DOI:
10.1039/D4GC05662K
(Critical Review)
Green Chem., 2025,
27, 1278-1299
Deep eutectic solvent-mediated hydrothermal treatment for biomass conversion: a review
Received
6th November 2024
, Accepted 18th December 2024
First published on 26th December 2024
Abstract
Hydrothermal treatment is an emerging technology for the processing of biomass into various value-added products. Deep eutectic solvents (DESs) emerge as green solvents for biomass processing. Recently, DES-mediated hydrothermal treatment (DES-HTT) has emerged as a cutting-edge technique for promoting biomass conversion, merging the principles of HTT with the unique properties of DESs. To keep up the pace of rapid development of DESs and HTT, it is necessary to summarize the progress of this green technology. Herein this review summarizes the progress of the use of DES-HTT in biomass conversion, including DES-mediated hydrothermal pretreatment for dewatering, bioconversion and/or fractionation of biomass, DES-mediated hydrothermal extraction of lipid from biomass, and DES-mediated hydrothermal carbonization for functional hydrochar and carbon dot synthesis. This review also analyzes the future challenges and research directions in biomass conversion via DES-HTT. Finally, this review facilitates the valorization of biomass via the combination of advantages from DESs and HTT.
Green foundation
1. Deep eutectic solvent-mediated hydrothermal treatment emerges as a cutting-edge technology for promoting biomass conversion, merging the principles of hydrothermal treatment with the unique properties of deep eutectic solvents. Here, we discuss the progress and challenges of this green technology for biomass conversion
2. This technology is highly efficient in enhancing the bioconversion of biomass, facilitating the fractionation of biomass components, promoting lipid extraction from biomass, and synthesizing functional biocarbon and carbon dots, which favors the high-value conversion of biomass
3. However, this technology still suffers from several challenges. For example, the molecular mechanisms mediated by the green solvent should be elucidated, the reusability and treatment of the solvent after its use should be addressed, and the economic viability and environmental impacts should be assessed
|
1. Introduction
In response to the escalating demand for renewable energy and resources and the imperative for environmental conservation, the innocuous management of biomass waste and efficient transformation of biomass resources have emerged as pivotal research areas.1 Among various biomass conversion/disposal routes, hydrothermal treatment (HTT), recognized for its advanced thermochemical conversion capabilities, is adept at transforming biomass feedstocks into a spectrum of value-added products. This technology leverages the thermochemical transformation of organic matter through reactions such as hydrolysis, dehydration, condensation, and decarboxylation, resulting in the phase change of biomass components and the production of solid, liquid, and gaseous products. This technology is particularly adept at processing biomass with high moisture content, circumventing the need for such energy-intensive drying processes, including but not limited to household food waste,2 kitchen residues,3,4 sewage sludge (SS),5 and animal manure.6,7 HTT of biomass encompasses hydrothermal pretreatment (HTPT) for enhancing further conversion of biomass, hydrothermal extraction (HTE) of chemicals (e.g., nutrients, aromatics, and fatty acids) from biomass, hydrothermal carbonization (HTC), hydrothermal liquefaction (HTL), and hydrothermal gasification (HTG).8
The promotion of these HTT processes is contingent upon the precise control of variables such as temperature, time, pressure, catalyst, and solution properties (e.g., pH and co-existing organics).9 Recently, the addition of deep eutectic solvents (DESs) in the hydrothermal reaction medium has been developed as an emerging strategy to enhance the efficacy of HTT for biomass conversion.10–12 For example, Xu et al. used a DES composed of urea and ZnCl2 during hydrothermal carbonization to enhance the physicochemical properties of hydrochar from SS,13 and then Lai et al. directly prepared a biocarbon coenriched with carboxyl and phenol groups via the HTC in a DES composed of choline chloride (ChCl) and citric acid (CA).10
DESs represent a class of innovative solvents synthesized via blending hydrogen bond donors (HBDs) with hydrogen bond acceptors (HBAs) in stoichiometrically balanced ratios, which are characterized by their low melting points, high solubility, and tunable properties.14,15 As an emerging solvent platform, DESs offer the benefits of ease of preparation, affordability, biodegradability, and commendable selectivity and stability, positioning them as a preferred alternative to traditional ionic liquids (ILs) for a broad spectrum of applications such as natural product extraction, chemical catalysis, organic synthesis, pharmaceutical dissolution, biotransformation processes, and carbon sequestration.16,17 In comparison with conventional ILs, DESs exhibit analogous physicochemical attributes, yet with the added benefits of reduced cost and enhanced environmental compatibility. These compelling attributes have garnered increasing attention for DESs across various research domains.18 The integration of DESs with alternative pretreatment techniques, such as microwave irradiation, ultrasonication, or the application of alkali and surfactants, presents a more economically viable, environmentally benign, and technologically efficient approach for overcoming the challenges associated with the sole use of DESs for biomass pretreatment.19 This synergistic application of DESs with complementary methods holds promise for advancing the efficiency and sustainability of biomolecule extraction and biomass conversion processes.
DES-mediated HTT (DES-HTT) emerges as a cutting-edge technique for biomass conversion, merging the principles of HTT with the unique properties of DESs to partially dismantle the complex biomass matrix and release its chemical constituents.20 This strategy distinguishes itself from traditional HTT by significantly improving biomass conversion rates and product selectivity using a DES, an environmentally friendly solvent that accelerates reaction kinetics, broadens the range of applicable reactions, and fine-tunes reaction conditions. The ability of a DES to enhance product selectivity by reducing by-product formation while promoting green chemistry principles, such as renewability and biodegradability, positions it as a sustainable alternative to conventional organic solvents. This innovative approach not only elevates the efficiency and selectivity of biomass conversion processes but also advances the chemical and biochemical industries towards greater sustainability and environmental responsibility.
With the fast development of human society, the requirement for bioenergy and biomaterials will quickly increase under the “carbon neutralization” policy. To keep up the pace of rapid development of DESs and HTT, it is necessary to summarize the progress of this green technology. Hence, this review summarizes the progress of the use of DES-HTT in biomass conversion, including DES-mediated hydrothermal pretreatment for dewatering, bioconversion and/or fractionation of biomass, DES-mediated hydrothermal extraction of lipid from biomass, and DES-mediated HTC for functional hydrochar and carbon nanodot (CD) synthesis. This review also analyzes the future challenges and research directions in biomass conversion via DES-HTT. Finally, the results from this review facilitate the valorization of biomass via the combination of advantages from DESs and HTT.
2. DESs for HTT of biomass
2.1 Types of DESs used for DES-HTT
Within the realm of green chemistry and sustainable chemical processes, conventional organic solvents have progressively fallen short of meeting the stringent demands of contemporary industries, primarily due to their propensity for environmental contamination, high toxicity, and lack of renewability.21,22 Prior to the advent of DESs, ILs emerged as a promising alternative to traditional solvents, owing to their distinctive physicochemical attributes, such as tunable viscosity, density, hydrophilicity, and solubility, which are achievable through diverse combinations of cations and anions. Nevertheless, ILs exhibit limitations in practical applications, including complex synthesis, high cost, and challenges associated with recycling and reuse. As such, there is an urgent need to identify a green, cost-effective, and efficient alternative solvent that can overcome the constraints of current application areas.
DESs are characterized as low-melting eutectic mixtures that are synthesized through the combination of HBAs and HBDs in defined molar proportions.23,24 Smith et al. categorized DESs into five distinct types, each distinguished by the unique combinations and properties of HBAs and HBDs (Fig. 1): Type I, which comprises quaternary ammonium salts in conjunction with anhydrous metal halides; Type II, featuring quaternary ammonium salts with crystalline water-containing metal halides; Type III, consisting of quaternary ammonium salts mixed with amides, carboxylic acids, and alcohols; Type IV, involving metal halides complexed with organic ligands; and Type V, which includes natural amino acids combined with natural phytates.25,26 Type I DESs predominantly consist of metal halide/imidazolium salt systems, such as chloroaluminate/imidazolium salt melts and ILs derived from imidazolium salts combined with various metal halides, e.g., FeCl3. In contrast, Type II DESs are based on hydrated metal halides and are well-suited for large-scale industrial applications due to their cost-effectiveness and resistance to air and moisture. Type III DESs, particularly those formed from mixtures of ChCl with HBDs, have garnered significant interest for their capacity to solvate a broad spectrum of transition metal substances, including chlorides and oxides. The exploration of diverse HBDs, including amides, carboxylic acids, and alcohols, has been a focus of research efforts. These DESs exhibit ease of preparation, non-reactivity with water, and are predominantly biodegradable, with a relatively low cost of production. They exhibit a diverse array of hydrogen bonding donors that can be readily tailored to meet specific application requirements. Their applications extend to the de-glycerolization of biodiesel,17,27 the treatment of metal oxides,28,29 and the synthesis of cellulose derivatives.30,31 Type IV DESs are formed through the combination of metal halides with urea, exhibiting melting points below 150 °C, which indicates their capacity to incorporate a broad spectrum of transition metals into the DES framework. Type V DESs, while less frequently employed, have been investigated, including combinations such as betaine-CA.
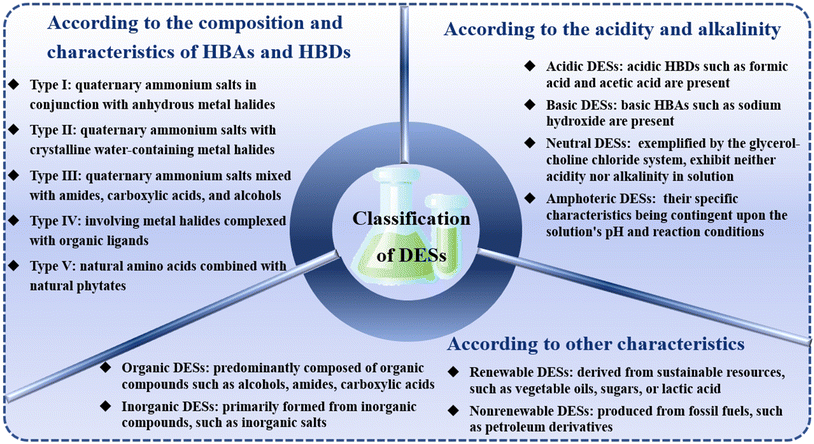 |
| Fig. 1 Types of DESs used for DES-HTT. | |
DESs can be further classified into acidic, basic, neutral, and amphoteric categories based on their acidity or alkalinity profiles (Fig. 1). Acidic DESs are characterized by the presence of acidic hydrogen bond donors, such as formic acid (FA) and acetic acid (AA), whereas basic DESs contain basic hydrogen bond acceptors, such as sodium hydroxide. Neutral DESs, exemplified by the urea-ChCl system, exhibit neither acidity nor alkalinity in solution. Amphoteric DESs, such as CA, are capable of displaying both acidic and basic properties, with their specific characteristics being contingent upon the solution's pH and reaction conditions.32 The acid–base properties of DESs are pivotal for their utility in catalysis, extraction, and synthesis, as they can engage in acid–base reactions with reactants or products, thereby influencing the efficiency and selectivity of chemical reactions. Furthermore, DESs can be broadly categorized into organic and inorganic groups based on their composition (Fig. 1). Organic DESs are predominantly composed of organic compounds, including alcohols, amides, carboxylic acids, and esters, such as ethanol, urea, FA, and ethyl acetate. In contrast, inorganic DESs are primarily formed from inorganic compounds, such as inorganic salts, alkali–metal hydroxides, and alkaline-earth metal hydroxides, such as sodium chloride, sodium hydroxide, and calcium hydroxide. In terms of raw material sourcing and regeneration potential, DESs can be further differentiated into renewable and non-renewable types. Renewable DESs are derived from sustainable resources, such as vegetable oils, sugars, or lactic acid (LA), which can be replenished through agricultural or biotechnological means and are amenable to physical or chemical regeneration post-use, thereby minimizing environmental impact. Conversely, non-renewable DESs are produced from fossil fuels, such as petroleum derivatives, and are dependent on finite resources that are challenging to recycle, potentially exerting greater environmental pressure, which is at odds with the principles of sustainable development.
2.2 Definition of DES-HTT
The DES-HTT process ingeniously combines the respective advantages of HTT and DESs to form an efficient integrated treatment methodology. The primary objective of this technology is to partially decompose the complex structure of biomass waste through the synergistic action of a high-temperature, high-pressure hydrothermal environment and the specific chemical properties of DES. This combined approach effectively liberates the abundant chemical components contained within biomass waste. This process not only significantly enhances the resource utilization efficiency of biomass waste but also greatly facilitates subsequent chemical or biochemical transformation steps. Recently, increasing attention has been paid to the application of DESs to enhance HTT, i.e., DES-mediated HTT (DES-HTT). Specifically, DESs have shown their abilities to enhance various HTT processes, including hydrothermal pretreatment, hydrothermal extraction, and HTC.13,33 Thus, herein, the DES-HTT is defined as the HTT process with the presence of DESs for enhancing biomass conversion into value-added products towards diverse applications (Fig. 2). It is worth noting that under high operation temperature, DES components could undergo dehydration, decomposition, and/or other thermochemical conversion, resulting in the loss of DES properties. Thus, DESs are not applicable for high temperature conditions.
