DOI:
10.1039/D4LC01011F
(Tutorial Review)
Lab Chip, 2025,
25, 1384-1403
Advances of dual-organ and multi-organ systems for gut, lung, skin and liver models in absorption and metabolism studies
Received
29th November 2024
, Accepted 7th February 2025
First published on 13th February 2025
Abstract
Drug development is a costly and timely process with high risks of failure during clinical trials. Although in vitro tissue models have significantly advanced over the years, thus fostering a transition from animal-derived models towards human-derived models, failure rates still remain high. Current cell-based assays are still not able to provide an accurate prediction of the clinical success or failure of a drug candidate. To overcome the limitations of current methods, a variety of microfluidic systems have been developed as powerful tools that are capable of mimicking (micro)physiological conditions more closely by integrating physiological fluid flow conditions, mechanobiological cues and concentration gradients, to name only a few. One major advantage of these biochip-based tissue cultures, however, is their ability to seamlessly connect different organ models, thereby allowing the study of organ-crosstalk and metabolic byproduct effects. This is especially important when assessing absorption, distribution, metabolism, and excretion (ADME) processes of drug candidates, where an interplay between various organs is a prerequisite. In the current review, a number of in vitro models as well as microfluidic dual- and multi-organ systems are summarized with a focus on absorption (skin, lung, gut) and metabolism (liver). Additionally, the advantage of multi-organ chips in identifying a drug's on and off-target toxicity is discussed. Finally, the potential high-throughput implementation and modular chip design of multi-organ-on-a-chip systems within the pharmaceutical industry is highlighted, outlining the necessity of reducing handling complexity.
1 Introduction
In drug development, nine out of ten drugs still fail during clinical trials, while up to 15 years pass for the successful candidate to enter the market.1 For anticancer drugs the success rate was even less with 3.4% in the study time of 2000 to 2015.2 Overall, the most abundant reasons for failure were reported to be a lack of efficacy (52%) and safety (24%) for the reporting period 2013–2015.3 Especially failure rates in the more expensive clinical phases II and III dramatically increase research and development costs, thus posing a great financial burden for companies.4 To minimize the risk of late-stage failure, it is indispensable to increase the predictive validity already in preclinical studies since animal models or 2D cell culture models often fail to accurately predict drug metabolism and the metabolites' toxicity on the human body. Consequently, advanced human cell-based in vitro models, such as organ-on-a-chip systems, have been developed to recapitulate key physiological functions and thereby provide better prediction.5 By establishing multi-organ chips, either by connecting single organ modules or by combining several organ models on a single chip, inter-organ communication can be resembled, thereby facilitating a better replication of physiological processes in the human body.6 Additionally, the simulation of a circulating stream is key in studying the effects of drug metabolites on other tissues, thereby identifying possible secondary drug toxicity early on that reduces costs and attrition rates during clinical trials.7,8 The Food and Drug Administration (FDA) recently paved the way for the use of these microphysiological systems (MPS) in drug safety and effectiveness testing as an alternative to animal testing before entering clinical trials, underlining the potential for broad applicability.9
Besides the need for better predictive models, the number of drug candidates has risen with the introduction of artificial intelligence in pharmaceutical research and development.10 As a result, the demand for pharmacokinetic studies with reliable and physiological-relevant in vitro models to investigate absorption, distribution, metabolism, and excretion (ADME) of drug candidates has significantly increased in recent years.11 Additionally, nanoparticles as advanced drug delivery systems have also been intensively studied for pharmaceutical applications, however, their behavior within the human body is hardly understood.12 Besides their application in drug delivery, their widespread usage in various industrial fields, including food additives and cosmetics, further highlights the need for reliable multi-organ models to study their ADME effects on the human body.13 As a potential technological solution that can also cope with the increasing number of drug candidates, miniaturized human-derived multi-organ platforms are capable of emulating physiologically-relevant metabolism.14 However, handling complexity needs to be reduced and/or operation automated to enable high-throughput screening with these platforms.
In attempt to summarize recent dual- and multi-organ-on-a-chip advances and highlight strategies to improve drug development, this review describes in detail the three main absorption organs (intestine, lung, and skin) involved in different administration routes (oral, pulmonary, and transdermal) and the metabolism organ (liver) as well as their already established in vitro models. Since the liver is the main metabolizing organ, available biochip systems connecting a liver model to various absorption organ models are compiled, and their ability to study a drug's toxicity on target and non-target organs is critically reviewed and discussed. Since the application of microfluidic multi-organ systems in the pharmaceutical industry is still in its infancy, high-throughput organ-on-a-chip systems that offer reduced handling complexity are highlighted and current unmet needs for a transition from the academic to the industrial sector are discussed.
2 Relevant organs in drug absorption and metabolism and their in vitro models
Before elaborating the advances of in vitro models, the following chapter discusses the organs' structural architecture and primary functions. It highlights organ-specific cell types and biophysical properties, such as characteristic flow rate or shear stress, that define the microenvironment. Understanding these key properties is essential for an accurate replications of organ functions in in vitro models.
2.1 Organ architecture
Intestine.
The intestine is an essential organ for drug absorption, requiring drugs to pass through a villi-shaped barrier formed by epithelial cells with microvilli on the apical cell surface to increase the absorption area. This specialized cell barrier comprises five different cell types (Fig. 1A) – enterocytes (drug metabolism via cytochrome P450 enzymes and transport regulation),15,16 goblet cells (mucus production),17 enteroendocrine cells (hormone production),18 Paneth cells (antimicrobial peptide secretion),15 and stem cells (regeneration).17 These five cell types are crucial for maintaining an intact 3D barrier that drugs have to overcome. In order to sustain this fragile system, it is exposed to oxygen levels between 2–8% and shear forces from 0.002–0.08 dyne cm−2.17,19,20
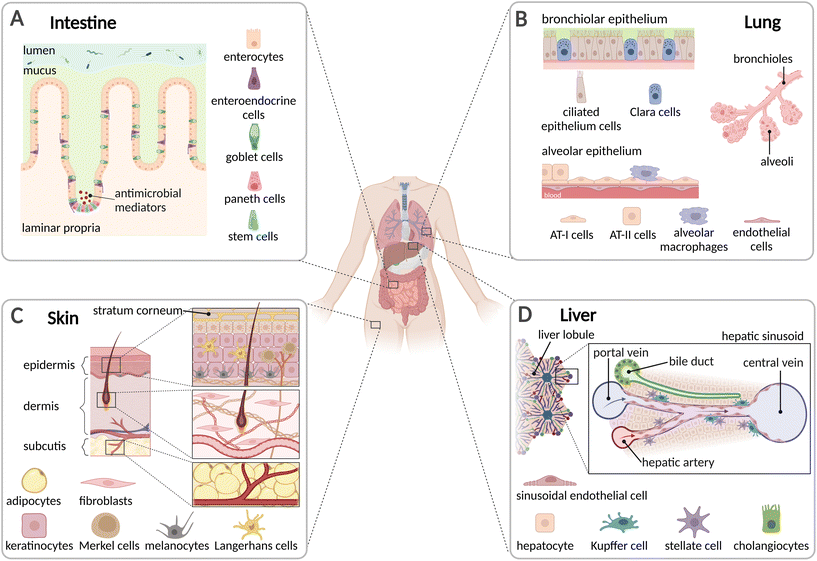 |
| Fig. 1 The architecture and main cell types in drug absorption organs such as the intestine (A), lung (B), and skin (C), as well as the drug metabolizing organ, the liver (D). | |
Lung.
