DOI:
10.1039/D4FO05524A
(Paper)
Food Funct., 2025, Advance Article
Gut microbiota modulation and inflammation mitigation in a murine model through a hull-less and purple grain barley genotype†
Received
11th November 2024
, Accepted 19th February 2025
First published on 20th February 2025
Abstract
Barley, increasingly recognized for its health benefits, contains bioactive compounds like beta-glucans and (poly)phenols. Newly developed purple barley varieties, enriched with anthocyanins, offer potential gut health benefits. This study examined the effects of a hull-less, purple-grain barley genotype, consumed as whole-grain or isolated fractions (bran and endosperm), on gut microbiota and inflammation in a murine model. Fifty male and female BALB/cB&J mice were assigned to five diets over six weeks: standard diet (SD), rice diet (RD), whole-grain barley (WGB), anthocyanin-rich barley bran (BB), and beta-glucan-rich endosperm (PG). The BB diet triggered anti-inflammatory signals as it reduced IFN-γ and IL-4 in females, lowered TNF-α in both sexes, and decreased C-Reactive Protein (CRP) in males compared to SD. The PG diet improved gut barrier integrity by lowering LPS-binding protein levels. Barley-based diets enhanced gut microbiota diversity, particularly, by increasing beneficial bacteria like Lactobacillus, Lachnospiraceae UCG-001, and Akkermansia. Notably, BB and PG elicited stronger effects than WGB, suggesting that grain fractionation modifies the food matrix, potentially enhancing the bioaccessibility and bioavailability of key bioactive compounds. These results underscore the benefits of purple barley-derived fractions in promoting gut health and reducing inflammation, supporting their potential role to protect against inflammation-related conditions.
1. Introduction
Numerous studies highlight the benefits of cereal consumption for health, particularly in the prevention of chronic diseases.1,2 The European Food Safety Authority (EFSA) has recognized the health-promoting properties of oats and barley, particularly due to their content of beta-glucan, a soluble fiber known to improve heart health and aid in the control of blood sugar levels.3 Specifically, the consumption of barley has been linked to a reduced risk of chronic diseases, such as cardiovascular disease, type 2 diabetes, and certain cancers, which can be attributed to its bioactive compounds.4–7 Despite this, barley is not a popular grain in Spain, being primarily used by the brewing industry rather than as a staple food.
Gut health relies on the digestive tract's ability to effectively digest and absorb nutrients, block harmful substances from entering the bloodstream, and maintain a balanced immune system, with the gut microbiota playing a crucial role in supporting these functions.8 Dietary fibers and (poly)phenols, such as those in barley, promote beneficial gut bacteria, which helps reduce inflammation and strengthen gut barrier integrity, highlighting the importance of diet in gut health.9
The presence of beta-glucan in the diet has been shown to promote the growth of beneficial bacteria, which can enhance gut health and boost the immune system.10 The beta-glucan in barley passes through the upper intestinal tract undigested, reaching the distal intestinal tract where it serves as a carbon source for beneficial gut bacteria.11 This interaction between barley and the gut microbiota may play a crucial role in the health benefits associated with barley consumption, including its anti-inflammatory effects.
Cereals displaying black, purple, blue or red pigments are gaining attention due to their rich (poly)phenolic content. Colored grain barley, enriched with anthocyanins (ACN), presents significantly higher levels of antioxidant capacity compared to common barley varieties.12 ACN, in addition to protecting against metabolic risk diseases, can reduce the degree of inflammation.13 Studies in rodents have shown that 88–94% of dietary ACN and other (poly)phenols are recovered in the intestinal tract and feces, where they are biotransformed by the gut microbiota into simpler phenolic compounds. This transformation allows (poly)phenols to exert bioactivity enabling them to remodel the gut microbiota and reduce inflammation.14
Considering the connection between gut microbiota and host inflammation, we hypothesize that barley can reduce inflammation through the gut microbiota regulation. Specifically, we aim to understand how a diet enriched with hull-less and purple grain barley, in its whole-grain form or as isolated bioactive-rich fractions, affects these parameters. Using BALB/cB&J mice we seek to uncover the effects of barley-based diets on gut microbiota composition and inflammatory processes.
2. Materials and methods
2.1. Plant material
The double haploid barley line 151340, a hull-less and purple-grain barley, was used in this study. This genotype was provided by Semillas Batlle SA (Bell-lloc d'Urgell, Lleida, Spain). Whole-grain barley and its isolated fractions (bran and endosperm) were used to prepare the supplemented diets. Whole-grain barley was pearled using a TM-O5C pearling machine (Stake Corporation, Hiroshima, Japan) at 1060 rpm. During this process, the outer layers of the grain were gradually removed through abrasion, resulting in a weight loss equivalent to 10% of the original grain weight. This removed fraction corresponded to the bran layer, while the remaining inner fraction was the endosperm. Both fractions, along with whole-grain barley, were milled to a particle size smaller than 0.5 mm (Foss Cyclotec 1093TM mill, Foss Iberia, Barcelona, Spain) before inclusion in the diets.
2.2. Preparation of diets
Five diets were used in this study and were prepared as follows. The purified standard diet (SD) (Envigo Teklad Diets; TD.94045; AIN-93G Purified Diet) served as a baseline allowing to measure the effects of the experimental barley-based diets against a basic nutritional standard. Unlike chow diets, which include (poly)phenol-rich plant ingredients, the purified diet was designed to exclude these sources, minimizing (poly)phenol content. The SD included casein as a protein source, L-cystine, corn starch, maltodextrin, soybean oil, sucrose, cellulose, vitamins, choline bitartrate and minerals. The refined rice diet (RD), formulated using refined long-grain white rice (Nomen, Spain), provided a comparison with a cereal lacking barley's bioactive compounds, such as (poly)phenols and beta-glucans. To prepare the experimental barley diets, purified diet pellets and barley fractions were crushed in a mill (MC300132, Moulinex, Alençon, France) until a homogeneous powder was obtained and mixed in different proportions. The whole-grain barley diet (WGB) was formulated with a ratio of 75% purified diet and 25% whole-grain barley, while the pearled barley grain diet (PG) consisted of 80% purified diet and 20% pearled barley grain to match the beta-glucan dosage of the WGB diet. The barley bran diet (BB), on the other hand, comprised 95% purified diet and 5% barley bran to deliver the equivalent ACN dose in WGB but without beta-glucans. The RD diet was obtained by mixing 25% refined white rice and 75% purified diet. Distilled water was added, the mixture was homogenized, and diet pellets were prepared and lyophilized using a Lyobeta 15 TELSTAR Lyophilizer (Terrassa, Spain). The approach followed to formulate the experimental diets was to normalize as much as possible carbohydrates, proteins, and caloric density to SD. Barley-supplemented diets were designed to achieve a supplementation of (poly)phenols and beta-glucans based on the human equivalent dose of 140 mg day−1 of ACN (WGB and BB) and 8 g day−1 of beta-glucans (WGB and PG), according to.15
Beta-glucan and arabinoxylan contents of diets were determined by means of the mixed-linkage beta-glucan assay (K-BGLU) and D-xylose assay (K-XYLOSE) kits from Megazyme (Wicklow, Ireland) (For more details, consult ESI Table 1†). To extract and analyze the (poly)phenolic compounds, the methodology used follows the approach reported by;16 further details are provided in ESI Table 2.† For specific details regarding the energy densities and nutritional compositions of the control and experimental diets, please refer to ESI Table 1.†
2.3. Ethical considerations
The animal procedures were conducted in accordance with the guidelines of the European Communities Directive 2010/63/EU regulating animal research. The protocols were approved by the Animal Ethical Committee of the University of Lleida (CEEA of UdL 03-03/20) and performed under a Generalitat de Catalunya Project License (10038). The study complies with the ARRIVE guidelines developed by the NC3Rs.17
2.4. Animals and experimental procedure
A total of 50 BALB/cB&J mice (25 males and 25 females) at 4 weeks of age were purchased from Charles River Laboratories (Barcelona, Spain). Individuals were acclimated to the animal facility with ad libitum access to tap water and the standard diet and then were fed the experimental diets starting at 6 weeks of age. Animals, with a mean body weight of 22.57 ± 1.25 g in males and 17.90 ± 0.44 g in females, were allocated to the five different groups, each comprising 10 animals, 5 males and 5 females. Experimental diets were offered for a period of 6 weeks. Animals were housed in cages of 5 mice per cage on a 12 h light–12 h dark schedule. Temperature was controlled at a mean of 21 ± 1 °C throughout the experiment, levels of CO2 were controlled as well, and humidity was at 55 ± 10%. Feed intake per cage and the body weight of each mouse were recorded every 3 days. Moreover, fecal samples were collected at defecation time at the beginning (6 weeks old, T0), at the middle (9 weeks old, T1), and at the end of the experiment (12 weeks old, T2).