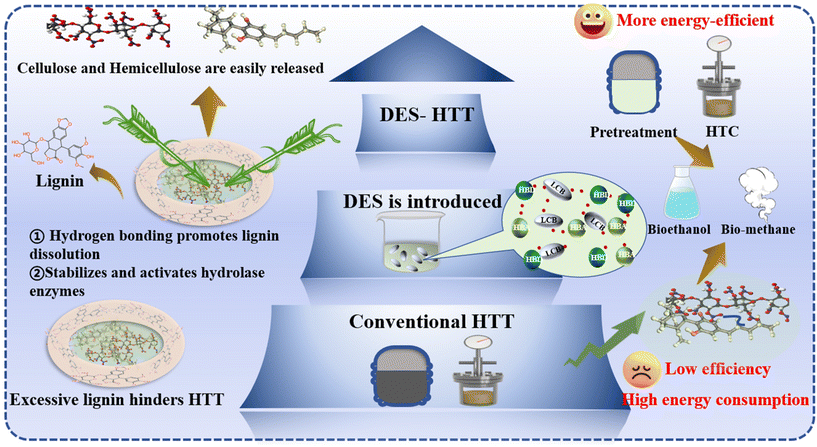 |
| Fig. 2 Definition of DES-HTT. | |
2.3 Potential roles of DESs in HTT processes
Fig. 3 shows the potential roles of DESs in HTT processes. The roles of DESs in the HTT process are characterized by their multifaceted and intricate nature, encompassing various levels of interactions and effects. Firstly, DESs demonstrate an exceptional capacity to dissolve and disperse previously insoluble components, particularly lignin and hemicellulose, during biomass processing. These components typically envelop cellulose and hemicellulose, creating a protective laminar structure that impedes the efficient conversion of primary biomass components in hydrothermal treatments. The unique molecular structure and polar groups of DESs enable the disruption of this protective layer, thereby mitigating the protective effect of lignin on cellulose and hemicellulose and significantly enhancing the accessibility of these components.34 Secondly, DESs are capable of significantly modifying the physical structure of the substrate. For instance, DESs can increase the porosity of biomass materials or disrupt the crystalline regions of cellulose, rendering the biomass structure more open and loose. This structural alteration facilitates the penetration of water molecules and reaction reagents, thereby promoting the efficient conversion and reaction of cellulosic biomass during hydrothermal treatment. Thirdly, at the chemical reaction level, the catalytic activity of DESs plays a pivotal role. DESs can accelerate chemical reactions such as hydrolysis, oxidation, and reduction and significantly enhance the reaction rate by reducing the activation energy of the reaction.35 The interaction of DESs with water can lead to the hydrolysis of polar groups or functional groups within the DES molecules, potentially resulting in the decomposition of DESs into smaller molecules or ions, which may exhibit higher reactivity and positively influence the hydrothermal treatment process. The hydrogen-bonding acceptors and donors within DESs are also integral to their mechanism of action. Hydrogen-bonding donors in DESs can form new hydrogen bonds with hydrogen bond acceptors in the reactants, thereby disrupting the internal structure of these biopolymers and the hydrogen bonding network between the reactants. This disruption enhances the interactions between reactant or product molecules.36 This interaction not only aids in increasing the solubility of the reactants but also fosters hydrothermal reaction.
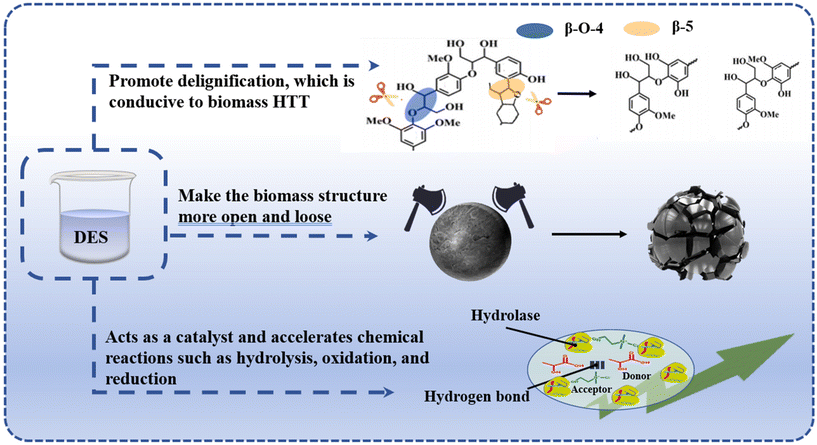 |
| Fig. 3 Potential roles of DESs in HTT processes. | |
3. DES-mediated hydrothermal pretreatment
3.1 Enhancing dewatering of SS
The SS is a dominant biomass waste around the world, which poses potentially hazardous substances, including organic pollutants, heavy metals, and pathogens.37 The latest statistical data indicates that China generates an average annual output exceeding 13.3 million tons of SS on a dry solid basis.38 The dewatering of SS serves as a pivotal process, not only for its effectiveness in volume reduction but also for its role in facilitating subsequent treatment procedures and cost minimization, which is essential for the operational efficiency of wastewater treatment plants. SS is rich in a multitude of hydrophilic functional groups, including hydroxyl, carboxyl, and carbonyl groups, which engage in hydrogen bonding formation with water molecules, thereby facilitating the formation of a hydration layer on the surface of SS particles.39,40 This hydration layer augments the surface energy of the SS particles, impeding the detachment of water molecules from the SS particle surfaces and complicating the dewatering process, which in turn results in elevated energy consumption.41
To effectively diminish the moisture of SS, it is imperative to develop dewatering technologies capable of severing these hydrogen bonds and eliminating adsorbed water. Based on excellent dissolving capacity, DESs exhibit a multifaceted influence on the dewatering process of SS. Initially, DESs can notably diminish the surface's hydrophilicity of SS by engaging with hydrophilic functional groups, such as hydroxyl and carboxyl groups, thereby curtailing the water molecule adsorption capacity on the surface of SS. This interaction mechanism is instrumental in reducing the aggregation of water molecules within the SS, enhancing dewatering efficiency. Liu et al. demonstrated that the presence of DESs promotes sludge dewatering at elevated temperatures, with DESs playing a critical role in sludge dewatering during thermal treatment.42 Liu et al. detailed a methodology that combines DESs (composed of betaine and oxalic acid dihydrate) with hydrothermal pretreatment to ameliorate the dewaterability of SS, substantiating that DES-mediated hydrothermal pretreatment can degrade the surface functional groups of SS and augment its hydrophobicity.43 They observed that a significant improvement in sludge dewaterability only occurred when hydrothermal treatment was coupled with DES, while it did not occur when hydrothermal treatment and blending DESs with sludge were conducted alone. Specifically, the capillary suction time (CST) was reduced from 115.5 to 21.4 s after the DES-HT, while slightly increased to 141.1 and 130.9 s after the DES and hydrothermal treatment, respectively (Fig. 4). The density functional theory (DFT) calculation further suggested that the DES weakened the H-bond strength between extracellular polymeric substance (EPS) components and enhanced the dissolution of proteins and polysaccharides in SS during the DES-HT.
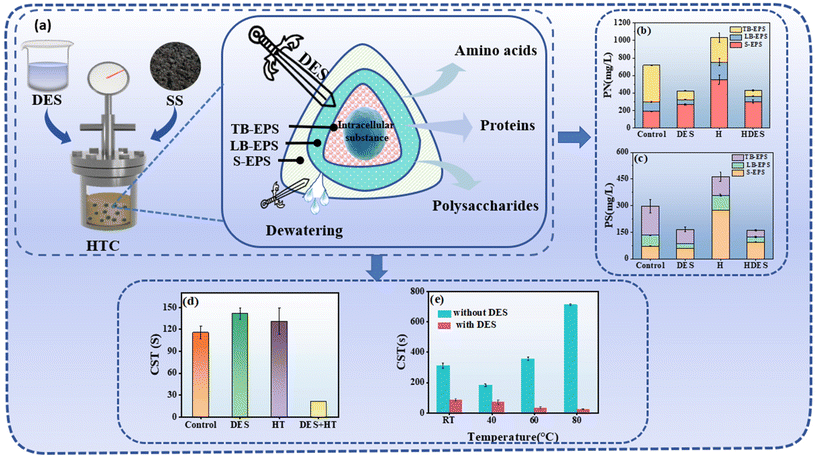 |
| Fig. 4 Enhancing dewatering of SS via DES-mediated hydrothermal pretreatment. (a) Schematic illustration of SS subjected to DES-mediated hydrothermal pretreatment. (b) Content of proteins in different EPS layers.43 (c) Content of polysaccharides in different EPS layers. (Note: control, sludge only stirred at RT without any other treatments; DES, sludge conditioned with 2.5 ‰ DES; H, heat treatment of 60 °C; and HDES, heat treatment of 60 °C with 2.5 ‰ DES).43 (d) The effects of DES dosages (magnetically stirred at 300 rpm for 30 min, 10 ± 2 °C, intrinsic pH).42 (e) Reaction temperatures on sludge dewatering (RT – magnetically stirred at 300 rpm for 30 min; other temperatures – water bath at 120 rpm for 30 min, intrinsic pH, 2.5 ‰ DES).43 | |
From the above results, the DES-HTT method provides an effective method to remove water from high moisture organic matter. As research into DESs deepens, it is anticipated that this technology will assume an increasingly pivotal role in the realm of SS treatment and resource recovery.
3.2 Enhancing the bioconversion of lignocellulosic biomass
3.2.1 Enzymatic saccharification.
Lignocellulose is a rich and cheap renewable resource, mainly including cellulose, hemicellulose and lignin.52 Both cellulose and hemicellulose are composed of monomeric sugars that can be converted into fermentable sugars by pretreatment and enzymatic hydrolysis. Enzymatic saccharification is a biochemical process involving the use of enzymes to break down polysaccharides (e.g., starch, cellulose, or pectin) into simpler sugars, usually monosaccharides (e.g., glucose), and this process has a wide range of applications in the food industry, biofuel production, and textile processing.
DESs have garnered considerable interest in their roles in enhancing enzymatic saccharification (Table 1), attributed to their distinctive chemical attributes and commendable environmental compatibility. The multifaceted potential of DESs in optimizing the enzymatic process is evident in several key areas. Firstly, DESs have the capacity to significantly modify the physical structure of substrates. This is achieved by increasing the substrate's porosity or by disrupting the crystalline regions within cellulose, thereby rendering the substrate more accessible and susceptible to enzymatic contact and degradation. Shen et al. demonstrated that after pretreatment of Eucalyptus in ChCl-LA DES at 110 °C, enzymatic glycolysis yielded a remarkable glucose conversion rate of 94.3%, surpassing that of untreated biomass by a substantial 9.8-fold increase.44 Secondly, DESs exhibit exceptional solubility, effectively dissolving cellulose and other polysaccharides. This solubility facilitates the enzymatic digestion process by making the substrate more amenable to enzymatic action. For instance, the pretreatment of waste rice husk with a DES composed of AA and cetyltrimethylammonium bromide at 140–180 °C successfully removed hemicellulose and xylan, resulting in a 372.8% enhancement in enzymatic saccharification activity.45 The results showed that the DES-mediated hydrothermal pretreatment process resulted in increased accessibility of rice husk, increased porosity, and decreased surface area and hydrophobicity of lignin compared to the original feedstock. Moreover, DESs exhibit the unique ability to modulate the pH within the solvent, which is crucial for sustaining enzymatic activity within an optimal range. A stable pH environment is essential for maintaining enzyme functionality, thereby significantly augmenting the efficiency and yield of the enzymatic process. In summary, the integration of DESs in hydrothermal pretreatment offers a promising path for improving the conversion of lignocellulosic biomass into fermentable sugars, with implications for the advancement of sustainable biofuel and biochemical production processes.
Table 1 The summary of DES-mediated hydrothermal pretreatment for enhancing the bioconversion of biomass
Bioconversion process |
Feedstock |
DES |
Hydrothermal conditions |
Performance |
Ref. |
Enzymatic saccharification |
Eucalyptus camaldulensis
|
ChCl + LA |
110 °C, 6 h |
Glucose yield reached 94.3%, a 9.8-fold increase vs. untreated biomass. |
44
|
Rice hull |
CTAB + AA |
180 °C, 1 h |
Enzymatic saccharification enhanced 3.7× over raw biomass, with effective lignin and xylan removal. |
45
|
Green bamboo |
ChCl + LA |
180 °C, 35 min |
88.6% hemicellulose removed, yielding 50.9% XOS with 81.6% purity. |
46
|
AD |
Poplar stems |
ChCl + EG + 4-CSA |
170 °C, 40 min |
Max biomethane yield of 208 L kg−1 VS, 148% boost vs. untreated poplar |
47
|
Rice straw |
Betaine + LA |
130 °C, 3 h |
Resulting in a 33.4% higher methane yield of 467.84 mL g−1 VSinitial than in the ADS. |
48
|
Bioethanol fermentation |
Paulownia wood |
ChCl + LA |
130 °C, 1 h |
Removed 94% lignin, retained 93% glucan in solid, spent solid saccharified/fermented to 43.61 g of ethanol per L (89.7% yield). |
49
|
Banana peel waste |
ChCl + urea |
120 °C, 1 h |
The maximum yield of TRS reached 73.97%, the catalytic hydrolysis products were fermented to produce bioethanol, with a fermentation efficiency of 80.42%. |
50
|
Poplar wood |
Glycerin + ChCl + H2SO4 |
140 °C, 2 h |
Hemicellulose removal: 98%; enzymatic hydrolysis rate: >90%, obtaining a high concentration of glucose (137.4 g L−1) for ethanol fermentation |
51
|
3.2.2 Bio-methane production.
Due to the recalcitrance of the lignocellulosic biomass, the conversion of lignocellulose via anaerobic digestion (AD) faces huge challenges. The ability of DESs to enhance methane production from sludge has been well established. Xie et al. reported that ChCl-urea and ChCl-EG boosted cumulative methane production by 3.9 times and 5.4 times, respectively, demonstrating that DES pretreatment markedly improves the methane generation potential of sludge during anaerobic AD.53 DES-mediated hydrothermal pretreatment has been applied to enhance the biomethanation of lignocellulosic biomass (Table 1 and Fig. 5). It has been demonstrated that DESs combined with the hydrothermal process can efficiently extract lignin from lignocellulose. Basak et al. found that the hydrothermal process solubilized 96% of hemicellulose, and the subsequent DES treatment at 130 °C for 3 h removed 81.3% of lignin, leaving a pulp with 72% w/w of highly bio-accessible cellulose, resulting in a high methane yield of 467.84 mL g−1.48 Under the same hydrothermal conditions, the addition of a DES promoted lignin solubilization and increased methane production by 33.4%. Kang et al. found that hydrothermal pretreatment coupled with the DES (ChCl and LA) at 160 °C can achieve a removal rate of 80% of lignin, and the biomethane production can improve 36.3% compared to without treatment.54 Elmaci et al. found that when cork dust is used as a feedstock, after hydrothermal pretreatment with a DES composed of ChCl and FA at 130 °C for 60 min, the yield of bio-methane (115.1 ± 5.3 mL g−1 VS) in comparison with the samples without DES treatment, there was a 125% increase.55 However, Xie et al. found that the high lignin removal rate of the pretreated poplar (treated by the DES composed of ChCl, EG and 4-chlorobenzenesulfonic acid at 100 and 110 °C) led to a rapid accumulation of volatile organic acids during the hydrolysis and acidogenesis stages, resulting in methanogenesis inhibition and biomethane yield reduction.47 Thus, delignification is not a direct guarantee for highly efficient bio-methane production, and AD condition is also a key factor controlling the biomethane production from the pretreated biomass. In addition, due to the complexity of lignocellulose and DES, selecting a highly effective DES system is a key step to DES coupling with hydrothermal pretreatment to improve the bio-methane yield.