The lower respiratory tract, including the bronchial and alveolar epithelium (Fig. 1B), are critical for drug delivery via inhalation. Generally, the lung has a very low metabolizing environment, enhancing plasma drug bioavailability.21 Like the gut barrier, the airway epithelium's integrity relies on several cell–cell interactions, such as tight junctions, adherent junctions, and desmosomes.22,23 Additionally, mucus covers the bronchial and the bronchiolar epithelium layer produced by either goblet cells or so-called Clara cells, respectively.24 The air-blood barrier, consisting of alveoli, lacks a mucus layer but has a thin surfactant layer, leading to high gas permeability.25,26 The thin alveolar epithelium comprises type I (AT-I) and type II (AT-II) alveolar epithelial cells, whereby the cuboidal AT-II cells secrete surfactant, which regulates the entrance of substances into the blood.25,27 Shear forces of 0.45 dyne cm−2 and 21% oxygen sustain epithelial function.28
Skin.
The skin, comprising the epidermis, dermis and subcutis, forms a primary barrier, allowing transdermal drug delivery which reduces systemic exposure and premature drug degradation.29,30 The epidermis' main cell types (Fig. 1C) are keratinocytes (barrier formation) followed by Merkel cells (mechanosensitivity), Langerhans cells (immune response), and melanocytes (UV protection).31,32 Its' outmost layer, stratum corneum, built of a lipid matrix with dehydrated and dead keratinocytes is the most prominent absorption barrier for drugs and nanoparticles. The dermis mainly consists of connective tissue (collagen I and III and elastin) and fibroblasts secreting extracellular matrix (ECM) components.32 Drugs enter the blood stream through the endothelial barrier of the blood vessels pervading the dermis.33 Skin appendages such as glands, hair follicles, and nerves enable alternative routes for transdermal drug delivery. The transappendageal pathway is particularly favorable for small and rigid nanocarriers.30,33,34
Liver.
The liver plays a key role in drug metabolism and drug-induced liver injury is a common reason for drug withdrawal from the market. Accordingly, more physiological and translational in vitro models are indispensable for assessing a drug's hepatotoxicity.35 The liver houses hepatocytes (drug metabolism via cytochrome P450 enzymes, albumin and urea production),36,37 stellate cells (collagen storage and remodeling),38 Kupffer cells (immune response),39 cholangiocytes (bile duct formation),40 and liver sinusoidal endothelial cells (vascular lining).38,41 Its highly permeable sinusoidal endothelium enables efficient particle exchange, crucial for drug clearance and detoxification.38,42 The liver is generally organized in adjacent lobules (Fig. 1D) and highly vascularized, thereby providing the cells with oxygen rates of 0.9 nmol s−1 10−6 cells.43
2.2 From 2D to 3D: different cell types and culturing techniques in in vitro organ models
In vitro organ models which are of 2D and 3D spatial arrangement, are composed of various cell types, each with different functions leading to unique advantages and disadvantages. While commercial cell lines are easy to culture, reproducible, and long-lasting, they are often derived from cancerous tissues.44 In turn, primary cells represent more closely the in vivo phenotype, but have a limited lifespan, high donor variability, complex culturing conditions and access is limited through national and international ethical guidelines to obtain donor tissue. Although induced pluripotent stem cell (iPSC) technology can be used to differentiate into various human cellular phenotypes, it requires complex and labor-intensive differentiation protocols underlying strict quality control. A key advantage of iPSCs, however, is their potential to be produced from a living human as a source of autologous cells for personalized medical treatments showing higher success rates and fewer side effects in drug screening.45
Next to the choice of representative cell types, the cell culturing methods need to be considered, where often, for historical reasons and functionality, the implementation of 2D culture protocols is used for high-throughput screening applications (Fig. 2).46 To investigate tissue interactions while keeping simplicity and the possibility for high-throughput sampling, Transwell® systems, a two-reservoir setup separated by a porous membrane, are employed. By cultivating cells on the membrane, also considered as 2.5D cultivation, barrier formation, function and permeability can be investigated.47,48 Advancing from 2.5D cell culture, scaffolds derived from biological as well as non-biological materials have been routinely employed over the years to provide structural support for the cultivation of cells in a 3D spatial arrangement. Hydrogels, consisting of ECM or of synthetic origin, have been commonly used to embed cells in 3D, thereby resembling the host tissue more closely. Advancing from of this manual labor-intense work, bioprinting applications were developed, where cell-laden hydrogels are dispensed in a controlled and automated manner attempting to generate 3D functional human tissues.49 However, these systems have limitations in relation to up-scaling, drug adsorption on scaffolds, complex handling, and batch-to-batch variations of the used embedding matrix.50 The 3D matrices gave rise to a promising alternative, derived from pluripotent stem cells (iPSCs or embryonic stem cells) or primary donor tissues, namely organoids. These cell constructs are self-organized aggregates of a diverse range of differentiated cells and residual stem cells, resembling organ regions in a functional and cytoarchitectural manner. In the case of liver and intestinal organoids, the resemblance is so extensive that they are histologically indistinguishable from native tissue.46 These organ models were capable to demonstrate the toxicity of drugs, that had been FDA-approved but then were retracted from the market due to complications in patients, while preclinical models including 2D in vitro studies and animal studies failed to do so.51 However, generation and maintenance of organoids are both cost and time-intensive. Their 3D architecture with a not easily accessible lumen hinders barrier permeability assays, and reduced nutrient access of the inner cells often leads to necrotic cores of the aggregates.47 Furthermore, organoids still mainly model just one type of tissue and lack vascular, immunological, and direct connections to other organs, which are the base of the synergistic human body.52
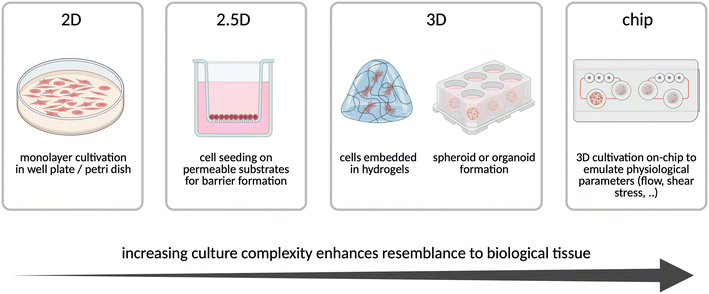 |
| Fig. 2 Overview of cell culture models sorted from left to right based on increasing cultivation complexity but enhanced resemblance of tissue from 2D to microfluidic organ models. | |
Besides the abovementioned advances of these models, they still lack key factors of the cells' in vivo environment. In the human body, several cell types, such as endothelial cells or intestinal epithelial cells, are exposed to fluid flow and associated mechanical stress, while other cells require polarization facilitated via nutrient gradients to form in vivo like tissue structures for example in the cartilage. While these parameters cannot be addressed in conventional static cell culture models, microfluidic systems allow the control of the microenvironment in higher precision. These platforms incorporate one or several organ models connected through microchannels or porous membranes under consideration of mimicking the physiological environment.53 The integration of chemical and electrical sensors furthermore allows non-invasive monitoring of parameters such as pH, oxygen, glucose levels or transepithelial electrical resistance (TEER).46,53,54
Hereafter, the progress from simple to more advanced in vitro organ models is summarized based on selected articles, highlighting drug absorbing and metabolizing organs. The articles were selected because of their relevance for pharmacological and toxicological studies.
Intestine in vitro models.