At the end of the experimental period, mice were sacrificed by an intracardiac perfusion after isoflurane anesthesia (IsoFlo, Veterinarian Esteve, Bologna, Italy). Blood samples were collected in EDTA tubes, and plasma samples were obtained by centrifugation (3000g, 10 min at 4 °C) and stored at −80 °C. After blood collection, mice were perfused with an isotonic solution of sodium chloride (0.9%) to remove the remaining blood in tissues. The descending colon tissue was excised, longitudinally opened, gently cleaned from fecal matter, and preserved in paraffin to perform histological analysis. The brain was stored in 4% paraformaldehyde, cryoprotected with 20% sucrose, and blocks were made with OCT Cryostat Mounting Media to subsequently make sections and perform immunohistochemistry assays. Individual ileum content and fecal samples were collected and stored at −80 °C in order to analyze the microbiota composition. The design of the study is shown in Fig. 1.
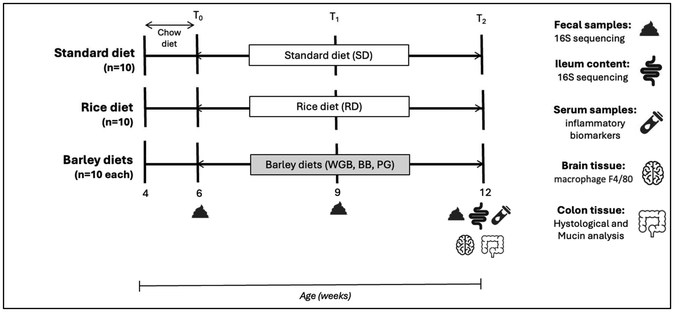 |
| Fig. 1 Experimental design of the study. SD: standard diet, 100% AIN-93G purified diet; RD: rice diet, formulated with 25% rice and 75% purified SD diet; WGB: whole-grain barley diet, formulated with 25% whole-grain barley and 75% purified diet; PG: pearled barley diet, formulated with a ratio of 20% pearled barley grain and 80% purified diet (equivalent beta-glucan dose of the WGB diet); BB: barley bran diet, formulated with 5% barley bran and 95% purified diet (equivalent anthocyanins dose of WGB without beta-glucans). | |
2.5. Inflammatory and immune biomarkers in plasma
C-Reactive Protein (CRP) was analyzed in serum samples using a commercial kit (Mouse CRP ELISA Kit, product number RAB1121, lot Number 0112J0564, Sigma Aldrich). Serum samples were used with a dilution of 2000-fold. The lipopolysaccharide-binding protein (LBP) was analyzed in serum samples using an enzyme-linked immunosorbent assay (ELISA) (Mouse LBP SimpleStep, ab269542, Abcam) with a dilution factor of 3. A MILLIPLEX Mouse High Sensitivity T-cell Magnetic Bead Panel (96-Well Plate Assay, MHSTCMAG-70K, Merck Millipore) was used to assess the levels of serum cytokines including interleukins IL-1α, IL-2, IL-4, IL-6, interferon-gamma (IFN-γ), and tumor necrosis factor-α (TNF-α) after a serum dilution 1
:
1.
2.6. Colon histological and immunohistochemical analysis
Descending colon samples were preserved in formalin between 24 and 48 hours. All of them were placed on “Vogel V0-5-8100” cassettes. The processing of the samples was carried out with a dehydration by immersion in increasing concentrations of alcohol. Then, samples were immersed in liquid paraffin in a “Myr EC 500” embedding apparatus to facilitate sectioning with a rotary microtome (ThermoScientific HM325) calibrated at a thickness of 5 μm. The sections were stained with a hematoxylin–eosin stain and a PAS stain (Periodic Acid-Schiff) and mounted on the coverslip. The histological preparations were studied with an optical microscope (Olympus BX50) equipped with an Olympus SC50 camera interfaced with the Cell Sens Entry® Olympus software.
An immunohistochemical (IHC) analysis of the colon tissue collected from each animal was performed, using the MUC2 monoclonal antibody (Thermofisher Scientific, Waltham, MA, USA). In order to count the percentage of the area occupied by the IHC staining, the ImageJ program was used, by adjusting the threshold color, the saturation to the area of interest and subsequent analysis. Finally, PAS staining was performed using Alzian-blue following the company's protocol.
2.7. Brain F4/80 immunohistochemical analysis
Criostat brain samples were sectioned at the hippocampus region with a criotome (ThermoScientific HM325) calibrated at a thickness of 15 μm. The sections were stained with a hematoxylin–eosin stain and mounted on the coverslip. The histological preparations were studied with an optical microscope (Olympus BX50) equipped with an Olympus SC50 camera interfaced with the Cell Sens Entry® Olympus software. An immunohistochemical analysis of the brain hippocampus tissue collected from each animal was performed, using the R&d system Mouse F4/80/EMR1 monoclonal antibody (Clone 521204, R&D systems, Canada, USA) to label the immunity-related microglia cells. The ImageJ software was used to count the intensity of the colored IHC staining with respect to the total image. The following regions were selected to measure the F4/80 antibody within the hippocampus: DG (dentate gyrus), CA3, and CA1 according to.18
2.8. Statistical analysis
Inflammatory biomarkers, colon and brain immunohistochemical analysis are presented as the mean ± SD or SEM for inflammatory biomarkers in serum. Two-way ANOVA analysis was used for comparison between diets by sex with SPSS 22.0 software. Student's unpaired t-test for comparison of means was used to compare between two groups. The non-parametric Mann–Whitney U-test was used to calculate differences for multiple group comparison. p-Value ≤ 0.05 was considered statistically significant.
