DOI:
10.1039/D4LC00859F
(Critical Review)
Lab Chip, 2025,
25, 1047-1080
Aptamer selection via versatile microfluidic platforms and their diverse applications
Received
10th October 2024
, Accepted 11th December 2024
First published on 8th January 2025
Abstract
Aptamers are synthetic oligonucleotides that bind with high affinity and specificity to various targets, making them invaluable for diagnostics, therapeutics, and biosensing. Microfluidic platforms can improve the efficiency and scalability of aptamer selection, especially through advancements in systematic evolution of ligands by exponential enrichment (SELEX) methods. Microfluidic SELEX methods are less time-consuming and labor-intensive and include critical steps like library preparation, binding, partitioning, and amplification. This review examines the contributions of microfluidic technology to SELEX-based aptamer identification, with alternative methods like conditional SELEX, in vivo-like SELEX and Non-SELEX for selecting aptamers and also discusses critical SELEX steps over the past decade. This work also examined the integrated microfluidic systems for SELEX, highlighting innovations such as conditional SELEX and in vivo-like SELEX. These advancements provide potential solutions to existing challenges in aptamer selection using conventional SELEX, especially concerning biological samples. A trend toward non-SELEX methods was also reviewed and discussed, wherein nucleic acid amplification was eliminated to improve aptamer selection. Microfluidic platforms have demonstrated versatility not only in aptamer selection but also in various detection applications; they allow for precise control of liquid flow and have been essential in the advancement of therapeutic aptamers, facilitating accurate screening, enhancing drug delivery systems, and enabling targeted therapeutic interventions. Although advances in microfluidic technology are expected to enhance aptamer-based diagnostics, therapeutics, and biosensing, challenges still persist, especially in up-scaling microfluidic systems for various clinical applications. The advantages and limitations of integrating microfluidic platforms with aptamer development are further addressed, emphasizing areas for future research. We also present a perspective on the future of microfluidic systems and aptamer technologies, highlighting their increasing significance in healthcare and diagnostics.
 Yi-Da Chung | Yi-Da Chung received his M.S. degree from the Department of Life Sciences at National Tsing Hua University, Taiwan, in 1998. Currently, he is a PhD candidate in the Department of Power Mechanical Engineering at National Tsing Hua University, Taiwan. His research expertise includes the technology of aptamer selection, SELEX, and the development of microfluidic chips and biosensors. |
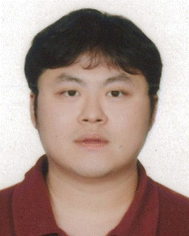 Yi-Cheng Tsai | Yi-Cheng Tsai received his BS and MS degrees from the Department of life science and the Institute of Biomedical sciences at Chang Gung University, Taiwan in 2007 and 2009, respectively. He completed his PhD study at the Graduate Institute of Biomedical Science, Division of Biochemistry, Molecular and Cellular Biology of Chang Gung University, Taiwan in 2014. Currently, he is a post-doctoral fellow in the Department of Power Mechanical Engineering at National Tsing Hua University, Taiwan. His research specialties are molecular biology, molecular diagnosis and nanobiotechnology. |
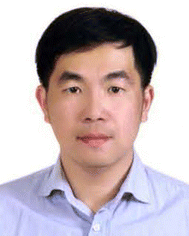 Chih-Hung Wang | Chih-Hung Wang received his B.S. and M.S. He obtained degrees from the Department of Plant Pathology and the Institute of Molecular Biology at National Chung Hsing University, Taiwan in 1989 and 1994, respectively. He completed his Ph.D. studies at the Institute of Basic Medical Science of National Cheng Kung University, Taiwan in 2005. He has worked at AsiaGen Corporation, exploring molecular diagnosis technologies. Currently, he is a postdoctoral fellow in the Department of Power Mechanical Engineering at National Tsing Hua University, Taiwan. His research specialties are molecular diagnosis, microbiology, and nanobiotechnology |
 Gwo-Bin Lee | Gwo-Bin Lee received his BS and MS degrees from the Department of Mechanical Engineering at National Taiwan University in 1989 and 1991, respectively. He received his PhD from the Department of Mechanical & Aerospace Engineering at the University of California, Los Angeles, USA in 1998. He is a Tsing Hua Chair Professor in the Department of Power Mechanical Engineering at National Tsing Hua University, Taiwan. His research interests are on microfluidics, bio-sensing, nanobiotechnology and its biomedical applications. He was an elected Fellow of NAI, ASME, RSC, IET, IEEE, AIMBE. He is a Member of European Academy of Sciences and Arts and a member of International Institute of Engineering. He is Editor-in-chief of Microfluidics and Nanofluidics. He is the recipient of the prestigious IEEE EDS Robert Bosch Micro and Nano Electro Mechanical Systems Award 2025. |
1. Introduction
Aptamers are short, single-stranded DNA or RNA molecules that can bind to a diverse range of targets, including small molecules (such as toxins & antibiotics), proteins, and even cells/tissues, with high specificity and affinity.1–4 This versatility stems from their ability to fold into unique three-dimensional (3D) structures, enabling them to interact with their targets in a highly specific manner (akin to antibody–antigen interactions5,6). The exceptional target-binding capabilities of aptamers have garnered significant scientific and biological interest, opening up applications in fields such as molecular diagnostics, therapeutics, and biosensing.7,8 Aptamers offer several advantages over traditional antibodies. They are far smaller in size (only 25–80 base-pairs (bp)), allowing for improved tissue penetration and pharmacokinetics.9–11 Unlike conventional antibodies, they lack immunogenicity, reducing the risk of adverse reactions,12 and they can withstand changes in temperature and humidity.13–15 Aptamers can be produced through cost-effective chemical synthesis at 100% accuracy, in contrast to the significant batch-to-batch variation caused by the complex animal-based production of antibodies.16,17 These advantages, alongside their versatility,18–20 have driven extensive research on the development of efficient aptamer selection methods for diverse applications over recent decades.4,21–23
The screening or selection of aptamers begins with a large pool of random nucleic acids (1012 to 1015 unique single-stranded DNAs (ssDNAs)) that is systematically screened to identify high-affinity and high-specificity probes to the target of interest.22,24 The “systematic evolution of ligands by exponential enrichment” (SELEX) process, originally developed in the 1990s, is the versatile and robust cornerstone of aptamer selection25,26 and involves binding, separation, and amplification (via polymerase chain reaction (PCR)) of screened ssDNA with the desired characteristics.5,20 However, SELEX suffers from lengthy, inefficient ssDNA library+target molecules incubations and ssDNA amplification processes. Moreover, incomplete removal of unbound or weakly-bound ssDNA, which may be amplified by the subsequent PCR and can compete with better candidates in subsequent screening rounds, are also critical issues. Moreover, the total number of the screening rounds may be at least 10–20, which may take weeks or months, and post-SELEX processes that require modification of screened aptamers can compromise aptamer integrity.
Microfluidic technology, which emerged in the late 1980s, is a multidisciplinary field that combines engineering, physics, chemistry, and biology.27–30 It enables precise manipulation and control of small fluid volumes (at nL–pL scale) within microchannels or other microfabricated devices (e.g., micropumps, micromixers, & microvalves).31–33 The advantages of microfluidics, namely precise control over flow rate, volume, and incubation time, have enabled the development of highly efficient and sensitive analytical screening platforms.34–36 The integration of microfluidic technology with aptamer selection methods, known as “microfluidic SELEX”, has revolutionized the field of aptamer development.37–43 These microfluidic features have also facilitated the lab-on-a-chip concept, integrating multiple laboratory functions onto a single, compact, automated device.44 Microfluidic SELEX offers advantages like reduced sample/reagent volume, improved target-binding capacity, enhanced selection stringency, and automated/parallel processing for improved throughput.45 These advantages have led to various microfluidic-based SELEX techniques that further improve efficiency and speed of aptamer screening.46–49 Originally, SELEX was used to select aptamers targeting proteins, eventually expanded to other targets like cells (cell-SELEX50–53) or tissues.39,54 Other variations include capillary electrophoresis SELEX (CE-SELEX),55–57 capture-SELEX,58,59 magnetic bead (MB)-SELEX,60–62 and many others.63–66 Notably, there are also methods like non-SELEX,67 or hydrogel for aptamer selection (HAS) method,68 applying other biomaterials and alternative platforms for overcoming amplification bias or non-specific binding issues to improve the efficiency on aptamer selection. Moreover, small molecules up to complex biological entities can be probed with aptamers screened through these methods.54,66 These targets and approaches have been demonstrated in microfluidic platforms in the past decades.
Previous reviews have highlighted the integration of microfluidic technology with SELEX,5,24,45,67,69–71 as well as the potential of aptamers to be used in diagnostic and therapeutic applications. Herein we focus instead on advancements in microfluidic SELEX and subsequent aptamer applications over the past 10 years. We first discuss how microfluidic technology has enhanced key steps of the SELEX process. Secondly, we review the diverse applications of aptamers enabled by microfluidic technology over the last five years. Finally, we discuss major challenges with, and a future prospective of, these technologies.
2. Microfluidic platforms and aptamer selection methodologies
2.1 The SELEX method
SELEX involves an iterative process of 1) binding a random oligonucleotide library to the target, 2) separating the bound sequences, and 3) amplifying them with PCR. This process gradually enriches the library with high-affinity and high-specificity probes as follows (Fig. 1).
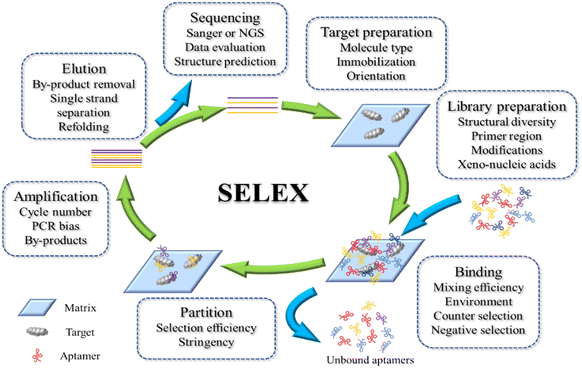 |
| Fig. 1 Schematic view of conventional SELEX with critical steps. Several important issues of each step are also listed. | |
1. Library preparation: generating a diverse pool of ssDNAs/RNA oligonucleotides.
2. Binding: incubating and mixing the oligonucleotide library with the target analytes.
3. Partition: separating bound from unbound oligonucleotides.
4. Elution and amplification: enrichment of the high-affinity aptamers.
5. Cloning, sequencing/synthesis, and characterization: analyzing the sequences of the aptamer candidates, followed by modeling/measuring their binding characteristics.
Miniaturized microfluidic platforms enhance the efficiency of aptamer selection by automating labor-intensive, time-consuming processes. When compared to conventional methods, microfluidic approaches are faster, require considerably less precious samples and expensive reagents, and can be automated with less human intervention. Furthermore, they allow for more precise control of reaction/washing conditions (e.g., pH, temperature, & shearing forces) such that more efficient screening for high-affinity and highly specific aptamers can be performed. Aptamer probes targeting proteins, viruses, bacteria, cancer cells, and even cancer tissue biomarkers are now available and will likely replace conventional antibodies.
2.2 Library preparation
The diversity of the initial oligonucleotide library is crucial for the success of aptamer selection. Aptamer libraries normally consist of random, unique DNA or RNA sequences (n = 1012 to 1015) flanked by fixed primer-binding sites. Such a large number of potential probes better ensures that at least some bind to the target of interest. The screened pool from each selection round then serves as the library for the subsequent one. Thus, preparing a high-quality candidate pool is critical. Although some original SELEX protocols used random RNAs,25,26 most SELEX libraries in use today are DNA-based, as DNA oligonucleotides are more stable and easier to synthesize.72,73 Generally speaking, microfluidic technologies do not play a role in library preparation, though several intriguing exceptions are highlighted below and more detail information are listed in Table 1.
Table 1 Microfluidic applied in SELEX steps
SELEX steps |
Targets |
Major microfluidic techniques |
Aptamer characteristics |
Duration |
Ref. |
Library preparation |
XNA polymerase |
DrOPs; droplet based microfluidics |
n/a |
n/a |
74–77
|
Ang2 |
AD-MAP; microarray |
K
d: 20.5 ± 7.33 nM (ABA1); Kd: 27.6 ± 5.92 nM (ABA65); Kd: 97 pM (bidentate ABA1-ABA65) |
n/a |
78
|
ssDNA |
Microdialysis |
n/a |
n/a |
79
|
ssDNA |
Droplet based microfluidics |
n/a |
n/a |
80, 81 |
Binding/partition |
Myeloperoxidase (MPO) |
Pro-SELEX; microfluidic sorting, magnetic bead-based, microstructures |
Primary selection: Kd: ranging 227 pM to 27.8 nM |
1 round (including 3 pre-concentrations) |
82
|
Quantitative selection with desired affinities: Kd: 166 pM (MPO-16, the best) |
Success rate in “great fit (<25% differ from desired Kd)” was between 20% and 50% |
var2CSA (variant of the plasmodium falciparum erythrocyte membrane protein 1 (PfEMP1) family); malaria parasite |
I-SELEX; inertial focusing; spiral microfluidic channels |
K
d: ∼14 nM (8.1–1); Kd: ∼84 nM (8.2–1) |
3–4 rounds |
83
|
IgE |
Microbeads, microstructure, hydrodynamic transfer, integration of affinity selection and amplification |
K
d: 83.9 nM (enriched pool of candidates) |
2 rounds, 2 h per round |
84
|
IgE |
Electrokinetic, hydrodynamic, pressure-driven |
K
d: 12 nM |
4 rounds/10 h |
37
|
IgA1 |
Electrokinetic, microbeads |
n/a |
4 h |
85
|
Patient's monoclonal immunoglobulins (M-Ig) |
Integrated, IMS; automated, strategic combination |
K
d < 50 nM; Kd: 36 nM (P3S10) |
12 h |
86
|
IgE; bisboronic acid (BA) in glucose |
Electrophoretic, microbeads |
Against IgE: Kd: 10 ± 2.1 nM (SIGE5); Kd: 18 ± 3.6 nM (SIGE7); |
(3 rounds + counter)/10 h |
87
|
Against BA-glucose: Kd: 2 ± 0.7 μM (SBG2); Kd: 1 ± 0.5 μM (SBG5) |
His-tag & IgE (his-tagged IgE) |
Microfluidic SELEX, magnetic bead-based |
K
d: 26.1 ± 4.9 nM (HisA1-T63); Kd: 14.3 ± 3.5 nM (IgEA-T35); LOD: 7.1 nM (dual-aptamer assay of his-tagged IgE in cell culture media in 10 min) |
n/a |
88
|
IgE |
Comparison among conventional, microfluidic chip, and full-chip SELEX |
K
d: 96.2 ± 9.4 nM (IGE1), Kd: 14.5 ± 1.4 nM (IGE1-T) |
Conventional/on-chip/full-chip: 4/1.5/1 days |
89
|
Plasmodium vivax lactate dehydrogenase (PvLDH) |
Electrodynamic microfluidic channel |
K
d: range from 31.4 to 97.3 nM; Kd: 31.4 ± 3.3 nM (L1); linear dynamic range: 25 fM−2 pM (R = 0.985) and 10–125 pM (R = 0.994); LOD: 7.8 ± 0.4 fM; |
3 h |
90
|
G4DNA |
GO, electrophoresis, G4DNA binding drug screening |
n/a |
n/a |
91
|
Prostate-specific antigen (PSA) |
Acoustofluidics; magnetic beads |
K
d: 0.7 nM (AS2) |
8 rounds |
92
|
IgE |
Surface acoustic wave-assisted (SAW); microfluidic chip |
K
d: 90.8±17.2 nM (full-length); Kd: 22. nM (truncated) |
3 rounds in 5 h |
93
|
Candida albicans (C. albicans) |
MACD (magnetic force magnetically activated continuous deflection); magnetic nanospheres; microfluidic chip |
K
d: 21.5 nM (C.al2); Kd: 7.9 nM (C.al3) – the best; Kd: 50.2 nM (C.al9); Kd: 9.2 nM (C.al10) |
6 rounds |
94
|
Adenosine |
Graphene oxide (GO)-coated magnetic nanoparticles; microfluidic chip |
K
d: 18.6 ± 1.5 μM |
∼2 h per round; 8 rounds |
95
|
Transcription factors (TFs); Pho4 & AtERF2 |
SELMAP (SELEX affinity landscape MAPping; microarray-like |
n/a |
3 rounds |
96
|
Lactoferrin |
PMM-SELEX (protein microarray microfluidic SELEX); microarray based; |
K
d: 1.04 ± 0.50 nM (Lac-6a); Kd: 0.63 ± 0.06 nM (Lac-3a); Lac-6a for detection: linear detection range: 0.78–50 μg mL−1; LOD: 0.39 μg mL−1 |
7 rounds |
97
|
Glycated hemoglobin (HbA1c); β-chain hemoglobin (Hb) |
Integrated microfluidic chip; magnetic bead |
K
d: 7.6 ± 3.0 nM (HbA1c aptamer); Kd: 7.3 ± 2.2 nM (Hb aptamer) |
5–7 rounds |
98
|
Ovarian cancer tissues |
Integrated microfluidic system (IMS); microfluidic chip for optimizing ion condition of selection buffer |
K
d: 266 ± 48 nM (H-45); Kd: 920 ± 436 nM (L-25) |
6 rounds |
40
|
Pseudomonas aeruginosa
|
Cell-SELEX; microfluidic flow cell |
Four primary aptamers selected:JN17, JN21, JN08, JN27; truncated tested: JN17. SH, JN21.SH, JN08.SH; chimeric tested: St21Lp17, St17Lp21, St08Lp17; all Kd ranged from 10–55 nM |
7 rounds |
99
|
Porcine aortic endothelial cells expressing KDR (PAE+) |
Cell SELEX; dielectrophoresis (DEP) and electrophoresis |
n/a |
3 rounds |
100
|
Ovarian cancer cell lines: TOV-21G; TOV-122D; BG-1; IGROV-1 |
Cell-SELEX; IMS |
2 to 4 aptamers for each cell line selected, Kd ranging from 1.8 nM to 201.3 nM; best affinity aptamer for each cell line: Kd: 1.8 nM (21G-2); Kd: 22.4 nM (112D-12); Kd: 1.3 nM (BG1-10); Kd: 10.5 nM (IGR5) |
5 rounds |
101
|
Colorectal cancer (CRC); colorectal cancer stem cell (CR-CSC) |
Cell-SELEX; magnetic beads; IMS |
K
d of eight aptamers ranging from 12.3 nM to 157.2 nM selected; particularly studied: for CRC: Kd: 28.5 ± 8.5 nM (HCT-17); Kd: 12.3 ± 4.5 nM (HCT-34); for CR-CSC: Kd: 27.4 ± 4.9 nM (CSC-16); |
5 rounds |
102
|
Ovarian cancer tissues |
Tissue-SELEX; on-chip SELEX; automated microfluidic SELEX |
K
d: 1348.0 nM (cTX-24); Kd: 129.2 nM (cTX-36); Kd: 178.0 nM (cTX-45) |
6 rounds (1st and 6th are negative selection) |
39
|
Ovarian cancer tissues |
Tissue-SELEX; phage display; microfluidic chip SELEX |
ssDNA aptamer: Kd: 53.8 ± 14.9 nM (Tx-01); Kd: 2.9 ± 0.8 nM (Tx-02); peptides: Kd: 4.8 ± 1.0 nM (Tp-01); Kd: 3.2 ± 1.5 nM (Tp-02) |
3 rounds |
54
|
Cholangiocarcinoma (CCA) cells; SNU-478, HuCCT-1 |
Cell-SELEX; microfluidic chip SELEX, IMS; strategic combination |
K
d: 29.0 ± 6.8 nM (SN8); Kd: 41.0 ± 5.1 nM (HN2); Kd: 37.0 ± 5.8 nM (HN16) |
6 rounds |
103
|
Elution/amplification or others |
Not specified |
Dual-MAS (dual-microfluidic amplified system); on-chip real-time PCR; microfluidic PCR device |
n/a |
30 minutes per round |
104
|
XBP1 pre-mRNA |
SPR-SELEX; surface plasmon resonance (SPR) |
K
d: 8 ± 1 nM (sRNA5); Kd: 22 ± 4 nM (sRNA1) |
6 rounds |
105
|
2.2.1 Screening for xeno-nucleic acid polymerases.
Synthetic nucleic acids, such as xeno-nucleic acids (XNA), have garnered attention due to their unique properties.106 One type, α-L-threofuranosyl nucleic acid (TNA), exhibits not only high stability but also the ability to form base pairs with DNA/RNA.74 A droplet-based optical polymerase sorting (DrOPS) method was developed to evolve polymerases capable of synthesizing TNA. DrOPS involves 1) encapsulating E. coli cells expressing polymerase variants in microfluidic droplets, 2) lysing the cells, and 3) sorting the droplets based on activity of the polymerases.75,76 Using DrOPS, researchers evolved a highly efficient TNA polymerase: Kod-RSGA.77 The DrOPS method has proven valuable for engineering polymerases that can synthesize diverse XNAs. The DrOPS method was not directly used for library preparation but could enhance polymerase evolution, expanding the diversity of nucleic acid candidates for SELEX and enabling new possibilities in synthetic genetics and biotechnology.
2.2.2 Pre-selected aptamers for enhancing dual-aptamer applications.
An “array-based discovery platform for multivalent aptamers” (AD-MAP) featuring microarray technology was used to enhance the SELEX process for dual-aptamer selection against proteins like angiopoietin-2 (Ang2);78 sequences from the enriched aptamer pool were first acquired through microfluidic selection and high-throughput sequencing. These sequences were next utilized to construct a custom array and perform parallel affinity assays to identify the strongest Ang2 aptamer (Kd = 20.5 ± 7.3 nM). This aptamer was then complexed with Ang2 to block its primary binding site, and the identical array was employed to identify secondary epitope-binding aptamers. The latter process is challenging, as conventional selection methods typically screen aptamers competing for the same binding site. AD-MAP effectively solved this issue, and Ang2 affinity for the other three isolated secondary aptamers were between 38 and 57 nM.78 These aptamer pairs were found to be linked to create bidentate probes with >200-fold higher affinity and dissociation constants as low as 97 pM.78 Microfluidic selection was used to integrate high-throughput affinity-based isolation and partitioning, producing enriched aptamer pools for downstream processing that promoted the dual aptamer applications.
2.2.3 ssDNA collection via microfluidics.
ssDNA recovery is critical for successive SELEX rounds, and a microfluidic dialysis device that collected ssDNA from double-stranded DNA (dsDNA) at high purity using 25 mM NaOH79 was shown to be rapid, highly sensitive, and cost-effective. At 0.2 mL h−1, the recovery rate was 24%, and the microfluidic approach outperformed manual procedures at higher NaOH concentrations (likely due to faster processing). Alternatively, Lee et al.80,81 produced ssDNAs using droplet-based microfluidics and synthetic, co-polymerizable oligo-microspheres, microspheres of 272 ± 7.1 μm (<3% variation80) and 150 ± 13 μm (30 per second with 8.5% variation81) were generated, and these particles were covalently attached to acrydite-modified DNA probes for efficient ssDNA amplification via asymmetric PCR. Tightly controlled flow rates and optimized channel geometries were critical for generating precisely sized microspheres; this high-ssDNA yield protocol is therefore suitable for automated SELEX and other applications (see Table 1). The collection of ssDNA using microfluidics is a common method to simplify and enhance intermediate SELEX rounds.
2.3 Binding and partitioning
Microfluidic platforms have been developed to improve efficiency in both the binding and partitioning steps,67 and so we discuss these steps together. The key challenge in aptamer selection is rapidly and efficiently isolating high-affinity candidates from a vast pool of sequences that are either in solution or immobilized.
2.3.1 Novel SELEX methods using fluidic forces.
Microfluidics offers straightforward hydrodynamic forces for efficient mixing and separation, enabling improved execution of the binding and partitioning steps. For instance, Chang et al.82 introduced Pro-SELEX, a method that could efficiently generate aptamers with desired binding affinities in a single selection round. This approach utilized particle display, emulsion PCR, and a microfluidic device with differential capture zones to sort aptamers based on their target-binding levels. The device demonstrated high capture specificity and could isolate aptamers with different binding affinities. The sorted aptamers were further sequenced and analyzed using the AptaZ algorithm, which compares sequences and selects candidates with the desired binding characteristics. For the human myeloperoxidase (MPO) protein, the first eight aptamers exhibited Z-scores that correlated linearly with binding affinities ranging from 227 pM to 28 nM,82 and experimental dissociation constant (Kd) values differed from the desired one by <25%. The strongest binding affinity was demonstrated by aptamer MPO-16 (Kd = 166 pM). Alternatively, I-SELEX instead employs centrifugal acceleration and Dean vortices in spiral microchannels to efficiently separate cell-bound aptamers from unbound nucleic acids.83 This high-resolution particle separation approach generated high-affinity aptamers 8.1–1 (Kd = 14 nM) and 8.2–1 (Kd = 84 nM) that could recognize var2CSA protein on malarial parasites and may have potential for use in malarial diagnostics, therapeutics, and vaccine development. These two cases demonstrate that innovative microchannel and/or microstructure designs, combined with hydrodynamic forces, can screen high-affinity aptamers.