The most established and investigated in vitro intestinal epithelial cell model is the colon carcinoma cell line Caco-2. These cells are suitable for studying absorption effects since they have been shown to spontaneously differentiate under physiological shear stress into different cell types resembling the cells of the small intestine. They form a 3D villus structure linked with tight junctions and express various properties of the human intestine under dynamic cultivation. In addition, after only five to seven days of aerobic and dynamic cultivation, the Caco-2 cells secrete a mucus layer on the apical surface resembling aspects of the human small intestine barrier, making them suitable to study ADME effects.55 Other commonly used cell lines are HT29 and T84, originating, like the Caco-2 cells, from human colon adenocarcinomas. HT29 cells have been shown to secrete mucins while T84 cells show high similarities to the Caco-2 cell line and are therefore often used interchangeably.56,57 Due to the complex geometry of the intestine, numerous in vitro models implement scaffolds that are shaped to mimic the 3D in vivo structure of the intestinal epithelium. Both biological and non-biological derived scaffolds are applied and shaped in the form of villi. The success of this method is evidenced by cellular heterogeneity from the crypt to the villus tip, which mirrors the cell distribution found in vivo. The use of a collagen-based scaffold enabled Yi et al. to also investigate absorption properties with Caco-2 cells, as the collagen alone did not show any alteration in the diffusion of fluorescent proteins.58 A further advancement in the direction of 3D cell cultures presents the use of organoids. Intestinal organoids show in vivo-like 3D structures and microstructures in the inward direction and a mucus layer was formed in the lumen. As a result, the absorptive apical side of the intestinal epithelium is directed inwards and, therefore, not easily accessible for drug testing and barrier permeability experiments.47,52,59 However, dissociation and replating of the organoids leads to the development of an intestinal monolayer barrier model from patient-derived cells.60 In the small intestine, the cells are exposed to peristaltic fluid flow and this mechanical strain was shown to have an impact on the genetic profile of the cells in vitro, which could be successfully mimicked in fluidic cell culture in microfluidic systems.61 For instance, Kasendra et al. developed a dynamic gut-on-a-chip model with dissociated cells from patient-derived organoids, resulting in a 2.5D epithelium exhibiting in vivo-like characteristics on physiological, phenotypical as well as on gene and protein expression levels.62 A more detailed overview of small intestinal in vitro models can be found in the review of Jung and Kim47 as well as by Fedi et al.,61 who put an additional focus on ADME studies.
Lung in vitro models.
The development of in vitro models of the lung is very challenging due to the varying architecture of the lung e.g. bronchiolar and alveolar regions require different distinct cell types. The majority of lung in vitro models that are currently available are based on immortalized or cancer-derived epithelial cells. A comprehensive discussion of pros and cons of the various cell options can be found in the review provided by Rothen-Rutishauser et al.63 Overall, one of the most used cell lines to model the bronchial and the alveolar epithelium are the Calu-3 and A549 cell lines, respectively.25,64 In order to induce epithelial differentiation, cells are commonly cultivated on a porous membrane with an air–liquid interface (ALI) to mimic the physiological environment, triggering the cells to secrete either mucus or surfactant.25 Culturing Calu-3 cells at an ALI creates a human pulmonary barrier model with a pseudostratified layer, enhanced ciliogenesis, tight junctions and TEER values, suitable for drug permeability studies.26,65 The alveolar cell line A549 is extensively used to study the toxicity of drugs since this cell line is able to produce surfactants, secrete cytokines, and transport substances like AT-II cells in vivo, despite only forming a leaky barrier due to fragmented tight junctions.25,66 While most of these cell lines fail to achieve full epithelial differentiation, primary airway epithelial cells are able to differentiate fully, but culturing these cells remains a challenge, due to their tendency to dedifferentiate.67 However, ALI culture extends the differentiation period for human bronchial epithelial cells68 and results in a transcriptional profile69 and mucociliary cell layer that closely mimics in vivo conditions, making them a promising tool for ADME studies.70
Interestingly, comparative analysis of MucilAir™, a commercially available ALI culture using primary cells, demonstrated that those primary cultures were more resilient to toxins compared to lung cell lines.71 Likewise, EpiAirway™ was observed to be more resilient than A549 cells.72 These commercial models can additionally be utilized in preliminary drug discovery research, and toxicity screening.73 However, although ALI cultures are reproducible in vitro systems, they lack the 3D structure, the flow dynamics, and the mechanical stretch of the human lung. By combining ALI with a dynamic culture condition, including fluid flow and mechanical stretch, lung-on-chip systems overcome these limitations, creating models that better replicate features of the human lung.74 The first lung-on-chip model was developed by Huh et al., stimulating natural breathing by applying a vacuum to the side chambers, which are separated by a thin wall from the cell-containing microchannels and cause mechanical stretching of the PDMS membrane.75 This model was later applied to mimic the drug-induced pulmonary edema found in cancer patients. The study found that while increased barrier permeability emerged with IL-2 exposure alone, it was significantly enhanced when IL-2 exposure was combined with mechanical stretching to simulate physiological breathing movements. Performing a drug study, potential new therapies were identified by demonstrating that angiopoietin-1 and transient receptor potential vanilloid 4 restored barrier function.76 However, these investigations are limited by the use of the cancer cell line NCI-H441. Advancements to those “first-generation” lung-on-chip models have been made by replacing conventional membranes with stretchable biological membranes77 or hydrogels78 or by using more physiological cyclic stretch instead of unidirectional stretching.79 Detailed information about the application of lung models for drug and toxicity screenings can be found in the review of Moreira et al.80 Lastly, iPSCs advance lung-on-a-chip models, however, they have not yet been applied for drug screenings in microfluidic systems.81
Skin in vitro models.
In general, in vitro skin models encompass cells cultured on permeable substrates that allow cultivation at the ALI for epidermis differentiation and drug permeation measurements across the skin barrier. Thereby, the models can be categorized according to the portrayed layers: Reconstructed human epidermis models (RHE) solely constitute the epidermis, full thickness skin models the epidermis and dermis layer, and three-layered models additionally include the subcutis.82–84 Cell types for RHE models are patient-derived primary normal human epidermal keratinocytes and as immortalized alternatives N/TERT or HaCaT cells.85 Cells are cultivated on permeable substrates for three to seven days as a submerged culture before exposing the tissue to the ALI and promote keratinization with stratum corneum formation in calcium enriched medium.84,86–88 Full-thickness models usually comprise primary or immortalized normal human dermal fibroblasts in a collagen type I hydrogel with keratinocytes seeded on top of the gel, followed by the differentiation at the ALI.83 Three-layered models additionally include adipose-derived stem cells or mature adipocytes embedded in a 3D matrix such as fibrin or collagen as a basis for the dermal and epidermal layers.89–91
With a wide range of commercially available models such as EpiSkin™, EpiDerm™, SkinEthic™ and KeraSkin™, RHE are the most used in vitro skin models for drug testing and still the only approved model for skin irritation testing by the Organization for Economic Co-operation and Development (OECD).92,93 Schäfer-Korting et al. conducted a validation study regarding the absorption of nine compounds including the drugs clotrimazole and digoxin with SkinEthic™, EpiSkin™ and EpiDerm™ in comparison to excised human and pig skin showing the feasibility of RHE models for skin penetration studies despite a higher barrier permeability.82 To demonstrate the physiological skin barrier and crosstalk between the skin layers more accurately, open-source or commercial full-thickness models like Phenion® (Henkel) have been applied for permeation studies with hydrophilic and lipophilic compounds such as caffeine and testosterone.88,94 Schmidt et al. developed an advanced three-layered in vitro skin model with isolated mature adipocytes preventing the dermal-mediated contraction of the epidermis by using a filter membrane as an artificial basal layer. The model was able to distinguish between heptanal and 2-propanol as irritating and non-irritating agents with a correlating cytokine expression.90,95
Regarding transdermal drug delivery and absorption, it is critical to incorporate vascularization and perfusion into in vitro skin models as drugs are distributed mainly over the capillaries in the dermis.96,97 For this reason, skin-on-a-chip models have emerged in recent years that allow for dynamic cultivation of in vitro models or ex vivo skin biopsies.98 Ataç et al. demonstrated the positive influence of shear stress by prolonged cultivation times of EpiDermFT™ and ex vivo skin on their microfluidic platform including two circuits with micropumps and wells for incorporating permeable inserts.99 Multiple PDMS-based pumpless microfluidic devices for drug testing have been established that incorporate patient-derived full-thickness skin models and were tested through doxorubicin exposure or antioxidant α-lipoic acid with regard to skin barrier integrity.100,101 The importance for vascularization in drug screening was shown by Salameh et al., developing a full-thickness perfusable skin model that was vascularized with HUVEC cells and additional microvascularization by self-assembly of HUVEC cells in the same layer. This model has shown improved barrier performance compared to commercial RHE models and has been tested for topical and systemic application of caffeine, minoxidil and benzo-a-pyrene.87 iPSC-derived skin models are considered as a promising tool, but due to their laborious culture conditions they have not been applied for drug screening yet.102
Liver in vitro models.