2.9. Bacterial DNA extraction and 16S rRNA gene sequencing
Total bacterial DNA was isolated from ileum (N = 50) and fecal (N = 150) samples using the HigherPurity™ Soil DNA Isolation Kit (Canvax Reagents SL, Valladolid, Spain), according to the manufacturer instructions and with the following modifications: frozen wet samples (0.1 g) were disrupted twice in a bead homogenizer (BeadMill 4, ThermoFisher) with two borosilicate 2 mm beads and the kit's lysis buffer, and incubated at 95 °C for five minutes. Then, sample tubes were spun at 10
000g for 30 seconds and the supernatant was transferred to a fresh tube to follow with the manufacturer instructions. In the final step, the DNA was eluted from the column in a volume of 100 μl. DNA concentration and quality were evaluated by spectrophotometry (Nanodrop-100, ThermoFisher) and fluorometry with the dsDNA HS Assay kit (Qubit 4, Invitrogen, Carlsbad, CA). Then, to assess the microbiota composition of mice, the hypervariable region V3–V4 of the 16S rRNA was amplified using primers previously described in19 and sequenced with paired-end reads using the Illumina MiSeq platform (Illumina, San Diego, CA, USA) at the Centre for Genomic Regulation (CRG, Barcelona, Spain). Raw sequence reads were processed into Amplicon Sequence Variants (ASVs) using the Divisive Amplicon Denoising Algorithm 2 (DADA2) pipeline.20 Briefly, raw sequence reads were filtered to remove low-quality bases, primers and adapter sequences were trimmed, and ASVs were created and denoised. Chimeric sequences were identified and removed, taxonomy was assigned using the SILVA database,21 and singletons were filtered out. After data processing, each sample had an average of approximately 61
930 sequencing reads, and the final ASV table contained a total of 12
840 ASVs from 198 samples (ileum (N = 49) and feces (N = 149)).
2.10. Microbial alpha-diversity, beta-diversity and differential abundance analysis
The microbiota composition of ileum and fecal samples were analyzed separately at the genus level. The alpha- and beta-diversities were assessed in order to investigate the effects of (i) diet on the microbiota composition of ileum and fecal samples and (ii) time on the microbiota composition of fecal samples. In addition, differential abundance analyses (DAA) were carried out to detect differentially abundant genera between the different diets. Data were rarefied to an even depth for the alpha- and beta-diversity analyses. The alpha-diversity of each sample was evaluated by the observed and Shannon indices using the phyloseq package in R.22 Analyses of variance were performed for each alpha-diversity index of ileum samples using a linear model including the fixed effects diet (5 levels) and sex (2 levels), as well as the body weight at T0 as a covariate. The effect of time (3 levels) and the interaction between diet and time were added to the model when analysing fecal samples. Statistical analyses were performed using JMP Pro v16 (SAS Institute Inc, Cary, NC, USA). Beta-diversity was assessed by the Bray–Curtis distance using the phyloseq package. Non-metric multidimensional scaling was performed to visualise possible sample clustering. Further, Permutational Multivariate Analysis of Variance (PERMANOVA) based on Bray–Curtis distances was performed for ileum and fecal samples at T2 to assess dissimilarities between the mice microbiota composition using the adonis2 function in R. Finally, DAA were performed to detect differentially abundant genera among diets at the end of the experiment (T2). DAA were carried out using the analysis of compositions of microbiomes with bias correction (ANCOM-BC)23 in R. Five contrasts were investigated: (i) SD vs. barley, where barley refers to WGB, PG and BB, (ii) RD vs. barley, (iii) WGB vs. BB, (iv) WGB vs. PG, and (v) PG vs. BB. To overcome multiple testing, p-values were corrected by a false discovery rate. Genera with a false discovery rate lower than 0.05, and a log fold-change higher than 2 or lower than −2 were deemed statistically significant and, therefore, were further investigated to better understand their associations with the different diets.
3. Results
3.1. Daily dose of (poly)phenolics and dietary fiber administered through diets
The characterization of (poly)phenols and key dietary fiber components, including beta-glucans and arabinoxylans, was performed to assess their potential biological activities based on the product administered. The dose of beta-glucans was similar between WGB and PG diets (1.3 and 1.2 g per 100 g, respectively), while BB contained only 0.1 g per 100 g (ESI Table 1†). Regarding total arabinoxylans content, WGB contained the highest levels (1.6 g per 100 g diet), followed by BB (1.1 g per 100 g diet) and PG (0.8 g per 100 g diet) (ESI Table 1†). The daily dose of total (poly)phenols administered through WGB, BB and PG was 1467.23 ± 15.75, 1098.22 ± 5.53 and 817.83 ± 32.90 μg per day per mouse, respectively (ESI Table 2 and ESI Fig. 1†). The analysis of (poly)phenolic compounds revealed that fiber-bound (poly)phenols represented a significant proportion of the total (poly)phenol content across diets, accounting for 41.9% in WGB, 31.7% in BB, and 54.5% in PG. Among the free (poly)phenols, the most abundant compounds were anthocyanins and flavan-3-ols in the three barley diets. In contrast, the bound (poly)phenolic fraction was primarily composed of phenolic acids (ferulic acid, p-coumaric acid, and their derivatives) in all diets (ESI Table 2†).
As observed, the combined amount of (poly)phenols and arabinoxylans in BB and PG diets were significantly higher compared to WGB. This difference is likely attributed to the structural characteristics of each barley fraction rather than differences in ingredient quantity, as BB and PG together accounted for the same total proportion of barley-derived ingredients as WGB. The mechanical disruption caused by pearling, grinding, and mixing in BB and PG may have enhanced the extractability and accessibility of these compounds. In contrast, the intact grain structure of WGB may have restricted the release of bound (poly)phenols and arabinoxylans.
3.2. Feed consumption and body weight gain
Least square means of body weight gain and feed intake after adjusting for the initial body weight at T0 are given in ESI Table 3.† No significant differences were detected in average body weight gain between diets (p-value of 0.16 for males and 0.25 for females). It is important to note that statistical analyses of individual feed intake were not possible due to the lack of individual data; feed intake measurements were taken on a per-cage basis.