2.3.2 Microbeads and microstructures in microfluidic aptamer selection.
Lin et al.37,84,85,87 successfully integrated selection and amplification into a single microfluidic SELEX device. Key innovations included using microbeads as the immobile phase, designing weir-like structures to confine microbeads in microchambers, and incorporating thermal control and nucleic acid transport methods to improve isolation efficiency. These improvements significantly reduced the time, cost, and labor associated with conventional SELEX. Similarly, Hilton et al.84 created a microfluidic chip with two microchambers, one for affinity selection and another for PCR, connected by a serpentine microchannel (Fig. 2a). The chip featured thin-film resistive heaters and temperature sensors for precise thermal control, with target molecules immobilized on microbeads packed in the selection chamber. This approach successfully isolated aptamer candidates with desired temperature-dependent binding characteristics, enhancing the Kd for human IgE to 84 nM after two selection rounds in just 4 h (significantly improving on conventional SELEX).
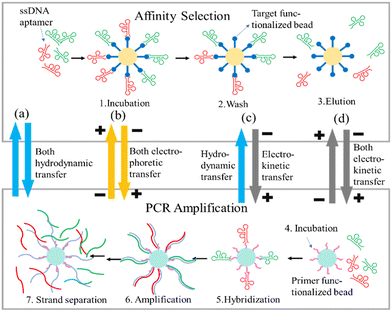 |
| Fig. 2 An overview of different approaches for transfer on microfluidic chips to enable SELEX cycles. The scheme shows that for on chip SELEX using two separated chambers for affinity selection and PCR amplification. In Lin's group,37,84,85,87 several works utilized different ways for the aptamers transfer between two chambers within the microfluidic chip. (a) Hilton et al.84 used both hydrodynamic driven transfers (light blue arrowheads). (b) Kim et al.87 displayed both electrophoretic transfer through gel filled channels (yellow arrowheads). (c) Olsen et al.37 in this work used hydrodynamic (light blue arrowhead) plus electrokinetic (gray arrowhead) ways on transfer. (d) In another work, Olsen et al.85 adopted both electrokinetic transfers (gray arrowheads) instead. These critical considerations can increase successful rate on enabling on-chip automated SELEX. The figure concept was adapted from Fig. 1 in ref. 37. | |
Alternatively, Kim et al.87 presented a microfluidic approach that integrated the isolation of DNA aptamers through electrophoretic oligonucleotide manipulation (Fig. 2b) within a single chip, allowing the single selection to be completed within ∼10 h. The aptamer candidates demonstrated robust binding affinities, with equilibrium dissociation constants of 18 nM or better, showing the technique's versatility in selecting aptamers for both surface-bound and solution-borne targets. Moreover, Olsen et al.37,85 introduced two new microfluidic SELEX technologies to improve aptamer selection efficiency and specificity. The first used electrokinetic and hydrodynamic manipulation in a microfluidic device to immobilize targets and precisely control the selection environment to isolate an IgE aptamer with a Kd of 12 nM after four rounds of selection in 10 h37 (Fig. 2c). In the second study, free-solution electrokinetics simplified the SELEX process by actualizing aptamer selection in a continuous flow system85 (Fig. 2d). The enriched pool of aptamers bound IgA more strongly than the initial random library. This method simplified and accelerated selection, reducing non-specific interactions.
Furthermore, Olsen et al.86 presented a microfluidic device for the rapid isolation of aptamers targeting patient- and tumor-specific monoclonal immunoglobulins in multiple myeloma patients. This device integrated SELEX, counter-selection, and PCR within a closed fluidic loop, reducing the time required per round. After six rounds, an aptamer was identified with a binding affinity of 36 nM, demonstrating the promise of this approach for personalized diagnostics. Similarly, Meng et al.89 compared three SELEX strategies for IgE aptamer isolation. Microfluidic SELEX achieved higher enrichment (4236 counts per million) than conventional SELEX (25 counts per million) after four rounds. The full-chip SELEX method completed isolation in ∼12 h; the selected aptamer, IGE1, exhibited high affinity for IgE (Kd = 96 ± 9.4 nM).89 Microfluidic SELEX reduced reagent consumption, leading to lower costs while enhancing selection stringency and binding. While full-chip SELEX offers integration and automation benefits, chip-selection SELEX is simpler and often provides better PCR efficiency. Finally, another microfluidic SELEX device enabled a 10 min dual-aptamer sandwich assay that detected tag-fused recombinant proteins88 His-tag and IgE by aptamers screened in 3 and 4 rounds, respectively88 within 2 days; the limit of detection (LOD) of the former was 7 nM,88 and the aptamer-functionalized surfaces could be thermally regenerated for up to 20 uses, greatly reducing costs. Collectively, these findings indicate that microfluidic SELEX is a more efficient, cost-effective, and time-saving alternative to traditional SELEX.
2.3.3 Enhancing screening efficiency by introducing additional force(s).
To further enhance SELEX, researchers have explored integrating additional physical forces within microfluidic platforms. For instance, Chung et al.90 presented a novel method using an electrodynamic microfluidic channel device for rapid aptamer generation. The channel walls were modified with poly-benzoic acid to facilitate the covalent attachment of the malaria protein lactate dehydrogenase (PvLDH). A random pool of ssDNAs was incubated in the device under an alternating current electric field for 60 min, followed by a washing step to remove unbound DNA. The process achieved a high partitioning efficiency of 1.7 × 107 measured by qPCR, and the resulting aptamers exhibited dissociation constants ranging from 31 to 97 nM. The aptamer-modified sensor effectively detected PvLDH concentrations from 0 to 2 pM at a LOD of only 7.8 ± 0.4 fM.
Alternatively, a microfluidic device was developed to rapidly screen and isolate natural compounds that specifically bind G-quadruplex (G4DNA) using a SELEX-like procedure.91 This approach integrated an aptamer-carboxyfluorescein/graphene oxide energy transfer optical sensor with on-chip electrophoretic separation, allowing for efficient and rapid screening of active ligands such as daidzein, berberine hydrochloride, jatrorrhizine hydrochloride, fangchinoline, and a new potential G4DNA active drug, jujuboside A, while demonstrating high sensitivity and effective electrophoretic separation. Microfluidic platforms have also utilized acoustophoresis, which employs acoustic waves to control particles, enabling simultaneous washing and separation of target-bound ssDNA aptamers in continuous flow.92 This enhances efficiency and specificity, as a sheath flow aligns particles along the channel walls while acoustic forces direct target-bound particles to the center for collection. The continuous flow design improved throughput, and seven aptamers binding prostate-specific antigen were identified; the superior Kd was found to be only 0.7 nM. Integrating acoustophoresis with next-generation sequencing further could also accelerate aptamer selection.92 Additionally, a surface acoustic wave-enhanced microfluidic chip for aptamer screening demonstrated improved mass transfer, resulting in more effective binding and selection of high-affinity aptamers, including a DNA aptamer that specifically binds IgE within three rounds over 5 h, with a Kd of only ∼23 nM.93
2.3.4 Introducing magnetic forces into microfluidic SELEX platforms.
The use of magnetic forces in microfluidic SELEX platforms provides advantages for enhancing aptamer screening efficiency. Magnetic separation via superparamagnetic beads enables more efficient washing and partitioning of bound aptamers from unbound ones, improving enrichment. For instance, a magnetically activated continuous deflection microfluidic chip facilitated dynamic selection in continuous flow, allowing simultaneous binding and separation of aptamers.94 This magnetically activated continuous deflection system pumps targets, a DNA library, and a wash buffer through the chip, with a magnetic field guiding the target-bound sequences. During washing, low-affinity aptamers are removed, and the chip also allowed for the tuning of selection stringency by adjusting target concentrations. Within six rounds of selection, this approach successfully identified a high-affinity aptamer for Candida albicans with a 7.9 nM dissociation constant.94
Another study reported a microfluidic SELEX method using graphene oxide-coated magnetic nanoparticles (GOMNPs) to select adenosine-specific aptamers.95 The ssDNA library was incubated with GOMNPs, and adenosine was introduced, allowing specific aptamers to bind. Applying a magnetic field separated the GOMNPs with bound aptamers from unbound ssDNAs. Bound aptamers were then eluted and amplified, and the process was repeated to enrich for high-affinity ones. The microfluidic device enabled efficient, rapid (2 h cycle−1) selection, identifying adenosine-specific aptamers with a Kd of ∼20 μM (comparable to existing aptamers). Similarly, another study presented a microfluidic system for on-chip SELEX to select aptamers specific to glycated hemoglobin and total hemoglobin.98 The system automated multiple SELEX steps, reducing time and reagent use compared to traditional methods. The process began with a DNA library incubated with target protein-coated magnetic beads (MBs). High-affinity aptamers were bound to the MBs, which were then separated via a magnet, and the bound aptamers were amplified by PCR. The study successfully selected glycated hemoglobin (HbA1c)- and β-chain hemoglobin (Hb)-specific aptamers with binding affinities of 7.6 ± 3.0 and 7.3 ± 2.2 nM, respectively.
2.3.5 Advanced SELEX.
“SELEX affinity landscape mapping” (SELMAP) enables high-throughput measurement of transcription factor-binding affinities to diverse DNA sequences.96 Briefly, a dsDNA library was loaded into the chip, and unbound DNAs were degraded with DNase; bound DNAs were released via proteinase K. The recovered oligonucleotides were then PCR-amplified. The approach allowed simultaneous analysis of up to 16 proteins, minimizing reagent use while enhancing throughput. SELMAP's rapid nature, versatility, and precise fluid control make it a powerful tool for understanding gene regulation.96 Additionally, a SELEX method integrated a protein microarray with a microfluidic chip, enhancing the efficiency and speed of SELEX while allowing quantitative monitoring of aptamer enrichment.97 This approach successfully identified five high-affinity aptamers for lactoferrin that were specific enough for biosensing and diagnostics.97
2.3.6 Cell- and tissue-SELEX by microfluidic platforms.
Microfluidic platforms have been used for cell- and tissue-SELEX, which allow the selection of aptamers that recognize native target proteins on cells or tissues.100,101,107,108 For example, DNA aptamers specific for Pseudomonas aeruginosa (Kd = 10–55 nM) were demonstrated to effectively bind live bacterial cells.99 Another study reported a microfluidic chip that combined dielectrophoresis and electrophoresis100 to effectively remove nonspecifically bound oligonucleotides and found that increasing the flow rate during successive SELEX rounds enhanced selection pressure, yielding aptamers with higher affinities and specificities to the target HEK293 cells.
Another study has reported a microfluidic system for automated on-chip cell-SELEX, which rapidly selected high-affinity aptamers specific to different ovarian cancer (OvCa) cell lines.101 The system reduced the selection process from 22 to 5 rounds, identifying three aptamers with dissociation constants of 1.3–8.3 nM (comparable to antibodies). Similarly, a compact microfluidic system was reported for aptamer selection against colorectal cancer and colorectal cancer stem cells.102 This “micro-total-analysis-system” efficiently identified eight aptamers, including three with high affinities (Kd = 12–29 nM), and the selective cell capture rate difference exceeded ∼30%. These findings demonstrate the effectiveness of MB-based microfluidic platforms for isolating cancer-specific aptamers with the potential for early diagnosis and targeted therapy. Moreover, an integrated microfluidic system (IMS) capable of automatically identifying aptamers specific to cholangiocarcinoma cells was developed, requiring only 6 rounds of cell-SELEX compared to the typical 15–20 rounds.103 This system successfully screened three high-affinity aptamers-HN2 (41 ± 5.1 nM), HN16 (37 ± 5.8 nM), and SN8 (29 ± 6.8 nM) that exhibited significant selectivity towards the target cell line.
An innovative microfluidic platform combined tissue-SELEX and phage display technology to rapidly screen cancer affinity reagents for OvCa.54 This system used a micromixer to wash out weakly bound ssDNA and phages, resulting in selected aptamers and peptides with Kd between 3 and 60 nM (all comparable to typical antibody affinities). The optimal incubation time was 30 min, reducing screening time from 2–3 days to only 5–6 h. The microfluidic chip, which fitted 2 cm tissue slides, showed potential for identifying personalized cancer biomarkers. The same group39 developed an IMS for efficiently selecting aptamers targeting OvCa tissues by integrating multiple microdevices. This system combined reagents effectively with a mixing index >90% at 50 kPa gauge pressure and a 1 Hz mixing frequency of the micromixer in 4 s. The system also performed 20 PCR cycles in 60 min. Six rounds of tissue-SELEX successfully identified an aptamer with a Kd of 129.2 ± 24.8 nM, indicating strong affinity for OvCa tissues. Moreover, Lin et al.40 reported another automated microfluidic SELEX system with optimized aptamer selection conditions for tissue in a unique IMS that automatically mixed Ca2+, Mg2+, Na+, and K+ solutions to create selection binding buffers. Ion species and concentrations could be fine-tuned, and an optimization-SELEX chip improved buffer composition and solution transport, reducing reagent use and human intervention. H-45 and L-25 aptamers passed six optimization-SELEX rounds, and the former exhibited strong affinity (266 ± 48 nM) and specificity for OvCa tissues.
2.4 Elution and amplification
Elution is often combined with other steps, namely amplification. For nucleic acid aptamers, heat is commonly used to elute via denaturing, while protein-based SELEX uses competing ligands or buffer exchange since protein could be fragile. After elution, aptamers are amplified via PCR for subsequent selection rounds. For instance, a study introduced a dual-microfluidic amplified system that integrated real-time PCR detection and large-volume PCR amplification within an IMS,104 enhancing PCR and SELEX efficiency. The system split the enriched library into two streams, and qPCR provided concentration and diversity data in only 30 min with minimal contamination and sample loss by using one stream, while another was amplified for next round selection. It is worth noting that characterizing the selected aptamers is critical yet normally follows SELEX. For example, Dausse et al.105 developed a microfluidic platform featuring recovery with surface plasmon resonance (SPR), allowing simultaneous selection and evaluation. The study identified RNA aptamers forming stable loop–loop complexes (Kd = 8 nM), and high-throughput sequencing revealed candidates binding 79 times faster than those with fully complementary loops. A comprehensive table for providing an overview of the advantages and disadvantages of various microfluidic SELEX techniques and quick comparisons is listed in Table 2.
Table 2 Overview of microfluidic SELEX techniques
Microfluidic technique |
Advantages |
Disadvantages |
Typical applications |
Pro-SELEX |
- Single-round selection |
- Requires complex microfluidic devices |
Protein targets (e.g., MPO) |
- High capture specificity |
- Needs specialized algorithms for analysis |
I-SELEX |
- Efficient separation of cell-bound aptamers |
- Limited to cell or particle-based targets |
Cell surface proteins (e.g., var2CSA) |
- High-resolution particle separation |
- May require multiple rounds |
Microbead-based SELEX |
- Integration of selection and amplification |
- Potential for non-specific binding to beads |
Protein targets (e.g., IgE, IgA) |
- Reduced time and reagent consumption |
- May require optimization of bead size/type |
Electrophoretic SELEX |
- Continuous flow system |
- Limited to charged molecules |
Small molecules and proteins |
- Reduced non-specific interactions |
- May require careful buffer optimization |
Acoustic wave-enhanced SELEX |
- Improved mass transfer |
- Requires specialized acoustic equipment |
Proteins and cells (e.g., PSA, IgE) |
- Simultaneous washing and separation |
- May have limitations with certain sample types |
Magnetic bead-based SELEX |
- Efficient target immobilization and separation |
- Possible magnetic bead aggregation |
Proteins, cells, and small molecules |
- Compatibility with various targets |
- May require optimization of magnetic field strength |
3. IMS for SELEX-based aptamer selection
3.1 Challenges in aptamer selection and SELEX
The primary objective of SELEX is to discover aptamers that exhibit both high affinity and specificity, and this can be achieved via SELEX66 and alternative67 methods (Table 3). The former is lengthy, and the selected ssDNAs must confirm to rigid specificity and affinity requirements. The prolonged operational duration represents the most significant bottleneck, and researchers are exploring methods to increase efficiency. One such approach is microfluidic SELEX, which can automate and accelerate the selection process. By reducing the number of cycles and enhancing the interaction between aptamers and target molecules, microfluidic SELEX offers a promising solution to the time-consuming, labor-intensive nature of traditional methods. Additionally, advancements in bioinformatics are aiding in the design of aptamers with improved affinity and specificity, further streamlining the selection process.
Table 3 Current aptamer selection methods
Non-SELEX67 |
1. In silico aptamer design |
2. High-throughput screening |
3. Phage display method |
4. Ribosome display method |
5. CE based (NECEEM, ECEEM) |
6. Competitive |
7. Centrifuge based |
SELEX66 |
1. Non-chip SELEX |
a. Conventional SELEX |
b. Bead-based SELEX |
c. Plate-based SELEX |
d. In silico and computational SELEX |
2. Chip SELEX |
a. On-chip SELEX |
b. Lab-on-a-chip SELEX |
c. Microfluidic SELEX |
i. Droplet-based SELEX |
ii. Continuous-flow SELEX |
iii. Paper-based SELEX |
iv. Digital SELEX |
d. Integrated microfluidic devices for SELEX |
i. Simple SELEX |
ii. Complex SELEX |
iii. Conditional SELEX |
iv. In vivo-like SELEX |
3.2 IMS for aptamer selection
3.2.1 Benefits of integrated microfluidic SELEX systems.
IMS present numerous benefits over conventional SELEX methods. Firstly, IMS facilitate automated, rapid, and continuous (rounds of) SELEX,40 thereby enhancing the identification of candidate aptamers. Furthermore, should the initially selected aptamer fail to satisfy the criteria during subsequent validation, a new selection process can be swiftly commenced. Prior IMS methods have employed straightforward, pressure-driven, and electrokinetic transport mechanisms to facilitate the interactions between ssDNA libraries and target molecules.87 Furthermore, a novel, integrated SELEX method has been established that employs target-bead affinity selection and facilitates automated liquid transport to isolate specific aptamers without the need for off-chip procedures. Other IMS feature MBs, immobilization of affinity agents onto substrates, employment of external devices (e.g., pneumatic, electrical, or magnetic forces) to manage mixing and movement of substrates, automation of binding, washing, and enrichment, and the implementation of various SELEX methodologies, which will be reviewed in the following sections.
3.2.2 MBs in aptamer selection.
Aptamers can be conjugated to a variety of materials, such as MBs,101 gold,109 nanogold,110 polymeric resins,111 and polystyrene,112 for application in on-chip diagnostics. MBs with surface-modifiable functional groups also possess the ability to bind nucleic acids, proteins, antibodies, biomolecules, and cells, rendering them suitable for the selection of aptamers.62 The small, mobile nature of MBs facilitate their movement within fluids, allowing them to traverse different chambers across various processing stages. Furthermore, their significantly greater surface compared to the flat surfaces of chambers enhances the likelihood of successful capture of candidate ssDNA. Therefore, in many integrated microfluidic chips (IMC), MBs are commonly employed as the target carrier for SELEX selection of specific aptamers.
3.3 IMC SELEX types
The IMC depicted in Fig. 3 can be categorized to four types: simple SELEX, complex SELEX, conditional SELEX, and in vivo-like SELEX. The former entails immobilizing affinity agents onto a planar substrate and employing fluid flow to facilitate the processes of binding, washing, and enrichment for the selection of candidate ssDNAs. This process is commonly referred as “positive selection”. Furthermore, IMC may further incorporate MBs to augment the likelihood of interactions between ssDNA and affinity agents, thereby increasing the yield of captured aptamers. Conventional SELEX usually involves >20 rounds of selection, while simple, on-chip SELEX may require only 4–5.36,113,114
 |
| Fig. 3 Aptamer selection methods in integrated microfluidic chip. (A) The core of IMS is simple SELEX, commonly referred to as positive SELEX which already integrated necessary steps for SELEX cycles. (B) Complex SELEX combines positive and negative SELEX to enhance aptamer performance. (C) Conditional SELEX varies the sequence and number of positive and negative SELEX to improve specificity and affinity. (D) More complex strategies, like in vivo-like SELEX, enable aptamer screening in ambient environments, isolating aptamers that may retain functionality in complex conditions, such as therapeutic applications. | |
IMC can employ external devices like pneumatic, electrical, or magnetic forces62,115,116 to manage the mixing or movement of substrates (or MBs) within the fluid. This can occur in a single or back-and-forth manner to speed up reaction times or facilitate ongoing selection processes (discussed in detail below). The simple SELEX method relies on incubation of probe-coated MBs (or planar substrates) with random ssDNA libraries (Fig. 3A). The target-bound MBs are then collected via magnet, and unbound ssDNAs are removed. The collected ssDNAs are enriched via PCR. These steps constitute a single positive selection round. After several rounds, high-affinity aptamer candidates are obtained (Fig. 3A). Consequently, microfluidic SELEX chips continuously and automatically perform multiple rounds of SELEX for screening various specific aptamers, targeting molecules such as Hb98 and C-reactive protein.36
3.3.1 Complex SELEX.
To enhance specificity, “negative selection” may be employed. In this process, structurally analogous molecules capture ssDNAs with diminished specificity, while aptamers exhibiting higher specificity remain in the supernatant after separation.117 By implementing multiple cycles of positive and negative selection, more specific aptamers with high affinity can be obtained vs. via positive selection alone (i.e. simple SELEX) (Fig. 3B).118,119 Incorporating of negative selection could improve aptamer specificity by removing non-specific binders, decreasing off-target interactions, and increasing affinity in complex environments. However, it adds complexity and screen time to the selection process and may exclude valuable aptamers due to over-stringency. As a result, while it improves target specificity, careful optimization is required to avoid restricting the diversity of the aptamer pool.
3.3.2 Conditional SELEX.
ssDNAs are susceptible to conformational changes induced by ion type and concentration, pH, temperature, and other factors (Table 4).120–129 To enhance stability of the screened aptamers, several strategies for chemical and structural modifications can be employed. These include (1) chemical modifications,130–132 such as 2′-O-methyl RNA, locked nucleic acids, or phosphorothioate linkages, which can improve the stability of aptamers by increasing their resistance to nuclease degradation and enhancing binding affinity; (2) PEGylation,133 which increases molecular weight, reduces renal clearance, and enhances stability in fluids; (3) backbone modifications,134,135 where the sugar-phosphate backbone of aptamers is altered using peptide nucleic acids or morpholinos (thereby significantly enhancing resistance to enzymatic degradation & improving stability); (4) aptamer dimerization and multi-merization,136 which involves linking multiple aptamers together and can enhance binding affinity and stability through cooperative effects; and (5) G-quadruplex stabilization,137 which contributes to the structural integrity and functionality of aptamers (as examined through post-SELEX processing). Future developments in bioinformatics, crystallography, and 3D structure analysis are anticipated to facilitate more accurate assessments of aptamer-target interactions, thereby improving the selection process.
Table 4 Factors influencing ssDNA conformation
Factors |
Effects on conformation |
Ref. |
Ion species and concentration |
Stabilizes or destabilizes secondary structures |
62
|
1. Cations (e.g., Mg2+, Na+, K+) |
These stabilize the negative charges on the phosphate backbone, facilitating folding and the formation of secondary structures like hairpins or loops |
2. Anions |
Influence nucleic acid stability and folding through ionic interactions |
pH |
Alters base protonation, affecting hydrogen- bonding |
98
|
1. Acidic pH |
To protonate nucleobases, altering hydrogen- bonding patterns and thus affecting secondary structure formation |
2. Alkaline pH |
Lead to deprotonation, affecting base-pairing and possibly leading to strand denaturation or misfolding |
Temperature |
Denatures or stabilizes secondary structures |
36
|
1. High-temperature |
Causes denaturation of secondary structures, leading to a more linear conformation |
2. Low-temperature |
Promotes the formation of secondary structures through stable base-pairing |
Molecular crowding |
Promotes compact folding through excluded volume effects |
118
|
Solvent conditions |
Affects hydration and hydrogen bonding |
119
|
1. Water activity |
Lower water activity can stabilize certain nucleic acid structures by reducing the hydration shell |
2. Organic solvents (e.g., ethanol, DMSO) |
Disrupt hydrogen bonds and affect the overall stability of the secondary structure |
Base sequence and composition |
Influences base-pairing and structural stability |
120
|
1. GC content |
Higher GC content typically increases stability due to stronger hydrogen-bonding |
2. Sequence repeats and palindromes |
Lead to the formation of specific secondary structures such as hairpins or cruciform structures |
Secondary structure formation |
Hairpins, loops, bulges, and G-quadruplexes |
121
|
1. Hairpins, loops, and bulges |
These structures form based on complementary base-pairing within the strand and are influenced by the factors mentioned above |
2. G-Quadruplexes |
These are formed by guanine-rich sequences and are stabilized by the presence of specific cations like potassium |
Chemical modifications |
Alter base pairing and backbone flexibility |
122
|
1. Methylation or alkylation |
Alter base-pairing properties and overall conformation |
2. Phosphorothioate linkages |
These modifications in the backbone can influence the flexibility and binding interactions of nucleic acids |
Binding proteins or ligands |
Induce specific conformations |
123
|
Mechanical forces |
Modify structure through stretching or twisting |
124
|
SELEX must be performed under diverse conditions to ensure that aptamers screened in vitro are effective/accurate in real life scenarios. IMC allows for automation and concurrent performance evaluation under various conditions, supporting the continuous and condition-specific SELEX of aptamers that are customized to meet the demands of subsequent assays. Conditional SELEX, which involves alternating positive and negative selection as well as the incorporation of various conditions throughout the selection process, can be further refined through the application of broad or condition-specific positive and negative selection strategies. For instance, IMC can automate and control the types and concentrations of ions (e.g., Mg2+, Na+, K+, Ca2+) and other critical factors within each chamber. This capability is particularly important given the differences between the microenvironments of cancer and normal cells, as a dedicated IMC facilitated the selection of a specific aptamer for ovarian cancer (Fig. 3C).40 Methodologies that utilize the structural-switching characteristics of aptamers in response to varying environmental conditions, namely different ion concentrations, have been designed to differentiate influenza A from influenza B viruses.138
3.3.3
In vivo-like SELEX.
