2015, 95(11), 2167–2178, DOI:10.1002/jsfa.6993.
- M. M. Navami, B. Abraham, H. Archana and P. Nisha, JSFA Rep., 2023, 3(8), 377–386, DOI:10.1002/jsf2.141.
- D. Gojiya, P. Davara, V. Gohil and M. Dabhi, J. Food Process. Preserv., 2022, 46(12), e17203, DOI:10.1111/jfpp.17203.
- G. Karun, A. Sukumar, G. Nagamaniammai and R. Preetha, J. Food Sci. Technol., 2023, 60(3), 947–957, DOI:10.1007/s13197-022-05390-8.
- J. Delić, P. Ikonić, M. Jokanović, T. Peulić, B. Ikonić, V. Banjac, S. Vidosavljević, V. Stojkov and M. Hadnađev, Innovative Food Sci. Emerging Technol., 2023, 87, 103419, DOI:10.1016/j.ifset.2023.103419.
- A. Aussanasuwannakul, C. Teangpook, W. Treesuwan, K. Puntaburt and P. Butsuwan, Foods, 2022, 11(19), 2967, DOI:10.3390/foods11192967.
- Y. J. Han and T. T. T. Tran, LWT--Food Sci. Technol., 2018, 96, 1–6, DOI:10.1016/j.lwt.2018.05.014.
- D. A. Pai, O. A. Blake, B. R. Hamaker and O. H. Campanella, J. Cereal Sci., 2009, 50(2), 227–234, DOI:10.1016/j.jcs.2009.05.007.
- P. Wang, Y. Fu, L. Wang, A. S. Saleh, H. Cao and Z. Xiao, Starch/Staerke, 2017, 69(7–8), 1600201, DOI:10.1002/star.201600201.
- L. C. Oliveira, C. M. Rosell and C. J. Steel, Int. J. Food Sci. Technol., 2015, 50(6), 1504–1514, DOI:10.1111/ijfs.12778.
- M. S. Alam, J. Kaur, H. Khaira and K. Gupta, Crit. Rev. Food Sci. Nutr., 2016, 56(3), 445–473, DOI:10.1080/10408398.2013.779568.
- S. S. Sobowale, Y. O. Kewuyemi and A. T. Olayanju, SN Appl. Sci., 2021, 3, 1–12, DOI:10.1007/s42452-021-04808-w.
- S. Yagci, R. Calıskan, Z. S. Gunes, E. Capanoglu and M. Tomas, Food Chem., 2022, 368, 130847, DOI:10.1016/j.foodchem.2021.130847.
- A. Rodríguez-Vidal, H. E. Martínez-Flores, E. González Jasso, G. Velázquez de la Cruz, A. K. Ramírez-Jiménez and E. Morales-Sánchez, J. Texture Stud., 2017, 48(3), 249–257, DOI:10.1111/jtxs.12234.
- F. U. H. Shah, M. K. Sharif, M. S. Butt and M. Shahid, J. Texture Stud., 2017, 48(3), 221–230, DOI:10.1111/jtxs.12231.
- N. Y. Sinaki and F. Koksel, Int. J. Food Sci. Technol., 2024, 59(4), 2236–2248, DOI:10.1111/ijfs.16943.
- I. Zahari, F. Ferawati, J. K. Purhagen, M. Rayner, C. Ahlström, A. Helstad and K. Östbring, Foods, 2021, 10(10), 2397, DOI:10.3390/foods10102397.
- M. Gupta, A. S. Bawa and A. D. Semwal, Cereal Chem., 2008, 85(2), 115–122, DOI:10.1094/CCHEM-85-2-0115.
- M. A. Brennan, E. Derbyshire, B. K. Tiwari and C. S. Brennan, Plant Foods Hum. Nutr., 2013, 68, 78–82, DOI:10.1007/s11130-012-0330-0.
- E. Hoglund, L. Eliasson, G. Oliveira, V. L. Almli, N. Sozer and M. Alminger, LWT--Food Sci. Technol., 2018, 92, 422–428, DOI:10.1016/j.lwt.2018.02.042.
- D. Gumul, R. Ziobro, H. Gambuś and A. Nowotna, CyTA--J. Food, 2015, 13(3), 353–360, DOI:10.1080/19476337.2014.984336.
- C. Philipp, I. Oey, P. Silcock, S. M. Beck and R. Buckow, J. Food Eng., 2017, 212, 165–173, DOI:10.1016/j.jfoodeng.2017.05.024.
- M. R. Bertolo, L. B. B. de Paiva, V. M. Nascimento, C. A. Gandin, M. O. Neto, C. E. Driemeier and S. C. Rabelo, Ind. Crops Prod., 2019, 140, 111591, DOI:10.1016/j.indcrop.2019.111591.
- L. Dai, Y. Li, F. Kong, K. Liu, C. Si and Y. Ni, ACS Sustainable Chem. Eng., 2019, 7(15), 13497–13504, DOI:10.1021/acssuschemeng.9b02966.
- A. Czaikoski, A. Gomes, K. C. Kaufmann, R. B. Liszbinski, M. B. de Jesus and R. L. da Cunha, Ind. Crops Prod., 2020, 154, 112762, DOI:10.1016/j.indcrop.2020.112762.
|
This journal is © The Royal Society of Chemistry 2025 |
Click here to see how this site uses Cookies. View our privacy policy here.