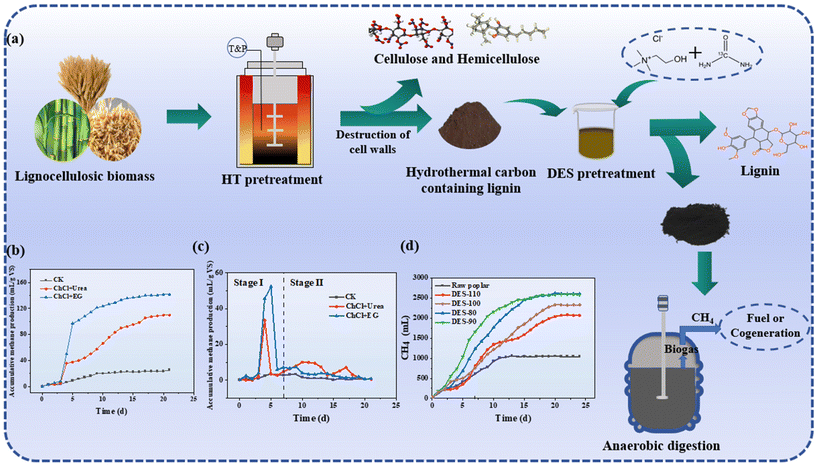 |
| Fig. 5 DES-mediated hydrothermal pretreatment for enhancing bio-methane production. (a) Process flow diagram for coupling DES with hydrothermal treatment to produce biomethane. (b) Daily methane production.53 (c) The methane fraction of biogas.53 (d) CH4 yields of the solid products after two-step pretreatment under different conditions.47 | |
3.2.3 Bioethanol production.
As an important biofuel, bioethanol has attracted much attention around the world, with an expected production amount of 140 billion liters in 2022.56 During bioethanol production, breaking down the recalcitrant structure of lignocellulose is the key step to improve enzymatic saccharification of cellulose and hemicellulose. Hydrothermal pretreatment has been extensively studied due to its environmentally friendly advantages in using water as a medium.57 Recently, DES-mediated hydrothermal pretreatment has also attracted much attention due to its high efficiency in removing lignin,58 which would benefit bioethanol production (Table 1 and Fig. 5). Despite the advantages of hydrothermal pretreatment for lignocellulosic processing, some remaining challenges still need to be overcome, such as relatively high operation temperature and high sugar concentration able to produce an ethanol titer of ≥4%.57 As an industrial application, how to save energy and improve the product yield are key issues. Recently, DES-mediated hydrothermal pretreatment produces synergistic effects and better dissolution of cellulose and hemicellulose in lignocellulose.59,60 The hydrothermal pretreatment selectively removes the hemicellulose portion, whereas the DES efficiently extracts out lignin to expose amorphous cellulose from biomass.61 Overall, combining DESs and hydrothermal pretreatment provides an excellent eco-friendly strategy for the comprehensive utilization of lignocellulose.
Recently, Pérez-Pérez et al. found that about 76% of hemicelluloses were recovered in oligomeric form, delignification was about 86% and the bio-ethanol yield reached 83% in the solid phase.62 Rodríguez-Rebelo reported that hydrothermal pretreatment with DESs at 130 °C for 60 min enabled the removal of 94% of lignin and recovery of 93% glucan from Paulownia wood, resulting in an 89.7% bioethanol yield.49 Guo et al. found that 89.5% of lignin was removed by silicotungstic acid (heteropoly acid) catalyzed DES (ChCl and glycerol) pretreatment due to the proton from heteropoly acids significantly contributing to lignin degradation, and the ethanol yield was significantly increased from 9.9% to 81.8% in the semi-simultaneous saccharification and fermentation process.63 Manivannan et al. reported that hydrothermal pretreatment with a DES at 120 °C for 1 h to remove lignin mainly assists in the hydrolysis process to increase the cellulose surface area and porosity, which can enhance the possibility of contact with the cellulose via hydrogen bonding during magnetic carbon acid catalytic process, leading to a bioethanol yield of 59.2%.50 The basic reason for selecting acidic catalyst assessment is that based on the significant effect of hydrogen-bond acidity on the cleavage of the lignin-carbohydrate complex,64 an acidic DES system was selected to remove lignin and promote the cleavage of β-O-4′ linkages to deconstruct the recalcitrance of bamboo.65 He et al. found that after DES pretreatment with H2SO4 at 140 °C, the hemicellulose removal can reach 98% and the enzymatic hydrolysis rate of pretreated solids exceeds 90%, which can be beneficial for the bioethanol product (57.7 g L−1).51
In summary, compared to conventional hydrothermal pretreatment, the main principle by which the addition of DESs to hydrothermal pretreatment significantly increases the ethanol yield is that DESs can effectively dissolve the recalcitrant components such as lignin and hemicellulose in biomass, breaking through their protective layers. This makes cellulose and hemicellulose more accessible to hydrothermal pretreatment, significantly enhancing their conversion efficiency. Additionally, DESs stabilize and activate hydrolytic enzymes, ensuring their activity under extreme conditions such as elevated temperature and pressure, thereby increasing the efficiency of enzymatic hydrolysis and boosting the production of fermentable sugars. This leads to more efficient ethanol production. This integrated green pretreatment technology offers an excellent and eco-friendly strategy for the comprehensive utilization of biomass in the current bio-refinery process.
3.3 Fractionation of lignocellulosic biomass
3.3.1 Fabrication of lignin-containing nanocellulose fibrils (LCNFs) and cellulose nanofibrils (CNFs).
Lignocellulosic biomass is increasingly recognized for its potential as a sustainable alternative to conventional energy and materials.66 The extraction of CNFs from this biomass has emerged as a key area of interest within various industrial sectors, including food, biomedicine, agriculture, and electronics, due to their exceptional advantages such as renewability, tunable surface chemistry, biocompatibility, biodegradability, and a high specific surface area.67 CNFs are typically produced via a top-down mechanical process that involves the deconstruction of cellulose fibers within the lignocellulosic matrix. This process necessitates a series of intricate refining pretreatments to remove non-cellulosic components, such as lignin, hemicellulose, and pectin, through methods including steaming, alkali treatment, and bleaching.68 Despite the advancements, the delignification process remains costly, time-intensive, and environmentally impactful, characterized by the extensive use of chemicals, energy, and labor.69 Recent studies have highlighted that lignin, traditionally viewed as a hindrance to cellulose fiber decomposition, when integrated into nanofibrillated cellulose, confers enhanced properties to the resulting LCNFs. These include improved hydrophobicity, thermal stability, UV-blocking capabilities, and antioxidant activity compared to conventional CNFs.70,71 Moreover, LCNFs can be directly isolated from unprocessed lignocellulosic feedstocks, such as unrefined wood pulp and cost-effective agricultural residues, offering a more sustainable and economically viable alternative to CNFs, with the added benefit of higher yields and reduced environmental footprint.72 The direct extraction of LCNFs from lignocellulosic biomass is thus posited as an eco-friendly, streamlined, and efficacious strategy. However, prevalent methods for LCNF preparation are often energy-intensive, involve complex procedures, and require specialized equipment.73
The synergistic integration of DESs with complementary pretreatment technologies, such as ultrasonication, microwave irradiation, hydrothermal treatment, or surfactant-assisted processes, opens novel avenues for addressing the economic, technological, and environmental challenges inherent in lignocellulosic biorefinery operations.74 This multi-technology integration strategy is instrumental in realizing the more effective high-value utilization of biomass, thereby fostering the sustainable progression of biomass-based energy and material industries.
Studies have demonstrated that the synergistic application of microwave irradiation with DESs can augment the polarity of the constituent molecules, potentially facilitating a reduction in the temperature and duration necessary for the pretreatment process without compromising its effectiveness.60 Hui et al. reported DES-mediated hydrothermal pretreatment under microwave irradiation as a robust nanofabrication strategy for the synthesis of LCNFs.75 Their findings indicate that under optimized DES pretreatment conditions (120 °C for 20 min), the delignification rate achieved a remarkable 92.10%, while the cellulose retention rate was impressively high at 92.95%. Based on this, the amalgamation of hydrothermal and DES technologies emerges as a promising biorefinery approach for the efficacious disentanglement of cellulose, hemicellulose, and lignin from lignocellulosic feedstocks, thereby optimizing product yields.61 Hydrothermal processing not only augments hydrolysis efficiency but also releases cellulose protofibers through the disruption of cell wall architecture and partial lignin removal. It is worth noting that the efficacy of standalone hydrothermal treatment in lignin extraction is constrained.76,77
In the context of DES-mediated hydrothermal pretreatment, the synergistic interplay between hydrothermal activity and DESs facilitates a more efficacious dissolution of biomass constituents (Table 2 and Fig. 6). The hydrothermal pretreatment paves the way for DESs to extract lignin and unveil amorphous cellulose by selectively eliminating hemicellulose.59 Within an optimal temperature spectrum, the rate of lignin removal escalates with the elevation of the temperature of hydrothermal treatment. Nevertheless, this increase is not indefinite. The plausible rationale is that at elevated temperatures, the lignin removal efficacy is capped by the thermal stability of DESs, which is intricately linked to intermolecular forces, including hydrogen bonding. Hydrogen bonding, being a tenuous intermolecular force, is susceptible to dissociation upon reaching certain temperature thresholds. Furthermore, the onset of DES component decomposition at higher temperatures initiates the weakening of hydrogen bonding within DESs, culminating in diminished lignin removal capacity.78 This hypothesis was corroborated by Chen et al., who observed an optimal lignin removal rate at 130 °C during the DES-mediated hydrothermal pretreatment of the poplar wood hydrolysis residue, with a slight decline at 140 °C.79 This discovery furnishes vital experimental evidence for the fine-tuning of the DES-mediated hydrothermal pretreatment process, guiding the selection and calibration of the process parameters for practical applications. Hong et al. introduced an innovative methodology to produce cellulose nanofibers (CNFs) from birch wood. By incorporating birch-derived cellulose feedstock into a DES composed of betaine hydrochloride and glycerol at a specified temperature (130, 140, or 150 °C) and subjecting it to continuous stirring for a predetermined duration (0.5 or 1 h), they achieved a CNF mass yield of up to 72.5% under the most favorable conditions.80 This pioneering approach promises the effective extraction of CNFs from birch wood, offering significant technical advancements for the sustainable exploitation of biomass resources and the innovation of high-value products.
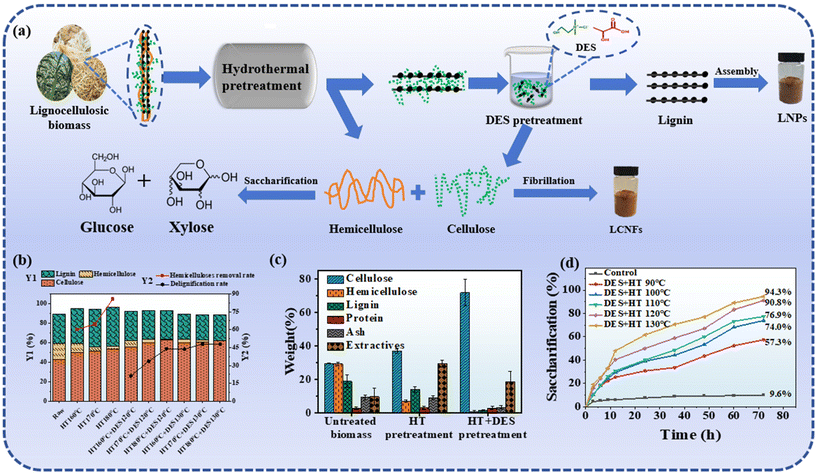 |
| Fig. 6 Synthesis of LCNFs, CNFs and LNPs with the help of DES-mediated hydrothermal pretreatment. (a) Scheme for DES-mediated hydrothermal pretreatment for the synthesis of LCNFs, CNFs, and LNPs.48 (b) Chemical compositions, hemicellulose removal rate, and hemicellulose removal ratio, delignification ratio of raw materials and the pretreated residues.93 (c) Composition of different lignocellulosic biocomponents in solid fractions (pulp) and the release of different soluble biocomponents in the liquid fractions.48 (d) Glucose yields of enzymatic hydrolysis of the original and the DES pretreated substrates at different temperatures.44 | |
Table 2 The summary of biomaterials prepared from lignocellulosic biomass with DES-mediated hydrothermal pretreatment
Bioproduct |
Feedstock |
DES |
Hydrothermal pretreatment conditions |
Key properties of bioproducts |
Ref. |
LCNFs |
Bamboo |
CHPTAC + FA |
120 °C, 20 min |
Exhibited significantly improved mechanical properties and hydrophobicity (water contact angle: 106.26°). |
75
|
LCNFs |
Bamboo |
ChCl + LA |
130 °C, 20 min |
Excellent hydrophobicity (water contact angle of 103.3°). |
81
|
LNCs |
Poplar |
ChCl + LA + p-TsOH |
120 °C, 3 h |
Good dispersion stability; high lignin content of 27.65%; high thermal stability (Tmax = 370 °C). |
82
|
LCNFs |
Radiata pine |
BTMAC + FA + MA |
130 °C, 1.5 h |
Excellent UV-blocking, higher thermal stability, mechanical strength, and strong barrier properties to oil and air |
83
|
LNPs |
Moso bamboo shoot shells |
TEBAC + EM |
160 °C, 1 h |
Lower S/G ratio (0.5) and higher reactive sites, good antioxidant activity (RSI, 11.40–12.58) and thermal stability. |
84
|
LNPs |
Camellia oleifera shell |
ATMAC + AA |
150 °C, 15 min |
Smaller particle size (203.07 nm) and excellent stability |
85
|
LNPs |
Poplar |
ChCl + EG + AlCl3 |
140 °C, 1 h |
Displayed less-cleaved structural features with homogeneous morphological features |
86
|
Nano-lignin |
Wheat straw |
ChCl + LA |
120 °C, 4 h |
600 nm average particle size and 0.10 polydispersity index |
87
|
Nano-lignin |
Eucommia ulmoides pericarp |
ZnCl2 + LA |
120 °C, 2 h |
Excellent antioxidant activity (IC50 = 46.3 μg mL−1) comparable to those of commercial antioxidants |
88
|
In the LCNF aspect, Hui et al. reported that after DES-mediated hydrothermal pretreatment at 120 °C, LCNFs exhibit a well-dispersed reticulated structure after modification, facilitating fibre interweaving and enhancing mechanical properties.75 Xu et al. found that during ChCl-LADES-mediated hydrothermal pretreatment, in situ esterification (carboxylic acid in the DES and –OH in cellulose) occurred and enhanced the water contact angle of the LCNF film to 103.3°, which is beneficial for the intertwining of the nanofibers to form a network.81 Shu et al. found that after DES-mediated hydrothermal pretreatment, an LCNF of about 23.29 nm wide and 1.16 nm thick was obtained from poplar, which exhibited good dispersion stability in water and maintained the cellulose I crystal structure.82 In addition, with the favor of DES-mediated hydrothermal pretreatment, the LCNF synthesized by Xie et al. exhibited a length of up to several microns and a diameter in the 5–90 nm range (about 35 nm of mean diameters).83 These studies used different lignocellulosic biomasses and DESs. Ascribed to the complexity of lignocellulosic biomass and DESs, there is still a lack of universal patterns for the synthesis of CNFs or LCNFs using DES-mediated hydrothermal pretreatment.