The complex nature of natural environments means that reliance solely on conditional SELEX may be insufficient for the identification of aptamers that meet the diverse conditions necessary for clinical detection or therapeutic applications. For instance, the tumor micro-environment139 differs markedly from that of normal cells. Due to rapid proliferation, altered metabolism, and metastatic behavior of cancer cells, factors such as pH, ion concentrations, lactate levels, and the presence of associated cells and molecules are significantly modified.
Numerous microfluidic investigations employing tumor-on-a-chip technology have concentrated on the advancement of anti-cancer pharmaceuticals.140 The use of aptamer-containing microfluidic chips for detecting circulating tumor cells (CTCs),141,142 exosomes,143,144 and modeling 3D environments145,146 have also become an emerging trend in cancer diagnostics.147,148 Epigenetic biomarkers have also been employed in liquid biopsies to facilitate the early diagnosis and prognosis of cancer.149 For instance, a microfluidic system was utilized for the screening of aptamers in conditions that simulated diverse tumor microenvironmental conditions in patient blood (Fig. 3D), and highly specific, methylated BRCA1 and BRCA2 aptamers suitable for clinical applications were identified through an in vivo-like SELEX approach.150
3.4 Summary
Aptamer-based microfluidic systems represent a significant advance in clinical diagnostics, offering high specificity, sensitivity, and versatility for identifying a wide range of biomarkers. These technologies are poised to play a crucial role in the future of clinical diagnostics, providing tools for early detection, personalized treatment, and continuous monitoring of cancer and other diseases. It is envisioned that advancement of microfluidic SELEX may open up great opportunities for screening and applications of aptamers.
Various SELEX methodologies have distinct advantages and limitations. Conventional SELEX offers versatility across various targets but faces challenges with long selection times and PCR-induced biases. Magnetic bead-based SELEX improves binding efficiency and microfluidic integration but requires optimization for specificity. Droplet-based SELEX enables high-throughput screening with minimal reagent use, particularly effective for small molecules and proteins, though complex device microfabrication may limit its practical use. Capillary electrophoresis SELEX excels in precise complex separation but faces issues such as scalability challenges. On-chip SELEX reduces selection rounds and automates processes, while conditional SELEX improves clinical relevance by incorporating physiological conditions. Researchers can select optimal methods based on target complexity, application needs, and available resources.
4. Non-SELEX
4.1 Alternative methods for aptamer selection
4.1.1 Introduction.
Alternative techniques have emerged recently that address limitations such as PCR in SELEX, which was referred as “non-SELEX” to distinguish them from amplification-based approaches associated with conventional SELEX. By avoiding PCR, amplification biases caused by varied template sequence composition preferences may be avoided.17,151 Additionally, there is no need to convert PCR products back to ssDNA, and integration into IMS is simplified on account of the reduced need for precise thermal control, thus shortening the total SELEX time.17,151 Non-SELEX methods generally utilize high-resolution partitioning techniques like CE and/or implementing microfluidics to directly isolate aptamers based on their binding affinity. Therefore, it can enhance efficiency and reduce time and costs.45,152,153 Non-SELEX methods can significantly improve selected pool affinity in just hours, compared to days or weeks for SELEX.154 Additionally, strategies to minimize or eliminate fixed primer regions have been developed to reduce non-specific binding and false positives.155,156 These advancements, along with high-throughput analyses, are expected to accelerate the development of aptamers for biosensing, diagnostics, and therapeutics47,67,157 (Fig. 4). Because of the lack of amplification cycles, non-SELEX methods risk missing low-abundance but high-affinity aptamers. Furthermore, the lack of iterative selection limits the ability to differentiate between high- and low-affinity binders, lowering the sensitivity of the screening process. Despite the efficiency of SELEX, its reliance on amplification might introduce biases and could limit throughput of the screening. Alternatively, Non-SELEX methods address these challenges by utilizing direct selection strategies.
 |
| Fig. 4 Schematic overview of non-SELEX methods. (a) Working principle of non-equilibrium capillary electrophoresis of equilibrium mixtures (NECEEM). (b) Overview of centrifuge-based method, the targets should be able to separate from free diffusing library. (c) Competition based method overview, including systematic evolution of ligands by competition-enhanced ligand selection (SELCOS) and competition-enhanced ligand selection (ComELS). | |
4.1.2 CE-based non-SELEX.
CE-based non-SELEX technologies were developed to select aptamers without amplification.56,158,159 One such method, “non-equilibrium capillary electrophoresis of equilibrium mixtures,”152,154 separated oligonucleotide-target complexes from free oligonucleotides and reduced the selection period to an hour. To overcome the limitations of SELEX, including amplification bias and challenges with RNA/XNA libraries, the development of Non-SELEX alternatives has been reported.49,157,160 Other methods include MB-assisted screening,161 which also offers more streamlined and cost-effective selection.
4.2 Non-SELEX methods: applications and advancements
4.2.1 Non-SELEX in mobile formats.
Aptamer selection can be conducted in either immobilized or non-immobilized manners for non-SELEX;67 each has distinct advantages and disadvantages. As an example of the latter, fluorescence anisotropy and CE enabled isolation of high-affinity DNA aptamers targeting tau protein isoforms at a 28 nM detection limit,162 without the need for PCR. Another selection pressure-controlled CE approach yielded aptamers for holo-transferrin (H-Tf) and platelet-derived growth factor-BB with Kd of 50 and 81 nM, respectively.163 This method featured one round of pressure-controllable selection that relied on high-efficiency separation, single round selection, and target competition with controllable pressure via balanced or dominant protein competition. Alternatively, centrifugation-based partitioning rapidly selected E. coli-specific aptamers with Kd values as low as 4 nM.164 This method isolated aptamers by serially removing unbound DNA from a mixture of bacteria and DNA libraries via centrifugation, followed by separation and cloning of target-bound DNA. A similar approach with E. coli involved a dual-aptamer colorimetric assay and was characterized by a 10 CFU mL−1 detection limit.165 Finally, another centrifugation-based approach including positive and negative selection stages isolated aptamers targeting airborne Citrobacter braakii with Kd values of 16 and 27 nM, in liquid and air, respectively.166 (Table 5).
Table 5 The research works of non-SELEX methods reviewed in the past five years
Reference |
Main method |
Target |
Aptamer performance |
Tapp et al.167 |
Competition-enhanced ligand selection (CompELS) |
PlanarAu, non-biological targets |
K
d = 0.56 nM for high affinity aptamer |
Lisi et al.162 |
Non-SELEX-based aptamer selection, fluorescence anisotropy |
Tau proteins (t-441, t-381, t-352, t-383) |
K
d: 13 ± 3 nM for t-441 aptamer, 116 ± 6 nM for t-381 aptamer, 84 ± 6 nM for t-352 aptamer, 49 ± 4 nM for t-383 aptamer; LOD: 28 nM for t-441, 3.2 nM for t-381, 6.3 nM for t-352, 22 nM for t-383 |
Sullivan et al.168 |
Secondary structure analysis of DNA aptamers |
Gold nanorods |
Multiple secondary structure families identified |
Kushwaha et al.169 |
Systematic evolution of ligands by COmpetitive selection (SELCOS); competitive non-SELEX for rapid aptamer enrichment |
Influenza virus subtype-specific aptamers |
High selectivity, Kd = 82 pM for H1N1 and 88 pM for H3N2 |
Kim et al.164 |
Non-SELEX-based aptamer selection |
Escherichia coli, specific bacterial strains |
K
d: 3.9 nM for 20–5 aptamer, 8.0 nM for 20–7 aptamer, 10.1 nM for 20–10 aptamer; specific binding to E. coli, negligible binding to other bacteria |
Wu et al.170 |
Flow-cell-based massively parallel aptamer characterization |
Base-modified DNA aptamers |
K
d: 3.3 nM for aptamer VEGF-4, 16.9 nM for aptamer SL2B; screening of aptamers in human serum conditions |
Yang et al.163 |
One-round pressure-controllable aptamer selection |
Human holo-transferrin, PDGF-BB |
K
d: 81 nM for PDGF-BB, 50 nM for holo-transferrin, improved specificity and affinity using competitive pressure selection |
Biyani et al.171 |
Competitive selection for DNA aptamer inhibition of CYP24 |
CYP24A1 (cancer-related enzyme) |
Inhibition: 41% of CYP24 endogenous activity |
Liu et al.165 |
Non-SELEX-based aptamer selection |
Escherichia coli
|
K
d: 101.76 nM for aptamer 2–17–2, LOD: 10 CFU mL−1, specificity: excellent selectivity over other bacterial strains such as S. aureus, V. parahaemolyticus, and L. monocytogenes |
Parashar et al.172 |
Non-SELEX-based magnetic bead approach |
β-Casomor-phin-7 peptide |
K
d: 28.93 ± 0.783 nM for aptamer Seq. ID no. 3 |
Jeong et al.166 |
Non-SELEX-based aptamer selection |
Citrobacter braakii (airborne bacteria) |
CB-5 aptamer: Kd: 16.42 nM in liquid culture, 26.91 nM in aerosolized samples; LOD: for liquid culture, 1.8 × 103 CFU mL−1, for aerosol 1.6 × 103 CFU mL−1; high specificity for C. braakii |
Lozoya-Colinas et al.173 |
Parallelized library screening for XNA aptamers |
SARS-CoV-2 S1 protein (spike protein) |
K
d: sub-nanomolar affinity, 0.8 to 3.7 nM; enhanced specificity against off-target proteins (HSA and SA); excellent target discrimination |
Yoshikawa et al.174 |
Massively parallel screening platform for molecular switches |
Aptamers converted to molecular switches |
For ATP, Kd: ranged from 12–157 μM; for glucose, Kd is 1.9 mM for aptamer NNG, over 8000 aptamer switches exhibiting >2-fold fluorescence intensity change |
4.2.2 Non-SELEX methods in immobilized formats.
The most obvious and well adapted format for immobilized Non-SELEX is bead-based partitioning. For instance, a MB-based method rapidly selected β-casomorphin-7 aptamers, including one with a 29-nM Kd.172 Several other studies were instead based on competitive selection. For instance, the “competition-enhanced ligand selection” (ComELS) approach identified high-affinity DNA aptamers (Kd = 560 pM) for non-biological targets, demonstrating the potential for microfluidic integration to reduce selection cycles.167 Further analysis of these aptamers' secondary structures provided insight into how they would be bound to gold nanorods.168 Another competitive method, “systematic evolution of ligands by competitive selection (SELCOS),” rapidly enriched DNA aptamers specific to influenza virus subtypes, exhibiting Kd of 82 and 88 pM for H1N1 and H3N2, respectively; this approach could complement microfluidic-based sensor development.169 Similarly, SELCOS also identified a novel DNA aptamer that selectively inhibited CYP24A1, achieving sub-nM Kd values and showing potent anti-proliferative activity in cancer cells.171 These studies demonstrate the versatility and efficiency of non-SELEX methods across diverse targets and platforms (Table 5).
There are a few difficult-to-categorize but worthwhile methods worth mentioning. For instance, flow cell-based screening on a modified Illumina MiSeq platform allowed parallel analysis of base-modified DNA aptamers, achieving Kd values of 3.3 and 16.9 nM for VEGF and SL2B aptamers, respectively.170 A single-round screening method produced XNA aptamers targeting the SARS-CoV-2 S1 protein with affinities between 0.8 and 3.7 nM.173 These two cases used modified DNAs, which are difficult to incorporate into other SELEX methods. Additionally, a parallel platform for converting aptamers into molecular switches was associated with Kd values from 12 to 157 μM, offering the potential for biosensor development.174 These advances demonstrate the growing capabilities in aptamer screening and characterization for therapeutic and diagnostic applications (Table 5). Non-SELEX methods rely on the high efficiency of partitioning via hydrodynamic, magnetic, or electrokinetic forces and are particularly suitable for integration with microfluidics. Utilizing platforms with innovative materials or specialized microstructures can improve their performance. The miniaturization and automation of non-SELEX processes on a chip may enable high-throughput, rapid, and more resource-efficient aptamer discovery.
5. Versatility of aptamer-based detection strategies via microfluidics
5.1 Detection of a wide range of targets by aptamers
Aptamers offer advantages over traditional antibodies, including chemical stability and ease of 1) synthesis, 2) modification, and 3) tissue penetration.175,176 Aptamers have been successfully applied to detect ions, small molecules, proteins, viruses, and cells.177,178 This structural based versatility179–181 has led to the development of aptamer-based biosensors for pathogen detection,182 disease diagnosis,183,184 food safety,185 and environmental monitoring.186,187
5.2 Ions and chemical compounds
5.2.1 Ions.
Aptamer and microfluidics have been used for detection of various ions. For instance, an IMC combined with MB-tagged aptamer probes for performing multibranched hybridization chain reactions was developed for high-throughput, multiplex detection of kanamycin, 17β-estradiol, and lead ions in food samples.188 Another study developed an IMC that integrated solid-phase extraction with a graphene-oxide quantum dot array for automated, high-sensitivity detection of As3+, Cd2+, and Pb2+, achieving low detection limits (Kd = 5–41 nM) across a wide dynamic range within 45 min.189 Similarly, a microfluidic sensor featuring graphene-oxide and FAM/HEX-labeled DNA aptamers achieved highly sensitive detection of Hg2+ and Pb2+ in water, with LODs of 0.7 and 0.5 ppb, respectively.190 Another study utilized an electrochemical paper-based microfluidic chip for detecting ions, achieving LODs of 23 pM for Cd2+ and 46 pM for Pb2+, with a detection range of 0.1–1000 nM.191 In another work, the researchers employed a DNA aptamer-linked hydrogel integrated with a microfluidic heating system for repeatable detection of silver (Ag+) ions, offering a detection range from 10 μM to 10 mM, with repeatability across three cycles.192 Similarly, another group developed a dual-modality aptasensor for detection of mercury,193 and the microfluidic device demonstrated an LOD of 0.01 ppm (linear range = 0.01 to 1 ppm) for electrochemical detection, and a colorimetric limit of 0.5 ppm (0.5 to 20 ppm). Finally, a microfluidic aptasensor point-of-care (POC) device was developed for determining K+ concentration in chronic kidney disease patient blood (LOD = 0.01 mM).194 More detail information could be found in Table 6.
Table 6 List of research works using aptamers targeting ions to small molecules
Targets |
Microfluidic methodology |
Detection performance |
Ref. |
Mixed, small molecule, lead ion, kanamycin, 17β-estradiol |
Microfluidic chip (MC); magnetic bead (MB); multibranched hybridization chain reaction (mHCR) |
Detection limits: 1.76 × 10−4 nM (kanamycin), 1.18 × 10−4 nM (17β-estradiol), 1.29 × 10−4 nM, (Pb2+); linear range: 5.89 × 10−4−10 nM (kanamycin), 3.94 × 10−4−3.5 nM (17β-estradiol), 4.29 × 10−4−3.5 nM (lead ion); sensor reusable for 4000 samples; temperature and pH sensitive, reaction time for mHCR needed |
188
|
As3+, Cd2+, Pb2+ |
Solid-phase extraction (SPE); micropump, micromixer; graphene oxide quantum dot (GOQD) array chip; DNA aptamer |
Detection limits for As3+ (5.03 nM), Cd2+ (41.1 nM), Pb2+ (4.44 nM); linear range: As3+ (0.1 μM to 10 mM), Cd2+ (1 μM to 10 mM), Pb2+ (1 μM to 10 mM); recovery efficiency >80%; pH-dependent metal adsorption |
189
|
Hg(II)(Hg2+), Pb(II)(Pb2+) |
T-type micromixer with a tortuous channel; PDMS microfluidic device; graphene oxide (GO); DNA aptamer with FAM or HEX fluorescent dyes |
Hg(II): linear range: 10–250 nM or 2.0–50 ppb, LOD: 0.70 ppb; Pb(II): linear range: 10–100 nM or 2.1–20.7 ppb, LOD: 0.53 ppb |
190
|
Cd+2, Pb+2, |
Electrochemical paper-based microfluidic chip; Gold nanoparticles (AuNP); DNA aptamer paper-based microfluidic analytical devices (μPADs) |
LOD: 46.23 pmol L−1 for cadmium (Cd2+); LOD: 23.31 pmol L−1 for lead (Pb2+); detection linear range from 0.1 nmol L−1 to 1000 nmol L−1; detection in just 15 minutes; stay good at −20 °C for 5 days; chip recovery rate of 93.20% to 95.80%; not used in extreme pH |
191
|
Ag+ |
DNA aptamer-linked hydrogel; sensor: hydrogel, micro-heater, and micro-channel; aptamers cross-linked to acrylamide hydrogel |
Concentration range: 10−5–10 mM); Concentration dependent shrinking ratio (ε) of DNA aptamer-linked hydrogel; stable at temperatures (4–100 °C) |
192
|
Hg2+ |
Dual detection system-colorimetric detection: aptamer coated poly-styrene (PS) beads; AuNP; silver nanoparticles (AgNP); electrochemical detection; paper-based microfluidic component (μ-PAD); DNA aptamer |
Electrochemical detection: LOD of 0.01 ppm; dynamic range: 0.01–1 ppm (adj. R2 = 0.935); colorimetric system: PS-AuNPs-based system – qualitative detection (LOD 5 ppm), stable >7 days (97.59% signal retention); PS-AgNPs-based system – LOD 0.5 ppm, linear range 0.5–20 ppm (adj. R2 = 0.986), stable >30 days (94.95% signal retention) |
193
|
K+ |
Microfluidic aptasensor; PMMA/paper-microchip; integrated RGB analy-sis system; AuNPs; DNA aptamer; smartphone app |
Detection range of 0.05 to 9 mM (R2 = 0.994), (LOD) of 0.01 mM. 137 whole blood and 287 serum samples of chronic kidney disease (CKD) patients consistent (R2 = 0.968 and R2 = 0.980) with ion-selective electrodes (ISE) method |
194
|
Diacetyl |
Cell-free expression system; ODR-10 receptor (protein); anti-His6-tag aptamer; electrolyte-insulator–semiconductor (EIS) sensors; capacitance–voltage (C–V) and constant-capacitance (ConCap) |
LOD: 0.01 nM, dynamic range: 0.01–1 nM |
195
|
3,3′,4,4′-Tetrachlorobiphenyl (PCB77) |
Microfluidic electro-chemical aptamer sensing platform; exonuclease I (Exo I); DNA/AuNPs/HRP nanoprobes; Au/MoS2-CNTs-GO (Au/MCG) hybrids electrode |
Concentration range of 0.01–1000 ng L−1, LOD: 0.00885 ng L−1 |
196
|
3,3′,4,4′-Tetrachlorobiphenyl (PCB77) |
Electrochemical apta-sensor; Au/Nb2CTx/GO hybrids electrode; methylene blue/thiol-labeled cDNA (MB-COOH-cDNA-SH); ferrocenyl-modified aptamer (aptamer-fc) |
Detection range: 0.0001 to 1000 ng mL−1, LOD: 1.56 × 10−5 ng mL−1 |
197
|
Imidacloprid, carbendazim (pesticide) |
μ-PAD; PS-AuNP-aptamer-BSA; colorimetric detection; smartphone-integrated biosensor |
LOD: imidacloprid (3.12 ppm), LOD: carbendazim (1.56 ppm), detection range: 0.39–100 ppm |
198
|
Tobramycin |
PDMS micro-channels integrated with rGO/Au aptamer FET; 6-mercapto-1-hexanol (MCH)/1-pyrenebutanol (PBA) blocking enhanced sensing |
LOD: 0.3 nM (0.14 μg L−1), detection range: 10−8 M-10−4 M, detection within 5 s |
199
|
Ochratoxin A (OTA) |
Lab-on-Chip (LoC) integrated aptasensor; fluorescent molecule [Ru(phen)2(dppz)]2+, 2(hydroxylethyl methacrylate) (PHEMA) functionalized glass; aptamer |
LOD: 1.3 ng mL−1, detection range: 5–200 ng mL−1, small sample volume (∼10 μL), short time (5 min) |
200
|
Kanamycin |
Microfluidic chip-based ratiometric aptasensor with rolling circle amplification (RCA) |
LOD: 0.3 pg mL−1, detection range: 0.8 pg mL−1 to 10 ng mL−1, small sample volume (150 pL) |
201
|
Ochratoxin A (OTA) |
Graphene field-effect transistor (GFET) |
LOD: 4 pg mL−1, detection range: 10 pg mL−1–4 ng mL−1 |
202
|
Okadaic acid |
Phosphorene-gold nanocomposite-based aptasensor; screen-printed carbon electrodes (SPCE); black phosphorous (BP); BP-Au nanocom-posite (BP-Au NC) |
LOD: 8 pM, linear range of detection: 10–250 nM (R2 = 0.9887) |
203
|
Kanamycin, aflatoxin M1 (AFM1), 17β-estradiol (E2) |
Magnetic tripartite DNA aptasensor with microfluidic chip; RCA; phi29 polymerase |
LOD: 0.32 pg mL−1 (KANA), 0.95 pg mL−1 (AFM1), 6.8 pg mL−1 (E2); detection range: 0.001–100 ng mL−1 (KANA), 3–50 pg mL−1 (AFM1), 0.02–2 ng mL−1 (E2) |
204
|
Aflatoxin M1 |
Microfluidic paper-based analytical device (μPAD); AuNP; 21-mer aptamer |
LOD: 3 pM in buffer, 10 nM in milk; detection range: 1 μM–1 pM |
205
|
Enrofloxacin (ENR) |
Lateral flow strip with smartphone readout; lateral flow-based biosensor (LFB); end-regulated AuNP clustering (TAuC) |
LOD: 0.1 μg L−1, detection range: 0.1–104 μg L−1 |
206
|
Ochratoxin A (OTA) |
Liquid crystal-based aptasensor |
LOD: 0.63 aM, ultra-low detection in food and human serum samples, range: 0.001–400 fM |
207
|
Aflatoxin-M1 |
Microfluidic electro-chemical biosensor; graphene quantum dot-gold nanoparticle, GQDA |
LOD: 0.397 nM, detection range: 100 pM–2 nM |
208
|
Δ9-Tetrahydrocannabinol (THC) |
Electrochemical aptasensor on a microfluidic platform |
LOD: 1 nM in PBS, 10 nM in 10% diluted saliva, 5 nM in size excluded filtration pretreated saliva, rapid detection in 1 min |
209
|
Enrofloxacin (ENR) |
Localized Surface Plasmon Resonance (LSPR) sensing chip with microfluidic device; AuNP |
LOD: 0.001 ng mL−1; detection range: 0.01–100 ng mL−1; recovery: 91.94–108.38% in real water samples |
210
|
Ochratoxin A (OTA) |
Thermal-regulated sensor integrated with covalent organic framework (COF); COF-Au-MB-Apt signal probe |
LOD: 0.12 pg mL−1, detection range: 0.2 pg mL−1 to 0.6 μg mL−1 |
211
|
5.2.2 Chemical compounds.
Chemical compounds can also be detected using aptamer-based microfluidic sensors. For instance, a study has demonstrated the use of olfactory receptors immobilized by anti-His6-tag aptamers to detect diacetyl with high specificity and a 0.01 nM detection limit.195 Microfluidic electrochemical aptasensors have also been developed for detecting polychlorinated biphenyls like 3,3′,4,4′-tetrachlorobiphenyl (PCB77) using exonuclease I and DNA/AuNPs/HRP nanoprobes (LOD = 9 pg L−1).196 Another microfluidic ratiometric electrochemical aptasensor was developed for detecting PCB77 with a broad detection range (0.1 pg mL−1–1000 ng mL−1) and an exceptionally low LOD of 0.16 fg mL−1.197 Moreover, smartphone-integrated aptasensors employing gold-coated polystyrene microparticles have demonstrated rapid, label-free, and selective detection of pesticides like imidacloprid and carbendazim, achieving LOD as low as 3 and 1.5 ppm, respectively, within a 0.39 to 100 ppm range198 (Table 6).