The prerequisites for hepatic drug metabolism and excretion are a well-polarized hepatocyte morphology, as well as the exhibition of distinct cell membrane domains for the xenobiotic uptake.103,104 2D cultivated human primary hepatocytes are a common in vitro model for drug testing, however, limitations include de-differentiation of hepatocytes, reduced CYP450 enzyme activity, low-density cultivation conditions, and incorrect cell differentiation.105 In contrast, the ease of availability and cultivation of cell lines, such as HepGR or HepG2/C3A, lead to a broad applicability in drug testing studies.106 While HepG2/C3A show good hepatic characteristics such as albumin secretion, variability in the expression of CYP450 reduces the potential for comprehensive drug testing. In contrast, HepGR show high expression of drug metabolizing enzymes but need long culture processes and require DMSO for differentiation.50 Primary hepatic spheroids generate essential molecules such as urea and albumin and show long-term viability. Moreover, the cells exhibit a polarized morphology and functional bile ducts can be observed inside the spheroids. The spheroidal co-cultivation of hepatocytes with non-parenchymal cells, including stellate cells, Kupffer cells, and biliary cells, further supports the long-term viability.103,104 However, necrotic core formation within the spheroid because of low oxygen diffusion limits the applicability of spheroids.50 To control gradients and fluid flow, organ-on-a-chips are promising platforms, enabling the emulation of the physiological environment more closely. In addition, the integration of sensors enables time-resolved monitoring, as an example, Yang et al. connected a liver-on-a-chip platform - a perfusion chip with hepatocytes embedded in collagen – with a downstream sensing module for non-invasive measurements of albumin and GST-α by utilizing a label-free photonic crystal-total internal reflection biosensor. Thereby, the changes of albumin and GST-α secretion as a response to doxorubicin exposure could be monitored continuously.107 Wang et al. used a perfusable chip system to generate liver organoids from human iPSCs (hiPSCs), demonstrating a long-term culture (30 days) with cellular heterogeneity. Perfusion was compared to static cultivation, showing enhanced expression of endodermal (FOXA2, SOX17), hepatocyte (ALB), and CYP450 markers, as well as urea and albumin secretion, underlining the generation of characteristic liver functions. The ability for studying drug-induced hepatoxicity was shown by exposing the liver organoids to different concentrations of acetaminophen, resulting in a dose- and time-dependent reduction of viability.108 In another study, the commercially available liver-chip, a membrane-based chip with hepatocytes in the upper channel and liver sinusoidal endothelial, Kupffer, and stellate cells in the lower channel, was tested with 27 hepatotoxic and non-toxic drugs. Compared to 3D spheroid cultivation of hepatocytes, the sensitivity could be improved from 47% to 87% when using the heterocellular liver-chip, while specificity was 100% for both models. This study demonstrated the capabilities of chip models for better predictive drug studies, underlining the potential of reducing time and costs for the pharmaceutical industry.109 Bircsak et al. evaluated high-throughput drug screening with the commercially available OrganoPlate® in the format of a 96-well plate with automated liquid handling, consisting of a parallel 2-channel system with endothelial and differentiated THP-1 (to Kupffer-like cells) in a channel next to hepatocytes from differentiated iPSC. The platform was used for the screening of 159 compounds to investigate hepatotoxicity and dose–response testing of 21 compounds by analysing viability and albumin secretion.110 A comprehensive overview of recent developments in liver-on-a-chip systems is provided by Moradi et al.111
3 Microfluidic dual-and multi-organ studies for drug testing
Some drugs are specifically activated through metabolism in a non-target tissue (e.g. the liver) to be effective when reaching the target organ, or contrastingly, are inactivated by other organs. Consequently, single organ studies are not representative for studying ADME processes and might lead to false conclusions about drug candidates in pharmacological studies.112,113 Therefore, to investigate potential off-target toxicity of drugs and their metabolites, MPS incorporating several organ models have been developed.114 In this chapter, dual-organ systems including liver and an absorption organ will be described and summarized (Table 1), to give an overview of existing models representing absorption and drug metabolism in the liver. After that, the importance and advances of off-target toxicity models are highlighted, based on recent developments utilizing multi-organ chips. Finally, unmet needs are summarized, directing a potential path towards broad applicability of multi-organ MPS in drug development.
Table 1 Overview of dual-organ models comprising of a liver and absorption organ model (gut, skin, lung) for drug studies. MoDC: primary human monocyte-derived dendritic cells; PHH: primary human hepatocytes; PRH: primary rat hepatocytes; KCs: kupffer cells; NPC: non-parenchymal cells; HHSteC: human primary hepatic stellate cells; skin explant: human juvenile prepuce skin explant; nHEK: normal human keratinocytes; hiPSC HEPs: human induced pluripotent stem cell-derived hepatocyte-like cells; HDMEC: human dermal microvascular endothelial cells; NHBE: normal human bronchial epithelial; PMDS: polydimethylsiloxane; PEEK: polyetheretherketone; PMMA: poly(methyl methacrylate); PC: polycarbonate; PEI: polyetherimide; PSU: polysulfone; PFPE: perfluorpolyether; PBT: polybutylene terephthalate
Organ models |
Cell type(s) |
Complexity |
Material |
Modularity |
Drug |
Ref. |
Liver–gut |
Caco-2-BBE, HT29-MTX, MoDC |
2.5D |
PSU |
+ |
Diclofenac, hydrocortisone |
Tsamandouras et al.115 |
PHH, KCs |
3D |
Caco2 |
2.5D |
PDMS |
— |
Phenacetin |
Bricks et al.116 |
HepG2 |
2D |
Caco-2, HepG2, PHH/PRH |
2.5D |
PC, PDMS |
+ |
Paracetamol |
Prot et al.117 |
Caco-2 |
2.5D |
Veroclear polymer |
+ |
— |
Esch et al.118 |
PHH, NPC |
3D |
|
Caco-2 |
2.5D |
PFPE |
— |
Midazolam |
Wang et al.119 |
PHH |
2D |
|
Caco-2, HT29 |
2.5D |
— |
— |
Mycophenolate mofetil/mycophenolic acid |
Milani et al.113 |
PHH |
3D |
|
Caco-2, HepaRG, HUVEC |
2.5D |
PBT |
— |
Irinotecan |
Lucchetti et al.112 |
THP-1 |
2D |
Liver–skin |
Skin explant |
2.5D |
PDMS, PC, glass (HUMIMIC Chip2) |
+ |
Troglitazone |
Wagner et al.120 |
HepaRG, HHSteC |
3D |
Skin explant |
2.5D |
Troglitazone |
Maschmeyer et al.121 |
HepaRG, HHSteC |
3D |
HDMEC |
2D |
Primary nHEK, nHDF |
2.5D |
Terbinafine |
Tavares et al.122 |
HepaRG, HHSteC |
3D |
EpiDerm™ |
2.5D |
Permethrin, hyperforin |
Kühnl et al.123 |
HepaRG, HHSteC |
3D |
PhenionFT™, SkinEthic™ |
2.5D |
4-Amino-2-hydroxytoluene |
Brandmair et al.124 |
HepaRG, HHSteC |
3D |
EpiDerm™ |
2.5D |
Genistein |
Tao et al.125 |
HepaRG, HHSteC |
3D |
nHEK |
3D |
PDMS, glass |
— |
Tretinoin, acetaminophen, camphor |
Lee et al.126 |
hiPSC HEPs |
3D |
Liver–lung |
MucilAir™ |
2.5D |
PDMS, PC, glass (HUMIMICChip3plus) |
+ |
Aflatoxin B1 |
Schimek et al.127 |
HepaRG |
3D |
NHBE |
2.5D |
PEEK |
+ |
Aflatoxin B1 |
Bovard et al.128 |
HepaRG |
3D |
A549 |
2.5D |
PMMA, PC |
+ |
Curcumin |
Miller et al.129 |
HepG2 C3A, MDA-MB-231 |
3D |
HepG2, A549 |
2D |
PDMS |
— |
Irinotecan |
Shinha et al.130 |
PHH, KCs |
3D |
PEI, Kapton® polyimide |
+ |
— |
Coppeta et al.131 |
NHBE |
2.5D |
3.1 Combined absorption and metabolism studies
Liver–intestine.