3.3.2 Fabrication of lignin nanoparticles (LNPs).
LNPs represent a class of nanoscale materials that are derived from lignin.89 These nanoparticles are characterized by their diminutive size, elevated specific surface area, superior dispersion and stability, and commendable light scattering and thermal stability. They maintain the inherent aromatic structure of lignin and are replete with reactive functional groups such as phenolic hydroxyl, alcoholic hydroxyl, and carbonyl moieties. These features confer LNPs with a high level of chemical tunability and functionality.90 The applications of LNPs are manifold, encompassing areas such as composite material reinforcement, energy storage, drug delivery systems, environmental remediation, and biomedical engineering. The fabrication of LNPs requires the efficient separation of lignin from lignocellulosic biomass. However, hydrothermal pretreatment encounters certain constraints in its ability to remove lignin.91 Considering that the fabrication of LNPs is contingent upon the efficacious segregation of lignin, the integration of hydrothermal pretreatment with technologies designed for lignin separation is projected to yield more productive biomass conversion and the generation of value-added products within the biorefinery process. In this aspect, DESs demonstrate significant potential for the efficient extraction or removal of lignin from lignocellulosic biomass (Table 2 and Fig. 6).92
Recently, two strategies have been developed for the extraction of lignin via DES-mediated hydrothermal pretreatment (Table 2 and Fig. 6). One is the direct extraction from raw biomass via a one-step hydrothermal process; another strategy is composed of two subprocesses, i.e., initial conventional HTT and subsequent HTT of the lignin-rich residue in DESs (Table 2 and Fig. 6). For example, Xu et al. directly extracted nano-lignin from wheat straw via the HTT in the DES composed of ChCl and LA at 120 °C for 4 h. Notably, these nanoparticles have been applied to the fabrication of supercapacitor electrode materials for the first time, exhibiting superior specific capacitance performance compared to conventional lignin.87 Wu et al. directly extracted LNPs from the Camellia oleifera shell via the HTT in the DES composed of ATMAC and LA at 150 °C for 15 min.85 The results indicate that the carboxyl hydrogen in LA with a strong positive charge and the Cl-ion in ATMAC with a strong negative charge form strong hydrogen bonds, leading to a significant delocalization of hydrogen protons, which enhances the acidity of the solvent. Consequently, this increased acidity facilitates the extraction of lignin from the Camellia oleifera shell. While Gong et al. initially treated wood meal by conventional HTT, then the residue after conventional HTT was further hydrothermally treated in the DES composed of ChCl and MA at 120 °C for 3 h. The lignin extracted from the hydrothermal treatment residue via DES pretreatment is characterized by its low molecular weight, uniform distribution, abundance of phenolic hydroxyl groups, and robust antioxidant properties.93 While Zuo et al. initially treated Moso bamboo shoot by conventional HTT, then the residue after conventional HTT was further hydrothermally treated in the DES composed of TEBAC and EM at 120 °C for 3 h.75 The LNPs obtained through these two steps exhibit unique structural characteristics, including a low syringyl/guaiacyl ratio (0.5) and a high density of active sites. Additionally, they exhibit excellent antioxidant properties (with an RSI ranging from 11.40 to 12.58) and superior thermal stability. From the above results, selecting a suitable DES system is very important in LNP preparation.
Overall, during the DES-mediated hydrothermal pretreatment for the fabrication of LNPs, the incorporation of DESs exerted several pivotal effects. Firstly, the presence of DESs markedly enhanced the solubility of lignin, thereby substantially improving the extraction efficacy of this polymer. In addition, DESs facilitated the disruption of the lignin's macromolecular architecture, rendering it more amenable to the formation of nanoscale particles. Finally, the pretreatment with DESs significantly improved the dispersion of the resulting lignin nanoparticles, a factor of considerable importance for the advancement of subsequent application research. For example, Yao et al. investigated a method for the preparation of LNPs using a DES synthesized from ChCl and 5-sulfosalicylic acid (5Saa). The results revealed that LNPs prepared after pretreatment with ChCl-5Saa exhibited a significantly higher ζ-potential of −21 mV. This value was notably higher compared to that of LNPs obtained without DES treatment (−12 mV and −16 mV), indicating that the LNPs produced via DES pretreatment were finer and more stable, thereby demonstrating enhanced dispersibility.94 The use of DESs coupled with hydrothermal pretreatment provides a novel pathway for the efficient extraction of LNPs, which opens up a new path for the sustainable utilization of biomass resources.
3.3.3 Other functional biomaterials assisted by DES-mediated hydrothermal pretreatment.
With the favor of DES-mediated hydrothermal pretreatment, biomass fiber fabrics and biomass-derived carbon fibres were developed. For example, Ye et al. found that ChCl-urea can modify bamboo fibres under hydrothermal conditions to obtain modified fibers, which can have easy spinnability and antibacterial activity to develop functionalized bamboo fiber fabrics.95 Sazali et al. reported that lignin-derived oil palm biomass using a ChCl-based DES can be obtained and used as a carbon fibre precursor.96
In addition, bio-adhesives were also developed from the DES-mediated pretreatment of biomass. Based on excellent dissolving capacity, DESs can destroy the EPS structure on the surface of hydrochar from SS, leading to relatively low wet shear strength due to the formation of lower molecular compounds when hydrochar from SS was used as a precursor of bio-adhesives.97 When hydrochar from bamboo powder after DES treatment was used as a precursor of bio-adhesives, a similar issue was found.98 Probably the active sites on the surface of hydrochar were quenched due to DES promoting hydrolysis and dehydration, leading to it having a relatively low wet shear strength of bio-adhesives with DES. Due to its excellent dissolving capacity, DES-HTT could be promising as a green refinery process for the conversion of both lignocellulosic biomass and high-moisture organic waste.
4. DES-mediated hydrothermal extraction
4.1 Extraction of lipids from microalgae
Microalgae stands out as a preferred feedstock in the production of biofuels and high-value industrial chemicals, offering several significant advantages over traditional crops.99 However, a critical challenge in the microalgae-based biofuel industry is the efficient and cost-effective extraction of lipids from microalgae cells. The rigid cell walls of microalgae, characterized by complex polysaccharides and selective permeability, along with the extensive intermolecular interactions between lipid molecules and organic macromolecules, hinder the extraction process. Cell wall disruption has emerged as a pivotal pretreatment step to augment the lipid yield from microalgal biomass. Current cell wall disruption methods are broadly categorized into biochemical approaches, such as alkali/thermal treatment or enzymolysis, and physical methods, including bead milling, osmotic shock, autoclaving, ultrasound, and microwave treatment. Despite their application in lipid extraction from various species, these methods suffer from some drawbacks, including high energy consumption and operational challenges under extreme conditions like high pressure and temperature. In recent years, researchers have investigated the use of ILs for microalgae pretreatment, recognizing their potential. However, the application of ILs is constrained by factors such as the lengthy and complex synthesis and purification processes, as well as concerns regarding their toxicity and the high costs associated with the reaction process.
DESs represent an innovative green solvent system that offers a compelling alternative to traditional organic solvents in the biofuel extraction process. This alternative addresses concerns related to inflated costs, environmental risks, and thermal stability. Microalgal lipids are within microspheres or enclosed by lipid membranes, shielded by robust cell walls composed of cellulose, hemicellulose, and pectin, among other polymeric substances. The inherently strong and complex nature of these cell walls presents a significant barrier to efficient lipid extraction. Hydrophobic DESs have demonstrated the capacity to weaken the integrity of cell walls while concurrently solubilizing lipids. Huang et al. demonstrated that the use of DESs to facilitate hydrothermal treatment of microalgae can significantly enhance the extraction of green lipids, with the efficiency increasing from 19.03% to 95.75% under optimal conditions.100 Lo et al. further showcased the recoverability of DESs using methanol as an antisolvent, thereby enhancing the viability of DESs as an alternative for microalgal lipid extraction.101 DESs are capable of effectively solubilizing a range of lipids, including triglycerides, fatty acids, and phospholipids, within microalgal cells, attributed to their superior solubility properties. The hydrogen bonding in DES molecules plays a pivotal role in disrupting the cell wall structure of microorganisms such as Chlorella nucleatum (Fig. 7). In this process, the HBD molecule forms new hydrogen bonds with the polar groups of lipid molecules, while metal ions from the DESs engage in ion–dipole interactions with the lipid molecules, thereby enhancing their solubility.102 Additionally, DESs have the ability to reduce lipid crystallinity, facilitating their release from the cell wall and potentially inducing further breakdown of lipid molecules through chemical reactions such as hydrolysis. Operationally, DESs are typically employed at lower temperatures and pressures compared to conventional solvents, which is advantageous for preserving the bioactivity of heat-sensitive lipids. Yu et al. found that DESs (ChCl and LA) had high fractionation efficiency, the lipid extraction rate reached 89.4%, the protein dissolution rate reached 84.2%, and the carbohydrate saccharification rate reached 96.8% (Fig. 7).103
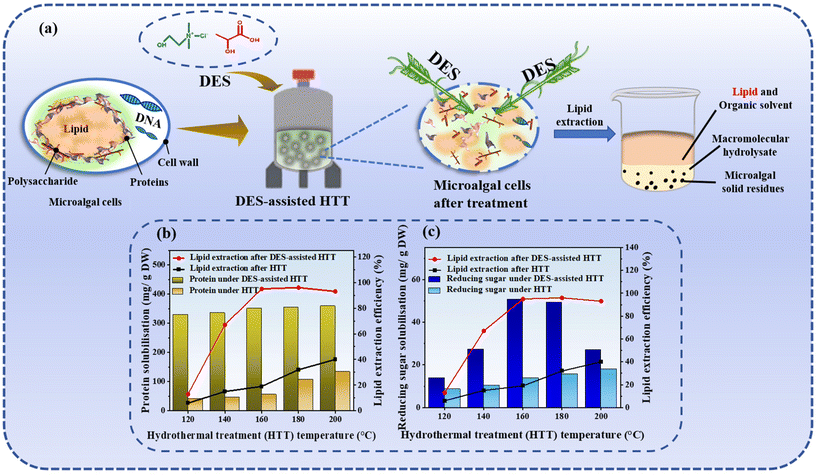 |
| Fig. 7 Lipid extraction from microalgae using DES-HTT. (a) Flowchart of lipid extraction from microalgae via DES-HTT. (b) Lipid extraction and protein solubilisation from wet microalgae by conventional HTT and DES-HTT.101 (c) Lipid extraction and reducing sugar solubilisation after the disintegration of wet microalgae by conventional HTT and DES-HTT.101 | |
In summary, the pivotal role of DESs in the lipid extraction process from microalgae via hydrothermal treatment augmented with DESs lies in its osmotic action, which aids in the disruption of microalgal cell walls and cell membranes, thereby facilitating the liberation of lipids. Given that lipids are nonpolar molecules, the nonpolar characteristics of DESs enable their effective solubilization, ensuring their extraction from the cellular matrix. Furthermore, the synergistic use of DESs with hydrothermal treatment enhances the interfacial contact between lipids and the solvent, thereby augmenting the extraction efficiency of lipids. As research progresses, DES-assisted hydrothermal treatment for lipid extraction from microalgae is anticipated to emerge as a leading strategy for the sustainable utilization of microalgal biomass in the future.