5.3 Small molecules and biomolecules
5.3.1 Antibiotics.
Various studies have demonstrated the versatility of microfluidic-based aptasensor technologies for detecting a wide range of antibiotics and contaminants. For instance, Chen et al.199 utilized a graphene-based field-effect transistor sensor to achieve ultra-sensitive detection of tobramycin, with an LOD of 0.3 nM and a detection range of 10−8 to 10−4 M. Another group developed a IMC-based ratiometric aptasensor using rolling circle amplification for kanamycin detection, reaching an LOD of 0.3 pg mL−1 (range = 0.8 pg mL−1 to 10 ng mL−1).201 The same team also introduced a simultaneous detection method for kanamycin, aflatoxin M1, and 17β-estradiol using a magnetic tripartite DNA aptasensor, with LODs of 0.3, 1, and 7 pg mL−1, respectively.204 Alternatively, another group demonstrated a localized SPR sensing chip for detecting enrofloxacin at an LOD of 0.001 ng mL−1 and a range of 0.01–100 ng mL−1.210 For the same target, Tian et al. presented a lateral flow aptasensor using gold nanoparticles (AuNPs) for enrofloxacin detection, with mobile phone-based readout for on-site analysis.206 The sensor showed an LOD of 0.1 μg L−1 and a detection range of 0.1–104 μg L−1. These studies highlight advancements in microfluidic aptasensors and their high sensitivity across broad detection ranges and differing applications (Table 6).
5.3.2 Toxins.
Several groups have developed microfluidic technologies for detecting toxins, including ochratoxin A (OTA) and aflatoxin M1. For instance, Costantini et al.200 used a lab-on-chip system with an aptasensor to achieve an OTA detection range of 5–200 ng mL−1 and an LOD of 1.3 ng mL−1 Similarly, Nekrasov et al.202 developed a graphene field-effect transistor for on-chip OTA detection, reaching an LOD of 4 pg mL−1 at a range of 10 pg mL−1 to 4 ng mL−1. Khoshbin et al.207 also developed a liquid crystal-based aptasensor using a π-shaped DNA structure for ultra-low detection of OTA. The sensor achieved a detection limit of 0.63 aM, with high selectivity testing in grape juice, coffee, and human serum. Moreover, another group designed a ratiometric thermal-regulated sensor with a COF-Au-MB-Apt signal probe for detecting OTA as well.211 The sensor achieved an LOD of 0.12 pg mL−1 and a detection range of 0.2 pg mL−1 to 0.6 μg mL−1, with 98.5% regeneration efficiency over seven cycles. For aflatoxin M1, Kasoju et al.205 micro-fabricated a microfluidic paper-based device that detected levels as low as 3 pM in buffer and 10 nM in milk, while Ramalingam et al.208 used a biochip with graphene quantum dot-gold nanoparticles to achieve an LOD of 0.4 nM. The same group also developed an electrochemical microfluidic biochip for okadaic acid (OA) detection using a polydimethylsiloxane (PDMS) platform and aptamer-modified screen-printed carbon electrodes.203 In real-world shellfish samples, the sensor showed excellent selectivity and performance with 8 pM LOD and a linear detection range of 10–250 nM. Moreover, another group utilized an electrochemical aptasensor with a 3D-printed microfluidic cartridge detects Δ9-tetrahydrocannabinol (Δ9-THC) in saliva.209 This sensor had high specificity with a LOD of 1 nM in PBS and 5 nM in saliva, making it a promising point-of-care cannabis detection tool. These works have demonstrated great potential for aptamers on microfluidics for toxin detection (Table 6).
5.3.3 Large biomolecules.
Aptamers have been used for detection of large molecules on microfluidic systems. For instance, Ma et al.212 developed a microfluidic electrochemical biosensor for endotoxin detection, achieving an LOD of 0.5 ng mL−1 at a dynamic range of 0.5 to 200 ng mL−1. Similarly, Nascetti et al.213 developed an embedded sensor chip featuring chemiluminescence-based adenosine triphosphate (ATP) detection, with a detection limit of 60 nM and a detection range from 0.3 nM to 20 μM; Niu et al.214 employed continuous injection-electrostacking within an IMC for lipopolysaccharide detection (LOD = 7.9 fM in water). Moreover, Nandimandalam et al.215 used a lab-on-chip platform integrating split aptamers immobilized on polymer brushes for ATP detection with an LOD of 0.89 μM, and Weng et al.216 presented a portable 3D microfluidic origami biosensor for cortisol detection, achieving an LOD of 6.8 ng mL−1 at a detection range of 10 to 1000 ng mL−1. These studies have paved a way for developing compact devices for detecting various molecules. More detail information could be found in Table 7.
Table 7 List of research works using aptamers targeting biomolecules to proteins
Targets |
Microfluidic methodology |
Detection performance |
Ref. |
Endotoxin |
Microfluidic electro-chemical biosensor; electrochemical impe-dance spectroscopy (EIS); aptamer/AuNP/SPCE |
LOD: 0.5 ng mL−1, detection range: 0.5 to 200 ng mL−1 |
212
|
Adenosine triphosphate (ATP) |
PLEIADES chip with chemiluminescence detection; combine of an autonomous capillary force-driven microfluidic network; an array of thin-film hydrogenated amorphous silicon photosensors, and chemiluminescence bioassays |
With immunoassay for ATP: LOD: 60 nM, detection range: 0.3 nM to 20 μM; demonstrated feasibility of combining aptamer nanoswitch |
213
|
Lipopolysaccharide (LPS) |
Continuous injection-electrostacking (CI-ES) biochip |
LOD: 7.9 fM (water), 8.3 fM (serum), detection range: 50 fM–106 fM in water, 50–104 fM in serum |
214
|
ATP |
Lab-on-chip with PHEMA-aptamer fragments; amorphous silicon (a-Si:H) photosensor; polyhydroxyethyl-methacrylate (PHEMA); [Ru(phen)2(dppz)]2+ light switch |
LOD: 0.89–0.98 μM, detection range: 0.1–1000 μM |
215
|
Cortisol |
3D microfluidic origami biosensor; molybdenum disulfide (MoS2) nanosheet-mediated fluorescence resonance energy transfer (FRET); fluorescently labeled aptamers |
LOD: 6.76 ng mL−1, detection range: 10–1000 ng mL−1 |
216
|
Thrombin, immunoglobulin E (IgE) |
Acoustofluidic separation using traveling surface acoustic waves (TSAW) |
Separation efficiency: 99.04% for thrombin, 98.85% for IgE; recovery rate: 97.82% for thrombin, 96.31% for IgE |
217
|
C-reactive protein, thrombin |
Microring resonator (MRR)-based biosensor with aptamer-functionalization |
Best condition for MRR surface immobilization of aptamer: argon plasma, 1% v/v mercaptosilane, concentration and time optimized 1 μM and 3 h |
218
|
His-tagged proteins (D2 protein in arabinanase family) |
3D-printed polyacrylate microfluidic device integrated with porous silicon (PSi) aptasensors |
Detection range: 0.25–18 μM, LOD: 0.04 μM, LOQ: 0.16 μM, RSD 12–22% |
219
|
Leptin |
PCR amplification of short DNA aptamers in a lab-on-chip implementation |
LOD: 100 pg mL−1 for leptin, significant reduction in assay time to less than 2 hours |
220
|
Alpha-defensin human neutrophil peptide 1 (HNP1) |
Integrated microfluidic system for aptamer selection |
Aptamer selected: Kd 19 nM, LOD: 0.1 mg L−1, dynamic range: 0.1–100 mg L−1 |
41
|
Human neutrophil peptide 1 (HNP1) |
Paper-based aptamer-sandwich assay |
Detection limit (LOD): 0.5 mg L−1; dynamic range: 0.5–100 mg L−1 |
221
|
Alpha-defensin human neutrophil peptide 1–3 (HNP1–3) |
Integrated microfluidic platform for diagnostic assays |
LOD: 0.3 mg L−1, dynamic range: 0.32–100 mg L−1, sample volume 50 μL, assay time <3 hours |
43
|
SARS-CoV-2 nucleocapsid protein |
Aptamer/antibody sandwich method on a microfluidic chip |
LOD: 33.28 pg mL−1, 100 pg mL−1 to 100 ng mL−1 |
222
|
Peanut allergen Ara h1 |
Microfluidic origami electrochemical nano-aptasensor, black phosphorus nanosheets, BPNSs |
LOD: 21.6 ng mL−1; linear range: 50–1000 ng mL−1, detection time 20 minutes, sensitivity: 0.05 μA·ng mL−1; recovery rate: 98.3–107.9% in spiked cookie dough samples; relative standard deviation (RSD) < 5% |
223
|
Thrombin biomarker |
Integrated microfluidic platform with graphene FET biosensor |
LOD: 2.6 pM for thrombin; dissociation constant Kd = 375.8 ± 165.6 pM, selectivity tested against lysozyme |
224
|
Thrombin, C-reactive protein (CRP) |
Acoustofluidic separation using aptamer-functionalized micro-particles, surface acoustic waves (SAW); interdigital transducer (IDT) |
Separation efficiency >95%, flow rate 1.6 ml h−1, maximum flow velocity: 37.04 mm s−1; LOD not explicitly stated |
225
|
NT-proBNP, fibrinogen, cTnI, CRP |
Self-driven, microfluidic integrated-circuit (IC)-based biosensing chip with aptamer-coated interdigitated electrodes (IDE) |
LOD: NT-proBNP: 1.53 pg mL−1, fibrinogen: 59.4 mg dL−1, cTnI: 0.54 pg mL−1, CRP: 0.39 mg L−1; dynamic ranges: NT-proBNP (0.1–10 000 pg mL−1), fibrinogen (50–1000 mg dL−1), cTnI (0.1–10 000 pg mL−1), and CRP (0.5–9 mg L−1), total 15 min detection time |
226
|
α-Synuclein (αSyn) in saliva, Parkinson's disease (PD) patient |
Organic electrolyte-gated field-effect transistor (OEGFET) with soft microfluidics |
LOD: 10 fg L−1; Kd: 10 ng L−1 (6.6 pM), linear range: 100 fg to 10 μg L−1, recovery rate: 88.6% to 104%; repeatability over a month |
227
|
Odontogenic ameloblast-associated protein (ODAM) |
IoT-based microfluidic point-of-care (POC) biosensing chip with aptamer-based sandwich-type binding |
LOD: 0.011 nM (∼0.36 ng mL−1); detection time: 30 min; specificity: 100% for ODAM detection; linear range: 0.15–6.25 nM |
228
|
Plasmodium falciparum LDH (PfLDH) |
Microfluidic paper analytical device (μPAD) with aptamer-based detection |
LOD: 17 nM, Kd: 24 ± 11 nM in buffer, specific recognition of 133 nM rPfLDH in serum and blood samples |
229
|
Peanut allergen Ara h1 |
Paper-based microfluidic device with BP-Au nanocomposites for signal amplification; μ-PAD |
LOD: 11.8 ng mL−1; linear range: 25–800 ng mL−1; analysis time: 20 min; recovery rate: 93.50%–101.86% with RSDs of 0.36%–2.97% (n = 3) |
230
|
Tumor necrosis factor alpha (TNFα) |
Lab-on-chip multigate organic transistor (EGOFET); peptide affimer |
LOD: 3 pM; dynamic range: 0.1–1000 pM |
183
|
Gliadin |
Electrochemical microfluidic biochip with MoS2/graphene/Au nanocomposite |
LOD: 7 pM, linear detection range: 4–250 nM (R2 = 0.982), total assay time: 20 min |
231
|
Thrombin |
Integrated microfluidic pneumatic circuit (iPC) for point-of-care diagnostics |
LOD: 133.37 pM, detection range: up to 600 pM |
115
|
Carcinoembryonic antigen (CEA) |
Cloth-based closed bipolar solid-state electrochemiluminescence (CBP-SS-ECL) aptasensor |
LOD: 1.6 pg mL−1; linear range: 5–100 000 pg mL−1 |
232
|
Cardiac troponin T (cTnT) |
Smartphone-based paper microfluidic aptasensor with colorimetric detection; μ-PAD |
LOD: 0.39 ng mL−1; linear ranges: 0.01–0.8 μg mL−1 and 6.25–50 μg mL−1, platform stable >23 days |
233
|
Prostate-specific antigen (PSA) |
Microfluidic chip integrated with dielectrophoretic (DEP) separation and differential pulse voltammetry (DPV) detection |
LOD: 0.25 pg mL−1; linear range: 1 pg mL−1–10 ng mL−1, good stability to 15 days at 4 °C |
184
|
His-tagged recombinant proteins, his-IgE |
Microfluidic dual-aptamer sandwich assay |
LOD: 7.1 nM; detection time: 10 min in culture media; dual-aptamer approach enhanced sensitivity and reusability |
88
|
SARS-CoV-2 S1 protein |
Automatic integrated microfluidic system for aptamer screening |
LOD: 48 copies per mL (Wuhan strain); LOD: 195 copies per mL (omicron strain); affinity (Kd): 63 nM |
42
|
Thrombin |
Microfluidic paper-based photoelectrochemical sensing platform, μ-PEC; electron-transfer tunneling distance regulation (ETTDR); nicking enzyme signaling amplification (NESA) |
LOD: 6.7 fM, detection range: 0.02 pM to 100 pM |
234
|
Biomarker and drug uptake in single cells, protein tyrosine kinase 7 (PTK7) protein on cancer cells |
Microfluidic flow cytometry with mass spectrometry (μCytoMS), 6-FAM-Sgc8 aptamer functionalized AuNP |
K
d: 0.23 nM for PTK7 detection; throughput: 500 cells per min; single-cell drug uptake analysis using oxaliplatin (OXA) |
235
|
5.4 Proteins
5.4.1 Thrombin.
Proteins are the most-targeted molecules for detection via microfluidic platforms, and thrombin has been particularly well studied. For instance, aptamer-functionalized microparticles were used to separate thrombin, IgE, and mCardinal2 simultaneously using acoustofluidics for clinical diagnostic applications.217 In another work, a microfluidic-integrated graphene field-effect transistor biosensor was developed to selectively and sensitively detect thrombin biomarkers at an LOD of 2.6 pM for POC diagnostics.224 An integrated pneumatic microfluidic circuit with autonomous pumps was designed to separate plasma for rapid POC molecular diagnostics.115 Additionally, Xue et al.234 developed a microfluidic, paper-based photoelectrochemical sensing platform for thrombin detection using electron-transfer tunneling distance regulation and aptamer target-triggering nicking enzyme signaling amplification; this device demonstrated high sensitivity and clinical diagnostic potential (Table 7).
5.4.2 Allergens.
All kinds of allergens have been demonstrated using aptamers on microfluidics. For instance, food safety requires detection of the peanut allergen Ara h1, a protein that causes mild skin irritation to life-threatening anaphylaxis in a large percentage of peanut-induced allergic reactions. A microfluidic origami electrochemical nano-aptasensor with black phosphorus nanosheets could detect Ara h1 across a linear range of 50–1000 ng mL−1 and an LOD of 22 ng mL−1 for low-cost, rapid food safety-testing.223 Another group further developed a paper-based microfluidic device with black phosphorus-gold nanocomposites that could detect Ara h1 across a linear range of 25–800 ng mL−1 and an LOD of 12 ng mL−1 in food products230 (Table 7).
5.4.3 Proteins as infectious disease biomarkers.
Proteins could serve as valuable biomarkers for a wide range of acute and chronic diseases. For instance, one IMC employed an aptamer/antibody sandwich method to detect the COVID-19 nucleocapsid protein at a detection limit of 33 pg mL−1 (300-fold more sensitive than enzyme-linked immunosorbent assay (ELISA)), making it ideal for early diagnosis.222 Another group developed an IMS for screening SARS-CoV-2 S1 aptamers, achieving an LOD of 48 copies mL−1 for the Wuhan strain and 195 copies/mL for the Omicron strain, thus enabling efficient and accurate diagnosis42 (Table 7).
Periprosthetic joint infections (PJIs) are serious complications that can result from joint replacement surgeries and cause severe joint pain, implant failure, and the need for additional surgeries. Gondotra et al.41 developed an IMS for selecting aptamers against alpha-defensin human neutrophil peptide 1 (HNP1), which is a biomarker for PJIs in synovial fluid with a Kd of 19 nM using an ELISA-like assay. A paper-based aptamer-sandwich assay integrated into an IMS to detect HNP1 was further characterized by a detection limit of 0.5 mg L−1 across a dynamic range of 0–100 mg L−1, making it a rapid, cost-effective POC diagnostic tool.221 Finally, another work introduced an IMS for selecting high-affinity aptamers targeting HNP1–3 with a detection limit of 0.3 mg L−1 and efficient SELEX screening with minimal human intervention, thereby improving PJI diagnostics.43 These works have demonstrated that aptamers could be used to capture protein biomarkers targeting infectious diseases (Table 7).
5.4.4 Proteins as cardiovascular disease biomarkers.
Cardiovascular disease (CVD), the leading cause of death globally, can lead to heart attacks, strokes, and heart failure. Aptamers have been used for CVD diagnosis. For instance, Li et al.226 developed a self-driven microfluidic, integrated-circuit biosensing microfluidic chip that detected four cardiovascular disease biomarkers, including N-terminal pro-B-type natriuretic peptide (NT-proBNP), fibrinogen, cardiac troponin I (cTnI), and C-reactive protein (CRP; an inflammation biomarker) - with low detection limits and aptamer-coated interdigitated electrodes for rapid, POC diagnostics. Another group utilized a colorimetric approach and gold-decorated microparticles to detect cTnT at a detection limit of 0.39 ng mL−1 with a smartphone-based, paper microfluidic aptasensor for sensitive and portable acute myocardial infarction diagnosis.233 CRP has been targeted in multiple studies using microfluidic aptasensors.218,225,226,233,236 For instance, one article reported a micro-ring resonator-based biosensor that used optimized surface functionalization and specific aptamers to improve thrombin and CRP detection sensitivity.218 Another acoustofluidic device used aptamer-functionalized microparticles to separate thrombin and CRP from platelets in plasma at 1.6 mL h−1 flow rate, >95% efficiency, demonstrating its potential for multiplexed liquid biopsy applications in CVD detection225 (Table 7).
5.4.5 Protein as biomarkers for cancer diagnosis.
Several groups have explored the use of aptamers and microfluidic technologies for cancer biomarker detection, which is essential for early diagnosis, treatment monitoring, and patient outcomes. For instance, an electrolyte-gated organic field-effect transistor with microfluidic channels could detect tumor necrosis factor α (TNFα) at a 3 pM detection limit, improving POC diagnostics.183 Similarly, Su et al.232 developed a cloth-based, bipolar, solid-state, electrochemiluminescence aptasensor for carcinoembryonic antigen detection; with an LOD of 1.6 pg mL−1, this durable, cost-effective device is also suitable for POC applications. Additionally, an IMC enabling dielectrophoretic separation and differential pulse voltammetry rapidly detected prostate-specific antigen (PSA) in blood at 0.25 pg mL−1.184 Another group235 devised an IMS combining flow cytometry and mass spectrometry for high-throughput biomarker and drug uptake profiling in single cells, enabling personalized cancer treatment and therapeutic monitoring. It is a growing trend to use aptamers on microfluidics for cancer biomarker detection (Table 7).
5.4.6 Other proteins.
Microfluidic platforms have demonstrated versatility in detecting diverse protein targets. For instance, a 3D-printed, polyacrylate-based system with optical, nano-structured, porous silicon enhanced biosensing of arabinanase D2, reducing sample volumes and improving sensitivity (0.25 to 18 μM) over other PDMS-based methods219 and could be used in medical diagnostics and environmental monitoring.219 Alternatively, a lab-on-chip platform used PCR-amplified DNA aptamers to quantify leptin more rapidly (∼2 h) and sensitively (LOD = 100 pg mL−1) than ELISA.220 Moreover, an internet of things (IoT)-based aptasensor biochip was developed to detect the periodontal disease biomarker odontogenic ameloblast-associated protein (ODAM).228 Alternatively, a sandwich-type fluorescence aptasensor using an aptamer pair (MB-OD64 & FAM-OD35) selectively bound target ODAM within 30 min at an LOD of 0.01 nM (Table 7).
Another study introduced an organic electrolyte-gated field-effect transistor (FET) with microfluidic channels for non-invasive α-synuclein detection in saliva.227 The detection limit was 10 fg L−1, demonstrating potential for early Parkinson's disease diagnosis. Ogunmolasuyi et al.229 developed a microfluidic paper analytical device to rapidly detect Plasmodium falciparum lactate dehydrogenase, offering low-cost malaria diagnosis.229 Another microfluidic electrochemical aptasensor was integrated into a polydimethylsiloxane (PDMS)-based flexible microfluidic device with a MoS2/graphene/gold nanocomposite-modified electrode for detection of gliadin at an LOD of 7 pM within 20 min at a detection range of 4–250 nM.231 These specifications make it suitable for POC food safety assessments, notably in identifying gluten-free foods. Moreover, a microfluidic, dual-aptamer sandwich assay for rapid detection of His-tagged recombinant proteins88 screened aptamers for His-tag and IgE via microfluidic SELEX and was associated with an LOD of 7.1 nM within 10 min.88 The aptamer surfaces were reusable for up to 20 cycles, maintaining consistent performance; this could lead to reduced costs (Table 7).
5.5 Virus and exosomes/extracellular vesicle (EV) targets
5.5.1 Viruses.
Several studies have advanced viral detection using microfluidic and biosensing technologies via aptamers. For instance, a study237 developed a microfluidic chip for selective isolation of active SARS-CoV-2 virus particles, using surface-bound aptamers targeting the spike protein's receptor-binding domain. The chip design included 1.5 million diamond-shaped micropillars, optimizing surface area for efficient virus capture. The system achieved 94% recovery of virus particles, with 89% release efficiency using blue light. In clinical trials, the device demonstrated a 95% sensitivity and relatively high specificity for detecting active SARS-CoV-2, distinguishing patients with active disease from those with non-active viral fragments. Another strategy used a microfluidic enrichment system based on stoichiometric balanced DNA computation, utilizing dual-domain aptamers targeting different spike protein regions and cholesterol-DNA for viral envelope interaction.238 This herringbone microfluidic chip enhanced viral particle capture by inducing chaotic flow and thus improving interactions and demonstrated an LOD of 37 active virions μL−1 and a 90% release efficiency of intact SARS-CoV-2 particles via DNase I treatment, enabling high-throughput viral culture and downstream analysis. Moreover, a microfluidic device with aptamer-functionalized gold nanoparticles detected Zika and Chikungunya viral envelope proteins at 1 pM in PBS and 100 pM in calf blood.239 Similarly, an on-chip integrated graphene aptasensor detected SARS-CoV-2 particles and neutralizing antibodies at 103 particles per mL and 160 aM, respectively, for POC diagnostics.240 These studies demonstrate the effectiveness of microfluidic biosensing technologies employing aptamers for high-specificity/high-sensitivity viral detection (Table 8).