The GIT is one of the main players in drug absorption, especially when the drug is administered orally.113 The intestine communicates directly with the liver since 70% of the blood comes from the intestine and metabolites are exchanged via venous flow.132 Many microfluidic systems incorporating the intestine and the liver are similar in structure, trying to recapitulate the absorption route in vivo, thereby, the intestinal part is cultured apically on a membrane, and the basolateral part is linked by flow to the hepatic culture.113,116,119 This design approach was also followed by Tsamandouras et al. in an automated device, where fluid flow was generated by peristaltic pumps that supplied the intestinal tissue and, thereafter, the hepatic chamber. The system included two further chambers, offering the possibility to add two more organs of interest. Polysulfone was chosen as the device's material as it shows minimal drug adsorption properties. Thereby, pharmacokinetics of diclofenac and hydrocortisone could be studied.115 Bricks et al. showed with an intestine–liver model that dynamic cultivation of HepG2 C3A upregulates CYP1A activity, resulting in an increased metabolization of phenacetin, a painkiller and fever-reducing drug, that is transported through the intestinal barrier to the liver compartment.133 A platform for the co-culture of a gut and liver model with two circuits (apical and basal) was developed by Prot et al. to study the metabolism of paracetamol and establish a mathematical model for in vitro estimations and in vivo predictions.134 Esch et al. developed a modular pumpless platform for a co-culture of the intestine and liver tissue with a gravity-based unidirectional flow, demonstrating a functional barrier of the intestine, through TEER measurements with integrated electrodes, as well as metabolically active hepatocytes, indicating possible usage for drug screening applications.135 Recently, Wang et al. were able to investigate oral bioavailability and the first-pass effect, which describes the metabolization of an orally administered drug during its first passage through the liver after absorption in the GIT. This effect can lower the systemic availability of the active substance at the site of action, which has a significant impact on the medication's efficacy. Therefore, a gut–liver-on-a-chip device was developed and fabricated from perfluoropolyether as it was shown to have reduced drug absorption properties. The three-channel design enabled drug transport to the liver (primary human hepatocytes) after penetrating the intestinal epithelial layer (Caco-2).119 The prodrug mycophenolate mofetil is metabolized through both the gut and liver. Milani et al. used this mechanism to evaluate their gut–liver organ-on-a-chip device. The design of the Physiomimix® gut–liver-on-a-chip, which is commercially available from CN-Bio, connects the basolateral part of the gut chamber with the liver chamber through controlled media spillover.113 Lucchetti et al. combined their previously developed microbiome-gut-on-chip platform with a liver-on-chip to investigate the gut–liver axis. With this, they delved into the topic of the influence of the intestinal microbiome on drug metabolism which has been brought to focus in recent years.112
Liver–lung.
Similar to the liver–intestine model of Maschmeyer et al.136 described above, Schimek et al. developed a liver–lung model with integrated medium and air exchange to study the effect of inhaled substances. Here, bronchial MucilAir in ALI culture and liver spheroids (HepaRG) were cultured in the commercial Chip3plus membrane-based microfluidic device.127 A comparable method was used by Bovard et al. with normal human bronchial epithelial cells and HepaRG liver spheroids.128 Both studies showed that the presence of HepaRG spheroid culture was able to reduce the toxicity of aflatoxin B1, highlighting the importance of including the metabolic capabilities of liver tissue to fully assess the toxicity profile of inhaled substances. Moreover, Miller et al. developed a lung–liver–breast cancer on chip using A549, HepG2 C3A and MDA-MB-231. The design revealed that curcumin treatment reduced the viability of breast cancer cells compared to the control. Notably, within the microfluidic device, inhalation therapy resulted in a more substantial decrease in MDA-MB-231 cell viability than intravenous therapy.129 Shinha et al. studied a liver–lung cancer chip model to evaluate its effectiveness for pharmacokinetic and pharmacodynamic (PK/PD) models by applying an anticancer prodrug (CPT-11) and investigating the inhibitory effect of simvastatin and ritonavir afterwards. Experimental results from the chip were compared with a PK/PD model, demonstrating its similarity.130 To precisely control and automate the cultivation of a multi-organ platform, Coppeta et al. developed a programmable platform for dynamic perfusion and crosstalk consisting of 62 actuators and 20 micropumps. The platform's feasibility was demonstrated by the co-cultivation of a liver–lung model for 14 days, however, the platform has not yet found application in a drug study.131
Liver–skin.
Skin models are primarily used to evaluate cosmetic ingredients and topically applied sensitizers for their irritant and toxic properties.92,120 Few models have been developed that solely focus on the combination of liver and skin tissues outside of multi-organ chip approaches. However, Wagner et al. created a co-culture system including liver spheroids assembled of HepaRG and human hepatic stellate cells and a skin biopsy maintained on 96-well Transwell® inserts for ALI cultivation. Herein, after exposure to the hepatotoxic drug troglitazone, elevated mRNA and protein expression of CYP450 was determined, indicating the drug's toxicity.137 To recapitulate the physiological environment more closely, primary human epidermal microvascularization cells were cultivated in the microchannels, mimicking vascularization.121 Further improvements resulted in a commercialized two-organ model system, the HUMIMIC Chip2138 (TissUse), applied for several topical and systemic drug studies with in vitro skin models. For instance, the topical and systemic exposure to antifungal drug terbinafine was investigated with a reconstructed full thickness skin model122 and the pharmacokinetic and metabolic activity of anti-lice drug permethrin as well as herbal hyperforin was evaluated for topical application with EpiDerm™.123 Furthermore, recent studies with full-thickness and epidermal models accurately portrayed the metabolization of the hair dye 4-amino-2-hydroxytoluene124 and the effects of different concentrations of genistein as a potential cosmetic ingredient.125 Another cultivation approach was chosen by Lee et al., developing a pumpless chip with six parallel units, each consisting of four channels for the serial arrangement of a skin model and other 3D tissues. Due to the vertical chip arrangement, an epidermis model of human epidermal keratinocytes at the ALI was established in the top channel, followed by a hydrogel-based permeation barrier, a 3D liver model with iPSC hepatocyte-like cells, and a medium channel. The integrity of the epidermis and response to the exfoliating reagent tretinoin was accurately determined and hepatoxicity was investigated by topical application of analgetic acetaminophen and the pharmaceutic and cosmetic ingredient camphor. As a result, dose-dependent liver toxicity could be monitored by decreasing glutathione and increasing reactive oxygen species.126
3.2 Advances through dual- and multi-organ models in drug testing
Off-target metabolism and toxicity studies.