3.3. Serum inflammatory biomarkers
Regarding the inflammatory parameters, when the results were compared considering both males and females together, no significant differences were observed between the barley-based diets and the control diets (SD and RD), except for TNF-α and LBP, which presented a significant reduction after BB and PG supplementation, respectively (ESI Table 4†). When analyzing data by sex (Fig. 2), females supplemented with the BB diet showed lower levels of IFN-δ (p-value = 0.027) and IL-4 (p-value = 0.047) compared to females fed with SD. Similarly, both males and females fed with the BB diet had lower levels of TNF-α (p-value = 0.016 for females, p-value = 0.05 for males) compared to the SD group (Fig. 2A, C and F). Additionally, males consuming BB exhibited a significant decrease in plasmatic CRP levels (p-value = 0.009) compared to SD (Fig. 2B) and females fed with BB exhibited significant decrease in IL-4 levels (p-value = 0.016) compared to the rice diet (RD) (Fig. 2C). Moreover, a significant decrease in LBP was observed in the PG group versus SD in both males (p-value = 0.009) and females (p-value = 0.009) (Fig. 2F).
 |
| Fig. 2 Mouse serum protein levels of inflammatory biomarkers. The bars representing males are shown with a dotted pattern, while the bars for females are displayed with a solid fill. (A) Interferon-gamma (IFN-γ), (B) C-reactive protein (CRP), (C) interleukine-4 (IL-4), (D) tumor necrosis factor-alfa (TNF-α), (E) interleukine-2 (IL-2), (F) lipopolysaccharide binding protein (LBP); SD: standard diet, RD: rice diet, WGB: whole-grain barley, PG: pearled grain, BB: barley bran; the results are shown as the mean ± SEM. Significant differences (p-value < 0.05) are expressed as * compared with SD; and ^ compared with RD. | |
3.4. Colon histological and MUC2 immunohistochemical analysis
Regarding mucins in gut slides, no significant differences were observed between diets (ESI Fig. 2 and 3†). The mucin analysis focused on the expression and distribution of MUC2, a major component of the gut mucus layer. Results indicated that MUC2 distribution in the gut epithelium remained consistent across all dietary groups, showing that the consumption of different barley fractions did not alter mucin production or secretion in the colon.
3.5. Immunohistochemistry for F4/80 in brain
Fig. 3 presents the immunohistochemical analysis of F4/80 expression across different hippocampal regions in mice subjected to the experimental diets. The expression of F4/80 was found significantly lower in all the hippocampal regions (DG, CA3, and CA1) of females from the BB group compared to the SD group. Similarly, the expression of F4/80 was significantly lower in females from the WGB group compared to SD. In contrast, no significant differences were detected between mice fed with RD and PG with those fed with SD.
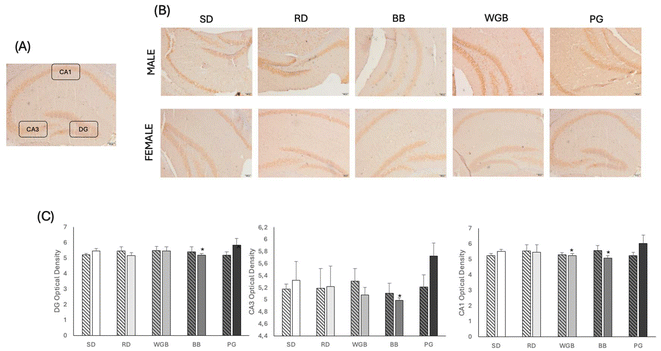 |
| Fig. 3 Immunohistochemical analysis of F4/80 expression (microglial activation) across different brain hippocampal regions—dentate gyrus (DG), CA3 and CA1—in mice subjected to SD: standard diet, RD: rice diet, WGB: whole-grain barley, PG: pearled grain, BB: barley bran. (A) Selected brain hippocampal regions; (B) staining intensity of F4/80 across different dietary groups; (C) quantification of the optical density of F4/80 staining (4×). Significant differences with respect to the SD group* (p-value < 0.05). | |
3.6. Microbial diversity and taxonomy in ileum and feces
The overall microbiota composition of ileum content and fecal samples is displayed at the phylum level in ESI Fig. 4.† In ileum, Firmicutes was the most abundant phylum with an average relative abundance of 89.7%, followed by Actinobacteriota (4.5%) and Proteobacteria (3.9%). However, in fecal samples, Firmicutes and Bacteroidetes were the highly enriched phyla, accounting for 63.0% and 28.4% of the relative abundance, respectively.
3.6.1. Ileum content. The gut microbial alpha- (observed and Shannon indices) and beta- (Bray–Curtis distance) diversities of ileum were assessed at the genus level. Analyses of variance for alpha-diversity indices of rarefied data showed no significant differences among the different diets in ileum (observed: p-value = 0.40 and Shannon: p-value = 0.92). However, permutational multivariate analysis of variance (PERMANOVA) based on Bray–Curtis distances indicated that ileum samples from different diets were significantly different at the end of the experiment (T2) (p-value ≤ 0.001). The differential abundance analysis of ileum microbiota composition identified a total of 19 differentially abundant genera across the five analyzed contrasts (Fig. 4). We observed significant modulation in the number of genera across different dietary contrasts as follows: 6 genera in SD vs. barley, 3 genera in RD vs. barley, 8 genera in WGB vs. PG, 11 genera in WGB vs. BB, and 6 genera in PG vs. BB.
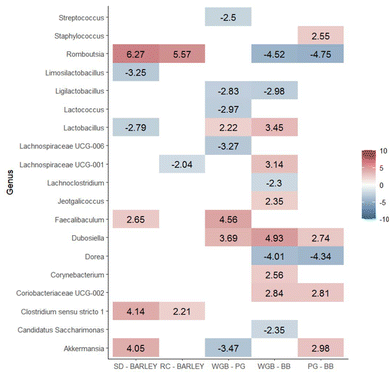 |
| Fig. 4 Differentially abundant genera in ileum for the analyzed diet contrasts. Values represent the log fold change of the differentially abundant genera with a false-discovery rate below 0.05 and a |log(fold-change)| ≥ 2. SD: standard diet, RD: rice diet, WGB: whole-grain barley, PG: pearled grain, BB: barley bran. | |
3.6.2. Fecal content. Regarding fecal samples, analyses of variance for alpha-diversity indices of rarefied data showed significant differences among the different time points (observed: p-value ≤ 0.0001 and Shannon: p-value ≤ 0.0001). The alpha-diversity of fecal samples increased with time, with T2 (12 weeks of age) showing the highest diversity (Fig. 5). Diet, on its own, did not have a significant effect on alpha-diversity (observed: p-value = 0.40, Shannon: p-value = 0.23). However, the interaction between diet and time was significant for the observed (p-value ≤ 0.01) and Shannon (p-value ≤ 0.05) indices, indicating that the effect of time on microbial diversity depends on the diet consumed. For the observed alpha-diversity index (Fig. 5A), no significant differences were detected among time for mice consuming the SD diet. In contrast, significant time-related changes were observed in mice fed barley-based diets (BB, PG, WGB), indicating that these diets promoted increasing microbial diversity over time. Regarding the Shannon index (Fig. 5B), no significant differences were found among time for the SD and WGB diets. However, significant differences among time were detected for mice fed with RD, BB and PG diets. These findings suggest that the type of diet modulates the effects of time on the microbiota composition of mice and that barley-based diets modulate microbiota diversity over time, while the SD does not. In addition, PERMANOVA based on Bray–Curtis distances further strengthens these findings, by demonstrating that the overall composition of microbial communities in fecal samples was significantly different between diets at the end of the experiment (T2) (p-value ≤ 0.001).