Table 8 List of research works using aptamers virus, exosome/EVs, and bacteria
Targets |
Microfluidic methodology |
Detection performance |
Ref. |
Active SARS-CoV-2 virus particles |
Microfluidic chip with surface-bound aptamers and blue-light release |
Recovery: 94%; release efficiency: 89%; sensitivity: 95% (for 19 out of 20 COVID-19 positive patients) |
237
|
Intact SARS-CoV-2 particles |
Microfluidic enrichment with stoichiometric balanced DNA computation, AND logic gate, cholesterol-DNA, dual spike protein aptamer |
LOD: 37 virions per μL, viral release efficiency: 90%; sensitivity: 100% for 14 COVID-19 patients, specificity: 100% |
238
|
Zika and Chikungunya virus proteins |
Polydimethylsiloxane-based microfluidic device with gold nanoparticle (AuNP) aptamers |
LOD: 1 pM (50 pg mL−1) (Zika); sensitivity: 100 pM in calf blood; 10 pM in dilute (10%) mechanically defibrinated calf blood |
239
|
SARS-CoV-2 particles, antibodies |
On-chip integrated graphene field-effect transistor (GFET) aptasensor,aptamer-functionalized GFET (aptasensor, Apt-GFET) |
LOD: 103 particles per mL; 160 aM for neutralizing antibodies |
240
|
Exosomes from human serum and cell culture |
Dean-flow-coupled elasto-inertial focusing (DEIC), spiral microchannel with dimensional confined concave structures |
Recovery rate: 70.6%; protein removal rate: 91.4%; single-vesicle-level biomarker profiling using aptamers for EpCAM and PD-L1 |
241
|
Non-small cell lung cancer (NSCLC)-related exosomes |
Ship-shaped microfluidic device with PDMS microcolumn array, CD63 protein aptamer, catalytic hairpin assembly (CHA) |
LOD: 83 exosomes per μL; linearity range: 104–109 exosomes per mL; multiplex detection of EpCAM, EGFR, miRNA-21, and miRNA-200, distinguishing cancer cell subtypes and lung cancer patients |
242
|
Colorectal cancer exosomes |
Aptamer-magnetic biosensor with transverse magnetic field, rotating magnet assembly system (rMAS) |
LOD: 1457 particles per mL; linear range: 1.75 × 103 to 8.75 × 107 particles per mL; specificity tested with closely related proteins; aptamer-magnetic bead method for isolation and pre-concentration |
243
|
Exosomal miRNA-210 (cancer biomarker) |
Microfluidic aptamer-conjugated magnetic nanobeads for separation |
LOD: 5 pM (miRNA-210); linear range: 0.05–100 nM; fluorescence-based detection with carbon nanomaterial-coated magnetic beads |
244
|
Exosomal membrane proteins (PD-L1, EpCAM, CA125) |
Centrifugal microfluidic disc and aptamer-based fluorescence detection, automated centrifugal microfluidic disc system (Exo-CMDS), aptamer fluorescence system (Exo-AFS) |
LOD: 1.58 × 105 particles per mL for PD-L1, exosome yield: 5.1 × 109 particles per mL in 8 minutes; diagnostic accuracy: 91% for lung cancer |
245
|
Cancer-associated exosomes |
Drop-shaped micropillar microfluidic chip, cationic perylene tetracarboxylic acid diimide derivative (PTCDI), aptamers targeting CD63, PD-L1, CA125, nucleolin, EpCAM, PTK-7, PSMA |
LOD: 8.69 × 103 particles per mL, detection range: 8.74 × 103 to 1.37 × 108 particles per mL |
246
|
Exosomes carrying CD63 and PTK7 |
Aptamer-based microfluidics system with surface-modified channels |
Capture efficiency: 107–108 particles per mL in 20 min, specific isolation of exosomes based on CD63 and PTK7 aptamers |
247
|
Extracellular vesicles (EVs) |
Integrated ExoID-Chip with photonic crystal nanostructure, aptamer targeting CD63 |
LOD: 8.9 × 103 EVs mL−1; sample volume: 20 μL; successfully distinguishes breast cancer patients from healthy controls |
248
|
PD-L1 EV subpopulations (tumor and non-tumor origin) |
DNA computation mediated microfluidic tandem separation |
LOD: 4.79 pg mL−1 for PD-L1; selective isolation of tumor and non-tumor PD-L1 EVs |
249
|
Escherichia coli (E. coli) |
Non-faradaic impedance biosensor, IDE arrays, OMP Ag1 aptamer targeting E. coli |
LOD: 9 CFU mL−1; sensitivity: 1.8 ohm CFU−1; analytical range: 25–1000 CFU mL−1 |
250
|
E. coli O157:H7 |
Dendrimer-aptamer modified PDMS microfluidic channels |
LOD: 102 CFU mL−1 in G7 channels, comparison between G7 and G4 dendrimers showed higher detection efficiency for G7-modified surfaces |
182
|
Streptococcus pneumoniae
|
Aptamer decorated PDA@magnetic silica microparticles, SELEX |
Capture efficiency: 87% in PBS, 66% in blood, aptamer affinity: Kd = 72.8 nM |
251
|
Campylobacter jejuni, Aliarcobacter butzleri |
Fusarinine C-magnetic nanoparticles and aptamer-red/green carbon dots |
LOD: 1 CFU mL−1 for each bacterium, detection range up to 1 × 107 CFU mL−1; RGB color detection enabled detection with LOD of 5 CFU mL−1 in 65 min |
252
|
Salmonella typhimurium
|
Colorimetric biosensor, smartphone APP, thiolated microspheres |
LOD: 6.0 × 101 CFU mL−1, detection time: 45 minutes; recovery in salad samples: 91.68–113.76% |
253
|
Bacillus subtilis in soil |
Toggle-cell SELEX, portable aptamer-based detection technology with magnetic beads |
K
d: 23.74 ± 1.19 nM; Bmax: 3.24 ± 0.16 nM; detection in soils with inoculated B. subtilis: 3.67 × 106 to 3.37 × 107 cells per mL |
254
|
Salmonella typhimurium
|
Digital PCR Chip with aptamer-coated magnetic beads |
Detection as low as 90 CFU per reaction, with a capture efficiency of 94.5%. The process takes about 2 hours |
255
|
Helicobacter pylori
|
Superparamagnetic nanoparticles with fluorescence-based detection |
Detection range of 10–107 CFU mL−1 with an LOD of 1 CFU mL−1, Total detection time: 65 minutes |
256
|
Acinetobacter baumannii
|
Nitrocellulose membrane-based microfluidic system with electromagnetic micro-devices |
Detection limit as low as 450 CFU per reaction within 40 minutes |
257
|
Vibrio parahaemolyticus
|
Capillary microfluidic chip with magnetic beads manipulation |
Complete detection in 40 minutes with high selectivity using capillary force |
258
|
Bacillus cereus
|
Portable dual-aptamer microfluidic chip biosensor, rolling circle amplification (RCA) method |
On bench assay: LOD: 4.85 CFU mL−1, detection range: 1.15 × 101 to 1.15 × 106 CFU mL−1, portable microfluidic chip: LOD: 9.27 CFU mL−1, detection range: 1.59 × 102–1.59 × 107 CFU mL−1 |
259
|
E. coli O157:H7 |
Tetrahedral DNA nano-structure (TDN)-aptasensing microcavity, nano-bio aptasensing interface, integrated microfluidic chip, framework nucleic acids (FNAs) |
LOD: 10 CFU mL−1, enrichment efficiency: Enhanced with TDN-engineered microchannel |
260
|
Pseudomonas aeruginosa
|
PDMS-paper hybrid microfluidic chip |
LOD: 10 CFU mL−1, detection time: 15–20 minutes, linear detection range: 10 CFU mL−1 to 1000 CFU mL−1 |
261
|
5.5.2 Exosomes and EVs.
Exosomes are EVs measuring 30–150 nm that play crucial roles in intercellular communication and disease pathogenesis.262,263 Their presence in bodily fluids and potential as biomarkers make them attractive for clinical applications,264 and microfluidic technologies can significantly enhance the isolation and detection of exosomes and EVs via aptamers. For example, Bai et al.241 employed Dean-flow coupled elasto-inertial microfluidics to separate exosomes from proteins in biological fluids, utilizing spiral microchannels and viscoelastic lift forces to enhance separation by using aptamers. The system achieved 71% recovery of exosomes with 91% removal of protein contaminants, enabling single-vesicle profiling for downstream analyses. Another report by Chen et al.242 introduced a microfluidic device with a micro-column array for isolating exosomes and simultaneously detecting surface proteins and miRNAs using a catalytic hairpin assembly signal amplification strategy composed by aptamers. It achieved an LOD of 83 exosomes per μL, allowing for the distinction of different cancer subtypes, as well as cancer patients from healthy individuals (Table 8).
Moreover, Chinnappan et al.243 utilized an aptamer-magnetic microfluidic platform for isolating and detecting colorectal cancer exosomes, with anti-CD63 aptamers conjugated to magnetic nanobeads used for recognition. This platform achieved a detection limit of 1457 particles per mL and high specificity against closely related proteins, showing potential for rapid exosome isolation and cancer biomarker detection in clinical applications.243 Another study demonstrated a microfluidic device using aptamer for the isolation and detection of exosomal Mir-210 using carbon nanomaterial-coated MBs244 with a fluorescence-based switching assay. The system was characterized by a detection limit of 5 pM, showing no cross-reactivity with other miRNAs; it therefore could serve as a minimally invasive method for cancer biomarker detection (Table 8).
Furthermore, Zhao et al.245 reported the development of an automated centrifugal microfluidic disc system for exosome isolation, paired with an aptamer fluorescence system for detecting exosomal surface proteins. The system isolated 5 × 109 particles per mL in just 8 min and achieved an LOD of 1.6 × 105 exosomes mL, offering 91% diagnostic accuracy for lung cancer. Alternatively, Zheng et al.246 demonstrated a microfluidic chip with alternating drop-shaped micropillars for efficient exosome capture using Tim4-modified MBs. The system using aptamer as signal probe achieved a detection limit of 8.7×103 particles/mL, enabling detailed profiling of exosomal surface proteins for distinguishing liver cancer patients from healthy individuals. Moreover, Zhou et al.247 presented an aptamer-functionalized microfluidic system for isolating exosomes based on CD63 and PTK7 markers, achieving a capture efficiency of 107–108 particles/mL in just 20 min; these specifications make it suitable for cancer diagnostics (Table 8).
Besides, Dong et al.248 developed the ExoID-Chip, which integrated nanofiltration and photonic crystals for exosome isolation via CD63 aptamer and quantification, achieving an LOD of 8.9×103 EVs mL−1 in a 20-μL sample volume. This method distinguished serum samples from breast cancer patients and healthy donors with high sensitivity. Alternatively, Lu et al.249 introduced a DNA computation-mediated microfluidic tandem separation platform for isolating PD-L1-positive EV sub-populations via dual-aptamer recognition. The platform achieved highly efficient sequential isolation, providing insights into immuno-heterogeneity and enabling downstream proteomic analysis with high specificity and efficiency. In summary, sensitive, non-invasive, and high-throughput detection of both exosomes and EVs are now realized on novel IMC using aptamers (Table 8).
5.6 Bacteria and mammalian cells
5.6.1 Bacteria.
Aptamers and microfluidic-based technologies can rapidly detect bacteria. For instance, Abdelrasoul et al.250 developed a non-faradaic impedance biosensor functionalized with DNA aptamers targeting E. coli, utilizing interdigitated electrodes. The biosensor demonstrated an LOD of 9 CFU mL−1, and the system's performance showed a linear range from 25 to 1000 CFU mL−1, offering high sensitivity and selectivity for bacterial detection. Similarly, Hao et al.182 developed an IMS functionalized with poamidoamine dendrimers conjugated with aptamers for enhanced E. coli O157:H7 detection. The system achieved an LOD of 100 CFU mL−1, with the generation 7 dendrimer channels providing better performance compared to generation 4 dendrimer channels due to more aptamer binding sites. Furthermore, Kavruk et al.251 introduced aptamer-decorated magnetic silica microparticles in a microfluidic platform for purifying S. pneumoniae. The system achieved a capture efficiency of 87% in phosphate buffered saline (PBS) and 66% in blood, demonstrating high specificity and effectiveness in complex matrices (Table 8).
Furthermore, Liu et al.252 developed a dual-channel fluorescent biosensor using aptamer-functionalized carbon dots to detect Campylobacter and Aliarcobacter. The system exhibited an LOD of 1 CFU mL−1 and RGB color detection achieved a detection limit of 5 CFU mL−1 within 65 min when integrated into an IMS.252 Additionally, Man et al.253 introduced a microfluidic colorimetric biosensor using gold nanoparticles and aptamer linked thiolated polystyrene microspheres to detect Salmonella in fresh vegetables. The platform had an LOD of 60 CFU mL−1, with recovery rates between 92 and 114%, making it highly effective for food analysis. In addition, Manfredini et al.254 utilized aptamers for detecting Bacillus subtilis in soil, utilizing a toggle-cell SELEX method for aptamer selection. The aptamers showed strong binding affinity with a Kd in the nM-range, allowing for effective monitoring of bioinoculants in diverse soil environments254 (Table 8).
Moreover, Suo et al.255 developed an aptamer-coated MB-based IMC with digital PCR for the detection of Salmonella typhimurium that achieved an LOD of 90 CFU per reaction with a capture efficiency of 95% in ∼2 h.255 Similarly, Wang et al.256 designed a biosensor using aptamer-functionalized superparamagnetic nanoparticles coupled with carbon dots to detect Helicobacter pylori. This system demonstrated a detection range of 10–107 CFU mL−1 at an LOD of 1 CFU mL−1.256 Alternatively, Wu et al.257 utilized a nitrocellulose membrane-based microfluidic system with magnetic-composite membranes for detecting Acinetobacter baumannii by using specific aptamer, achieving an LOD of 450 CFU per reaction in 40 min. Zhang et al.258 introduced a capillary microfluidic chip with aptamer-modified MBs manipulated by an external magnetic field for efficiently capturing Vibrio parahaemolyticus within 40 min, and Zhou et al.259 developed a portable dual-aptamer microfluidic chip for the detection of Bacillus cereus in 1 h at an LOD of 9 CFU mL−1. Zhu et al.260 presented a tetrahedral DNA framework-engineered aptasensing microfluidic system for rapidly detecting E. coli O157, achieving an LOD of 10 CFU mL−1. Finally, Zhu et al.261 designed a pump-free paper/PDMS hybrid microfluidic chip for bacteria enrichment via aptamer and detection, demonstrating an LOD of 10 CFU mL−1 across a detection range of 10–1000 CFU mL−1 in <20 min. These studies demonstrate that even low concentrations of bacteria can be detected using aptamer- and microfluidics-based technologies in near-real-time (Table 8).
5.6.2 CTCs.
CTCs are rare cancer cells shed from tumors into the bloodstream and hold potential for cancer diagnosis and monitoring.265,266 The primary challenge in CTC detection is their low concentration: 1–10 CTCs mL−1 of blood in metastatic patients.265 Aptamers show promise in isolating CTCs,267 though obstacles persist, including CTC heterogeneity, viability concerns, and the need for improved sensitivity and specificity.268,269 Recent research on microfluidic systems for CTC capture has advanced. For instance, one study isolated CTCs from biological fluids with 80% efficiency using EpCAM alongside vimentin monoclonal antibodies and Fc6 aptamers.270 Hepatocellular carcinoma CTCs were captured 84% efficiently at 95% purity using a SERS-aptamer chip.271 Using magnetic nanoparticles functionalized with MUC1 aptamers isolated CTCs with comparable efficiency to commercial kit in clinical samples.272 An aptamer-cocktail (EpCAM + vimentin + EGFR + CD44) nano-microfluidic chip captured 71–83% of breast cancer CTCs.273 Another group employed a deterministic lateral displacement (DLD) micropillar array integrated with a the multimarker aptamer cocktail DNA nanostructures (TP-multi-marker) DNA nanostructure on an IMC to enhance CTC capture.274 The TP-multi-marker chip achieved 90% capture efficiency for MDA-MB-231 cells (& >90% for MCF-7 cells), outperforming the TP-3 EpCAMs and aptamer chips.274 In blood the TP-multi-marker chip maintained efficiencies >90%, with minimal nonspecific white blood cell binding (0.04%). These methods show how microfluidic + aptamer-based systems can improve CTC detection274 (Table 9).
Table 9 List of research works using aptamers cells and more complex objects
Targets |
Microfluidic methodology |
Detection performance |
Ref. |
Cancer cell, CTCs |
Microfluidic Chip, AuNP, monoclonal antibody-aptamer conjugation; AuNP-Fc6-mAb |
K
d for AuNP-Apt-mAb: ∼0.17 nM, better affinity than conjugated with either Apt or mAb; capture efficiency using AuNP-Apt-mAb was significantly higher than the other conjugates. Capture efficiency: 80% from clinical samples |
270
|
MCF-7 and Escherichia coli O157 |
Magnetic spatial confinement microfluidic strategy; microfluidic magnetic cell immunoassays (μMCI) |
LOD: 2 cells per mL (MCF-7), 34 CFU mL−1 (E. coli O157); satisfactory selectivity and reproducibility |
275
|
Cancer cell, hepatocellular carcinoma (HCC), CTCs |
Surface-enhanced Raman scattering (SERS)-aptamer based microfluidic chip |
Capture efficiency: 84%, purity: 95% |
271
|
Cancer cell, MCF-7, MDA-MB-231, HUVEC, CAF |
Aptamer-targeted magnetic nanoparticles |
Capture efficiency: comparable to commercial kit for clinical samples; capture efficiency >91% after 60 min when added 50 mg mL−1 Apt-MNPs with 10 to 106 cancer cells |
272
|
A549 cells – captured by IDA aptamer (integrin α6β4) |
Microfluidic electrochemical aptasensor |
LOD: 14 cells per mL, dynamic range: 50–5 × 105 cells per mL, sensitivity for A549 cells |
276
|
Cancer cell, specific substances |
Dynamically deformable microfilter |
High selective separation with dynamic deformation; elastic deformation of 40 to 820 μm at flow rates of 5–40 ml min−1; precise size-selective capture |
277
|
Cancer cell, CTCs, breast cancer |
Aptamer-cocktail functionalized nano-microfluidic chip |
Capture efficiency: 71–83% for CTCs; 100% detection rate in breast cancer patients; CTC detection range of 6–33 cells per 2 ml of blood |
273
|
Cancer cell, CTC, breast cancer |
Heterovalent DNA framework recognition element-functionalized microfluidic chip; deterministic lateral displacement (DLD) pattern |
Improved capture efficiency (>80%) of CTCs through deterministic lateral displacement and multimarker aptamer-based synergy |
274
|
HepG2-derived CTCs |
Hybrid microfluidic device with passive viscoelastic and active magnetophoretic separation; device with aptamer-functionalized diatom frustules |
CTC recovery rate: 94.6%, purity: 89.7% |
278
|
Human lung adenocarcinoma cells |
Microfluidic platform with aptamer-functionalized self-assembled monolayer (SAM) |
Cell binding coefficient: >90%; capture efficiency not directly quantified; selective capture of A549 cells confirmed |
279
|
Circulating tumor cells (CTCs) |
DNA-programmed orientation-ordered multivalent microfluidic interface; cubic DNA nanostructure (CDN)-programmed strategy to precisely control the orientation and valency of the aptamer on a microfluidic interface (CDN-Apt-Chip) |
High selectivity for CTCs; enhanced capture efficiency (>80%) with CDN-Apt: binding affinity improved; successfully isolated CTCs from patient blood |
280
|
Lung cancer cells (A549) |
Immunomagnetic separation with varying magnetic bead size; serpentine microchannel with added cavities (SMAC) structure |
Effective capture rate: up to 88.4% for 4.50 μm beads; efficiency increases with bead size |
281
|
Lung adenocarcinoma cells |
Immunomagnetic separation in serpentine microchannel |
Capture rate >70% for A549 cells in less than 15 minutes; 4% capture of non-target cells |
282
|
Cancerous cells |
Dielectrophoresis-based microfluidic platform |
Capture specificity up to 80.4% for A549 cells; LOD: 2 × 104 cells per mL |
283
|
Lung carcinoma cells |
Immunomagnetic separation in microfluidic channel |
Capture efficiency: 77.8% |
284
|
Circulating tumor cells |
Tetrahedral DNA framework-based microfluidic technology; aptamer-hybridization chain reaction (Apt-HCR) |
Capture efficiency: 83.3–94.2% for MCF-7 cells, release efficiency: 96.2% and 94.6% viability |
285
|
CTCs |
Fluidic multivalent membrane nanointerface; aptamer functionalized nanovesicles (Apt-nanovesicles); fluidic aptamer functionalized soft and high-affinity nanointerface microfluidic chip (FLASH-Chip) |
Over 70% capture efficiency using artificial clinical samples, 7-fold higher capture efficiency than monovalent aptamer chip, 97.6% CTC viability after release |
286
|
Different phenotypic CTCs (epithelial and mesenchymal) |
Poly(lactic-co-glycolic acid) (PLGA) nanofiber microfluidic device with dual aptamers, captured by dual aptamers targeting EpCAM and N-cadherin |
Capture efficiency: 91% for A2780 cells, 89% for OVCAR-3 cells; release efficiency: 95% (A2780), 88% (OVCAR-3) |
287
|
CCRF-CEM cells (leukemia) |
Thermo-chemo-mechanical coupling hydrogel in microfluidics; immunoaffinity-based cell catch – transport-release thermo-chemo-mechanical coupling hydrogel (iCatch) |
Catching efficiency: ∼40%, recovery rate: ∼70%, high-throughput (∼900 cells per mm s−1) platform |
288
|
Single circulating tumor cells (CTCs) - captured by dual multivalent aptamers |
Dual-multivalent aptamer-conjugated nanoprobes; dual- multivalent-aptamers (DMAs), Sgc8 and SYL3C |
Sorting separation rate: 93.6%; Kd: 0.26–0.34 nM; measurement efficiency: 73.8% |
289
|
3D tumor spheroids (MCF-7, A549) |
Aptamer-modified gold nanoshells; photothermal therapy (PTT); NIR-absorbing hollow gold nanoshells; anti-MUC1 aptamer; HGN@anti-MUC1 |
PTT efficiency increased by double irradiation; viability decreased by up to 69% (A549) |
148
|
Cryptosporidium parvum oocysts
|
Microfluidic electrochemical biosensor with gold nano-/microislands (NMIs) |
LOD: 5 oocysts per mL (buffer); LOD: 10 oocysts per mL (stool, tap water); detection range: 10–100 000 oocysts per mL |
290
|
Bone microenvironment cells |
Multicomponent bone-on-a-chip with TNF-α aptamer |
Therapeutic potential of TNF-α aptamer in reducing TNF-α-induced cell damage in glucocorticoid-induced osteonecrosis model |
291
|
A hybrid device featuring aptamer-functionalized diatom frustules recovered 95% and purified 90% of HepG2-derived CTCs,278 while a cubic DNA nanostructure-programmed interface enhanced multivalent aptamer on isolating CTCs.280 Multivalent CTC capture and release have been achieved with a tetrahedral DNA framework, combining aptamer-triggered hybridization chain reaction (apt-HCR) for sensing.285 Another fluidic multivalent membrane nanointerface with aptamer-functionalized leukocyte membrane nanovesicles improved CTC enrichment and recovery.286 poly lactic-co-glycolic acid (PLGA) nanofiber devices with dual-EpCAM and N-cadherin aptamers captured 91 and 89% of A2780 and OVCAR-3 cells, respectively.287 Additionally, dual-multivalent aptamer-conjugated nanoprobes captured single CTCs with high specificity.289 In conclusion, CTCs are now readily isolated by microfluidic technologies via aptamers (Table 9).
5.6.3 Lung cancer cells.
Microfluidic cancer detection has advanced lung carcinoma and adenocarcinoma cell diagnostics. For instance, a microfluidic electrochemical aptasensor detected A549 cells with a sensitivity of 14 cells per mL using Integrin α6β4-specific DNA Aptamer (IDA) aptamers targeting integrin α6β4.276 Another platform with aptamer-functionalized self-assembled monolayers bound lung adenocarcinoma cells at >90%.279 Immunomagnetic separation in serpentine microchannels with aptamer-conjugated MBs captured 70% of adenocarcinoma cells282 and 78% of A549 lung carcinoma cells in a microfluidic channel.284 These studies demonstrate that microfluidic methods using aptamers are capable of lung cancer cell detection (Table 9).
5.6.4 Other cancer cells.
Magnetic spatial confinement effectively detected MCF-7 and E. coli O157 using aptamer-functionalized MBs.275 A dynamically deformable microfilter functionalized with aptamer was developed for selective separation by adjusting pore size under hydrodynamic forces.277 Using 4.5 μm aptamer modified MBs, 88% of lung cancer cells were captured.281 A dielectrophoresis-based platform using aptamer-functionalized gold nanoparticles demonstrated high cancer cell capture efficiency,283 while a thermo-chemo-mechanical hydrogel system efficiently sorted leukemia cells.288 These studies demonstrate that diverse microfluidic methods are capable of cancer cell sensing (Table 9).
5.7 More complex objects
Developments in microfluidic systems have demonstrated the versatility and therapeutic potential of aptamer-based approaches. For instance, a recent study found that aptamer-modified gold nanoshells improved photothermal therapy efficiency, reducing 3D tumor spheroid viability by up to 69%.148 An aptamer based electrochemical microfluidic biosensor with gold nano-/micro-islands detected Cryptosporidium parvum at only 5 oocysts per mL in buffer and 10 oocysts per mL in stool/water.290 Furthermore, a bone-on-a-chip platform291 revealed the protective effects of TNF-α DNA aptamers on endothelial cells against glucocorticoid-induced damage in an osteonecrosis model. Microfluidic organ-on-chip platforms have also highlighted the versatility of aptamers in developing personalized medicine, modeling complex systems, and testing therapeutic agents50,292 (Table 9).