The metabolism of drugs does not rely on a single organ but on the interplay of multiple organs. As an example, vitamin D3 is metabolized into its active form in the liver and kidney, consequently, Theobald et al. developed a chip with two cell compartments, one for liver and the other for kidney cells. The study demonstrated an increased expression of vitamin D-metabolizing enzymes under dynamic on-chip cultivation compared to static controls. Additionally, the extracted culture medium containing vitamin D3 metabolites resulted in the expression of differentiation marker of HL60 cells.139 Moreover, the renal proximal tubule of the kidney is responsible for clearing xenobiotics by tubular secretion or resabsorption, which can lead to drug-induced nephrotoxicity. Huang et al. developed a circulating liver–kidney chip system to study drug-induced toxicity on 3D liver and kidney models, showing higher sensitivity of the liver–kidney chip compared to a liver-only chip.140 Another common side effect is cardiotoxicity of drug candidates, often leading to failure in clinical trials.141 Consequently, Oleaga et al. developed a chip with an integrated cantilever and MEA to study the cardiotoxicity of liver metabolized drugs on iPSC-derived cardiomyocytes by measuring viability, conduction velocity, contractile force, QT-interval, and beat frequency. When exposing the co-culture to cyclophosphamide, a prodrug activated in the liver with germ cell toxicity properties,142 cardiotoxicity was induced, while for terfenadine, cardiotoxicity was reduced, thereby reproducing the drugs' known pharmacological properties.143 This chip was extended to compromise a synthetic skin surrogate to study drug toxicity after topical administration, which is especially important for drugs with side effects on the GIT (Fig. 3A). Four different drugs (diclofenac, ketoconazole, hydrocortisone, and acetaminophen) were tested, demonstrating no significant changes in hepatic and cardiac function compared to systemic administration.144 Yin et al. developed a co-culture chip for the cultivation of hiPSC-derived liver and heart organoids in separated channels with an array configuration, respectively, placed on top of each other with a membrane in between. To study the antidepressant drug clomipramine, the liver organoid was analyzed on day 20 for the expression of the drug-metabolizing CYP450 enzymes (CYP1A2, CYP2C19, CYP2D6, and CYP3A4) to ensure reliable drug testing. Cardiac functions were further reduced in the presence of the liver organoids, demonstrating a cardiotoxic effect of the drug as well as the drug's metabolites.145
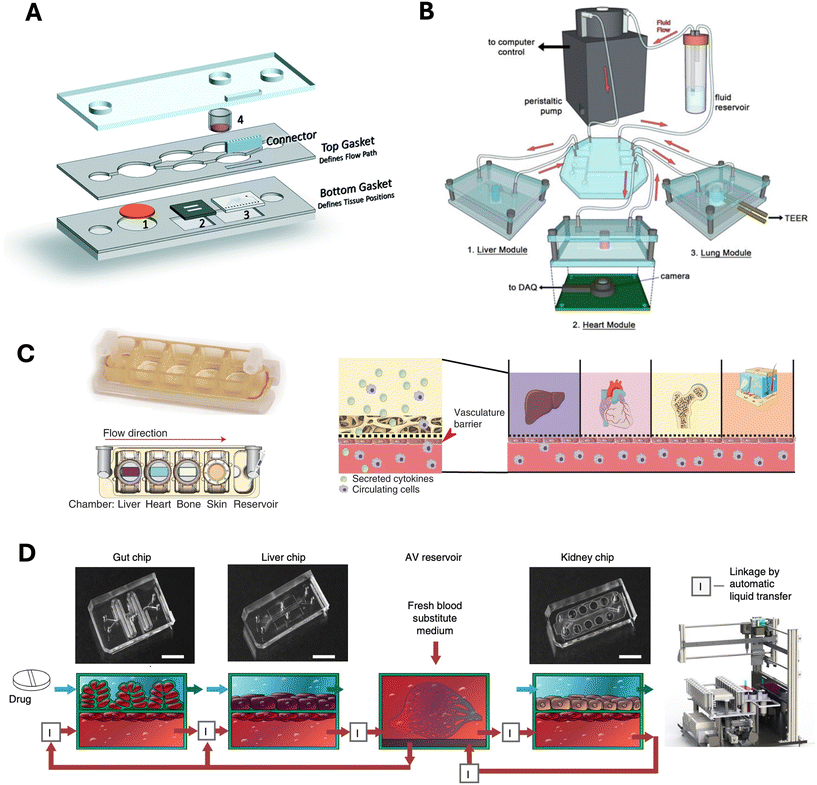 |
| Fig. 3 Selected examples of multi-organ chips. A) Multi-organ chip with liver, heart, and skin compartment for drug toxicity studies. Reproduced with permission from Pires de Mello et al.,144 Royal Society of Chemistry. B) Liver, lung, and heart module connected via tubing to study drug response. Reprinted with permission from Skardal et al.,146 Springer Nature Limited. C) Modular multi-organ chip with individual compartments for liver, heart, bone, and skin with a vascular barrier for crosstalk. Reprinted with permission from Ronaldson-Bouchard et al.,147 Springer Nature Limited. D) Organ modules connected via liquid transfer between the endothelium channels. Reprinted with permission from Herland et al.,148 Springer Nature Limited. | |
Multi-organ chips for on- and off-target drug toxicity studies.
To evaluate possible side effects of drugs on non-target tissues or the toxicity of secondary metabolites on target and off-target tissues, further organ models may be integrated.149 Oleaga et al. demonstrated a four-organ system (heart, liver, neurons, muscle) to study the toxicity of five different drugs with gravity-driven bidirectional flow, indicating that the developed in vitro model leads to comparable results to already published toxicity studies in humans or animals.150 Satoh et al. developed a pressure-driven circulating flow multi-organ platform that was used as a two-organ (liver, cancer) or four-organ system (intestine, liver, cancer, connective tissue) to study drug effects. As an example, the prodrugs capecitabine and tegafur (resulting in 5-fluorouracil after metabolism) as well as 5-fluorouracil itself were administered on the apical side of the intestine model, and reduced proliferation of HCT-116 (cancerous cell line) and TIG-121 (connective tissue cell line) could be measured on day 3.151 Skardal et al. connected a liver, heart and lung model, each cultivated on separate chips, and employed a peristaltic pump for circular fluid transport (Fig. 3B). On the third day of perfusion, the drug bleomycin (targeting lung tissue) was administered and, although the drug is not known for cardiotoxicity, changes in the heart organoid's morphology were observed. However, only bleomycin does not result in morphological changes, indicating that secondary products induced this change, likely the IL-1β secreted from the lung tissue.146 In a later study, the platform was extended to include six different tissue models (liver, heart, lung, colon, brain, vasculature) to study the toxicity of anticancer drugs that are metabolized in the liver to its active form. Both tested drugs, capecitabine and cyclophosphamide, showed toxicity in the heart and lung organoids when the liver organoid was present to metabolize the drug, demonstrating the applicability for studying non-target tissue toxicity.51 Edington et al. developed a pneumatically driven multi-organ platform for the cultivation and connection of four, seven or ten in vitro models with circulating flow. Thereby, the pharmacokinetics of diclofenac with the platform for seven in vitro models was studied. After administering the drug on the apical side of the gut, the drug's concentrations as well as the metabolite's (4′-Hydroxydiclofenac) concentration increased over time in the other organ models, while the concentration in the apical side decreased.152 However, to further increase the physiological relevance of the integrated tissue models and to study the crosstalk between the organ models better, the integration of a vascular systems is desirable. Thereby, also drug circulation throughout the body can be resembled more closely. However, to avoid waste accumulation and non-physiological drug concentrations, continuous fresh medium supply needs to be considered.153,154 The linking of organ models with vascular flow was addressed by Ronaldson-Bouchard et al., demonstrating a multi-organ chip (liver, heart, bone, skin, vasculature) that incorporated organ models that were cultivated and differentiated (from hiPSC) beforehand. After assembly, the tissues are separated by an endothelial barrier but connected with circulating vascular flow, and drug screening capability is tested with doxorubicin, a liver metabolizing drug (Fig. 3C). The study showed that the matured phenotypes could be maintained over four weeks in the chip and clinical PK/PD profiles were reproduced.147 Herland et al. studied PK responses of organ-on-a-chip models by transferring fluids between the endothelium-lined vascular channels of gut, liver and kidney models or bone marrow, liver and kidney models to simulate drug transport through blood circulation (Fig. 3D). Thereby, PK parameters of nicotine and cisplatin were predicted and moreover, PD responses as observed in humans replicated, demonstrating the potential for in vitro to in vivo translation of organ-on-a-chip models. In this study, an arteriovenous reservoir was integrated and organ-specific blood volume fraction, blood flow rate and vessel dimensions considered to scale the dimensions and flow rates accordingly.148 Taken together, while simpler systems, such as those based on bidirectional or circular flow, are cost-effective and user-friendly, they are limited in their ability to replicate certain aspects of human physiology. Key considerations, such as selecting all relevant organ models, incorporating more physiological flow dynamics, and integration of a vascular system, are essential to simulate human physiology more accurately and comprehensively, ultimately making the next generation of multi-organ-on-a-chip models more reliable for testing the efficacy and safety of drug candidates.