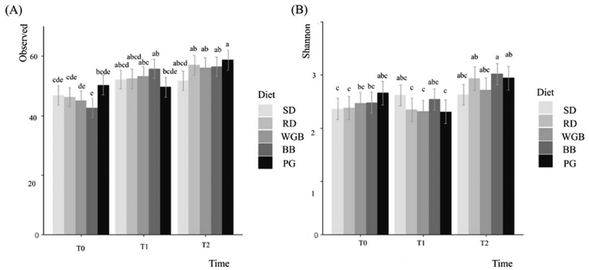 |
| Fig. 5 Diversity microbiota analyses of fecal samples. Least mean squares of alpha-diversity indices (A. observed; B. Shannon) of fecal samples presented by time (T0: 6 weeks of age, T1: 9 weeks of age, and T2: 12 weeks of age) and by diet. SD: standard diet, RD: rice diet, WGB: whole-grain barley, PG: pearled grain, BB: barley bran. Error bars represent standard errors. Columns lacking a common letter differ (p-value ≤ 0.05). | |
The microbial taxa at phylum level was illustrated among diets in feces at different time points (ESI Fig. 5†). An increase in Firmicutes and a decrease in Bacteroidota were observed as the animals aged. These changes in microbial populations may reflect age-related adaptations in gut function and nutrient utilization. Additionally, barley-enriched diets promoted greater diversity in minor phyla, such as Proteobacteria, Verrucomicrobiota and Actinobacteriota, compared to control diets.
Moreover, there were differentially abundant genera among diets in fecal samples collected at the end of the study (T2) (Fig. 6). A total of 39 differentially abundant genera were identified across the five analyzed contrasts. We observed a significant modulation in the number of genera across different dietary contrasts as follows: 13 genera in SD vs. barley, 14 genera in RD vs. barley, 12 genera in WGB vs. PG, 18 genera in WGB vs. BB, and 23 genera in PG vs. BB.
 |
| Fig. 6 Summary of the differentially abundant genera in feces for the analyzed diet contrasts. Values represent the log fold change of the differentially abundant genera with a false-discovery rate below 0.05 and a |log(fold-change)| ≥ 2. SD: standard diet, RD: rice diet, WGB: whole-grain barley, PG: pearled grain, BB: barley bran. | |
4. Discussion
In the present study, we investigated the effects of the diet supplementation with whole-grain purple barley and its isolated fractions on inflammation, as well as their impact on gut microbiota modulation. The comparison of different barley interventions revealed distinctive health benefits and microbial signatures associated with the isolated barley fractions, particularly the bran and endosperm, as compared to the control diet and the whole-grain barley.
In terms of inflammation, our study revealed that BB led to a significant reduction in pro-inflammatory cytokines, which was not observed with the other diets. Specifically, cytokine analysis showed a reduction in IFN-γ, IL-4, TNF-α, and CRP levels in the BB group compared to SD. These findings underscore the distinctive anti-inflammatory potential of the isolated barley bran fraction. This effect was initially attributed to the combined influence of arabinoxylans and anthocyanins, both present in the bran and recognized for their potential anti-inflammatory properties.24,25 However, given that the PG diet contained similar arabinoxylan levels and WGB had even higher amounts of arabinoxylans and (poly)phenols, additional factors must be contributing to the observed effects. Structural differences between dietary sources likely influence the bioaccessibility of their bioactive compounds. Previous studies have demonstrated that food matrix composition and processing techniques such as milling and fractionation play a crucial role in fiber functionality, as structural modifications can facilitate the release and absorption of bioactive compounds.26,27 Additionally, although WGB had higher total (poly)phenol content than BB, differences in matrix interactions likely influenced their release, absorption, and biological activity. Thus, (poly)phenols in BB may have been more accessible, enhancing systemic effects. This aligns with studies showing that food matrix composition and processing impact (poly)phenol absorption,28,29 with BB's simpler structure facilitating uptake, while WGB's complexity may reduce bioavailability and anti-inflammatory effects.
The potential effects on neuroinflammation were also observed, as the BB diet appeared to be associated with a reduction in microglial activation in key brain regions compared to SD, particularly in the DG, CA3, and CA1. The improvements were only observed in females, which aligns closely with the cytokine results. Given the pivotal role of microglia in neuroinflammatory processes, these findings suggest that the anti-inflammatory properties of the BB diet could extend to neuroprotective effects. However, while our results are in line with previous research,30 indicating that ACN from purple barley could influence microglial activity and modulate neuroinflammatory markers, the characterization of microglial activation in this study was based only on the analysis of F4/80 expression. F4/80 is widely recognized as specific markers of murine macrophages and microglia.31,32 Nevertheless, additional microglial markers, such as IBA-1 or CD40, would be necessary to confirm and better characterize this effect.
The sex-specific differences observed in inflammatory markers can be attributed to hormonal influences, as supported by previous research.33,34 Estrogen and testosterone play distinct roles in modulating immune responses, with estrogen generally amplifying immune activity, while testosterone has more immunosuppressive effects. As a result, females tend to exhibit a stronger inflammatory response compared to males, which is consistent with the patterns observed in this study.
The observed decrease in LBP levels in mice consuming the PG highlights its potential role in modulating gut health. LBP is a biomarker linked to gut barrier integrity and immune response to microbial products, particularly lipopolysaccharides from Gram-negative bacteria. Elevated LBP levels indicate an increased gut permeability and microbial translocation, leading to systemic inflammation and immune activation.35 Therefore, the reduction in LBP levels suggests an improvement in gut barrier function when consuming the pearled barley grain. The potential mechanism underlying the modulation of LBP levels by PG may involve its beta-glucan content, as they have been shown to enhance gut barrier function strengthening the intestinal epithelial barrier and reducing gut permeability.11,36
Regarding the microbiota results, microbiota richness showed that barley-based diets (WGB, PG, BB) increased gut microbiota diversity over time. The SD remained stable, while RD unexpectedly increased diversity. This effect may be related to the presence of digestible and resistant starches in rice, which, despite its refined nature, can serve as substrates for microbial fermentation, as previously reported in the literature.37 However, given that microbiota changes in the RD group were less pronounced than in barley-based diets, this also suggests a partial influence of age-related adaptations rather than diet alone. The increase in diversity observed with barley-based diets can be attributed to the presence of fiber and bioactive compounds, such as beta-glucans, arabinoxylans, phenolic acids, and ACN. These compounds likely play a crucial role in fostering a more diverse microbial ecosystem by providing fermentable substrates. This is consistent with previous findings,38 which demonstrated that barley food products can influence the composition of gut microbiota, associated in some cases with metabolic improvements.