6. Microfluidics for aptamers in therapeutic applications
6.1 Aptamers and their applications in therapeutic applications
Several internationally recognized firms, including Aptamer Group, Base Pair Biotechnologies, Aptagen, SomaLogic, NeoVentures Biotechnology, Aptamer Sciences, TriLink BioTechnologies, and Vivonics, are engaged in the development of aptamers. Their efforts are directed towards agriculture, food safety, cancer diagnostics, drug development, and biosensing markets.6 Notable examples of FDA-approved aptamers include Izervay (Astellas Pharma)293 and Macugen (Pfizer),294 both of which are utilized in the treatment of macular degeneration. Furthermore, significant advancements are being made in other aptamers as therapeutic agents.16 For instance, AS1411 is currently in a phase-II clinical trial for treating metastatic renal cell carcinoma.295 Aptamers have also been utilized in the identification of several endemic and epidemic diseases, such as cholera,296 Crimean-Congo hemorrhagic fever,297 meningitis,298 sepsis,299 tuberculosis,300 Ebola,301 influenza,302 MERS-CoV,303 SARS-CoV-2,42 monkeypox,304 smallpox,305 and Zika/Dengue.306
6.2 Microfluidic technologies in aptamer development
Microfluidic technologies have revolutionized the screening of aptamers by providing miniaturized, integrated platforms for high-throughput, cost-effective, and precise screening processes. These systems simplify the aptamer selection process by combining synthesis, modification, and screening in a single device, greatly increasing screening efficiency. Rapid optimization of high-specificity and high-affinity aptamers is now promising for therapeutic applications such as targeted drug delivery and diagnostics, where precision and efficiency are critical. Integrated microfluidic systems (IMS) have accelerated aptamer development by automating processes that were previously carried out in resource-intensive settings. These platforms therefore provide precise control over reaction conditions, reduce sample and reagent consumption, and speed up discovery timelines. Their compact design allows for portability and usability in both clinical and laboratory settings. By taking these advantages, microfluidics provides a fast and efficient approach to aptamer development, meeting the growing demand for advanced therapeutic solutions.
6.3 Therapeutic aptamer screening and development
6.3.1 Recent innovations in aptamer drug-screening.
Aptamers have been demonstrated for drug-screening applications on microfluidic systems. For instance, Balachandran et al.307 developed a platform for the synthesis of aptamer-functionalized BioZIF-8 targeting lymph nodes and tumors on an IMC in a two-stage process, reducing synthesis time from 15 h to only 10 min and encapsulating more biomolecules compared to conventional methods. This platform also highlighted microfluidics' ability to reduce time and resources in aptamer development. Alternatively, Rouco et al.308 demonstrated IMS effectiveness in surface modification of lipid-polymer hybrid nanoparticles, with microfluidics producing smaller particles (129 ± 1 nm) and a higher positive zeta potential (51 ± 1 mV). While microfluidics offered better control over particle synthesis and higher aptamer association efficiency (91 ± 2%), it was more complex and costly compared to traditional methods.308 Moreover, Shan et al.309 used a microfluidic mixing device (MMD) for small (diameter = 129 ± 5.5 nm) liposome formation at high encapsulation efficiency (90 ± 1.4%) for aptamers. Despite challenges in microchannel resolution and high fabrication costs, the MMD improved reproducibility and scalability, and even permitted successful in vivo tumor targeting and imaging.
6.4 Therapeutic applications of microfluidics-screened aptamers
6.4.1 Aptamers in personalized medicine.
Recent advancements in microfluidic systems have expanded the therapeutic applications of aptamers. For example, Xu et al.310 integrated microfluidics into a liquid biopsy-guided drug release system for precision tumor therapy via aptamers. The study featured a magnetically controlled IMC that integrated CTC detection using aptamer-functionalized magnetic nanospheres with controlled drug release, adjusting doxorubicin delivery based on the number of captured tumor cells. This system provided high specificity for personalized cancer treatment, though its complexity, reliance on EpCAM expression, and potential microchannel fouling may limit clinical scalability. Yi et al.311 highlighted that aptamer-functionalized exosomes combined with microfluidic chip, fluorescence-based detection, and SPR sensing for precise tumor targeting and drug delivery achieved high sensitivity in detecting tumor-derived exosomes (LOD ∼ 105 particles per mL). While this low-immunogenicity approach offers real-time, high-throughput analysis, challenges still remain in the time-consuming preparation of exosomes, lack of standardization, and expensive instrumentation required.
6.4.2 Microfluidic-aptamer synergies in targeted therapy.
The role of microfluidics in targeted therapy has been explored in thrombolysis studies for targeted therapy. For instance, one group312 utilized a microfluidic model of arterial occlusion to assess the thrombolytic efficacy of the RNA aptamer BB-031, which targeted von Willebrand Factor, to inhibit platelet aggregation, showing the most effective thrombolysis at 1692 nM BB-031.312 The microfluidic system demonstrated a 17% reduction in thrombus surface area and 60% patency at this dose, but higher doses (3384 nM) were less effective, indicating potential off-target effects. Another group rapidly isolated anti-idiotype aptamers for SARS-CoV-2 spike protein quantification,313 reducing the selection process to just five rounds in two days, and integrated these aptamers with graphene field-effect transistor (GFET) nanosensors for real-time, label-free detection in serum.313 The aptamers exhibited strong binding affinities (Kd from 32 ± 7 to 130 ± 25 nM) with detection limits as low as 1 pM, though the specialized equipment required for microfluidics and GFET may limit broader clinical accessibility.313 It may be further used targeting SARS-CoV-2 viruses.
6.5 Clinical translation of aptamer-based therapeutics
Microfluidic systems have played a critical role in translating aptamer-based research to the clinic. For example, Nguyen et al.314 developed a real-time monitoring platform for assessing drug responses in tumor biopsies, demonstrating the clinical relevance of aptamer-based therapies. This study employed a multi-well electrochemical aptasensor platform integrated with an IMC for label-free monitoring of apoptosis in micro-dissected tumor biopsies, detecting cytochrome C at a Kd of 6.2 ± 1.6 ng mL−1 across a dynamic range of 1 to 30
000 ng mL−1. While the platform offered precise, high-throughput monitoring, its slow dissociation rate and complex fabrication might thwart widespread clinical use. Similarly, Zhu et al.315 used aptamer-modified nanosubstrates to monitor lung cancer progression. Their polyethylene glycol-poly lactic acid-co-glycolic acid (PEG-PLGA) nanofiber microfluidic system, supplemented with a cocktail of aptamers, achieved >85% capture efficiency across four lung cancer cell lines and detected 3.5-fold more CTCs in mixed samples compared to traditional methods, which may be useful for theropetics.315 While offering high sensitivity and specificity for real-time monitoring of clonal evolution, the platform's complexity in aptamer conjugation and the need for customized aptamers may limit its scalability. Nevertheless, these studies depict how microfluidics may facilitate the transition of aptamer research from preclinical stages to clinical therapies, significantly enhancing the precision and effectiveness of personalized medicine.
6.6 Advantages and challenges of microfluidics in aptamer-based therapeutics
While microfluidic systems have made significant advances in aptamer-based therapeutics, several challenges still remain. For instance, handling diverse biological samples such as primary cell cultures may introduces challenges, which may hinder reproducibility and consistency across experiments. These issues are especially important in therapeutic applications, where high specificity and reproducibility are required for clinical use. Furthermore, the technical complexities of designing and operating IMS might present significant challenges. These systems frequently require specialized core knowledge and advanced equipment, making them inaccessible to research teams without extensive technical expertise. Moreover, the lack of standardization across microfluidic platforms also impedes scalability and clinical translation, complicating their integration into regulatory frameworks and real-world applications. Despite these challenges, ongoing advances in microfluidic design and automation show promise for overcoming these limitations. Improved system integration, reproducibility, and the development of standardized protocols will be critical to increasing the use of microfluidics in therapeutic settings. By overcoming these challenges, microfluidic systems can realize their full potential as transformative tools in the development of targeted aptamer-based treatments.
7. Challenges and perspectives
The traditional, on-bench SELEX process was once the cornerstone of aptamer selection having 1) been widely adopted over the past three decades and 2) paved the way for extensive exploration and application of these unique molecular probes. The major challenges of traditional SELEX can be summarized as follows. Firstly, binding of ssDNA libraries with target molecules is lengthy and inefficient. More importantly, the shear force during the binding process cannot be well controlled. Secondly, incomplete removal of unbound ssDNA and weakly-bound ssDNA due to residue issues in test tubes, which may be amplified by the subsequent round of PCR, reduces efficacy of screening process. Thirdly, the amplification of bound ssDNA requires off-chip PCR and human intervention, which cannot be automated. As a result, the total number of on-bench screening rounds may be at least 10–20, which may take weeks-months. More importantly, tedious post-SELEX processes are usually inevitable which can result in modified aptamer structures that then compromise their use in diverse applications. Furthermore, the number of screened candidates can be in the hundreds, which requires a large efforts and resources to identify the ones with optimal affinity and specificity.
Of these, the prolonged operational duration represents the most significant obstacle, and the one best-addressed by IMS over the past decade, as summarized below. Firstly, nano-beads with high surface-to-volume (S/V) ratios surface-coated with target molecules were used to enhance target-ssDNA binding within a short period of time (10–20 min for on-chip processes vs. weeks-months for on-bench processes), leading to a higher proportion of suitable aptamer candidate (dozens for on-chip processes vs. hundreds for traditional SELEX). Bead-based protocols which be easily performed on microfluidic platforms may result in confirmed, limited candidates with higher performance in terms of affinity and specificity. Secondly, optimization of washing stringency, such as by controlling shearing forces generated by the micropumps (in the range of pN, which is close to the binding force between ssDNA & proteins) leads to more effective removal of non-specific or low-affinity candidates after binding processes. Thirdly, on-chip PCR integrated with the other functional microfluidic devices may eliminate off-chip processes and might reduce human intervention to where the entire screening process can be automated on a single chip. As a result, the lower samples and reagent volumes also lead to 10-fold or greater reductions in price.
To enhance the specificity of the screened aptamers, both negative selection using other proteins abundant in bio-samples and competitive selection using biosamples (such as blood or serum) were developed on microfluidic platforms. More importantly, by using AI-based bioinformatics (such as AlphaFold) to predict aptamer structures, binding sites, docking scores, hydrogen bond numbers, receptor–ligand numbers, free energies, interactive amino acid numbers, and nucleotide/total nucleotide ratios to target high-affinity/high-specificity aptamers from selected pools and binding of screened aptamers with target proteins, tedious post-SELEX processes can be significantly alleviated. As a result, modified aptamers with higher affinity and specificity may be available for clinical applications. Screened aptamers can be therefore used for rapid, sensitive diagnostics for cardiovascular diseases, cancer, diabetes, infectious diseases and many others by using novel, dual-aptamer assays to replace conventional immunoassays. It is envisioned that high-affinity, high-specificity aptamers may replace conventional antibodies, enabling sensitive detection and quantification of emerging biomarkers for a variety of biological applications.
8. Conclusions
In the past decade, significant advances in microfluidic SELEX have been demonstrated. Microfluidic platforms have transformed the SELEX process by combining synthesis, modification, and screening into a single compact system, allowing for precise control of reaction conditions while significantly reducing time, reagent consumption, and manual intervention. These advancements have enabled high-throughput, cost-effective, and precise aptamer screening, paving the way for a wide range of applications in diagnostics, therapeutics, and biosensing.
This review has introduced the impact of microfluidic technology on SELEX, emphasizing how integrated systems simplify and enhance various steps in the aptamer selection process. Advanced microfluidic methodologies were extensively reviewed for their use in screening aptamers for a variety of targets, including proteins, cells, and complex biological systems. Furthermore, the versatility of aptamers selected through microfluidic SELEX in applications such as personalized medicine and drug delivery was discussed.
Despite these advances, major challenges remain. These include the technical complexity of integrated microfluidic systems, variability in the handling of biological samples, and a lack of standardization across platforms, all of which restrict broader clinical adoption. Future efforts must focus on addressing these challenges by improving bioinformatics tools, standardizing microfluidic SELEX protocols, and creating scalable platforms for real-world use.
Data availability
Data may be available provided by the corresponding author upon request.
Author contributions
Yi-Da Chung: writing - original draft; Yi-Cheng Tsai; writing - original draft; Chi-Hung Wang: writing - original draft; Gwo-Bin Lee: writing - original draft, reviewing and editing.
Conflicts of interest
There are no conflicts to declare.
Acknowledgements
The authors gratefully acknowledge the support from the National Science and Technology Council (NSTC) of Taiwan (grants NSTC 113-2218-E-007-027 and NSTC 112-2221-E-007-017-MY3).
References
- M. Famulok, J. S. Hartig and G. Mayer, Chem. Rev., 2007, 107(9), 3715–3743 CrossRef CAS PubMed.
- K. M. Ahmad, S. S. Oh, S. Kim, F. M. McClellen, Y. Xiao and H. T. Soh, PLoS One, 2011, 6(11), e27051 CrossRef CAS PubMed.
- M. Yüce, N. Ullah and H. Budak, Analyst, 2015, 140, 5379–5399 Search PubMed.
- H. Kaur, J. G. Bruno, A. Kumar and T. K. Sharma, Theranostics, 2018, 8(15), 4016–4032 CrossRef CAS PubMed.
- C. H. Weng, C. J. Huang and G. B. Lee, Sensors, 2012, 12(7), 9514–9529 CrossRef CAS PubMed.
- K.-M. Song, S. Lee and C. Ban, Sensors, 2012, 12(1), 612–631 CrossRef.
- W. H. Thiel, T. Bair, A. S. Peek, X. Liu, J. Dassie, K. R. Stockdale, M. A. Behlke, F. J. Miller Jr and P. H. Giangrande, PLoS One, 2012, 7(9), e43836 CrossRef CAS.
- A. B. Iliuk, L. Hu and W. A. Tao, Anal. Chem., 2011, 83(12), 4440–4452 CrossRef CAS.
- D. Xiang, C. Zheng, S. F. Zhou, S. Qiao, P. H. L. Tran, C. Pu, Y. Li, L. Kong, A. Z. Kouzani, J. Lin, K. Liu, L. Li, S. Shigdar and W. Duan, Theranostics, 2015, 5(10), 1083–1097 CrossRef CAS PubMed.
- L. Y. Wan, W. F. Yuan, W. B. Ai, Y. W. Ai, J. J. Wang, L. Y. Chu, Y. Q. Zhang and J. F. Wu, Expert Opin. Drug Delivery, 2019, 16(3), 207–218 CrossRef CAS.
- S. Ni, Z. Zhuo, Y. Pan, Y. Yu, F. Li, J. Liu, L. Wang, X. Wu, D. Li, Y. Wan, L. Zhang, Z. Yang, B.-T. Zhang, A. Lu and G. Zhang, ACS Appl. Mater. Interfaces, 2021, 13(8), 9500–9519 CrossRef CAS PubMed.
- H. Sun and Y. Zu, Molecules, 2015, 20(7), 11959–11980 CrossRef CAS PubMed.
- N. K. Jain, H. C. Jetani and I. Roy, Pharm. Res., 2013, 30, 1871–1882 CrossRef CAS PubMed.
- J. Zhong, J. Ding, L. Deng, Y. Xiang, D. Liu, Y. Zhang, X. Chen and Q. Yang, Drug Des., Dev. Ther., 2021, 15, 3985–3996 CrossRef PubMed.
- S. Liu, Y. Xu, X. Jiang, H. Tan and B. Ying, Biosens. Bioelectron., 2022, 208, 114168 CrossRef CAS PubMed.
- J. Zhou and J. Rossi, Nat. Rev. Drug Discovery, 2017, 16, 181–202 CrossRef CAS PubMed.
- T. Wang, C. Chen, L. M. Larcher, R. A. Barrero and R. N. Veedu, Biotechnol. Adv., 2019, 37(1), 28–50 CrossRef CAS PubMed.
- H. Sun and Y. Zu, Small, 2015, 11(20), 2352–2364 CrossRef CAS PubMed.
- A. Ruscito and M. C. DeRosa, Front. Chem., 2016, 4, 14 Search PubMed.
- Y. Zhang, B. S. Lai and M. Juhas, Molecules, 2019, 24(5), 941 CrossRef PubMed.
- M. Darmostuk, S. Rimpelova, H. Gbelcova and T. Ruml, Biotechnol. Adv., 2015, 33(6, Pt 2), 1141–1161 CrossRef CAS PubMed.
- Z. Zhuo, Y. Yu, M. Wang, J. Li, Z. Zhang, J. Liu, X. Wu, A. Lu, G. Zhang and B. Zhang, Int. J. Mol. Sci., 2017, 18(10), 2142 CrossRef PubMed.
- S. I. Hori, A. Herrera, J. J. Rossi and J. Zhou, Cancers, 2018, 10(1), 9 CrossRef PubMed.
- H. Lin, W. Zhang, S. Jia, Z. Guan, C. J. Yang and Z. Zhu, Biomicrofluidics, 2014, 8(4), 041501 CrossRef PubMed.
- C. Tuerk and L. Gold, Science, 1990, 249(4968), 505–510 CrossRef CAS PubMed.
- A. D. Ellington and J. W. Szostak, Nature, 1990, 346, 818–822 CrossRef CAS PubMed.
- M. D. Leonida and I. Kumar, Curr. Nanosci., 2012, 8(3), 458–473 CrossRef CAS.
- G. T. Vladisavljević, N. Khalid, M. A. Neves, T. Kuroiwa, M. Nakajima, K. Uemura, S. Ichikawa and I. Kobayashi, Adv. Drug Delivery Rev., 2013, 65(11–12), 1626–1663 CrossRef PubMed.
- D. G. Rackus, M. H. Shamsi and A. R. Wheeler, Chem. Soc. Rev., 2015, 44, 5320–5340 RSC.
- A. G. Niculescu, C. Chircov, A. C. Bîrcă and A. M. Grumezescu, Int. J. Mol. Sci., 2021, 22(4), 2011 CrossRef CAS PubMed.
- T. M. Squires and S. R. Quake, Rev. Mod. Phys., 2005, 77, 977–1026 CrossRef CAS.
- P. N. Nge, C. I. Rogers and A. T. Woolley, Chem. Rev., 2013, 113(4), 2550–2583 CrossRef CAS PubMed.
- M. J. Jebrail, M. S. Bartsch and K. D. Patel, Lab Chip, 2012, 12, 2452–2463 RSC.
- J. B. Nielsen, R. L. Hanson, H. M. Almughamsi, C. Pang, T. R. Fish and A. T. Woolley, Anal. Chem., 2020, 92(1), 150–168 Search PubMed.
- R. Vitorino, S. Guedes, J. P. da Costa and V. Kašička, Nanomaterials, 2021, 11(5), 1118 CrossRef CAS PubMed.
- C.-J. Huang, H.-I. Lin, S.-C. Shiesh and G.-B. Lee, Biosens. Bioelectron., 2010, 25(7), 1761–1766 CrossRef CAS.
- T. Olsen, J. Zhu, J. Kim, R. Pei, M. N. Stojanovic and Q. Lin, SLAS Technol., 2017, 22(1), 63–72 CrossRef PubMed.
- D. N. Mazaafrianto, M. Maeki, A. Ishida, H. Tani and M. Tokeshi, Micromachines, 2018, 9(5), 202 CrossRef.
- W.-T. Liu, W.-B. Lee, Y.-C. Tsai, Y.-J. J. Chuang, K.-F. Hsu and G.-B. Lee, Biomicrofluidics, 2019, 13(1), 014114 CrossRef.
- C.-S. Lin, Y.-C. Tsai, K.-F. Hsu and G.-B. Lee, Lab Chip, 2021, 21, 725–734 RSC.
- R. Gandotra, H. B. Wu, P. Gopinathan, Y. C. Tsai, F. C. Kuo, M. S. Lee and G. B. Lee, Lab Chip, 2022, 22, 250–261 RSC.
- H.-B. Wu, C.-H. Wang, Y.-D. Chung, Y.-S. Shan, Y.-J. Lin, H.-P. Tsai and G.-B. Lee, Anal. Chim. Acta, 2023, 1274, 341531 CrossRef CAS PubMed.
- R. Gandotra, H. B. Wu, F. C. Kuo, M. S. Lee and G. B. Lee, ACS Sens., 2024, 9(4), 1775–1784 CrossRef CAS PubMed.
- Y. Xu, X. Yang and E. Wang, Anal. Chim. Acta, 2010, 683(1), 12–20 CrossRef CAS PubMed.
- S. K. Dembowski and M. T. Bowser, Analyst, 2018, 143, 21–32 RSC.
- S. D. Jayasena, Clin. Chem., 1999, 45(9), 1628–1650 CrossRef CAS.
- A. Ozer, J. M. Pagano and J. T. Lis, Mol. Ther.--Nucleic Acids, 2014, 3, e183 CrossRef CAS PubMed.
- M. Blind and M. Blank, Mol. Ther.--Nucleic Acids, 2015, 4, e223 CrossRef PubMed.
- P. Bayat, R. Nosrati, M. Alibolandi, H. Rafatpanah, K. Abnous, M. Khedri and M. Ramezani, Biochimie, 2018, 154, 132–155 CrossRef CAS PubMed.
- B. J. Hicke, C. Marion, Y. F. Chang, T. Gould, C. K. Lynott, D. Parma, P. G. Schmidt and S. Warren, J. Biol. Chem., 2001, 276(52), 48644–48654 CrossRef CAS PubMed.
- D. A. Daniels, H. Chen, B. J. Hicke, K. M. Swiderek and L. Gold, Proc. Natl. Acad. Sci. U. S. A., 2003, 100(26), 15416–15421 CrossRef CAS PubMed.
- D. Shangguan, Y. Li, Z. Tang, Z. C. Cao, H. W. Chen, P. Mallikaratchy, K. Sefah, C. J. Yang and W. Tan, Proc. Natl. Acad. Sci. U. S. A., 2006, 103(32), 11838–11843 CrossRef CAS PubMed.
- M. Ye, J. Hu, M. Peng, J. Liu, J. Liu, H. Liu, X. Zhao and W. Tan, Int. J. Mol. Sci., 2012, 13(3), 3341–3353 CrossRef CAS PubMed.
- L.-Y. Hung, C.-Y. Fu, C.-H. Wang, Y.-J. Chuang, Y.-C. Tsai, Y.-L. Lo, P.-H. Hsu, H.-Y. Chang, S.-C. Shiesh, K.-F. Hsu and G.-B. Lee, Biomicrofluidics, 2018, 12(5), 054108 CrossRef PubMed.
- S. D. Mendonsa and M. T. Bowser, J. Am. Chem. Soc., 2004, 126(1), 20–21 Search PubMed.
- M. Berezovski and S. N. Krylov, J. Am. Chem. Soc., 2002, 124(46), 13674–13675 CrossRef CAS PubMed.
- C. Zhu, G. Yang, M. Ghulam, L. Li and F. Qu, Biotechnol. Adv., 2019, 37(8), 107432 CrossRef CAS PubMed.
- R. Stoltenburg, N. Nikolaus and B. Strehlitz, J. Anal. Methods Chem., 2012, 2012, 415697 Search PubMed.
- N. Nikolaus and B. Strehlitz, Sensors, 2014, 14(2), 3737–3755 CrossRef PubMed.
- J. G. Bruno, Biochem. Biophys. Res. Commun., 1997, 234(1), 117–120 CrossRef CAS PubMed.
- X. Lou, J. Qian, Y. Xiao, L. Viel, A. E. Gerdon, E. T. Lagally, P. Atzberger, T. M. Tarasow, A. J. Heeger and H. T. Soh, Proc. Natl. Acad. Sci. U. S. A., 2009, 106(9), 2989–2994 CrossRef CAS PubMed.
- I. Manea, M. Casian, O. Hosu-Stancioiu, N. de-Los-Santos-Álvarez, M. J. Lobo-Castañón and C. Cristea, Anal. Chim. Acta, 2024, 1297, 342325 CrossRef CAS PubMed.
- G. Hybarger, J. Bynum, R. F. Williams, J. J. Valdes and J. P. Chambers, Anal. Bioanal. Chem., 2006, 384, 191–198 CrossRef CAS PubMed.
- R. K. Mosing and M. T. Bowser, J. Sep. Sci., 2007, 30(10), 1420–1426 CrossRef CAS PubMed.
- G. Aquino-Jarquin and J. D. Toscano-Garibay, Int. J. Mol. Sci., 2011, 12(12), 9155–9171 CrossRef CAS PubMed.
- C. Zhu, Z. Feng, H. Qin, L. Chen, M. Yan, L. Li and F. Qu, Talanta, 2024, 266(Pt 1), 124998 CrossRef CAS PubMed.
- Z. Fang, X. Feng, F. Tang, H. Jiang, S. Han, R. Tao and C. Lu, Biosensors, 2024, 14(7), 350 CrossRef CAS PubMed.
- N. K. Singh, Y. Wang, C. Wen, B. Davis, X. Wang, K. Lee and Y. Wang, Nat. Biotechnol., 2023, 42, 1224–1231 CrossRef PubMed.
- L. Y. Hung, C. H. Wang, C. Y. Fu, P. Gopinathan and G. B. Lee, Lab Chip, 2016, 16, 2759–2774 RSC.
- L. A. Fraser, Y.-W. Cheung, A. B. Kinghorn, W. Guo, S. C.-C. Shiu, C. Jinata, M. Liu, S. Bhuyan, L. Nan, H. C. Shum and J. A. Tanner, Adv. Biosyst., 2019, 3(5), 1900012 CrossRef PubMed.
- Y. Liu, N. Wang, C.-W. Chan, A. Lu, Y. Yu, G. Zhang and K. Ren, Front. Cell Dev. Biol., 2021, 9, 730035 CrossRef PubMed.
- L. Gold, B. Polisky, O. Uhlenbeck and M. Yarus, Annu. Rev. Biochem., 1995, 64, 763–797 CrossRef CAS.