3.3 Advancing the operation of multi-organ chips
Despite the advances of multi-organ chips in drug toxicity studies, standardization and throughput still need to be improved to increase the use in routine drug screening studies.155 Thereby, automation and high-throughput capability of multi-organ chips are of high interest, since they reduce the cell culture handling complexity while simultaneously increasing parallelization. At the same time, the used technology needs to provide standardized operation, quality control steps to increase the predictiveness and comparability of experimental results. Among other advances, in this chapter we highlight two promising advances in the operation of multi-organ chips. Firstly, modular system configurations are introduced as powerful tool since they allow separate cultivation of different organ models before assembly, enabling individual quality control of the organ (disease) before starting multi-organ studies. Secondly, advances towards high-throughput and automation of cultivation processes are summarized.
Complexity and ease-of-use.
While advanced multi-organ models allow the study of complex multicellular interactions prior to, during, and after drug treatments, the increased complexity associated with device handling procedures makes them less user-friendly and prone to operator failure, especially when using long-term organoid cultures. Additionally, in many cases each individual organ model may require a specific growth medium during proliferation, differentiation, and maturation, thus adding an additional challenge to the development of automated and scalable multi-organ models. Another layer of complexity is generated when using diseased, aged, or inflamed tissue models, because they are known to affect the entire system, therefore complicating the precise study of individual organ responses. To address these challenges, one promising approach has been the spatial separation of the involved organ models during their initial development phase and following combination during the experimentation phase (Fig. 4). This modular strategy is emerging as an effective solution to manage the increasing complexity of multi-organ systems, while maintaining the possibility to perform quality control steps before assembling the multi-organ system and to simplify end-point analysis of individual compartments. An example, developed by Ronaldson-Bouchard et al., is the introduction of a modular platform consisting of a heart, liver, bone, and skin tissue model. The individual tissue structures were connected by vascular flow and separated via an endothelial barrier system following the maturation of each tissue type under their preferred conditions. This means that quality control assessment of each individual tissue model was possible prior to final assembly into the integrated system. Individual compartments were plugged into a holder platform and connected via active pumping, generating functional and structural integrity of the organ models over a four-week culture period.147 The main challenge with these modular microfluidic systems is to ensure sterile, safe and reliable connections between the tissue compartments using various tubings, luer and O-ring connections as well as adhesives.156 In an effort to standardize modular biochip systems, researchers have developed stable mechanical connections, similar to LEGO®-style connectors, magnet-aided, shape-complementary, and threaded fittings.157 Another example used by Esch et al. is built on a screw-based method to stack membrane models in a modular platform for co-culturing GIT and liver.118 Ong et al. developed a tetris™-like modular approach, connecting different modules via magnets. The feasibility of the introduced system was demonstrated by investigating the effect of quercetin on a liver–endothelium model and the chemotherapeutic properties of Cyclophosphamide on a three-tissue model consisting of liver, endothelium and tumor.158 Such flexible modular systems offer a strategic advantage, even allowing integration of complex 3D models such as organoids while minimizing costs. Carvalho et al. developed a LEGO®-like modular microfluidic platform for the cultivation of thyroid organoids, characterized by high modularity and scalability. This system maintained the shape and viability of the organoids, and its potential could be further exploited by using it for the co-cultivation of organoids with other tissues.159 In another study, Koh et al. presented a modular model of the blood–brain barrier and glioblastoma, highlighting the system's ability to integrate organoids in the future.160 However, the handling of the system needs to be improved to make it feasible for higher throughput applications. Additional challenges of operating multi-organ systems involve increasing throughput and transitioning from single-use to reusable designs. For instance, Sun et al. worked on reusable standardized modules that consisted of a PMMA-based connecting module,161 while Hou et al. focused on achieving high throughput using a biomimetic chip array for co-culturing liver and tumor microtissues in the absence of fluid flow.162 The above approaches represent some of the ongoing efforts to refine modular microfluidic systems, aiming for secure, efficient, and flexible connections that maintain high tissue quality and functionality.
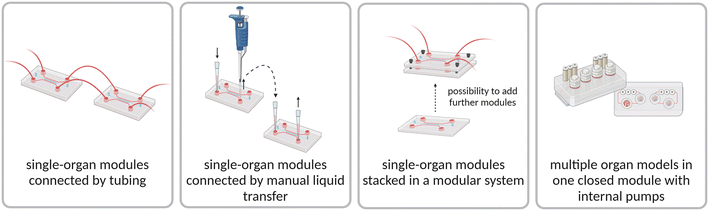 |
| Fig. 4 Illustrations of different technical approaches to connect various organ modules. | |
High throughput and automation.
An important aspect of on-chip models for ADME studies is the interconnection of several organ modules. Technically, various methods have been developed to connect multiple organ systems (Fig. 4). These methods include the use of tubing, manual medium transfer, modular systems, and closed modules with internal pumps. Thereby, the use of biochip materials with low non-specific adsorption characteristics is essential to account for the elimination of circulatory factors.154 In general, to enable high-throughput drug screening with multi-organ models, the medium exchange needs to be automatable. As an example, Novak et al. developed an ‘interrogator’ that automates liquid handling by using robotics and a customized software as well as imaging by an integrated microscope. Thereby, eight different fluidically coupled organ-chip models were maintained viable over three weeks.163 By using two-channel organ models with a vascular barrier, organ models can be supplied with their required medium, while mass transport can be maintained by transferring medium between the endothelium-lined vascular channels without requiring tubing for connections.148,163
Another approach has been the design of platforms based on the dimensions of a microtiter plate, enabling the use of pipetting robots and allowing readouts to be performed with standard laboratory equipment, such as a plate reader. As an example, Azizgolshani et al. developed an organ-on-chip platform for high-throughput screening based on a standard plate facilitating 96 cultivations. This platform can be applied for co-culture studies and has integrated electrical sensors for barrier function measurements and an optical sensor for dissolved oxygen determination.164 In a follow up study, the developed platform was used for co-culturing endothelial cells and pericytes to study the permeability as well as the response to shear stress and TNF-α stimuli.165 However, this platform has not been used for drug screening applications yet. In another study, Hou et al. developed a high-throughput platform based on a 384-well microplate consisting of two chips for cultivating liver and tumor microtissue separately and assembling them for compound screening later. The feasibility of the developed platform was verified with anticancer drugs such as the CPT-11, epirubicin, adriamycin and plumbagin.162 Important to highlight are the high-throughput capabilities of the OrganoPlate®, housing 96 chips on a well plate format. Each chip consists of a connected organ and endothelium compartment with perfusion induced by placing the plate on a rocker. The design based on a well plate allows automation with a liquid handler.110 By automizing the cultivation of organ-chip models, reproducibility and throughput are increased and thereby the time to identify lead drug candidates is reduced, leading to a broader applicability in the industry.46
4 Conclusion
Recent advances in the development of miniaturized and automated multi-organ chips have opened new avenues in the understanding of drug absorption and metabolism by investigating complex organ-organ interactions. It is crucial to emphasize that multi-organ chips, especially those incorporating a liver model, are essential for drug screening studies since the hepatic tissue plays a key role in drug metabolism and its metabolites can significantly affect other tissues. Thereby, not only the drug's hepatotoxicity but also the effect of a drug and/or its metabolites on off-target organs, such as the heart, can be evaluated. Furthermore, the constant crosstalk between different organs enables to further understand signaling as a response to drug exposure. However, besides the many promising organs-on-a-chip developments, limitations such as complex cultivation procedures for establishing and maintaining different organ models remain. The main drawback is their moderate throughput, which will continue to impede the transition from academic settings to the industrial sector. To make these systems more feasible for industrial usage and high-throughput screening applications, design adaptations along with automated liquid handlers or modular systems are needed. The separate cultivation of modular systems allows not only quality control before assembly and drug testing, but also fosters parallelization and throughput. Additionally, further improvement of the biological model, for example through vascularization of organ models, enhances the translation to the human body. Consequently, continuous improvements on the technological and biological side of multi-organ systems will result in broader applicability in drug testing, better predictability of drug candidates and thereby reduce animal testing in drug development.