5. DES-mediated HTC
5.1 Nutrient removal from hydrochar as a solid fuel
HTC of SS is recognized as a promising technological approach for the synthesis of solid fuels,104 such as hydrochar, and for the efficient utilization of nutrients including nitrogen (N) and phosphorus (P).105 The process of HTC involves the transformation of organic matter, the migration and conversion of N and P elements,106,107 and the management of the resultant liquid effluent, which are focal points for researchers and engineers in the field.108–110 In recent years, the elimination of nitrogenous compounds from fuels has garnered significant interest. This attention is driven by the tightening environmental regulations that aim to reduce the N content in transportation fuels, thereby mitigating the emission of N oxides, as well as the inhibitory impact of N compounds on the hydrodesulfurization process of fuels.111
The difficulty in removing N is that during HTC, the Maillard reaction occurs to form stable N-heterocyclic compounds.112 Hence, it needs to destroy the EPS structure and inhibit the Maillard reaction to enhance N removal. One promising strategy involves the incorporation of DESs into the HTC process. DESs can effectively disrupt the EPS structure through their dual functionality of solubilization and catalysis. A study by Xu et al. demonstrated that the use of ChCl and oxalic acid as DESs at 210 °C resulted in a 69.49% increase in N removal from hydrochar compared to the process without DESs (Fig. 8).113 When a DES was composed of ZnCl2 and urea, it was found that the N content in hydrochar also has a notable reduction based on dissolving organic matter prior to the aqueous phase to decrease the yield of hydrochar.13 The high solubility and solvent activity of DESs facilitate the solubilization of biomolecules within EPS, disrupting the non-covalent bonds that preserve their structural integrity, including hydrogen bonding, van der Waals forces, and hydrophobic interactions. The synergistic effect of these actions allows DESs to effectively destroy the EPS structure, providing essential support for N removal and the production of hydrochars with low N content. As we all know, except for hydrolysis and dehydration, the Maillard reaction is also a key reaction in the HTC of SS due to the presence of polysaccharides and proteins. On the other hand, during hydrolysis proteins are hydrolyzed to form a relatively low peptide, which will decompose to form NH4+ at high temperatures. From the results of Xu et al., it can be confirmed that a high NH4+-N value13,113 indicates that the Maillard reaction and denitrogenation occur at the same time but denitrogenation is the main reaction.
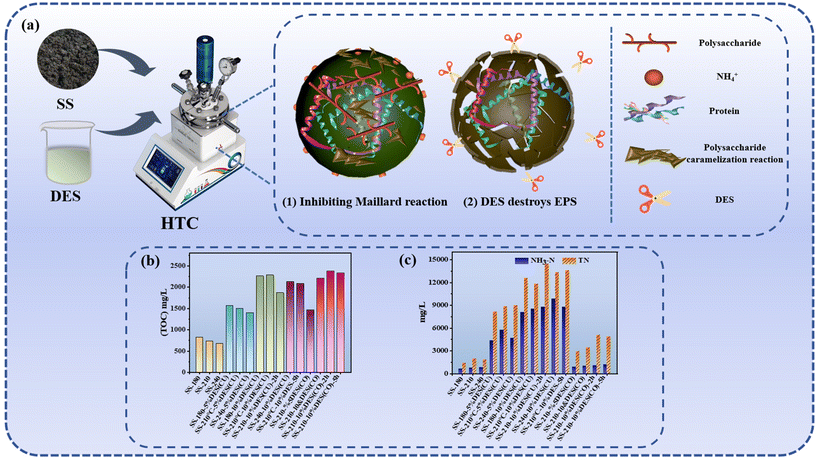 |
| Fig. 8 Enhancing nitrogen removal from SS using DES-mediated HTC. (a) Schematic diagram of DES-mediated HTC for enhancing nitrogen removal from SS. (b)Total organic carbon (TOC) concentrations in the aqueous phase.113 (c) NH3-N and total nitrogen (TN) concentrations in the aqueous phase.113 | |
DESs have also been documented for their use in extracting P minerals from the ash of SS.114 In this context, DESs have been shown to effectively disrupt both EPS and cellular structures, prompting the release of proteins and polysaccharides into the aqueous phase. The small molecular size and unique structure of DES molecules enable them to penetrate the micropores of EPS and interact directly with internal biomolecules, such as proteins and polysaccharides, leading to the disintegration of the EPS network structure. On the other hand, the organic-P can be found in organic matter of SS including orthophosphate diesters (diester-P) poly-P, phosphate and end groups of poly-P.115,116 Hence, when DESs break the EPS structure of SS during HTC, organic-P will also release and transfer to inorganic-P at high temperature. The P minerals in fresh SS can also be extracted using HTC of SS coupling with DESs.117 The key step is disrupting the EPS structure to release organic matter to the aqueous phase. The organic matter under HTC conditions is further decomposed into inorganic-P. It is a new path to recover P from SS with this clean technology.
5.2 Development of functional hydrochar
The synthesis of functional carbonaceous materials has been a hot topic, with the most important change being the shift in feedstock from fossil fuels to biomass. Functional carbonaceous materials have important potential roles in gas storage, adsorbents, electrodes, catalyst supports, carbon fixation, carbon fuel cells and drug delivery.15 The DES-mediated HTC has emerged as a promising approach to developing advanced carbon materials with enhanced functional properties. During the hydrothermal carbonization of biomass, DESs can enhance the dissolution of lignin and hydrolysis of cellulose and hemicellulose, catalyzing the progression of biomass carbonization. In addition, DESs can also directly react with biomass components or the resultant hydrochar via esterification between –OH groups and carboxyl groups.118,119 By altering the feedstock composition and HTC conditions through DES treatment, it is possible to tailor hydrochar characteristics to meet the demands of specific applications.
5.2.1 Enhancing surface chemistry and porosity.
The functional groups introduced or retained on the surface of hydrochar from DES-mediated HTC can significantly affect its performance in targeted applications.10,120 Oxygen-containing functional groups such as hydroxyl, carboxyl, and carbonyl are key active sites for complexing or reducing heavy metal ions.121,122 These groups enable hydrochar to effectively complex or exchange ions with heavy metals like lead, cadmium, and chromium in aqueous solutions, thereby improving its adsorption performance for pollutants such as heavy metals and organic dyes. For instance, Lai et al. successfully synthesized hydrochar with high uranium (U(VI)) adsorption capacity by green and simple carbonization in the DES composed of ChCl and CA, and the maximum U(VI) adsorption of hydrochar prepared at 180 °C reached 353 mg g−1, and the contents of carboxyl (4.0–4.4 mmol g−1) and phenol (3.6–6.7 mmol g−1) of the hydrochar were much higher than the hydrochar from conventional HTC (Fig. 9). In addition, hydrochar showed stable U(VI) adsorption with various competing cations at pH 4.0–8.0 and after five consecutive regeneration cycles.10 The rich content of carboxyl groups on the resultant hydrochar might be attributed to the esterification between hydroxyl groups of the hydrochar or biomass and the carboxyl groups of the DES component (organic acid).121–123
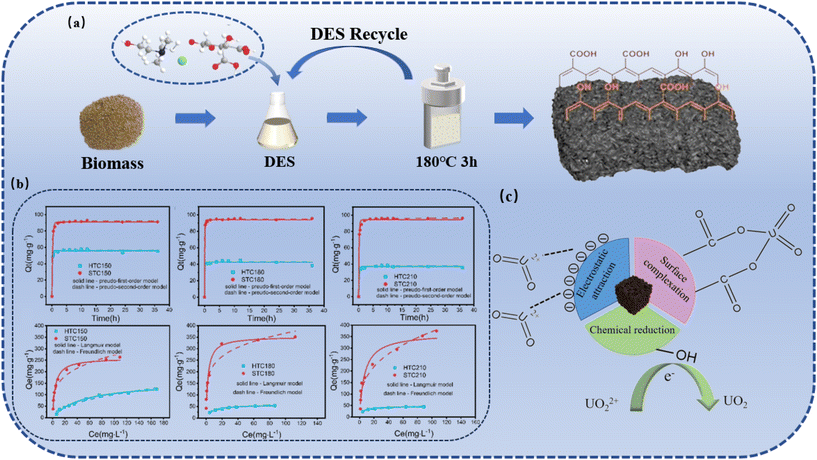 |
| Fig. 9 Synthesis of biocarbons with rich carboxyl and phenol groups via DES-mediated HTC for highly efficient uranium adsorption.10 (a) The scheme for functional biocarbon synthesis via DES-mediated HTC. (b) Uranium adsorption kinetics and isotherms for biocarbons from conventional HTC and DES-mediated HTC. (c) Uranium adsorption mechanisms for the resultant biocarbon. | |
Besides HTC in the pure DES, HTC in a water-containing DES also facilitates the direct preparation of functional hydrochar with tailored surface functionalities. For example, Yadav et al. prepared multifunctional hydrochar from alginate via HTC in the 0%–100% ChCl-EG DES.124 They observed that the hydrochar prepared via HTC in the 81.8% DES is richer in carboxyl groups than the hydrochars prepared at higher and lower DES ratios, suggesting that the ratio of the DES to water in the hydrothermal reaction solution is also a critical factor controlling the formation process of hydrochar during the HTC process.124 Thus, attention should be paid to the impacts of the ratio of the DES to water during the HTC process on the resultant hydrochar in future studies.
5.2.2 Synthesis of hydrochar composites.
DES containing inorganics can be applied to the direct synthesis of hydrochar composites.125,126 Recently, DESs containing metal salts, e.g., FeCl3125,127 and Al(NO3)3,126 have been applied to the synthesis of hydrochar composites via the DES-mediated HTC. For example, Liu et al. developed a DES formed by in situ DES (ChCl–H2O) and inorganic salt (FeCl3) for the preparation of hydrochar from garden waste under hydrothermal conditions from 160 to 260 °C, and the results showed that the adsorption capacity of the hydrochar for MB reached a maximum value of 169.19 mg g−1 at the optimum temperature of 180 °C for 5 h.125 However, there is a lack of comparisons between the conventional HTC with FeCl3 and the DES-mediated HTC with FeCl3. They only investigated the impacts of hydrothermal conditions on the performance of the resultant hydrochar from the DES-mediated HTC.125
5.2.3 Hydrochar as a precursor of heteroatom-rich activated carbon (AC).
Conventional HTC always results in the loss of heteroatoms from the biomass feedstock during the carbonization process, resulting in poorer contents in the resultant AC from hydrochar. Huang et al. prepared hydrochar via HTC in the solution containing 25% DES (ChCl and FA) from N-rich microalgae, and then the resultant hydrochar was converted into AC. They observed that the AC from the hydrochar via DES-mediated HTC was much higher in specific surface area and porosity and richer in N and oxygen than the AC from the hydrochar via conventional HTC.33 The higher porosity was ascribed to the fact that the richer content of thermally unstable oxygen-containing functional groups in the resultant hydrochar via DES-mediated HTC facilitates the pore formation during the activation process.
5.3 Development of CDs
The CDs are an intriguing class of nanocarbon that contains discrete, quasi-spherical nanoparticles with dimensions less than 10 nm. CDs can be easily and inexpensively synthesized in a number of simple ways and can be used in two different ways: top-down and bottom-up.128 The top-down approach involves the decomposition of carbon structures, where larger carbon structures (graphite, graphene, carbon nanotubes, carbon black, coal, etc.) are “broken” into smaller nanostructures under harsh and powerful conditions such as arc discharge, laser ablation, electrochemical etching, or chemical oxidation; and the bottom-up approach involves primarily the thermal decomposition of inexpensive organic molecular precursors or thermal decomposition of polymers to synthesize CDs from small molecules or polymer precursors under relatively simple and mild conditions, e.g., HTT, ultrasonic reaction, microwave-assisted pyrolysis, etc.128,129 Top-down methods are relatively more expensive and less scalable than bottom-up synthesis of CDs.128
With low cost, scalability, excellent chemical stability, and biocompatibility/non-toxicity, CDs show major potential for optical imaging and related biomedical applications.130 Extensive attention has been paid to the synthesis of CDs from biomass, e.g., orange peels,131 banana peels,132 grapefruit peels,133 and kiwi peels,134via HTC routes. In recent years, with the rapid development of research studies on DES, DES-mediated HTC has also been developed for the synthesis of biomass-derived CDs.135–137 For example, based on a novel “waste-treats-pollutant” concept, Guo et al. pretreated wheat straw with a DES composed of ChCl and LA at 90 °C for 12 h, and then the DES-extracted lignin was hydrothermally carbonized in the DES at 180 °C for 6 h to prepare lignin-derived CDs (Fig. 10a).137 The resultant CDs by Guo et al. can be used as a photoluminescence nanoprobe for the identification and sensing of metal ions in wastewater, which can be simply converted into CDs.137 In addition, Huang et al. synthesized CDs using tea residue via HTC in ChCl-urea DES and found that CDs are highly efficient for cadmium detection.138 Çalhan et al. synthesized hydrophilic and hydrophobic fluorescent CDs from molasses using DESs as green solvent media and doping agents (Fig. 10b).139
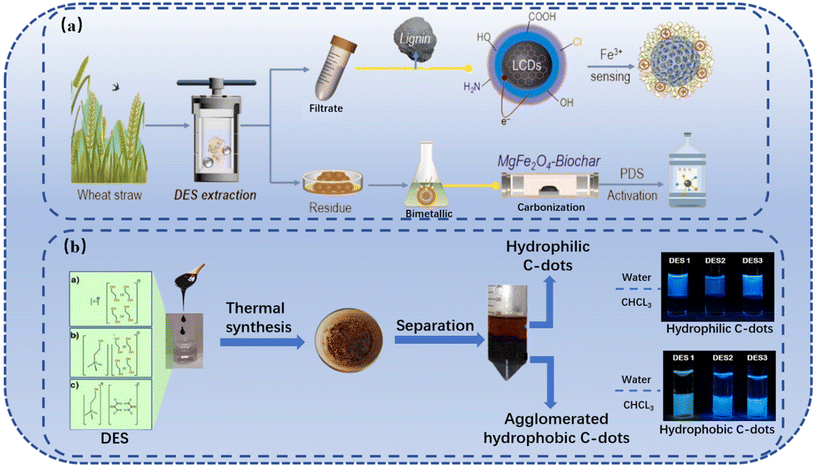 |
| Fig. 10 The synthesis of CDs with the help of DESs. (a) Scheme for the synthesis of CDs from DES-extracted lignin via the DES-mediated carbonization process.137 (b) Scheme for the synthesis of hydrophobic and hydrophilic CDs from molasses using DES as the reaction media.139 | |
While existing studies have focused on the optical properties of CDs, it is equally crucial to explore their other properties to better utilize these materials. For example, N,Cl-CDs were successfully synthesized using a longan shell as a precursor and a DES (chromium chloride/urea) as a solvent and dopant via DES-mediated HTC. The results showed that the negatively charged N,Cl-CDs exhibited good fluorescence properties, as well as rich functional group properties and good antioxidant activity; the anti-inflammatory results of the mouse ear oedema showed that a high dose (1.40 mg kg−1) of N,Cl-CDs had a good anti-inflammatory effect.140 In addition, novel N/Cl-CDs based on a ChCl–urea–glycine ternary DES were synthesized by a hydrothermal method by Zhu et al.141 The N/Cl-CDs exhibited oxidase-like activity and excellent antimicrobial activity against Escherichia coli (E. coli), Staphylococcus aureus (S. aureus), and methicillin-resistant Staphylococcus aureus.141 These findings not only provide new perspectives on the use of CDs in biomedical applications, such as playing important roles in targeted drug delivery, cellular imaging, and the development of antimicrobial agents, but also lay the groundwork for their use in environmental monitoring and antimicrobial material development. In addition, the antioxidant properties of CDs make them potentially applicable in the field of preservatives and nutraceuticals. In the future, through an in-depth study of these properties, the application scope of CDs can be further broadened to achieve broader environmental protection and health care goals.