In addition to increasing overall microbiota diversity, purple barley-based diets were found to cause specific changes in the composition of microbial populations. The differential abundance analysis of ileum and fecal microbiota revealed significant differences when comparing the standard or rice diets against all barley-based diets. Notably, Lactobacillus was predominant in both the ileum and feces of barley diets compared to the SD group, while Lachnospiraceae UCG-001 was predominant in both the ileum and feces compared to both the SD and rice diets. In feces, in addition to Lactobacillus, other beneficial probiotic genera such as Dubosiella were found to be more abundant after supplementation with barley diets. These findings are consistent with previous studies showing that barley consumption increases beneficial bacteria.10 These results are in line with other studies performed with dietary fiber and cereal beta-glucans.39,40 Lactobacillus is well-known for its beneficial effects on gut health, including enhancement of the gut barrier, modulation of the immune system, and production of lactic acid which inhibits the growth of pathogenic bacteria.41 Furthermore, the higher abundance of Lachnospiraceae UCG-001 in feces after supplementation with barley is noteworthy, as this genus has been implicated in butyrate production.42
Moreover, the microbiota analysis revealed that both the BB and PG diets led to distinct changes in gut microbial composition. BB diet specifically increased the abundance of Romboutsia and Dorea in both ileum content and feces. These genera are associated with fiber degradation and butyrate production.43,44 These results are consistent with findings from previous studies, such as those involving black barley,45 which also demonstrated similar microbial shifts. Also, the higher abundance of Ruminococcus observed in response to BB supplementation is particularly noteworthy as it is strongly correlated with the most important butyrate producers in the gut.46 Butyrate, a key SCFA, is crucial for maintaining gut health and is known to inhibit pro-inflammatory cytokine production and promote anti-inflammatory responses, which aligns with the observed reductions in serum markers like TNF-α, CRP, IFN-γ, and IL-4 in the BB group. The modulation of the gut microbiota by BB could be partly attributed to the ACN present in purple barley bran, which are known for their potential prebiotic effects.47 Although ACN content was the same in BB and WGB, differences in the structural properties and matrix interactions of these fractions may have influenced ACN and other (poly)phenols bioavailability, leading to a greater impact on microbiota modulation in the BB diet. Specifically, Dorea has been implicated in ACN metabolism,48 further suggesting that the observed microbial shifts in the BB group could be linked to the (poly)phenolic content of the diet. Unfortunately, the analysis of microbiota composition for each sex has not been possible due to the limited sample size, preventing drawing reliable conclusions regarding potential sex-specific microbial responses.
The differential abundance analysis of gut microbiota also revealed a specific microbial signature after PG supplementation. For instance, the genera Akkermansia was markedly enriched in PG compared to WGB and BB in both ileum and feces (Fig. 4 and 5). The enrichment of Akkermansia is particularly notable, as this genus has been associated with strong probiotic effects, including metabolic regulation, immune modulation and gut barrier protection,49,50 which suggests potential benefits of PG consumption. This specific effect of PG may be attributed to the pearling-removing the outer bran layer and exposing the inner endosperm- which could increase the accessibility of beta-glucans and potentially making them more available for fermentation by gut microbiota.51 Also, the unique microbial profile of the PG diet may be associated with the significant reduction observed in LBP levels after supplementation with the PG diet. There is strong evidence supporting that Akkermansia may reduce intestinal permeability and prevent the translocation of harmful bacterial components like LPS into the bloodstream, reducing the subsequent LBP production.52 These results highlight the potential of pearled barley to modulate gut inflammation through beneficial alterations in the gut microbiota. Muribaculum, a major mucin monosaccharide forager, which plays a key role in maintaining intestinal homeostasis,53 was also markedly enriched following PG diet. Specifically, beta-glucans were reported to promote this homeostasis by increasing the abundance of Muribaculum.54 Further analysis of the whole metagenome is needed to investigate differences in microbial functions.
The non-significant findings regarding MUC2 in the gut imply that the dietary intake of barley, whether whole-grain, bran, or pearled grain, does not compromise the structural integrity of the ileum or alter the mucin profile in the gut. The consistent MUC2 expression across different barley diets suggests that whole barley and its isolated fractions do not compromise gut mucosal health. This aligns with existing research highlighting the critical role of stable mucin production, particularly MUC2, in protecting the gut's epithelial barrier and maintaining overall gut health.55
5. Concluding remarks
This study emphasizes the significant health benefits of purple barley-based diets, particularly barley bran and pearled grain, in modulating inflammation and gut microbiota. The anti-inflammatory effects observed only with the barley bran diet, are likely influenced not only by its concentration of arabinoxylans and anthocyanins but also by differences in food matrix interactions and processing, which may have enhanced the bioaccessibility of its bioactive compounds.
All barley diets enhanced gut microbiota diversity, increasing beneficial bacteria like Lactobacillus, Lachnospiraceae UCG-001, and Akkermansia, which are associated with butyrate production and gut health. The pearled barley diet significantly reduced LBP levels, suggesting improved gut barrier integrity and reduced systemic inflammation. This effect may be linked to the promotion of beneficial bacteria, such as Akkermansia, which help reduce gut permeability. The pearling process enhances the accessibility of beta-glucans in the nutrient-rich endosperm, making them more available for fermentation by gut microbiota.
The whole-grain barley diet was beneficial but less effective in modulating inflammatory markers, possibly due to its intact structure, which may limit the bioavailability of (poly)phenols and fibers. Despite containing higher total levels of (poly)phenols and arabinoxylans, processing differences influenced their release, absorption, and activity. These findings highlight the potential of barley fractions, like pearled grain and isolated barley bran, in improving gut health and protecting against inflammation-related conditions. Future research should further explore the mechanisms underlying these effects, including the role of processing in the bioavailability of (poly)phenols and fiber, and evaluate their long-term health implications through clinical trials, considering factors such as age, sex, and metabolic status.
Data availability
The dataset generated and analyzed during the current study is available in the CSUC Dataverse repository. A private URL has been provided for peer review: https://dataverse.csuc.cat/privateurl.xhtml?token=4df3db08-f154-45d3-9a0c-ab2f967eb3f8.
Upon publication, the dataset will be publicly available at https://doi.org/10.34810/data1828.
Conflicts of interest
On behalf of all authors, the corresponding author states that there is no conflict of interest.
Acknowledgements
This study was supported by the Spanish Ministry of Industry, Economy and Competitiveness, the Agencia Estatal de Investigación (AEI) and the European Regional Development Fund (ERDF) through the PID2020-113009RB-100 INNOBAR project and by the Generalitat de Catalunya through the Maria Engracia Cortijo predoctoral FI SDUR grant. We would like to acknowledge Dr María-Paz Romero for her valuable contribution and supervision throughout this work.
References
- W. Wu, M. Sol, F. Chen, E. Cao, H. Liu and Y. Zhao, et al., Microbiota metabolite short-chain fatty acid acetate promotes intestinal IgA response to microbiota which is mediated by GPR43, Mucosal Immunol., 2017, 10(4), 946–956, DOI:10.1038/mi.2016.114.
- A. Nakashima, K. Yamada, O. Iwata, R. Sugimoto, K. Atsuji and T. Ogawa, et al., β-Glucan in Foods and Its Physiological Functions, J. Nutr. Sci. Vitaminol., 2018, 64(1), 8–17, DOI:10.3177/jnsv.64.8.
- E. De Marco Castro, P. C. Calder and H. M. Roche, β–1,3/1,6−Glucans and Immunity: State of the Art and Future Directions, Mol. Nutr. Food Res., 2021, 65(1), e1901071, DOI:10.1002/mnfr.201901071.
- J. Li, T. Kaneko, L.-Q. Qin, J. Wang and Y. Wang, Effects of barley intake on glucose tolerance, lipid metabolism, and bowel function in women, Nutrition, 2003, 19(11–12), 926–929, DOI:10.1016/S0899-9007(03)00182-5.
- B. Delaney, R. J. Nicolosi, T. A. Wilson, T. Carlson, S. Frazer and G.-H. Zheng, et al., β-Glucan Fractions from Barley and Oats Are Similarly Antiatherogenic in Hypercholesterolemic Syrian Golden Hamsters, J. Nutr., 2003, 133(2), 468–475, DOI:10.1093/jn/133.2.468.