- Y. Yu, C. Liang, Q. Lv, D. Li, X. Xu, B. Liu, A. Lu and G. Zhang, Int. J. Mol. Sci., 2016, 17(3), 358 CrossRef PubMed.
- J. C. Chaput, Acc. Chem. Res., 2021, 54(4), 1056–1065 CrossRef CAS PubMed.
- A. C. Larsen, M. R. Dunn, A. Hatch, S. P. Sau, C. Youngbull and J. C. Chaput, Nat. Commun., 2016, 7, 11235 CrossRef CAS PubMed.
- D. Vallejo, A. Nikoomanzar, B. M. Paegel and J. C. Chaput, ACS Synth. Biol., 2019, 8(6), 1430–1440 CrossRef CAS PubMed.
- A. Nikoomanzar, D. Vallejo, E. J. Yik and J. C. Chaput, ACS Synth. Biol., 2020, 9(7), 1873–1881 CrossRef CAS PubMed.
- M. Cho, S. S. Oh, J. Nie, R. Stewart, M. J. Radeke, M. Eisenstein, P. J. Coffey, J. A. Thomson and H. T. Soh, Anal. Chem., 2015, 87(1), 821–828 CrossRef CAS PubMed.
- Y. Sheng and M. T. Bowser, Analyst, 2013, 139, 215–224 RSC.
- S. H. Lee, J. H. Lee, H. W. Lee, Y.-H. Kim, O. C. Jeong and J.-Y. Ahn, PLoS One, 2016, 11, e0159777 CrossRef PubMed.
- S. H. Lee, H. W. Lee, D. S. Kim, H. G. Kwon, J. H. Lee, Y.-H. Kim, O. C. Jeong and J.-Y. Ahn, J. Visualized Exp., 2018, 141, e57703 Search PubMed.
- D. Chang, Z. Wang, C. D. Flynn, A. Mahmud, M. Labib, H. Wang, A. Geraili, X. Li, J. Zhang, E. H. Sargent and S. O. Kelley, Nat. Chem., 2023, 15, 773–780 CrossRef CAS PubMed.
- C. M. Birch, H. W. Hou, J. Han and J. C. Niles, Sci. Rep., 2015, 5, 11347 CrossRef PubMed.
- J. P. Hilton, T. Olsen, J. Kim, J. Zhu, T. H. Nguyen, M. Barbu, R. Pei, M. Stojanovic and Q. Lin, Microfluid. Nanofluid., 2015, 19, 795–804 CrossRef CAS PubMed.
- T. R. Olsen, C. Tapia-Alveal, K.-A. Yang, X. Zhang, L. J. Pereira, N. Farmakidis, R. Pei, M. N. Stojanovic and Q. Lin, J. Electrochem. Soc., 2017, 164(5), B3122–B3129 CrossRef CAS PubMed.
- T. R. Olsen, C. Tapia-Alveal, K. Wen, T. S. Worgall, M. N. Stojanovic and Q. Lin, Biomed. Microdevices, 2023, 25, 3 CrossRef CAS PubMed.
- J. Kim, T. R. Olsen, J. Zhu, J. P. Hilton, K.-A. Yang, R. Pei, M. N. Stojanovic and Q. Lin, Sci. Rep., 2016, 6, 26139 CrossRef CAS PubMed.
- K. Wen, Y. Chen, X. Meng, S. Botros, W. Dai, M. N. Stojanovic, R. Tomer and Q. Lin, Microchem. J., 2023, 188, 108454 CrossRef CAS PubMed.
- X. Meng, K. Wen, M. Citartan and Q. Lin, Analyst, 2022, 148, 787–798 RSC.
- S. Chung, N. G. Gurudatt, J. Jeon, C. Ban and Y.-B. Shim, Anal. Chem., 2021, 93(3), 1416–1422 CrossRef CAS PubMed.
- Y. Lu, S. Yu, F. Lin, F. Lin, X. Zhao, L. Wu, Y. Miao, H. Li, Y. Deng and L. Geng, Analyst, 2017, 142, 4257–4264 RSC.
- J.-W. Park, S. J. Lee, S. Ren, S. Lee, S. Kim and T. Laurell, Sci. Rep., 2016, 6, 27121 CrossRef CAS PubMed.
- C. Bai, X. Meng, K. Wen, M. Citartan, C. Wang, S. Yu and Q. Lin, Microfluid. Nanofluid., 2022, 26, 43 CrossRef CAS PubMed.
- K. Z. Yang, M. Wang, M. Y. Gao, Y. T. Wang and Z. L. Zhang, Chem. Commun., 2024, 60, 2772–2775 RSC.
- H. Lim, J. Chang, K.-I. Kim, Y. Moon, S. Lee, B. Lee, J. H. Lee and J. Lee, Biomicrofluidics, 2022, 16(4), 044102 CrossRef CAS PubMed.
- D. Chen, Y. Orenstein, R. Golodnitsky, M. Pellach, D. Avrahami, C. Wachtel, A. Ovadia-Shochat, H. Shir-Shapira, A. Kedmi, T. Juven-Gershon, R. Shamir and D. Gerber, Sci. Rep., 2016, 6, 33351 CrossRef CAS PubMed.
- X. Liu, H. Li, W. Jia, Z. Chen and D. Xu, Lab Chip, 2017, 17, 178–185 RSC.
- H.-I. Lin, C.-C. Wu, C.-H. Yang, K.-W. Chang, G.-B. Lee and S.-C. Shiesh, Lab Chip, 2015, 15, 486–494 RSC.
- J. Soundy and D. Day, PLoS One, 2017, 12(9), e0185385 CrossRef PubMed.
- H. Stoll, H. Kiessling, M. Stelzle, H. P. Wendel, J. Schütte, B. Hagmeyer and M. Avci-Adali, Biomicrofluidics, 2015, 9(3), 034111 CrossRef PubMed.
- L.-Y. Hung, C.-H. Wang, K.-F. Hsu, C.-Y. Chou and G.-B. Lee, Lab Chip, 2014, 14, 4017–4028 RSC.
- L. Y. Hung, C. H. Wang, Y. J. Che, C. Y. Fu, H. Y. Chang, K. Wang and G. B. Lee, Sci. Rep., 2015, 5, 10326 CrossRef PubMed.
- P. Gopinathan, L.-Y. Hung, C.-H. Wang, N.-J. Chiang, Y.-C. Wang, Y.-S. Shan and G.-B. Lee, Biomicrofluidics, 2017, 11, 044101 CrossRef PubMed.
- J. Chen, X. Liu, M. Xu, Z. Li and D. Xu, Biomicrofluidics, 2021, 15(2), 024107 CrossRef CAS PubMed.
- E. Dausse, A. Barré, A. Aimé, A. Groppi, A. Rico, C. Ainali, G. Salgado, W. Palau, E. Daguerre, M. Nikolski, J.-J. Toulmé and C. Di Primo, Biosens. Bioelectron., 2016, 80, 418–425 CrossRef CAS PubMed.
- I. Anosova, E. A. Kowal, M. R. Dunn, J. C. Chaput, W. D. V. Horn and M. Egli, Nucleic Acids Res., 2016, 44, 1007–1021 CrossRef CAS PubMed.
- S. Ohuchi, BioRes. Open Access, 2012, 1(6), 265–272 CrossRef CAS PubMed.
- S. Catuogno and C. L. Esposito, Biomedicines, 2017, 5(3), 49 CrossRef.
- J. Dai, J. Li, Y. Jiao, X. Yang, D. Yang, Z. Zhong, H. Li and Y. Yang, Food Chem., 2024, 456, 139955 CrossRef CAS PubMed.
- Z. Khoshbin, M. Moeenfard, K. Abnous and S. M. Taghdisi, Food Chem., 2023, 399, 133983 CrossRef CAS PubMed.
- C.-H. Wang and G.-B. Lee, Biomicrofluidics, 2020, 14(2), 024110 CrossRef CAS PubMed.
- Y. Lu, J. Wen, C. Wang, M. Wang, F. Jiang, L. Miao, M. Xu, Y. Li, X. Chen and Y. Chen, Small, 2024, 20(20), 2308424 CrossRef CAS PubMed.
- C. J. Huang, H. I. Lin, S. C. Shiesh and G. B. Lee, Biosens. Bioelectron., 2012, 35(1), 50–55 CrossRef CAS PubMed.
- A. Sinha, P. Gopinathan, Y. D. Chung, H. Y. Lin, K. H. Li, H. P. Ma, P. C. Huang, S. C. Shiesh and G. B. Lee, Biosens. Bioelectron., 2018, 122, 104–112 CrossRef CAS PubMed.
- S. Shin, B. Kim, Y.-J. J. Kim and S. Choi, Biosens. Bioelectron., 2019, 133, 169–176 CrossRef CAS PubMed.
- K. R. Wehmeyer, R. J. White, P. T. Kissinger and W. R. Heineman, Annu. Rev. Anal. Chem., 2021, 14, 109–131 CrossRef CAS PubMed.
- S. Saito, Anal. Sci., 2021, 37, 17–26 CrossRef CAS PubMed.
- S.-C. Tsai, L.-Y. Hung and G.-B. Lee, Biomicrofluidics, 2017, 11(3), 034122 CrossRef PubMed.
- Q. Wang, W. Liu, Y. Xing, X. Yang, K. Wang, R. Jiang, P. Wang and Q. Zhao, Anal. Chem., 2014, 86(13), 6572–6579 CrossRef CAS PubMed.
- R. Su, Z. Li, C. Yang, Y. Li, J. Wang and C. Sun, Spectrochim. Acta, Part A, 2024, 320, 124643 Search PubMed.
- Z. Di, J. Zhao, H. Chu, W. Xue, Y. Zhao and L. Li, Adv. Mater., 2019, 31(33), 1901885 CrossRef PubMed.
- A. Marton Menendez and D. J. Nesbitt, J. Phys. Chem. B, 2022, 126(1), 69–79 CrossRef CAS PubMed.
- A. Espah Borujeni, D. M. Mishler, J. Wang, W. Huso and H. M. Salis, Nucleic Acids Res., 2016, 44(1), 1–13 CrossRef PubMed.
-
L. Bao and Y. Xiao, Exploring the Binding Process of Cognate Ligand to Add Adenine Riboswitch Aptamer by Using Explicit Solvent Molecular Dynamics (MD) Simulation, in RNA Structure and Dynamics, ed. Y.-X. Wang, J. R. Stagno and J. Ding, Methods in Molecular Biology, Humana, New York, 1st edn, 2023, pp. 103–122 Search PubMed.
- L. M. Hertz, E. N. White, K. Kuznedelov, L. Cheng, A. M. Yu, R. Kakkaramadam, K. Severinov, A. Chen and J. B. Lucks, Nucleic Acids Res., 2024, 52(8), 4466–4482 CrossRef CAS PubMed.
- Y. Tian, S. Zhang, L. Wang and S. Liu, ChemBioChem, 2018, 19(7), 716–722 CrossRef CAS PubMed.
- V. Esposito, A. Russo, V. Vellecco, M. Bucci, G. Russo, L. Mayol, A. Virgilio and A. Galeone, Biochim. Biophys. Acta, Gen. Subj., 2018, 1862(12), 2645–2650 CrossRef CAS PubMed.
- Y. Takeuchi, M. Endo, Y. Suzuki, K. Hidaka, G. Durand, E. Dausse, J.-J. Toulmé and H. Sugiyama, Biomater. Sci., 2016, 4, 130–135 RSC.
- A. Gupta and M. Bansal, Phys. Chem. Chem. Phys., 2016, 18, 28767–28780 RSC.
- L. V. Croft, M. Fisher, T. K. Barbhuiya, S. El-Kamand, S. Beard, A. Rajapakse, R. Gamsjaeger, L. Cubeddu, E. Bolderson, K. O'Byrne, D. Richard and N. S. Gandhi, Nucleic Acid Ther., 2024, 34(3), 143–155 CrossRef CAS PubMed.
- R. Pal, I. Deb, J. Sarzynska and A. Lahiri, J. Biomol. Struct. Dyn., 2023, 41(6), 2221–2230 CrossRef CAS PubMed.
- H. Komine, S. Mori, K. Morihiro, K. Ishida, T. Okuda, Y. Kasahara, H. Aoyama, T. Yamaguchi and S. Obika, Molecules, 2020, 25(7), 1732 CrossRef CAS PubMed.
- B. Niu, Y. Wu, M. Zhou, R. Lin, P. Ge, X. Chen, H. Zhou, X. Zhang and J. Xie, Cancer Lett., 2023, 579, 216461 CrossRef CAS PubMed.
- Y. Kazemi, S. Dehghani, F. Soltani, K. Abnous, M. Alibolandi, S. M. Taghdisi and M. Ramezani, Nanomedicine, 2022, 45, 102588 CrossRef CAS PubMed.
- J. Rihon, C.-A. Mattelaer, R. W. Montalvão, M. Froeyen, V. B. Pinheiro and E. Lescrinier, Nucleic Acids Res., 2024, 52(6), 2836–2847 CrossRef CAS PubMed.
- C. Riccardi, E. Napolitano, D. Musumeci and D. Montesarchio, Molecules, 2020, 25(22), 5227 CrossRef CAS PubMed.
- L. Yao, L. Wang, S. Liu, H. Qu, Y. Mao, Y. Li and L. Zheng, Chem. Sci., 2024, 15, 13011–13020 RSC.
- C.-H. Wang, C.-P. Chang and G.-B. Lee, Biosens. Bioelectron., 2016, 86, 247–254 CrossRef CAS PubMed.
- I. de F. Souza, J. P. de J. Vieira, E. D. Bonifácio, B. A. de Avelar Freitas and L. A. G. Torres, Tissue Eng., Part B, 2024 DOI:10.1089/ten.teb.2024.0088.
- C. Tian, S. Zheng, X. Liu and K.-I. Kamei, J. Nanobiotechnol., 2022, 20, 338 CrossRef PubMed.
- J.-L. Chang, C.-J. Huang, Y.-C. Tsai, N.-J. Chiang, Y.-S. Huang, S.-C. Hung, Y.-S. Shan and G.-B. Lee, Lab Chip, 2024, 24, 375–382 RSC.
- P. Gopinathan, N. J. Chiang, A. Bandaru, A. Sinha, W. Y. Huang, S. C. Hung, Y. S. Shan and G. B. Lee, Adv. Healthcare Mater., 2020, 9(10), 1901875 CrossRef CAS PubMed.
- C. Wang, J. Qiu, M. Liu, Y. Wang, Y. Yu, H. Liu, Y. Zhang and L. Han, Adv. Sci., 2024, 11(28), 240163 Search PubMed.
- W.-J. Soong, C.-H. Wang, C. Chen and G.-B. Lee, Lab Chip, 2024, 24, 1965–1976 RSC.
- V. Bonnet, E. Maikranz, M. Madec, N. Vertti-Quintero, C. Cuche, M. Mastrogiovanni, A. Alcover, V. Di Bartolo and C. N. Baroud, Proc. Natl. Acad. Sci. U. S. A., 2024, 121(11), e2316500121 CrossRef PubMed.
- P. Xu, J. Chi, X. Wang, M. Zhu, K. Chen, Q. Fan, F. Ye and C. Shao, Lab Chip, 2024, 24, 3470–3479 RSC.
- D. Sun, Y. Ma, M. Wu, Z. Chen, L. Zhang and J. Lu, J. Pharm. Anal., 2023, 13(4), 340–354 CrossRef PubMed.
- D. Kalinowska, I. Grabowska-Jadach, M. Liwinska, M. Drozd, M. Pietrzak, A. Dybko and Z. Brzozka, Biosens. Bioelectron., 2019, 126, 214–221 CrossRef CAS PubMed.
- A. M. Rodríguez-Ces, Ó. Rapado-González, Á. Salgado-Barreira, M. A. Santos, C. Aroso, A. S. Vinhas, R. López-López and M. M. Suárez-Cunqueiro, Diagnostics, 2024, 14(14), 1465 CrossRef PubMed.
- C.-H. Wang, Y.-S. Shao, K.-F. Hsu and G.-B. Lee, Chem. Eng. J., 2024, 495, 153478 CrossRef CAS.
- M. Kohlberger and G. Gadermaier, Biotechnol. Appl. Biochem., 2022, 69(5), 1771–1792 CrossRef CAS PubMed.
- M. Berezovski, M. Musheev, A. Drabovich and S. N. Krylov, J. Am. Chem. Soc., 2006, 128(5), 1410–1411 CrossRef CAS PubMed.
- J. Tok, J. Lai, T. Leung and S. F. Y. Li, Electrophoresis, 2010, 31(12), 2055–2062 CrossRef CAS PubMed.
- M. V. Berezovski, M. U. Musheev, A. P. Drabovich, J. V. Jitkova and S. N. Krylov, Nat. Protoc., 2006, 1, 1359–1369 CrossRef CAS PubMed.
- W. Pan, P. Xin and G. A. Clawson, BioTechniques, 2008, 44, 351–360 CrossRef CAS PubMed.
- W. Pan and G. A. Clawson, Molecules, 2009, 14(4), 1353–1369 CrossRef CAS PubMed.
- Z. Xi, R. Huang, Y. Deng and N. He, J. Biomed. Nanotechnol., 2014, 10(10), 3043–3062 CrossRef CAS PubMed.
- S. D. Mendonsa and M. T. Bowser, Anal. Chem., 2004, 76(18), 5387–5392 CrossRef CAS PubMed.
- R. K. Mosing, S. D. Mendonsa and M. T. Bowser, Anal. Chem., 2005, 77(19), 6107–6112 CrossRef CAS PubMed.
- G. T. Rozenblum, V. G. Lopez, A. D. Vitullo and M. Radrizzani, Expert Opin. Drug Discovery, 2016, 11(2), 127–135 CrossRef CAS PubMed.
- W. He, M. A. Elizondo-Riojas, X. Li, G. L. R. Lokesh, A. Somasunderam, V. Thiviyanathan, D. E. Volk, R. H. Durland, J. Englehardt, C. N. Cavasotto and D. G. Gorenstein, Biochemistry, 2012, 51(42), 8321–8323 CrossRef CAS PubMed.
- S. Lisi, E. Fiore, S. Scarano, E. Pascale, Y. Boehman, F. Ducongé, S. Chierici, M. Minunni, E. Peyrin and C. Ravelet, Anal. Chim. Acta, 2018, 1038, 173–181 CrossRef CAS PubMed.
- G. Yang, C. Zhu, L. Zhao, L. Li, Y. Huang, Y. Zhang and F. Qu, Chin. Chem. Lett., 2021, 32(1), 218–220 CrossRef CAS.
- H. R. Kim, M. Y. Song and B. C. Kim, Anal. Biochem., 2020, 591, 113542 CrossRef CAS PubMed.
- M. Liu, L. Geng, F. Zhang, S. Dou, F. Li, Z. Liu, Y. Guo and X. Sun, J. Agric. Food Chem., 2022, 70(50), 15990–15998 CrossRef CAS PubMed.
- I. H. Jeong, H. K. Kim, H. R. Kim, J. Kim and B. C. Kim, Anal. Bioanal. Chem., 2022, 414, 7763–7771 CrossRef CAS PubMed.
- M. J. N. Tapp, J. M. Slocik, P. B. Dennis, R. R. Naik and V. T. Milam, ACS Comb. Sci., 2018, 20(10), 585–593 CrossRef CAS PubMed.
- R. Sullivan, M. C. Adams, R. R. Naik and V. T. Milam, Molecules, 2019, 24(8), 1572 CrossRef CAS PubMed.
- A. Kushwaha, Y. Takamura, K. Nishigaki and M. Biyani, Sci. Rep., 2019, 9, 6642 CrossRef PubMed.
- D. Wu, T. Feagin, P. Mage, A. Rangel, L. Wan, D. Kong, A. Li, J. Coller, M. Eisenstein and H. Soh, Anal. Chem., 2023, 95(5), 2645–2652 CrossRef CAS PubMed.
- M. Biyani, K. Yasuda, Y. Isogai, Y. Okamoto, W. Weilin, N. Kodera, H. Flechsig, T. Sakaki, M. Nakajima and M. Biyani, ACS Appl. Mater. Interfaces, 2022, 14(16), 18064–18078 CrossRef CAS PubMed.
- A. Parashar, V. Bhushan, N. C. Mahanandia, S. Kumar and A. K. Mohanty, J. Dairy Sci., 2022, 105(7), 5545–5560 CrossRef CAS PubMed.
- A. Lozoya-Colinas, Y. Yu and J. C. Chaput, J. Am. Chem. Soc., 2023, 145(47), 25789–25796 CrossRef CAS PubMed.
- A. M. Yoshikawa, A. E. Rangel, L. Zheng, L. Wan, L. A. Hein, A. A. Hariri, M. Eisenstein and H. T. Soh, Nat. Commun., 2023, 14, 2336 CrossRef CAS PubMed.
- H. Sun, W. Tan and Y. Zu, Analyst, 2016, 141, 403–415 RSC.
- V. Thiviyanathan and D. G. Gorenstein, Proteomics: Clin. Appl., 2012, 6(11–12), 563–573 CAS.
- E. Torres-Chavolla and E. C. Alocilja, Biosens. Bioelectron., 2009, 24(11), 3175–3182 CrossRef CAS PubMed.
- O. Alkhamis, J. Canoura, H. Yu, Y. Liu and Y. Xiao, TrAC, Trends Anal. Chem., 2019, 121, 115699 CrossRef CAS PubMed.
- A. D. Gelinas, D. R. Davies and N. Janjic, Curr. Opin. Struct. Biol., 2016, 36, 122–132 CrossRef CAS PubMed.
- T. Hermann and D. J. Patel, Science, 2000, 287(5454), 820–825 CrossRef CAS PubMed.
- Y. Nomura, S. Sugiyama, T. Sakamoto, S. Miyakawa, H. Adachi, K. Takano, S. Murakami, T. Inoue, Y. Mori, Y. Nakamura and H. Matsumura, Nucleic Acids Res., 2010, 38(21), 7822–7829 CrossRef CAS PubMed.
- X. Hao, P. Yeh, Y. Qin, Y. Jiang, Z. Qiu, S. Li, T. Le and X. Cao, Anal. Chim. Acta, 2019, 1056, 96–107 CrossRef CAS PubMed.
- V. Parkula, M. Berto, C. Diacci, B. Patrahau, M. Di Lauro, A. Kovtun, A. Liscio, M. Sensi, P. Samorì, P. Greco, C. A. Bortolotti and F. Biscarini, Anal. Chem., 2020, 92(13), 9330–9337 CrossRef CAS PubMed.
- X. Wang, X. He, Z. He, L. Hou, C. Ge, L. Wang, S. Li and Y. Xu, Biosens. Bioelectron., 2022, 204, 114057 CrossRef CAS PubMed.
- F. Pfeiffer and G. Mayer, Front. Chem., 2016, 4, 25 Search PubMed.
- Z. Li, M. A. Mohamed, A. M. Vinu Mohan, Z. Zhu, V. Sharma, G. K. Mishra and R. K. Mishra, Sensors, 2019, 19(24), 5435 CrossRef CAS PubMed.
- G. K. Mishra, V. Sharma and R. K. Mishra, Biosensors, 2018, 8(2), 28 CrossRef PubMed.
- X. Chen, J. Wang, H. Y. Shen, X. Su, Y. Cao, T. Li and N. Gan, ACS Sens., 2019, 4(8), 2131–2139 CrossRef CAS PubMed.
- M. Park and T. S. Seo, Biosens. Bioelectron., 2019, 126, 405–411 CrossRef CAS PubMed.
- W.-H. Huang, V.-P. Mai, R.-Y. Wu, K.-L. Yeh and R.-J. Yang, Micromachines, 2011, 12(11), 1283 CrossRef PubMed.
- S. Qian, Y. Han, F. Xu, D. Feng, X. Yang, X. Wu, L. Hao and M. Yuan, Talanta, 2022, 247, 123548 CrossRef CAS PubMed.
- K. Yoshida, T. Hayashi, M. Takinoue and H. Onoe, Sci. Rep., 2022, 12, 9692 CrossRef CAS PubMed.
- A. M. Ulloa-Gomez, A. Lucas, A. Koneru, A. Barui and L. Stanciu, Biosens. Bioelectron., 2023, 221, 114419 CrossRef CAS PubMed.
- C. C. Tseng, S. Y. Lu, S. J. Chen, J. M. Wang, L. M. Fu and Y. H. Wu, Anal. Chim. Acta, 2022, 1203, 339722 CrossRef CAS PubMed.
- F. Chen, J. Wang, L. Du, X. Zhang, F. Zhang, W. Chen, W. Cai, C. Wu and P. Wang, Biosens. Bioelectron., 2019, 130, 382–388 CrossRef CAS PubMed.
- B. Chen, D. Wang, S. Wei and J. Wang, Biosens. Bioelectron., 2024, 260, 116434 CrossRef CAS PubMed.
- Z. Ji, D. Wang and J. Wang, Anal. Methods, 2024, 16, 4160–4167 RSC.