6. Challenges and prospects
Accelerated advances in the DES-mediated HTT for biomass conversion have been achieved. However, future challenges should also be addressed to promote the application of DES-mediated HTT in biomass conversion (Fig. 11).
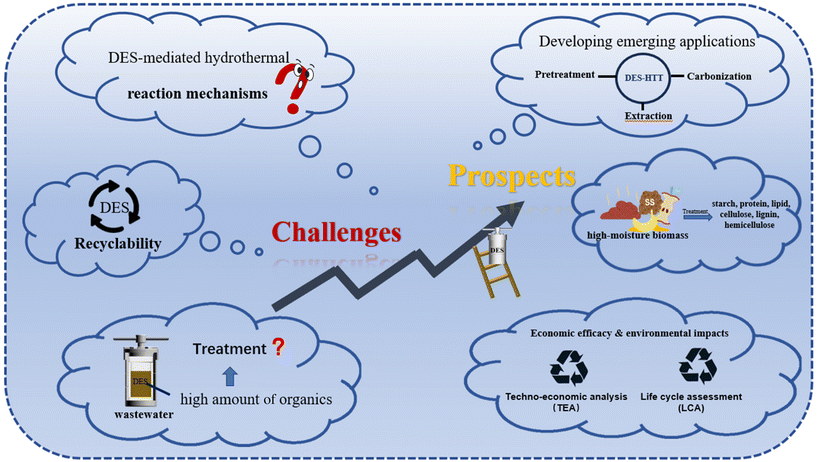 |
| Fig. 11 The illustration of the challenges and prospects of DES-HTT for promoting biomass conversion. | |
6.1 Elucidating DES-mediated hydrothermal reaction mechanisms
Booming advances in the DES-HTT have been achieved in recent years. However, there is still a lack of understanding of the molecular mechanisms of the roles of DESs in the process of hydrothermal treatment. Most studies only observed the impacts after the mediation with DESs in a hydrothermal pretreatment process. For example, the direct hydrothermal carbonization of cotton stalk in the DES composed of ChCl and CA would produce a hydrochar co-enriched with carboxyl and phenol groups, which cannot be achieved via conventional HTC with the presence of CA in water.10 However, the reaction pathways mediated by the DES are still unknown. More experimental and theoretical experiments, including intermediates analyses, active learning, reactive force field molecular dynamics simulations and DFT calculations, should be conducted to shed light on the reaction pathways mediated by the presence of DESs in a specific hydrothermal treatment process, favoring the development of DES-HTT technology for diverse applications.
On the other hand, due to the complexity of biomass, such as SS and lignocellulosic biomass, the pathway of the mechanisms is different. For instance, due to interfering with lignin, cellulose hydrolysis in lignocellulose is limited. During DES-mediated HTC, the hydrolysis of biomass components is accelerated. Is the key role that DES removes lignin then promotes hydrolysis, or HTC enhances hydrolysis to disrupt the interference, or DES coupling with HTC promotes the hydrolysis of cellulose? Finally, the potential reaction between DESs and biomass components during DES-HTT will occur. It would play positive or negative roles in biomass conversion. To date, no paper has focused on this. Hence, it needs further efforts to explore the detailed molecular mechanisms.
6.2 Recyclability of the used DES/DES-containing solution
Considering the cost of DESs, the recyclability of the used DES/DES-containing solution is also a major concern for the development of DES-HTT technology. Under hydrothermal conditions, a DES might react with the biomass component, e.g., through esterification, or undergo dehydration, condensation and even decomposition. Thus, the DES cannot be fully recovered after hydrothermal treatment. Recently, little attention has been paid to the recyclability of the used DES or DES-containing solution. The recovery ratio of the DES after DES-HTT and the efficacy of the recovered DES or DES-containing solution in enhancing biomass valorization through HTT should be specified in future research.
In addition, the recovered DES needs to further confirm its roles in biomass conversion. A variety of intermediates (e.g., glucose, furfuran, furan, FA, AA, and phenol) could be formed from biomass during the hydrothermal process. These intermediates could act as HBDs, resulting in the evolution of DES composition and properties. Thus, the recovered DES is not equal to fresh DES. Consequently, ascribed to the change of DES composition in the recovered DES, further attention should be paid to the functions of recovered DESs for biomass conversion. Tailored DES recovery methods need to be developed to enhance the reusability of DESs.
6.3 Treatment of DES-involved hydrothermal wastewater
When the DES-containing solution is not reusable for enhancing HTT, the treatment or utilization of the DES-containing hydrothermal solution emerges as a new concern. With the rapid progress of hydrothermal treatment, the treatment or valorization of hydrothermal wastewater has attracted increasing attention. However, it is still a huge challenge to treat or valorize hydrothermal wastewater, which contains high amounts of organics and even toxic chemicals.142–144 The composition of hydrothermal wastewater is highly dependent on the composition of biomass source and process conditions. The impacts of DESs in the liquid phase during HTT on the properties of hydrothermal wastewater are still unknown. Several strategies, including AD, supercritical gasification, microbial fuel cells, and algae cultivation, have been developed for the treatment of hydrothermal wastewater.144 Attributed to the presence of phenol chemicals, the hydrothermal wastewater has also shown its potential in antibacterial applications.145,146 Given the introduction of DESs into the liquid phase, the DES-containing hydrothermal solution might be more complex than the conventional hydrothermal solution. The potential positive or negative impacts of DESs on the properties of DES-involved hydrothermal wastewater, e.g., chemical composition, toxicity, and bio-digestibility are still unknown, which impedes the further treatment or valorization of hydrothermal wastewater. More efforts are needed to promote the treatment or valorization of DES-involved hydrothermal wastewater.
6.4 Developing emerging applications
Recently, DES-HTT technology has been divided into DES-mediated hydrothermal pretreatment, extraction and carbonization. DES-HTT has been applied to enhance the dewatering of SS, promote the bioconversion of biomass, facilitate the fabrication of biomaterials (e.g., CNFs, LCNFs, and nano-lignin), and assist the synthesis of biomass-derived carbon materials. Specifically, for the bioconversion of biomass, DES-HTT is applied to enhance enzymatic digestibility, biomethane production, and bioethanol fermentation from lignocellulosic biomass. More bioconversion routes, e.g., single cell protein production, are needed to further promote the valorization of the pretreated biomass via DES-HTT. For the fabrication of biomaterials from lignocellulosic biomass, the CNFs, LCNFs and nano-lignin with tailored surface functionalities should be further fabricated via the reaction with functional DESs during DES-HTT.118
In addition, for the DES-mediated HTC, the resultant biocarbon materials are still focused on energy and environmental applications. Considering the huge amount of biocarbon materials that have been developed via diverse strategies for energy and environmental applications, novel applications of the resultant biocarbon materials, e.g., antibacterial activity and drug delivery, should be developed via the assistance of DESs for tuning the surface functionalities of the biocarbon material. DESs have also shown potential in enhancing the HTL for bio-crude production from biomass waste,147 deserving more attention to developing DES-mediated HTL.
6.5 Furtherance of high-moisture biomass treatment
Hydrothermal treatment stands out as a promising process for the treatment of high-moisture biomass (e.g., SS, kitchen waste, algae, and manure), offering the advantage of bypassing the need for drying pretreatment, coupled with high processing efficiency and lower reaction temperatures. A variety of resources, including starch, protein, lipid, cellulose, lignin, hemicellulose, and/or nutrients, are available from these high-moisture biomasses, which are dependent on the specific source of the biomass.2 Considering the special dissolution capacity of functional DESs, the HTT with the favor of tailored DESs might be more efficient in the recovery of these resources. However, the tailored application of DES-HTT for the recovery of specific sources from these biomasses is still deficient. Due to complex components in the high-moisture biomass, how to obtain aligned components to improve biomass value is a key factor for biomass conversion based on the strong dissolution capacity of DES. On the other hand, dewatering is a huge engineering issue for high-moisture organic waste, such as SS, microalgae, poultry manure and so on. Developing industry-scale high-moisture biomass treatment technology is of critical importance to support the development of DES-HTT.
6.6 Economic efficacy and environmental impacts
Various biological, physical and chemical strategies or their combinations have been developed for the promotion of biomass conversion. Herein, although DES-HTT has shown its ability in the furtherance of biomass conversion, there is still a lag between the development and evaluation of DES-HTT technology. Techno-economic analysis (TEA) and life cycle assessment (LCA) are essential for the commercial viability of a specific biorefinery process, providing insights into economic feasibility, scale-up concerns, uncertainties, impactful parameters, and environmental impacts.118,148,149 The comparison between conventional HTT and DES-HTT should be systematically conducted to investigate how the use of DESs improves economic efficacy and alleviates environmental impacts during the biomass conversion process.
7. Conclusion
This review aims to provide a timely perspective on the DES-mediated hydrothermal treatment for biomass conversion, outlining the latest advances in DES-mediated hydrothermal pretreatment for biomass waste dewatering, bioconversion of biomass, and biomass fractionation, DES-mediated hydrothermal extraction of lipid from biomass, and DES-mediated HTC for functional hydrochar and CD synthesis. These results suggest that DESs could remarkably enhance the HTT processes, favoring the further conversion of biomass. However, further efforts are needed to shed light on the molecular mechanisms of the roles of DESs in HTT processes, point out the recyclability of the used DESs or potential disposal routes for the DES-containing hydrothermal wastewater, and the economic efficacy and environmental impacts of DES-HTT processes. Moreover, with the help of functional DESs, emerging surface functionalities for the product from DES-HTT could be developed to enhance the cost-effectiveness of this green technology.
Abbreviations
4-CSA | 4-Chlorobenzenesulfonic acid |
5Sa | 5-Sulfosalicylic acid |
AA | Acetic acid |
AC | Activated carbon |
AD | Anaerobic digestion |
ATMAC | Allyl trimethylammonium chloride |
BTMAC | Benzyltrimethylammonium chloride |
CA | Citric acid |
CDs | Carbon nanodots |
ChCl | Choline chloride |
CHPTAC | 3-Chloro-2-hydroxypropyltrimethyl ammonium chloride |
CNFs | Cellulose nanofibrils |
CST | Capillary suction time |
CTAB | Cetyltriethylammnonium bromide |
DES | Deep eutectic solvent |
DES-HTT | DES-mediated hydrothermal treatment |
DFT | Density functional theory |
EG | Ethylene glycol |
EM | Ethanolamine |
EPS | Extracellular polymeric substance |
FA | Formic acid |
HTT | Hydrothermal treatment |
HTC | Hydrothermal carbonization |
HTPT | Hydrothermal pretreatment |
HTE | Hydrothermal extraction |
HTL | Hydrothermal liquefaction |
HTG | Hydrothermal gasification |
HBDs | Hydrogen bond donors |
HBAs | Hydrogen bond acceptors |
ILs | Ionic liquids |
LA | Lactic acid |
LCA | Life cycle assessment |
LCDs | Lignin carbon dots |
LCNFs | Lignin-containing nanocellulose fibrils |
LNPs | Lignin nanoparticles |
N | Nitrogen |
p-TsOH |
p-Toluenesulfonic acid |
P | Phosphorus |
SS | Sewage sludge |
TEA | Techno-economic analysis |
TEBAC | Triethylbenzyl ammonium chloride |
UV | Ultraviolet |
Author contributions
Lichun Dai: conceptualization, supervision, funding acquisition, writing – original draft, and writing – review & editing. Zhi-Xiang Xu: conceptualization, supervision, funding acquisition, writing – original draft, and writing – review & editing. Han-Song Zhu: investigation, visualization, and writing – original draft. Xiaotong He: investigation, visualization, and writing – original draft.
Data availability
Data availability is not applicable to this article as no new data were created or analyzed in this study.
Conflicts of interest
The authors declare that they have no known competing financial interests or personal relationships that could have appeared to influence the work reported in this paper.
Acknowledgements
This work was supported by the Science and Technology Project of Sichuan Province (2022YFN0029), the Central Public-Interest Scientific Institution Basal Research Fund for the Chinese Academy of Agricultural Sciences (1610012022013-03102), the Science and Technology Innovation Project of the Chinese Academy of Agricultural Sciences (CAAS-ASTIP-2016-BIOMA), and the Inner Mongolia Major Science and Technology Major Project (2021ZD0022).
References
- S. Sudalai, S. Prabakaran, V. Varalakksmi, I. S. Kireeti, B. Upasana, A. Yuvasri and A. Arumugam, Energy Convers. Manage., 2024, 314, 118724 CrossRef CAS.
- X. Y. Zhang, Q. Y. Qin, X. Sun and W. L. Wang, Front. Nutr., 2022, 9, 986705 CrossRef PubMed.
- T. Xie, Z. Zhang, M. Sun, M. Lv, D. Li, J. Nan and Y. Feng, Environ. Res., 2022, 205, 112537 CrossRef CAS PubMed.
- Y. Zhou, N. Engler, Y. Li and M. Nelles, J. Cleaner Prod., 2020, 259, 121020 CrossRef CAS.
- O. S. Djandja, L. X. Yin, Z. C. Wang and P. G. Duan, Process Saf. Environ. Prot., 2021, 151, 101–127 CrossRef CAS.
- L. Dai, B. Yang, H. Li, F. Tan, N. Zhu, Q. Zhu, M. He, Y. Ran and G. Hu, Bioresour. Technol., 2017, 243, 860–866 CrossRef CAS PubMed.
- L. Dai, F. Tan, B. Wu, M. He, W. Wang, X. Tang, Q. Hu and M. Zhang, J. Environ. Manage., 2015, 157, 49–53 CrossRef CAS PubMed.