- K. M. Behall, D. J. Scholfield and J. Hallfrisch, Diets containing barley significantly reduce lipids in mildly hypercholesterolemic men and women, Am. J. Clin. Nutr., 2004, 80(5), 1185–1193, DOI:10.1093/ajcn/80.5.1185.
- J. M. Keenan, M. Goulson, T. Shamliyan, N. Knutson, L. Kolberg and L. Curry, The effects of concentrated barley β-glucan on blood lipids in a population of hypercholesterolaemic men and women, Br. J. Nutr., 2007, 97(6), 1162–1168, DOI:10.1017/S0007114507682968.
- F. Di Vincenzo, A. Del Gaudio, V. Petito, L. R. Lopetuso and F. Scaldaferri, Gut microbiota, intestinal permeability, and systemic inflammation: a narrative review, Intern. Emerg. Med., 2024, 19(2), 275–293, DOI:10.1007/s11739-023-03374-w.
- A. M. Valdes, J. Walter, E. Segal and T. D. Spector, Role of the gut microbiota in nutrition and health, Br. Med. J., 2018, k2179, DOI:10.1136/bmj.k2179.
- M. Jayachandran, J. Chen, S. S. M. Chung and B. Xu, A critical review on the impacts of β-glucans on gut microbiota and human health, J. Nutr. Biochem., 2018, 61, 101–110, DOI:10.1016/j.jnutbio.2018.06.010.
- R. P. Singh and A. Bhardwaj, β-glucans: a potential source for maintaining gut microbiota and the immune system, Front. Nutr., 2023, 10, 1143682, DOI:10.3389/fnut.2023.1143682.
- M. Martínez-Subirà, M. P. Romero, E. Puig, A. Macià, I. Romagosa and M. Moralejo, Purple, high β-glucan, hulless barley as valuable ingredient for functional food, LWT – Food Sci. Technol., 2020, 131, 109582, DOI:10.1016/j.lwt.2020.109582.
- J. Liu, H. Zhou, L. Song, Z. Yang, M. Qiu and J. Wang, et al., Anthocyanins: Promising Natural Products with Diverse Pharmacological Activities, Molecules, 2021, 26(13), 3807, DOI:10.3390/molecules26133807.
- C. Cueva, I. Gil-Sánchez, M. V. Moreno-Arribas and B. Bartolomé, Interactions Between Wine Polyphenols and Gut Microbiota, in Wine Safety, Consumer Preference, and Human Health, Springer International Publishing, Cham, 2016, pp. 259–278. DOI:10.1007/978-3-319-24514-0_13.
- S. Reagan-Shaw, M. Nihal and N. Ahmad, Dose translation from animal to human studies revisited, FASEB J., 2008, 22, 659–661 CrossRef CAS PubMed.
- M. E. Cortijo Alfonso, S. Yuste, I. Friero, M. Martínez-Subirà, M. Moralejo and C. Piñol-Felis, et al., Metabolic profiling of (poly)phenolic compounds in mouse urine following consumption of hull-less and purple-grain barley, Food Funct., 2024, 15(16), 8300–8309, 10.1039/D4FO01275E.
- N. Percie du Sert, A. Ahluwalia, S. Alam, M. T. Avey, M. Baker and W. J. Brown, et al., Reporting animal research: Explanation and elaboration for the ARRIVE guidelines 2.0, PLos Biol., 202014, 18(7), e3000411 CrossRef CAS PubMed.
- B. Almolda, C. de Labra, I. Barrera, A. Gruart, J. M. Delgado-Garcia and N. Villacampa, et al., Alterations in microglial phenotype and hippocampal neuronal function in transgenic mice with astrocyte-targeted production of interleukin-10, Brain, Behav., Immun., 2015, 45, 80–97, DOI:10.1016/j.bbi.2014.10.015.
- J. R. Willis, P. González-Torres, A. A. Pittis, L. A. Bejarano, L. Cozzuto and N. Andreu-Somavilla, et al., Citizen science charts two major ‘stomatotypes’ in the oral microbiome of adolescents and reveals links with habits and drinking water composition, Microbiome, 2018, 6(1), 218, DOI:10.1186/s40168-018-0592-3.
- B. J. Callahan, P. J. McMurdie, M. J. Rosen, A. W. Han, A. J. A. Johnson and S. P. Holmes, DADA2: High-resolution sample inference from Illumina amplicon data, Nat. Methods, 2016, 13(7), 581–583, DOI:10.1038/nmeth.3869.
- C. Quast, E. Pruesse, P. Yilmaz, J. Gerken, T. Schweer and P. Yarza, et al., The SILVA ribosomal RNA gene database project: improved data processing and web-based tools, Nucleic Acids Res., 2012, 41(D1), D590–D596, DOI:10.1093/nar/gks1219.
- P. J. McMurdie and S. Holmes, phyloseq: An R Package for Reproducible Interactive Analysis and Graphics of Microbiome Census Data, PLoS One, 2013, 8(4), e61217, DOI:10.1371/journal.pone.0061217.
- H. Lin and S. Das Peddada, Analysis of compositions of microbiomes with bias correction, Nat. Commun., 2020, 11(1), 3514, DOI:10.1038/s41467-020-17041-7.
- J. Khan, P. Gul, M. T. Rashid, Q. Li and K. Liu, Composition of Whole Grain Dietary Fiber and Phenolics and Their Impact on Markers of Inflammation, Nutrients, 2024, 16(7), 1047, DOI:10.3390/nu16071047.
- A. Francavilla and I. J. Joye, Anthocyanins in Whole Grain Cereals and Their Potential Effect on Health, Nutrients, 2020, 12(10), 2922, DOI:10.3390/nu12102922.
- M. S. Izydorczyka, T. McMillana, S. Bazina, J. Kletkea, L. Dushnickya and J. Dexter, et al., Milling of Canadian oats and barley for functional food ingredients: Oat bran and barley fibre-rich fractions, Can. J. Plant Sci., 2024, 94(3), 573–586, DOI:10.4141/cjps2013-229.
- O. Acar, et al., A research on milling fractions of biofortified and nonbiofortified hull–less oats in terms of minerals, arabinoxylans, and other chemical properties, Cereal Chem., 2023, 100(5), 1192–1202, DOI:10.1002/cche.10702.
- L. Arfaoui, Dietary plant polyphenols: Effects of food processing on their content and bioavailability, Molecules, 2021, 26(10), 2959, DOI:10.3390/molecules26102959.
- I. O. Minatel, C. V. Borges, M. I. Ferreira, H. A. Gomez Gomez, C.-Y. O. Chen and G. P. Pereira Lima, Phenolic compounds: Functional properties, impact of processing and bioavailability, Phenolic Compd.:Biol. Act., 2017, 8, 1–24, DOI:10.5772/66368.
- C. A. Zaa, Á.J Marcelo, Z. An, J. L. Medina-Franco and M. A. Velasco-Velázquez, Anthocyanins: Molecular Aspects on Their Neuroprotective Activity, Biomolecules, 2023, 13(11), 1598, DOI:10.3390/biom13111598.
- A. M. Jurga, M. Paleczna and K. Z. Kuter, Overview of General and Discriminating Markers of Differential Microglia Phenotypes, Front. Cell. Neurosci., 2020, 14, 198, DOI:10.3389/fncel.2020.00198.