- A. M. Ulloa-Gomez, J. F. Waimin, Y. C. Yu, A. Lucas and L. A. Stanciu, Microchim. Acta, 2024, 191, 194 CrossRef CAS PubMed.
- X. Chen, Y. Liu, X. Fang, Z. Li, H. Pu, J. Chang, J. Chen and S. Mao, Biosens. Bioelectron., 2019, 126, 664–671 CrossRef CAS PubMed.
- F. Costantini, N. Lovecchio, A. Ruggi, C. Manetti, A. Nascetti, M. Reverberi, G. de Cesare and D. Caputo, ACS Appl. Bio Mater., 2019, 2(12), 5880–5887 CrossRef CAS PubMed.
- L. He, Z. Shen, Y. Cao, T. Li, D. Wu, Y. Dong and N. Gan, Analyst, 2019, 144, 2755–2764 RSC.
- N. Nekrasov, D. Kireev, A. Emelianov and I. Bobrinetskiy, Toxins, 2019, 11(10), 550 CrossRef CAS PubMed.
- S. Ramalingam, R. Chand, C. B. Singh and A. Singh, Biosens. Bioelectron., 2019, 135, 14–21 CrossRef CAS PubMed.
- L. He, Z. Shen, J. Wang, J. Zeng, W. Wang, H. Wu, Q. Wang and N. Gan, Microchim. Acta, 2020, 187, 176 CrossRef CAS PubMed.
- A. Kasoju, D. Shahdeo, A. A. Khan, N. S. Shrikrishna, S. Mahari, A. M. Alanazi, M. A. Bhat, J. Giri and S. Gandhi, Sci. Rep., 2020, 10, 4627 CrossRef CAS PubMed.
- R. Tian, J. Ji, Y. Zhou, Y. Du, X. Bian, F. Zhu, G. Liu, S. Deng, Y. Wan and J. Yan, Biosens. Bioelectron., 2020, 160, 112218 CrossRef CAS PubMed.
- Z. Khoshbin, K. Abnous, S. M. Taghdisi and A. Verdian, Biosens. Bioelectron., 2021, 191, 113457 CrossRef CAS PubMed.
- S. Ramalingam, A. Elsayed and A. Singh, Food Chem., 2023, 403, 134302 CrossRef CAS PubMed.
- L. Kékedy-Nagy, J. M. Perry, S. R. Little, O. Y. Llorens and S. C. C. Shih, Biosens. Bioelectron., 2023, 222, 114998 CrossRef PubMed.
- P. Wang, L. Ding, Y. Zhang and X. Jiang, Biosensors, 2023, 13(12), 1027 CrossRef CAS PubMed.
- X. Zhou, Z. Sun, X. Su, K. Zheng, X. Zou and W. Zhang, Anal. Chem., 2023, 95(3), 1916–1923 CrossRef CAS PubMed.
- W. Ma, L. Liu, Y. Xu, L. Wang, L. Chen, S. Yan, L. Shui, Z. Wang and S. Li, Analyst, 2020, 145, 4204–4211 RSC.
- A. Nascetti, M. Mirasoli, E. Marchegiani, M. Zangheri, F. Costantini, A. Porchetta, L. Iannascoli, N. Lovecchio, D. Caputo, G. de Cesare, S. Pirrotta and A. Roda, Biosens. Bioelectron., 2019, 123, 195–203 CrossRef CAS PubMed.
- J. Niu, X. Hu, W. Ouyang, Y. Chen, S. Liu, J. Han and L. Liu, Anal. Chem., 2019, 91(3), 2360–2367 CrossRef CAS PubMed.
- M. Nandimandalam, F. Costantini, N. Lovecchio, L. Iannascoli, A. Nascetti, G. de Cesare, D. Caputo and C. Manetti, Materials, 2021, 14(23), 7210 CrossRef CAS PubMed.
- X. Weng, Z. Fu, C. Zhang, W. Jiang and H. Jiang, Anal. Chem., 2022, 94(8), 3526–3534 CrossRef CAS PubMed.
- M. Afzal, J. Park, J. S. Jeon, M. Akmal, T.-S. Yoon and H. J. Sung, Anal. Chem., 2021, 93(23), 8309–8317 CrossRef CAS PubMed.
- N. Ardoino, L. Lunelli, G. Pucker, L. Vanzetti, R. Favaretto, L. Pasquardini, C. Pederzolli, C. Guardiani and C. Potrich, Sensors, 2024, 24(10), 3107 CrossRef CAS PubMed.
- S. Arshavsky-Graham, A. Enders, S. Ackerman, J. Bahnemann and E. Segal, Microchim. Acta, 2021, 188, 67 CrossRef CAS PubMed.
- F. R. Cavallo, K. B. Mirza, S. de Mateo, K. Nikolic, J. Rodriguez-Manzano and C. Toumazou, ACS Sens., 2021, 6(3), 709–715 CrossRef CAS PubMed.
- R. Gandotra, F. C. Kuo, M. S. Lee and G. B. Lee, Anal. Chim. Acta, 2023, 1281, 341879 CrossRef CAS PubMed.
- C. Ge, J. Feng, J. Zhang, K. Hu, D. Wang, L. Zha, X. Hu and R. Li, Talanta, 2022, 236, 122847 CrossRef CAS PubMed.
- H. Jiang, Q. Guo, C. Zhang, Z. Sun and X. Weng, Food Chem., 2021, 365, 130511 CrossRef CAS PubMed.
- N. I. Khan, M. Mousazadehkasin, S. Ghosh, J. G. Tsavalas and E. Song, Analyst, 2020, 145, 4494–4503 RSC.
- S. H. Lee, B. Cha, J. Ko, M. Afzal and J. Park, Biomicrofluidics, 2023, 17, 024105 CrossRef CAS PubMed.
- P. R. Li, S. K. Boilla, C. H. Wang, P. C. Lin, C. N. Kuo, T. H. Tsai and G. B. Lee, Biosens. Bioelectron., 2024, 249, 115931 CrossRef CAS PubMed.
- R. S. Massey, E. M. McConnell, D. Chan, M. R. Holahan, M. C. DeRosa and R. Prakash, ACS Sens., 2023, 8(8), 3116–3126 CrossRef CAS PubMed.
- T. T. Q. Nguyen, E. M. Lee, T. T. T. Dang, E. R. Kim, Y. Ko and M. B. Gu, Biosens. Bioelectron., 2024, 251, 116097 CrossRef CAS PubMed.
- A. M. Ogunmolasuyi, R. Fogel, H. Hoppe, D. Goldring and J. Limson, Malar. J., 2022, 21, 174 CrossRef CAS PubMed.
- M. Pan, X. Han, S. Chen, J. Yang, Y. Wang, H. Li and S. Wang, Talanta, 2024, 267, 125188 CrossRef CAS PubMed.
- S. Ramalingam, A. Elsayed and A. Singh, Microchim. Acta, 2020, 187(12), 645 CrossRef CAS PubMed.
- Y. Su, W. Lai, Y. Liang and C. Zhang, Anal. Chim. Acta, 2022, 1206, 339789 CrossRef CAS PubMed.
- A. M. Ulloa-Gomez, A. Agredo, A. Lucas, S. B. Somvanshi and L. Stanciu, Biosens. Bioelectron., 2023, 222, 114938 CrossRef CAS PubMed.
- J. Xue, L. Zhang, C. Gao, P. Zhu and J. Yu, Biosens. Bioelectron., 2019, 133, 1–7 CrossRef CAS PubMed.
- X. Zhang, X. Wei, C. X. Wu, X. Men, J. Wang, J. J. Bai, X. Y. Sun, Y. Wang, T. Yang, C. T. Lim, M. L. Chen and J. H. Wang, ACS Nano, 2024, 18(8), 6612–6622 CrossRef CAS PubMed.
- A. Sinha, T. Y. Tai, K. H. Li, P. Gopinathan, Y. D. Chung, I. Sarangadharan, H. P. Ma, P. C. Huang, S. C. Shiesh, Y. L. Wang and G. B. Lee, Biosens. Bioelectron., 2019, 129, 155–163 CrossRef CAS PubMed.
- S. S. T. Gamage, T. N. Pahattuge, H. Wijerathne, K. Childers, S. Vaidyanathan, U. S. Athapattu, L. Zhang, Z. Zhao, M. L. Hupert, R. M. Muller, J. Muller-Cohn, J. Dickerson, D. Dufek, B. V. Geisbrecht, H. Pathak, Z. Pessetto, G. N. Gan, J. Choi, S. Park, A. K. Godwin, M. A. Witek and S. A. Soper, Sci. Adv., 2022, 8(39), eabn9665 CrossRef CAS PubMed.
- Y. Huang, Q. Wu, J. Zhang, Y. Zhang, S. Cen, C. Yang and Y. Song, ACS Nano, 2023, 17(21), 21973–21983 CrossRef PubMed.
- N. Saraf, M. Villegas, B. J. Willenberg and S. Seal, ACS Omega, 2019, 4(1), 2234–2240 CrossRef CAS PubMed.
- L. Xu, S. Ramadan, B. G. Rosa, Y. Zhang, T. Yin, E. Torres, O. Shaforost, A. Panagiotopoulos, B. Li, G. Kerherve, D. K. Kim, C. Mattevi, L. R. Jiao, P. K. Petrov and N. Klein, Sens. Diagn., 2022, 1, 719–730 RSC.
- J.-J. Bai, X. Zhang, X. Wei, Y. Wang, C. Du, Z.-J. Wang, M.-L. Chen and J.-H. Wang, Anal. Chem., 2023, 95(4), 2523–2531 CrossRef CAS PubMed.
- Y. Chen, D. Gao, Q. Zhu, B. Chu, J. Peng, J. Wang, L. Liu and Y. Jiang, Analyst, 2023, 148, 2387–2394 RSC.
- R. Chinnappan, Q. Ramadan and M. Zourob, Biosens. Bioelectron., 2023, 220, 114856 CrossRef CAS PubMed.
- R. Chinnappan, Q. Ramadan and M. Zourob, J. Funct. Biomater., 2023, 14(9), 441 CrossRef CAS PubMed.
- L. Zhao, H. Wang, J. Fu, X. Wu, X. Y. Liang, X. Y. Liu, X. Wu, L. L. Cao, Z. Y. Xu and M. Dong, Biosens. Bioelectron., 2022, 214, 114487 CrossRef CAS PubMed.
- L. Zheng, H. Wang, P. Zuo, Y. Liu, H. Xu and B.-C. Ye, Anal. Chem., 2022, 94(21), 7703–7712 CrossRef CAS PubMed.
- Z. Zhou, Y. Chen and X. Qian, Biosensors, 2022, 12(4), 257 CrossRef CAS PubMed.
- X. Dong, J. Chi, L. Zheng, B. Ma, Z. Li, S. Wang, C. Zhao and H. Liu, Lab Chip, 2019, 19, 2897–2904 RSC.
- Y. Lu, B. Lin, W. Liu, J. Zhang, L. Zhu, C. Yang and Y. Song, Small Methods, 2023, 7(9), 2300516 CrossRef CAS PubMed.
- G. N. Abdelrasoul, A. Anwar, S. MacKay, M. Tamura, M. A. Shah, D. P. Khasa, R. R. Montgomery, A. I. Ko and J. Chen, Anal. Chim. Acta, 2020, 1107, 135–144 CrossRef CAS PubMed.
- M. Kavruk, Z. Babaie, G. Kibar, B. Çetin, H. Yeşilkaya, Y. Amrani, A. D. Dursun and V. C. Özalp, Microchim. Acta, 2024, 191, 285 CrossRef CAS PubMed.
- W. Liu, C. Zhu, S. Gao, K. Ma, S. Zhang, Q. Du, K. Sui, C. Liu and Z. Chi, Talanta, 2024, 266(Part 2), 125085 CrossRef CAS PubMed.
- Y. Man, M. Ban, A. Li, X. Jin, Y. Du and L. Pan, Food Chem., 2021, 354, 129578 CrossRef CAS PubMed.
- A. Manfredini, E. Malusà and L. Canfora, Appl. Microbiol. Biotechnol., 2023, 107, 6963–6972 CrossRef CAS PubMed.
- Y. Suo, W. Yin, Q. Zhu, W. Wu, W. Cao and Y. Mu, Biosensors, 2022, 12(7), 458 CrossRef CAS PubMed.
- Z. Wang, H. Wang, X. Cheng, J. Geng, L. Wang, Q. Dong, C. Liu, Z. Chi and Z. Chi, Biosens. Bioelectron., 2021, 193, 113551 CrossRef CAS PubMed.
- J. H. Wu, C. H. Wang, Y. D. Ma and G. B. Lee, Lab Chip, 2018, 18, 1633–1640 RSC.
- Z. Zhang, X. Deng, W. Zhang, K. Chen, Y. Su, C. Gao, D. Gong, L. Zhu and J. Cai, Biomicrofluidics, 2024, 18(3), 034104 CrossRef CAS PubMed.
- Z. Zhou, X. Lan, L. Zhu, Y. Zhang, K. Chen, W. Zhang and W. Xu, J. Hazard. Mater., 2023, 445, 130545 CrossRef CAS PubMed.
- F. Zhu, X. Bian, H. Zhang, Y. Wen, Q. Chen, Y. Yan, L. Li, G. Liu and J. Yan, Biosens. Bioelectron., 2021, 176, 112943 CrossRef CAS PubMed.
- Z. Zhu, Z. Lv, L. Wang, H. Tan, Y. Xu, S. Li and L. Chen, Talanta, 2024, 275, 126155 CrossRef CAS PubMed.
- D. Raju, S. Bathini, S. Badilescu, A. Ghosh and M. Packirisamy, Micromachines, 2022, 13(5), 730 CrossRef PubMed.
- N. Shaabani, S. R. Meira, M. Marcet-Palacios and M. Kulka, ACS Pharmacol. Transl. Sci., 2023, 6(3), 387–398 CrossRef CAS PubMed.
- J. C. Contreras-Naranjo, H. J. Wu and V. M. Ugaz, Lab Chip, 2017, 17, 3558–3577 RSC.
- M. J. Alvarez Cubero, J. A. Lorente, I. Robles-Fernandez, A. Rodriguez-Martinez, J. L. Puche and M. J. Serrano, Methods Mol. Biol., 2017, 1634, 283–303 CrossRef CAS PubMed.
- S. Ju, C. Chen, J. Zhang, L. Xu, X. Zhang, Z. Li, Y. Chen, J. Zhou, F. Ji and L. Wang, Biomark. Res., 2022, 10, 58 CrossRef PubMed.
- D. D. Dickey and P. H. Giangrande, Methods, 2016, 97, 94–103 CrossRef CAS PubMed.
- G. Attard and J. S. de Bono, Curr. Opin. Genet. Dev., 2011, 21(1), 50–58 CrossRef CAS PubMed.
- M. M. Ferreira, V. C. Ramani and S. S. Jeffrey, Mol. Oncol., 2016, 10(3), 374–394 CrossRef CAS PubMed.
- Y. Chen, Y. Yang, J. Feng, A. J. Carrier, D. Tyagi, X. Yu, C. Wang, K. D. Oakes and X. Zhang, Acta Biomater., 2022, 152, 210–220 CrossRef CAS PubMed.
- R. Gao, C. Zhan, C. Wu, Y. Lu, B. Cao, J. Huang, F. Wang and L. Yu, Lab Chip, 2021, 21, 3888–3898 RSC.
- A. A. Kajani, L. Rafiee, M. Samandari, M. A. Mehrgardi, B. Zarrin and S. H. Javanmard, RSC Adv., 2022, 12, 32834–32843 RSC.
- M. Li, D. Liu, J. Zhang, X. Zhang and X. Dou, Anticancer Res., 2022, 42(9), 4345–4358 CrossRef CAS PubMed.
- Y. Liu, Z. Lin, Z. Zheng, Y. Zhang and L. Shui, ACS Sens., 2022, 7(2), 666–673 CrossRef CAS PubMed.
- J. Dong, G. Li and L. Xia, Anal. Chem., 2022, 94(48), 16901–16909 CrossRef CAS PubMed.
- S. Khaksari, A. R. Ameri, S. M. Taghdisi, M. Sabet, S. M. J. Ghaani Bami, K. Abnous and S. A. Mousavi Shaegh, Talanta, 2023, 252, 123781 CrossRef CAS PubMed.
- S. Kumamoto, K. Nakatake, S. Fukuyama, K. Yasuda, Y. Kitamura, M. Iwatsuki, H. Baba, T. Ihara, Y. Nakanishi and Y. Nakashima, Biomicrofluidics, 2020, 14(6), 064113 CrossRef CAS PubMed.
- R. Mohammadi, M. Asghari, M. Colombo, Z. Vaezi, D. A. Richards, S. Stavrakis, H. Naderi-Manesh and A. DeMello, Chimia, 2022, 76(No. 7/8), 661–668 CrossRef PubMed.
- N.-V. Nguyen and C.-P. Jen, Micromachines, 2019, 10(3), 195 CrossRef PubMed.
- J. Peng, Y. Liu, R. Su, L. Zeng, Z. Huo, R. Peng, X. Yu, H. Zhang, C. Yang, L. Yang and Z. Zhu, Anal. Chem., 2022, 94(24), 8766–8773 CrossRef CAS PubMed.
- T. C. Su, H. Vu-Dinh, S. H. Lin, L. Do Quang, T. Chu Duc and C. P. Jen, Biomed. Microdevices, 2024, 26, 7 CrossRef CAS PubMed.
- H. Vu-Dinh, L. Do Quang, C. C. Chang, C. N. Nhu, H. T. Thanh, T. T. Bui, T. C. Duc and C.-P. Jen, Biomed. Microdevices, 2021, 23, 51 CrossRef CAS PubMed.
- H. Vu-Dinh, L. Do Quang, Y. R. Lin and C. P. Jen, Electrophoresis, 2023, 44(11–12), 1002–1015 CrossRef CAS PubMed.
- H. Vu-Dinh, H. Feng and C. P. Jen, Biosensors, 2021, 11(1), 23 CrossRef CAS PubMed.
- C. Wang, Y. Xu, S. Li, Y. Zhou, Q. Qian, Y. Liu and X. Mi, Mater. Today Bio, 2022, 16, 100346 CrossRef CAS PubMed.
- L. Wu, H. Ding, X. Qu, X. Shi, J. Yang, M. Huang, J. Zhang, H. Zhang, J. Song, L. Zhu, Y. Song, Y. Ma and C. Yang, J. Am. Chem. Soc., 2020, 142(10), 4800–4806 CrossRef CAS PubMed.
- Z. Wu, Y. Pan, Z. Wang, P. Ding, T. Gao, Q. Li, M. Hu, W. Zhu and R. Pei, J. Mater. Chem. B, 2021, 9, 2212–2220 RSC.
- B. Zhang, C. Wang, Y. Du, R. Paxton and X. He, J. Mater. Chem. B, 2021, 9, 7196–7204 RSC.
- X. Zhang, X. Wei, X. Men, C. X. Wu, J. J. Bai, W. T. Li, T. Yang, M. L. Chen and J. H. Wang, ACS Appl. Mater. Interfaces, 2021, 13(36), 43668–43675 CrossRef CAS PubMed.
- R. Siavash Moakhar, R. Mahimkar, A. Khorrami Jahromi, S. S. Mahshid, C. del Real Mata, Y. Lu, F. Vasquez Camargo, B. Dixon, J. Gilleard, A. J Da Silva, M. Ndao and S. Mahshid, ACS Sens., 2023, 8(6), 2149–2158 CrossRef CAS PubMed.
- X. Fan, Y. Yan, L. Zhao, X. Xu, Y. Dong and W. Sun, Front. Cell Dev. Biol., 2023, 11, 1183163 CrossRef PubMed.
- N. I. Khan and E. Song, Micromachines, 2020, 11(2), 220 CrossRef PubMed.
- L. Shakeel, A. Khan and A. Akilimali, Ann. Med. Surg., 2024, 86(5), 2413–2416 Search PubMed.
- M. Kalathingal and Y. M. Rhee, J. Comput. Chem., 2023, 44(11), 1129–1137 CrossRef CAS PubMed.
- J. E. Rosenberg, R. M. Bambury, E. M. Van Allen, H. A. Drabkin, P. N. Lara Jr., A. L. Harzstark, N. Wagle, R. A. Figlin, G. W. Smith, L. A. Garraway, T. Choueiri, F. Erlandsson and D. A. Laber, Invest.
New Drugs, 2014, 32, 178–187 CrossRef CAS PubMed.
- M. Karthikeyan and P. Rathinasabapathi, Cell. Mol. Bioeng., 2024, 17, 229–241 CrossRef CAS PubMed.
- T. Jalali, M. Salehi-Vaziri, M. H. Pouriayevali and S. L. M. Gargari, Sci. Rep., 2021, 11, 12639 CrossRef CAS PubMed.
- A. Pourdadashi, R. Rezaei Adriani and S. L. Mousavi Gargari, J. Biosci. Bioeng., 2022, 134(5), 441–449 CrossRef CAS PubMed.
- M. K. Doherty, C. Shaw, L. Woods and B. C. Weimer, Microorganisms, 2023, 11(7), 1776 CrossRef CAS PubMed.
- O. Uhuo, T. Waryo, M. Oranzie, N. Sanga, Z. Leve, J. January, Z. Tshobeni, K. Pokpas, S. Douman and E. Iwuoha, Bioelectrochemistry, 2024, 158, 108693 CrossRef CAS PubMed.
- D. R. Martin, A. T. Mutombwera, A. M. Madiehe, M. O. Onani, M. Meyer and R. Cloete, J. Biomol. Struct. Dyn., 2024, 1–18, DOI:10.1080/07391102.2024.2302922.
- H. C. Lai, C. H. Wang, T. M. Liou and G. B. Lee, Lab Chip, 2014, 14, 2002–2013 RSC.
- G. Kim, J. Kim, S. M. Kim, T. Kato, J. Yoon, S. Noh, E. Y. Park, C. Park, T. Lee and J.-W. Choi, Sens. Actuators, B, 2022, 352(Part 2), 131060 CrossRef CAS PubMed.
- C. Han, Q. Liu, X. Luo, J. Zhao, Z. Zhang, J. He, F. Ge, W. Ding, Z. Luo, C. Jia and L. Zhang, Biosens. Bioelectron., 2024, 257, 116313 CrossRef CAS PubMed.
- M. Kołodziej, J. Joniec, M. Bartoszcze, R. Gryko, J. Kocik and J. Knap, Ann. Agric. Environ. Med., 2013, 20(1), 1–7 Search PubMed.
- G. Park, H. Park, S.-C. Park, M. Jang, J. Yoon, J.-H. Ahn and T. Lee, Nanomaterials, 2023, 13(2), 361 CrossRef CAS PubMed.
- Y. L. Balachandran, X. Li and X. Jiang, Nano Lett., 2021, 21(3), 1335–1344 CrossRef CAS PubMed.
- H. Rouco, P. García-García, C. Évora, P. Díaz-Rodríguez and A. Delgado, Int. J. Pharm., 2022, 624, 121973 CrossRef CAS PubMed.
- H. Shan, X. Sun, X. Liu, Q. Sun, Y. He, Z. Chen, Q. Lin, Z. Jiang, X. Chen, Z. Chen and S. Zhao, Small, 2023, 19(7), 2205498 CrossRef CAS PubMed.
- C.-M. Xu, M. Tang, J. Feng, H.-F. Xia, L.-L. Wu, D.-W. Pang, G. Chen and Z.-L. Zhang, Lab Chip, 2020, 20, 1418–1425 RSC.
- K. Yi, Y. Rong, L. Huang, X. Tang, Q. Zhang, W. Wang, J. Wu and F. Wang, ACS Sens., 2021, 6(4), 1418–1429 CrossRef CAS PubMed.
- S. M. Shea, K. A. Thomas, R. M. G. Rassam, E. P. Mihalko, C. Daniel, B. A. Sullenger, P. C. Spinella and S. M. Nimjee, Pharmaceuticals, 2022, 15(12), 1450 CrossRef CAS PubMed.
- K. Wen, W. Dai, X. Meng, Q. Lin, J. Wei, L. Tong, S. K. Taylor, S. A. Rudchenko, M. N. Stojanovic, G. Kalantarov and I. Trakht, Biosens. Bioelectron., 2024, 246, 115842 CrossRef CAS PubMed.
- T. N. H. Nguyen, L. F. Horowitz, T. Krilov, E. Lockhart, H. L. Kenerson, T. S. Gujral, R. S. Yeung, N. Arroyo-Currás and A. Folch, Sci. Adv., 2024, 10(36), eadn5875 CrossRef CAS PubMed.
- Y. Zhu, C. Zou, J. Zhang, W. Jiang, F. Guan, K. Tang, S. Li, G. Li, J. Wang and Z. Ke, ACS Appl. Mater. Interfaces, 2020, 12(5), 5671–5679 CrossRef CAS PubMed.
|
This journal is © The Royal Society of Chemistry 2025 |
Click here to see how this site uses Cookies. View our privacy policy here.