- M. Kumar, A. O. Oyedun and A. Kumar, Renewable Sustainable Energy Rev., 2018, 81, 1742–1770 CrossRef.
- Q. Xue, R. K. Wang, S. Meng, S. T. Tan, Z. H. Zhao, Q. Q. Yin and H. Li, Can. J. Chem. Eng., 2023, 101, 5519–5529 CrossRef CAS.
- P. Lai, H. Zhou, Z. Niu, L. Li, W. Zhu and L. Dai, Chem. Eng. J., 2023, 457, 141255 CrossRef CAS.
- Z. X. Xu, X. Q. Ma, Y. Q. Shan, B. Li, S. M. Osman, P. G. Duan and R. Luque, Chemosphere, 2022, 308, 135840 CrossRef CAS PubMed.
- M. Wang, C. H. Li, M. L. Zhou, Z. N. Xia and Y. K. Huang, Green Chem., 2022, 24, 6696–6706 RSC.
- Z. Xu, X. Ma, J. Liao, S. M. Osman, S. Wu and R. Luque, ACS Sustainable Chem. Eng., 2022, 10, 4258–4268 CrossRef CAS.
- Q. Zhang, K. D. O. Vigier, S. Royer and F. Jérôme, Chem. Soc. Rev., 2012, 41, 7108–7146 RSC.
- Y. Shen, H. Zhou, X. He, F. Shen, Z. Xu, B. Yang, L. Z. Kong and L. Dai, Green Chem., 2024, 26, 8123–8144 RSC.
- B. B. Hansen, S. Spittle, B. Chen, D. Poe, Y. Zhang, J. M. Klein, A. Horton, L. Adhikari, T. Zelovich and B. W. Doherty, Chem. Rev., 2020, 121, 1232–1285 CrossRef PubMed.
- L. J. He, L. Chen, B. H. Zheng, H. Zhou, H. Wang, H. Li, H. Zhang, C. C. Xu and S. Yang, Green Chem., 2023, 25, 7410–7440 RSC.
- M. H. Mruthunjayappa, N. S. Kotrappanavar and D. Mondal, Prog. Mater. Sci., 2022, 126, 100932 CrossRef CAS.
- S. Sharma, P. Nargotra, V. Sharma, R. Bangotra, M. Kaur, N. Kapoor, S. Paul and B. K. Bajaj, Bioresour. Technol., 2021, 333, 125191 CrossRef CAS PubMed.
- B. Shen, S. Hou, Y. Jia, C. Yang, Y. Su, Z. Ling, C. Huang, C. Lai and Q. Yong, Bioresour. Technol., 2021, 341, 125787 CrossRef CAS PubMed.
- L. Soh and M. J. Eckelman, ACS Sustainable Chem. Eng., 2016, 4, 5821–5837 CrossRef CAS.
- B. Kudłak, K. Owczarek and J. Namieśnik, Environ. Sci. Pollut. Res., 2015, 22, 11975–11992 CrossRef PubMed.
- C. Ruß and B. König, Green Chem., 2012, 14, 2969–2982 RSC.
- A. P. Abbott, D. Boothby, G. Capper, D. L. Davies and R. K. Rasheed, J. Am. Chem. Soc., 2004, 126, 9142–9147 CrossRef CAS PubMed.
- E. L. Smith, A. P. Abbott and K. S. Ryder, Chem. Rev., 2014, 114, 11060–11082 CrossRef CAS PubMed.
- L. I. Tomé, V. Baião, W. da Silva and C. M. Brett, Appl. Mater. Today, 2018, 10, 30–50 CrossRef.
- K. Shahbaz, F. Mjalli, M. Hashim and I. AlNashef, Energy Fuels, 2011, 25, 2671–2678 CrossRef CAS.
- I. M. Pateli, A. P. Abbott, G. R. Jenkin and J. M. Hartley, Green Chem., 2020, 22, 8360–8368 RSC.
- J. Richter and M. Ruck, Molecules, 2019, 25, 78 CrossRef PubMed.
- W. Ge, J. Shuai, Y. Wang, Y. Zhou and X. Wang, Polym. Chem., 2022, 13, 359–372 RSC.
- S. Magalhães, C. Fernandes, J. F. Pedrosa, L. Alves, B. Medronho, P. J. Ferreira and M. d. G. Rasteiro, Polymers, 2023, 15, 3138 CrossRef PubMed.
- Y. Zhu, T. X. Yang, H. Li, B. K. Qi, Q. S. Zhao and B. Zhao, Int. J. Biol. Macromol., 2023, 236, 123977 CrossRef CAS PubMed.
- R. Huang, A. Zhong, K. Huang, Y. Yu, Y. Tang and P. Xia, J. Environ. Chem. Eng., 2023, 11, 111474 CrossRef CAS.
- L. Wang, X. Li, J. Jiang, Y. Zhang, S. Bi and H.-M. Wang, Ind. Crops Prod., 2022, 180, 114696 CrossRef CAS.
- A. Bjelić, B. Hočevar, M. Grilc, U. Novak and B. Likozar, Rev. Chem. Eng., 2022, 38, 243–272 CrossRef.
- S. Hong, X. J. Shen, Z. M. Xue, Z. H. Sun and T. Q. Yuan, Green Chem., 2020, 22, 7219–7232 RSC.
- A. Raheem, V. S. Sikarwar, J. He, W. Dastyar, D. D. Dionysiou, W. Wang and M. Zhao, Chem. Eng. J., 2018, 337, 616–641 CrossRef CAS.
- J. Liang and Y. Zhou, Water Res., 2022, 218, 118499 CrossRef CAS PubMed.
- Z. Zhang, J. Lu, Z. Zhang, J. Yang, K. Xin, Z. Zhao, L. An and D. Kong, Chem. Eng. J., 2022, 431, 134025 CrossRef CAS.
- X. Zhuang, Y. Huang, X. Yin and C. Wu, Chem. Ind. Eng. Process., 2018, 37, 311–318 Search PubMed.
- X. Zhuang, X. Yin, Y. Huang and C. Wu, Chem. Ind. Eng. Process., 2017, 36, 4224–4231 Search PubMed.
- X. Liu, Y. Zhai, Z. Xu, Y. Zhu, Y. Zhou, Z. Wang, L. Liu, W. Ren, Y. Xie and C. Li, Sep. Purif. Technol., 2023, 306, 122714 CrossRef CAS.
- X. Liu, Y. Zhai, Z. Xu, Y. Zhu, Y. Zhou, Z. Wang, L. Liu, F. Liang, W. Ren and Y. Xie, Chem. Eng. J., 2023, 464, 142575 CrossRef CAS.
- X. J. Shen, J. L. Wen, Q. Q. Mei, X. Chen, D. Sun, T. Q. Yuan and R. C. Sun, Green Chem., 2019, 21, 275–283 RSC.
- Z. Y. Tang, L. Li, W. Tang, J. W. Shen, Q. Z. Yang, C. L. Ma and Y. C. He, Bioresour. Technol., 2023, 381, 129106 CrossRef CAS PubMed.
- L. Chang, R. Ye, J. Song, Y. Xie, Q. Chen, S. Yan, K. Sun and L. Gan, Appl. Sci., 2023, 13, 2429 CrossRef CAS.
- J. Xie, J. Zhao, H. Xu, N. Zhang, Y. Chen, J. Yang, K. Wang and J. Jiang, Int. J. Biol. Macromol., 2024, 274, 133443 CrossRef CAS PubMed.
- B. Basak, S. Patil, R. Kumar, G. S. Ha, Y. K. Park, M. A. Khan, K. K. Yadav, A. M. Fallatah and B. H. Jeon, Bioresour. Technol., 2022, 351, 127034 CrossRef CAS PubMed.
- F. Rodríguez-Rebelo, B. Rodríguez-Martínez, P. G. Del-Río, M. N. Collins, G. Garrote and B. Gullón, Ind. Crops Prod., 2024, 216, 118761 CrossRef.
- H. Manivannan and B. L. Anguraj, Biomass Convers. Biorefin., 2023, 13, 5731–5741 CrossRef CAS.
- C. He, L. Zhang, X. Zhao, J. Xin, C. Li, C. Li and X. Zhang, Renewable Energy, 2024, 229, 120745 CrossRef CAS.
- J. Lee, S. Kim, S. You and Y.-K. Park, Renewable Sustainable Energy Rev., 2023, 178, 113240 CrossRef CAS.
- Y. Xie, X. Liu, L. Liu, Y. Zhou, Z. Wang, C. Huang, H. He and Y. Zhai, J. Environ. Manage., 2024, 356, 120615 CrossRef CAS PubMed.
- X. Kang, C. Deng, R. Shinde, R. Lin and J. D. Murphy, Energy Convers. Manage., 2023, 288, 117115 CrossRef CAS.
- S. B. Elmaci, J. Schultz, A. Isci, M. Scherzinger, D. D. Aslanhan, M. D. Cam, O. Sakiyan and M. Kaltschmitt, Waste Manage., 2024, 181, 114–127 CrossRef PubMed.
- B. Sharma, C. Larroche and C.-G. Dussap, Bioresour. Technol., 2020, 313, 123630 CrossRef CAS PubMed.
- P. G. Del Río, V. D. Domínguez, E. Domínguez, P. Gullón, B. Gullón, G. Garrote and A. Romaní, Bioresour. Technol., 2020, 314, 123722 CrossRef PubMed.
- O. A. Fakayode, N. D. K. Akpabli-Tsigbe, H. Wahia, S. Tu, M. Ren, C. Zhou and H. Ma, Renewable Energy, 2021, 180, 258–270 CrossRef CAS.
- C. Y. Ma, L. H. Xu, C. Zhang, K. N. Guo, T. Q. Yuan and J. L. Wen, Bioresour. Technol., 2021, 341, 125828 CrossRef CAS PubMed.
- W. Wang and D. J. Lee, Bioresour. Technol., 2021, 339, 125587 CrossRef CAS PubMed.
- V. Sharma, M. L. Tsai, C. W. Chen, P. P. Sun, A. K. Patel, R. R. Singhania, P. Nargotra and C. D. Dong, Bioresour. Technol., 2022, 360, 127631 CrossRef CAS PubMed.
- A. Pérez-Pérez, P. G. Del-Río, A. Lobato-Rodríguez, G. Garrote and B. Gullón, Ind. Crops Prod., 2023, 203, 117157 CrossRef.
- Z. Guo, Q. Zhang, T. You, Z. Ji, X. Zhang, Y. Qin and F. Xu, Bioresour. Technol., 2019, 293, 122036 CrossRef CAS PubMed.
- Y. Liu, W. Chen, Q. Xia, B. Guo, Q. Wang, S. Liu, Y. Liu, J. Li and H. Yu, ChemSusChem, 2017, 10, 1692–1700 CrossRef CAS PubMed.
- X. Xu, J. Gai, Y. Li, Z. Zhang, S. Wu, K. Song, J. Hu and Q. Chu, Int. J. Biol. Macromol., 2024, 259, 129138 CrossRef CAS PubMed.
- X. Liu, Y. Li, C. M. Ewulonu, J. Ralph, F. Xu, Q. Zhang, M. Wu and Y. Huang, ACS Sustainable Chem. Eng., 2019, 7, 14135–14142 CrossRef CAS.
- Z. Chen, Y. Hu, G. Shi, H. Zhuo, M. A. Ali, E. Jamróz, H. Zhang, L. Zhong and X. Peng, Adv. Funct. Mater., 2023, 33, 2214245 CrossRef CAS.
- D. Pradhan, A. K. Jaiswal and S. Jaiswal, Carbohydr. Polym., 2022, 285, 119258 CrossRef CAS PubMed.
- S. Mondal, Carbohydr. Polym., 2017, 163, 301–316 CrossRef CAS PubMed.
- H. Bian, L. Chen, M. Dong, L. Wang, R. Wang, X. Zhou, C. Wu, X. Wang, X. Ji and H. Dai, Int. J. Biol. Macromol., 2021, 166, 1578–1585 CrossRef CAS PubMed.
- Y. Zhu, Z. Yu, J. Zhu, Y. Zhang, X. Ren and F. Jiang, Chem. Eng. J., 2022, 445, 136748 CrossRef CAS.
- H. Yousefi, V. Azari and A. Khazaeian, Ind. Crops Prod., 2018, 115, 26–31 CrossRef CAS.
- K. Liu, H. Du, T. Zheng, W. Liu, M. Zhang, H. Liu, X. Zhang and C. Si, Green Chem., 2021, 23, 9723–9746 RSC.
- V. R. Chourasia, M. Bisht, K. K. Pant and R. J. Henry, Bioresour. Technol., 2022, 351, 127005 CrossRef CAS PubMed.
- X. Y. Hui, C. Zuo, Y. Xu, B. Wang, J. L. Wen and T. Q. Yuan, Chem. Eng. J., 2024, 493, 152517 CrossRef CAS.
- Q. Sun, W. J. Chen, B. Pang, Z. H. Sun, S. S. Lam, C. Sonne and Q. Yuan, Bioresour. Technol., 2021, 341, 125807 CrossRef CAS PubMed.
- R. Wang, K. Wang, M. Zhou, J. Xu and J. Jiang, Bioresour. Technol., 2021, 328, 124873 CrossRef CAS PubMed.
- W. Chen, Z. Xue, J. Wang, J. Jiang, X. Zhao and T. Mu, Acta Phys.-Chim. Sin., 2018, 34, 904–911 CAS.
- X. Chen, Q. Liu, B. Li, N. Wang, C. Liu, J. Shi and L. Liu, Int. J. Biol. Macromol., 2024, 259, 129354 CrossRef CAS PubMed.
- S. Hong, Y. Yuan, P. Li, K. Zhang, H. Lian and H. Liimatainen, Ind. Crops Prod., 2020, 154, 112677 CrossRef CAS.
- Y. Xu, S. C. Sun, C. Zhang, C. Y. Ma, J. L. Wen and T. Q. Yuan, Chem. Eng. J., 2023, 462, 142213 CrossRef CAS.