- H.-H. Lin, D. E. Faunce, M. Stacey, A. Terajewicz, T. Nakamura and J. Zhang-Hoover, et al., The macrophage F4/80 receptor is required for the induction of antigen-specific efferent regulatory T cells in peripheral tolerance, J. Exp. Med., 2005, 201(10), 1615–1625, DOI:10.1084/jem.20042307.
- V. R. Moulton, Sex Hormones in Acquired Immunity and Autoimmune Disease, Front. Immunol., 2018, 4(9), 2279, DOI:10.3389/fimmu.2018.02279.
- F. Mauvais-Jarvis, Sex differences in metabolic homeostasis, diabetes, and obesity, Biol. Sex Differ., 2015, 6(1), 14, DOI:10.1186/s13293-015-0033-y.
- N. Perez-Diaz-del-Campo, G. Castelnuovo, D. G. Ribaldone and G. P. Caviglia, Fecal and Circulating Biomarkers for the Non-Invasive Assessment of Intestinal Permeability, Diagnostics, 2023, 13(11), 1976, DOI:10.3390/diagnostics13111976.
- J. Luo, D. Chen, X. Mao, J. He, B. Yu and L. Cheng, et al., Purified β-glucans of Different Molecular Weights Enhance Growth Performance of LPS-challenged Piglets via Improved Gut Barrier Function and Microbiota, Animals, 2019, 9(9), 602, DOI:10.3390/ani9090602.
- Z. Hitache, S. Al-Dalali, H. Pei and X. Cao, Review of the Health Benefits of Cereals and Pseudocereals on Human Gut Microbiota, Food Bioprocess Technol., 2023, 16(11), 2382–2399, DOI:10.1007/s11947-023-03069-2.
- M. E. Cortijo-Alfonso, M. P. Romero, A. Macià, S. Yuste, M. Moralejo and L. Rubió-Piqué, et al., Effect of Barley and Oat Consumption on Immune System, Inflammation and Gut Microbiota: A Systematic Review of Randomized Controlled Trials, Curr. Nutr. Rep., 2024, 13(3), 582–597, DOI:10.1007/s13668-024-00543-x.
- Q. Nie, Y. Sun, M. Li, S. Zuo, C. Chen and Q. Lin, et al., Targeted modification of gut microbiota and related metabolites via dietary fiber, Carbohydr. Polym., 2023, 316, 120986, DOI:10.1016/j.carbpol.2023.120986.
- G. A. Fabiano, L. M. Shinn and A. E. C. Antunes, Relationship between Oat Consumption, Gut Microbiota Modulation, and Short-Chain Fatty Acid Synthesis: An Integrative Review, Nutrients, 2023, 15(16), 3534, DOI:10.3390/nu15163534.
- R. D. Ayivi, R. Gyawali, A. Krastanov, S. O. Aljaloud, M. Worku and R. Tahergorabi, et al., Lactic Acid Bacteria: Food Safety and Human Health Applications, Dairy, 2020, 1(3), 202–232, DOI:10.3390/dairy1030015.
- M. Vital, A. C. Howe and J. M. Tiedje, Revealing the Bacterial Butyrate Synthesis Pathways by Analyzing (Meta)genomic Data, mBio, 2014, 5(2), e00889, DOI:10.1128/mBio.00889-14.
- J. Gerritsen, B. Hornung, B. Renckens, S. A. F. T. van Hijum, V. A. P. Martins Dos Santos and G. T. Rijkers, et al., Genomic and functional analysis of Romboutsia ilealis CRIB T reveals adaptation to the small intestine, PeerJ, 2017, 5, e3698, DOI:10.7717/peerj.3698.
- M. Rajilić-Stojanović and W. M. de Vos, The first 1000 cultured species of the human gastrointestinal microbiota, FEMS Microbiol. Rev., 2014, 38(5), 996–1047, DOI:10.1111/1574-6976.12075.
- L. Zhong, L. Qin, X. Ding, L. Ma, Y. Wang and M. Liu, et al., The regulatory effect of fermented black barley on the gut microbiota and metabolic dysbiosis in mice exposed to cigarette smoke, Food Res. Int., 2022, 157, 111465, DOI:10.1016/j.foodres.2022.111465.
- J. Teichmann and D. W. Cockburn, In vitro Fermentation Reveals Changes in Butyrate Production Dependent on Resistant Starch Source and Microbiome Composition, Front. Microbiol., 2021, 29(12), 640253, DOI:10.3389/fmicb.2021.640253.
- T. A. Verediano, H. Stampini Duarte Martino, M. C. Dias Paes and E. Tako, Effects of Anthocyanin on Intestinal Health: A Systematic Review, Nutrients, 2021, 13(4), 1331, DOI:10.3390/nu13041331.
- L. Bresciani, D. Angelino, E. I. Vivas, R. L. Kerby, C. García-Viguera and D. Del Rio, et al., Differential Catabolism of an Anthocyanin-Rich Elderberry
Extract by Three Gut Microbiota Bacterial Species, J. Agric. Food Chem., 2020, 68(7), 1837–1843, DOI:10.1021/acs.jafc.9b00247.
- Q. Zhai, S. Feng, N. Arjan and W. Chen, A next generation probiotic, Akkermansia muciniphila, Crit. Rev. Food Sci. Nutr., 2019, 59(19), 3227–3236, DOI:10.1080/10408398.2018.1517725.
- A. Everard, C. Belzer, L. Geurts, J. P. Ouwerkerk, C. Druart and L. B. Bindels, et al., Cross-talk between Akkermansia muciniphila and intestinal epithelium controls diet-induced obesity, Proc. Natl. Acad. Sci. U. S. A., 2013, 110(22), 9066–9071, DOI:10.1073/pnas.1219451110.
- J. Slavin, Fiber and Prebiotics: Mechanisms and Health Benefits, Nutrients, 2013, 5(4), 1417–1435, DOI:10.3390/nu5041417.
- P. E. Gámez-Macías, E. Félix-Soriano, M. Samblas, N. Sáinz, M. J. Moreno-Aliaga and P. González-Muniesa, Intestinal Permeability, Gut Inflammation, and Gut Immune System Response Are Linked to Aging-Related Changes in Gut Microbiota Composition: A Study in Female Mice, J. Gerontol., Ser. A, 2024, 79(4), glae045, DOI:10.1093/gerona/glae045.
- N. Liu, S. Zou, C. Xie, Y. Meng and X. Xu, Effect of the β-glucan from Lentinus edodes on colitis-associated colorectal cancer and gut microbiota, Carbohydr. Polym., 2023, 316, 121069, DOI:10.1016/j.carbpol.2023.121069.
- M. Sung, Y. Yoon and J. Lee, The Immunomodulatory Effect of β-Glucan Depends on the Composition of the Gut Microbiota, Foods, 2023, 12(17), 3148, DOI:10.3390/foods12173148.
- P. Louis and H. J. Flint, Formation of propionate and butyrate by the human colonic microbiota, Environ. Microbiol., 2017, 19(1), 29–41, DOI:10.1111/1462-2920.13589.
|
This journal is © The Royal Society of Chemistry 2025 |
Click here to see how this site uses Cookies. View our privacy policy here.