- F. Shu, Y. Guo, L. Huang, M. Zhou, G. Zhang, H. Yu, J. Zhang and F. Yang, Ind. Crops Prod., 2022, 177, 114404 CrossRef CAS.
- J. Xie, J. Xu, Z. Zhang, B. Wang, S. Zhu, J. Li and K. Chen, Chem. Eng. J., 2023, 451, 138591 CrossRef CAS.
- C. Zuo, Y. Xu, X. Y. Hui, L. H. Xu, J. L. Wen and T. Q. Yuan, Chem. Eng. J., 2024, 485, 149622 CrossRef CAS.
- Y. Wu, M. Xie, X. Liu, S. Qiu, W. Zeng, Z. Jiang, R. Liu, Z. Xiao, C. Li and Y. Zhang, Ind. Crops Prod., 2024, 210, 118018 CrossRef CAS.
- Y. T. Yang, M. K. Qin, Q. Sun, Y. F. Gao, C. Y. Ma and J. L. Wen, Int. J. Biol. Macromol., 2022, 209, 1882–1892 CrossRef CAS PubMed.
- M. Xu, X. Zhu, Y. Lai, A. Xia, Y. Huang, X. Zhu and Q. Liao, Appl. Energy, 2024, 353, 122095 CrossRef CAS.
- X. Yao, Y. Pan, X. Ma, S. Yin and M. Zhu, Int. J. Biol. Macromol., 2023, 253, 127221 CrossRef CAS PubMed.
- S. Iravani and R. S. Varma, Green Chem., 2020, 22, 612–636 RSC.
- Z. Zhang, V. Terrasson and E. Guénin, Nanomaterials, 2021, 11, 1336 CrossRef CAS PubMed.
- S. L. Sun, S. N. Sun, J. L. Wen, X. M. Zhang, F. Peng and R. C. Sun, Bioresour. Technol., 2015, 175, 473–479 CrossRef CAS PubMed.
- Z. Chen, X. Bai, H. Zhang and C. Wan, ACS Sustainable Chem. Eng., 2020, 8, 9783–9793 CrossRef CAS.
- W. H. Gong, C. Zhang, J. W. He, Y. Y. Gao, Y. J. Li, M. Q. Zhu and J. L. Wen, Int. J. Biol. Macromol., 2022, 209, 188–197 CrossRef CAS PubMed.
- M. Yao, B. Liu, L. Qin, Z. Du, Z. Wang, C. Qin, C. Liang, C. Huang and S. Yao, Green Chem., 2024, 26, 4528–4543 RSC.
- X. Y. Ye, E. Q. Zhu, D. W. Wang, J. Yang, H. Y. Yang and Z. J. Shi, Ind. Crops Prod., 2022, 188, 115607 CrossRef CAS.
- A. L. Sazali, S. K. Amran, M. R. Anuar, K. F. Pa'ee and T. L. K. Yong, Biomass Convers. Biorefin., 2023, 14, 29451–29464 CrossRef.
- Z. X. Xu, R. Dou, Y. Tan, H. R. Xiong, Y. X. Chen, L. J. Leng, M. Vlaskin, S. M. Osman and R. Luque, Biomass Convers. Biorefin., 2024, 18, 1978–1993 CAS.
- Y. X. Chen, H. S. Zhu, F. Gao, H. R. Xiong, H. Yang, Z. X. Xu, P. G. Duan, L. J. Zheng, S. M. Osman and R. Luque, Chem. Eng. J., 2024, 498, 155667 CrossRef CAS.
- W. Lu, M. A. Alam, Y. Pan, J. Wu, Z. Wang and Z. Yuan, Bioresour. Technol., 2016, 218, 123–128 CrossRef CAS PubMed.
- R. Huang, Y. He, X. Yao, Y. Yu, W. Song, W. Yang and J. Cheng, Green Chem., 2022, 24, 1615–1626 RSC.
- C. Lo, R. H. Wijffels and M. H. Eppink, Food Bioprod. Process., 2024, 143, 21–27 CrossRef CAS.
- M. C. Matchim Kamdem, A. D. Tamafo Fouegue and N. Lai, Energies, 2023, 16, 3806 CrossRef CAS.
- D. Yu, H. Luo, J. Tan, J. Yuan, J. Chen, X. Liu and H. Li, Fuel, 2024, 363, 130892 CrossRef CAS.
- Z. X. Xu, X. Q. Ma, J. Zhou, P. G. Duan, W. Y. Zhou, A. Ahmad and R. Luque, J. Anal. Appl. Pyrolysis, 2022, 167, 105678 CrossRef CAS.
- Y. Tang, H. Xie, J. Sun, X. Li, Y. Zhang and X. Dai, Water Res., 2022, 211, 118036 CrossRef CAS PubMed.
- R. Wang, H. Lei, S. Liu, X. Ye, J. Jia and Z. Zhao, Sci. Total Environ., 2021, 776, 145922 CrossRef CAS PubMed.
- S. Zhou, Q. Wang, X. Huo, X. Zhu, R. Huang and S. Zhang, ACS ES&T Eng., 2023, 3, 1125–1134 Search PubMed.
- Z. X. Xu, H. Song, P. J. Li, Z. X. He, Q. Wang, K. Wang and P. G. Duan, Chem. Eng. J., 2020, 387, 123410 CrossRef CAS.
- A. S. Oliveira, A. Sarrión, J. A. Baeza, E. Diaz, L. Calvo, A. F. Mohedano and M. A. Gilarranz, Chem. Eng. J., 2022, 442, 136301 CrossRef CAS.
- Z. X. Xu, R. Dou, F. Gao, Y. X. Chen, L. J. Leng, S. M. Osman and R. Luque, Chem. Eng. J., 2024, 490, 151685 CrossRef CAS.
- M. C. Ali, Q. Yang, A. A. Fine, W. Jin, Z. Zhang, H. Xing and Q. Ren, Green Chem., 2016, 18, 157–164 RSC.
- Z. X. Xu, H. Song, P. J. Li, X. Zhu, S. Zhang, Q. Wang, P. G. Duan and X. Hu, J. Hazard. Mater., 2020, 398, 122833 CrossRef CAS PubMed.
- Z. Xu, Y. Tan, X. Ma, S. Wu, B. Zhang and R. Luque, J. Environ. Chem. Eng., 2023, 11, 109342 CrossRef CAS.
- A. Söldner, J. Zach and B. König, Green Chem., 2019, 21, 321–328 RSC.
- F. Gao, X. Ma, Y. Tan, B. Zhang, Y. Yang, H. Nie and Z. Xu, Molecules, 2023, 28, 6494 CrossRef CAS PubMed.
- Z. X. Xu, Y. Tan, X. Q. Ma, B. Li, Y. X. Chen, B. Zhang, S. M. Osman, J. Y. Luo and R. Luque, Environ. Res., 2023, 239, 117421 CrossRef CAS PubMed.
- R. Dou, F. Gao, Y. Tan, H.-R. Xiong, Z.-X. Xu, S. M. Osman, L.-J. Zheng and R. Luque, Sustainable Chem. Pharm., 2024, 37, 101408 CrossRef CAS.
- X. Shi, Z. Wang, S. Liu, Q. Xia, Y. Liu, W. Chen, H. Yu and K. Zhang, Nat. Sustain., 2024, 7, 315–325 CrossRef.
- J. Q. Wang, W. Q. Jing, H. Y. Tian, M. Liu, H. Y. Yan, W. T. Bi and D. D. Y. Chen, ACS Sustainable Chem. Eng., 2020, 8, 12080–12088 CrossRef CAS.
- Y. Zhang, L. Yu, W. X. Ge, W. T. Bi and D. D. Y. Chen, Int. J. Biol. Macromol., 2023, 253, 127394 CrossRef CAS PubMed.
- Y. Bian, Z. Bian, J. Zhang, A. Ding, S. Liu, L. Zheng and H. Wang, Chin. J. Chem. Eng., 2015, 23, 1705–1711 CrossRef CAS.
- M. Sevilla, J. A. Maciá-Agulló and A. B. Fuertes, Biomass Bioenergy, 2011, 35, 3152–3159 CrossRef CAS.
- Y. L. Zhang, Y. H. Meng, L. Ma, H. R. Ji, X. Q. Lu, Z. Q. Pang and C. H. Dong, J. Cleaner Prod., 2021, 324, 129270 CrossRef CAS.
- N. Yadav, M. M. Halanur, M. Bisht, S. K. Nataraj, P. Venkatesu and D. Mondal, Chem. Commun., 2020, 56, 9659–9662 RSC.
- Y. Liu, Y. Cao and Q. Yu, Biomass Bioenergy, 2022, 167, 106626 CrossRef CAS.
- H. M. Manohara, K. Aruchamy, S. Chakraborty, N. Radha, M. R. Nidhi, D. Ghosh, S. K. Nataraj and D. Mondal, ACS Sustainable Chem. Eng., 2019, 7, 10143–10153 CrossRef CAS.
- K. Aruchamy, M. Bisht, P. Venkatesu, D. Kalpana, M. R. Nidhi, N. Singh, D. Ghosh, D. Mondal and S. K. Nataraj, Green Chem., 2018, 20, 3711–3716 RSC.
- G. A. M. Hutton, B. C. M. Martindale and E. Reisner, Chem. Soc. Rev., 2017, 46, 6111–6123 RSC.
- C. Hu, M. Li, J. Qiu and Y.-P. Sun, Chem. Soc. Rev., 2019, 48, 2315–2337 RSC.
- S. N. Baker and G. A. Baker, Angew. Chem., Int. Ed., 2010, 49, 6726–6744 CrossRef CAS PubMed.
- P. Surendran, A. Lakshmanan, G. Vinitha, G. Ramalingam and P. Rameshkumar, Luminescence, 2020, 35, 196–202 CrossRef CAS PubMed.
- R. Atchudan, T. N. Jebakumar Immanuel Edison, M. Shanmugam, S. Perumal, T. Somanathan and Y. R. Lee, Phys. E, 2021, 126, 114417 CrossRef CAS.
- W. Lu, X. Qin, S. Liu, G. Chang, Y. Zhang, Y. Luo, A. M. Asiri, A. O. Al-Youbi and X. Sun, Anal. Chem., 2012, 84, 5351–5357 CrossRef CAS PubMed.
- R. Atchudan, S. Chandra Kishore, P. Gangadaran, T. N. Jebakumar Immanuel Edison, S. Perumal, R. L. Rajendran, M. Alagan, S. Al-Rashed, B.-C. Ahn and Y. R. Lee, Environ. Res., 2022, 204, 112365 CrossRef CAS PubMed.
- Y. Wang, M. Feng, B. He, X. Chen, J. Zeng and J. Sun, Appl. Surf. Sci., 2022, 599, 153705 CrossRef CAS.
- N. Wang, A. Q. Zheng, X. Liu, J. J. Chen, T. Yang, M. Chen and J. H. Wang, ACS Appl. Mater. Interfaces, 2018, 10, 7901–7909 CrossRef CAS PubMed.
- J. Guo, J. Xu, X. Liu, L. Dai, C. Zhang, X. Xiao and K. Huo, J. Hazard. Mater., 2022, 435, 129072 CrossRef CAS PubMed.
- Z. Y. Huang, W. Z. Wu, Z. X. Li, Y. Wu, C. B. Wu, J. Gao, J. Guo, Y. Chen, Y. Hu and C. Huang, Ind. Crops Prod., 2022, 184, 115085 CrossRef CAS.
- S. D. Çalhan, M. Alas, M. Asik, F. N. D. Kaya and R. Genç, J. Mater. Sci., 2018, 53, 15362–15375 CrossRef.
- M. Tian, D. Wang, Q. Liu, L. Wang, Y. Tao, J. Wang, Y. Zou, Y. Yang, Q. Zhou, L. Li, M. Wang, X. Li and D. Gao, J. Mol. Liq., 2024, 397, 124131 CrossRef CAS.
- J. Zhu, X. Li, X. Wu, T. Yuan and Y. Yang, Lett. Appl. Microbiol., 2022, 74, 684–694 CrossRef CAS PubMed.
- M. Usman, Z. J. Shi, N. Dutta, M. A. Ashraf, B. Ishfaq and M. G. El-Din, Environ. Res., 2022, 212, 113532 CrossRef CAS PubMed.
- L. J. Leng, L. H. Yang, J. Chen, Y. B. Hu, H. L. Li, H. Li, S. J. Jiang, H. Y. Peng, X. Z. Yuan and H. J. Huang, J. Cleaner Prod., 2021, 294, 126238 CrossRef CAS.
- L. J. Leng, W. J. Zhang, S. Q. Leng, J. Chen, L. H. Yang, H. L. Li, S. J. Jiang and H. J. Huang, Sci. Total Environ., 2020, 748, 142383 CrossRef CAS PubMed.
- Y. D. Xu, Y. Y. Wang, J. W. Lu, C. B. Yuan, L. L. Zhang and Z. D. Liu, Water Res., 2022, 226, 119318 CrossRef CAS PubMed.
- F. B. Yu, W. Zhao, T. Qin, W. Zhao, Y. L. Chen, X. Y. Miao, L. T. Lin, H. Shang, G. D. Sui, D. X. Peng, Y. Yang, Y. G. Zhu, S. C. Zhang and X. D. Zhu, Proc.
Natl. Acad. Sci. U. S. A., 2022, 119, e2106843119 CrossRef CAS PubMed.
- Y. Alhassan, N. Kumar and I. M. Bugaje, Bioresour. Technol., 2016, 199, 375–381 CrossRef CAS PubMed.
- A. W. Bartling, M. L. Stone, R. J. Hanes, A. Bhatt, Y. M. Zhang, M. J. Biddy, R. Davis, J. S. Kruger, N. E. Thornburg, J. S. Luterbacher, R. Rinaldi, J. S. M. Samec, B. F. Sels, Y. Roman-Leshkov and G. T. Beckham, Energy Environ. Sci., 2021, 14, 4147–4168 RSC.
- M. Hu, K. Guo, H. Zhou, W. Zhu, L. Deng and L. Dai, Energy, 2024, 308, 133037 CrossRef CAS.
Footnote |
† These two authors contributed equally to this work. |
|
This journal is © The Royal Society of Chemistry 2025 |
Click here to see how this site uses Cookies. View our privacy policy here.