Data availability
No primary research results, software or code have been included and no new data were generated or analysed as part of this review.
Conflicts of interest
There are no conflicts to declare.
Acknowledgements
This work was supported by the ActiTOX project (H2020-MSCA-RISE-2018, project no.: 823981). Illustrations were created in http://Biorender.com. The authors acknowledge TU Wien Bibliothek for financial support through its Open Access Funding Program.
References
- D. Sun, W. Gao, H. Hu and S. Zhou, Why 90% of Clinical Drug Development Fails and How to Improve It?, Acta Pharm. Sin. B, 2022, 12(7), 3049–3062, DOI:10.1016/J.APSB.2022.02.002.
- C. H. Wong, K. W. Siah and A. W. Lo, Estimation of Clinical Trial Success Rates and Related Parameters, Biostatistics, 2019, 20(2), 273–286, DOI:10.1093/BIOSTATISTICS/KXX069.
- R. K. Harrison, Phase II and Phase III Failures: 2013–2015, Nat. Rev. Drug Discovery, 2016, 15(12), 817–818, DOI:10.1038/NRD.2016.184.
- N. Franzen, W. H. van Harten, V. P. Retèl, P. Loskill, J. van den Eijnden-van Raaij and M. IJzerman, Impact of Organ-on-a-Chip Technology on Pharmaceutical R&D Costs, Drug Discovery Today, 2019, 24(9), 1720–1724, DOI:10.1016/J.DRUDIS.2019.06.003.
- B. Zhang, A. Korolj, B. F. L. Lai and M. Radisic, Advances in Organ-on-a-Chip Engineering, Nat. Rev. Mater., 2018, 3(8), 257–278, DOI:10.1038/s41578-018-0034-7.
- N. Picollet-D'hahan, A. Zuchowska, I. Lemeunier and S. Le Gac, Multiorgan-on-a-Chip: A Systemic Approach To Model and Decipher Inter-Organ Communication, Trends Biotechnol., 2021, 39(8), 788–810, DOI:10.1016/J.TIBTECH.2020.11.014.
- M. Malik, Y. Yang, P. Fathi, G. J. Mahler and M. B. Esch, Critical Considerations for the Design of Multi-Organ Microphysiological Systems (MPS), Front. Cell Dev. Biol., 2021, 9, 2353, DOI:10.3389/FCELL.2021.721338/BIBTEX.
- Y. I. Wang, C. Carmona, J. J. Hickman and M. L. Shuler, Multiorgan Microphysiological Systems for Drug Development: Strategies, Advances, and Challenges, Adv. Healthcare Mater., 2018, 7(2), 1701000, DOI:10.1002/ADHM.201701000.
- A. Stewart, D. Denoyer, X. Gao and Y. C. Toh, The FDA Modernisation Act 2.0: Bringing Non-Animal Technologies to the Regulatory Table, Drug Discovery Today, 2023, 28(4), 103496, DOI:10.1016/J.DRUDIS.2023.103496.
- M. A. Sellwood, M. Ahmed, M. H. S. Segler and N. Brown, Artificial Intelligence in Drug Discovery, Future Med. Chem., 2018, 10(17), 2025–2028, DOI:10.4155/fmc-2018–0212.
- S. H. Lee and J. H. Sung, Microtechnology-Based Multi-Organ Models, Bioengineering, 2017, 4(2), 46, DOI:10.3390/BIOENGINEERING4020046.
- A. Zhang, K. Meng, Y. Liu, Y. Pan, W. Qu, D. Chen and S. Xie, Absorption, Distribution, Metabolism, and Excretion of Nanocarriers in Vivo and Their Influences, Adv. Colloid Interface Sci., 2020, 284, 102261, DOI:10.1016/j.cis.2020.102261.
- M. Li, K. T. Al-Jamal, K. Kostarelos and J. Reineke, Physiologically Based Pharmacokinetic Modeling of Dermal Absorption, ASC Nano, 2010, 4(11), 6303–6317, DOI:10.3109/9781841848570.037.
- L. H. Chi, A. D. Burrows and R. L. Anderson, Can Preclinical Drug Development Help to Predict Adverse Events in Clinical Trials?, Drug Discovery Today, 2022, 27(1), 257–268, DOI:10.1016/J.DRUDIS.2021.08.010.
- H. J. Freeman and A. B. R. Thomson, The Small Intestine, Clin. Gastroenterol., 2006, 14(4), 689–723, DOI:10.1097/00000441-196608000-00027.
- K. Thelen and J. B. Dressman, Cytochrome P450-Mediated Metabolism in the Human Gut Wall, J. Pharm. Pharmacol., 2010, 61(5), 541–558, DOI:10.1211/JPP.61.05.0002.
- M. Herath, S. Hosie, J. C. Bornstein, A. E. Franks and E. L. Hill-Yardin, The Role of the Gastrointestinal Mucus System in Intestinal Homeostasis: Implications for Neurological Disorders, Front. Cell. Infect. Microbiol., 2020, 10, 248, DOI:10.3389/fcimb.2020.00248.
- F. M. Gribble and F. Reimann, Enteroendocrine Cells: Chemosensors in the Intestinal Epithelium, Annu. Rev. Physiol., 2016, 78, 277–299, DOI:10.1146/annurev-physiol-021115–105439.
- N. Zeitouni, J. Fandrey, H. Y. Naim and M. von Köckritz-Blickwede, Measuring Oxygen Levels in Caco-2 Cultures, Hypoxia, 2015, 53, DOI:10.2147/hp.s85625.
- H. J. Kim, D. Huh, G. Hamilton and D. E. Ingber, Human Gut-on-a-Chip Inhabited by Microbial Flora That Experiences Intestinal Peristalsis-like Motions and Flow, Lab Chip, 2012, 12(12), 2165–2174, 10.1039/c2lc40074j.
- M. Ghadiri, P. M. Young and D. Traini, Strategies to Enhance Drug Absorption via Nasal and Pulmonary Routes, Pharmaceutics, 2019, 11(3), 113, DOI:10.3390/PHARMACEUTICS11030113.
- P. Nighot and T. Ma, Endocytosis of Intestinal Tight Junction Proteins: In Time and Space, Inflammatory Bowel Dis., 2021, 27(2), 283–290, DOI:10.1093/IBD/IZAA141.
- N. Noureddine, M. Chalubinski and P. Wawrzyniak, The Role of Defective Epithelial Barriers in Allergic Lung Disease and Asthma Development, J. Asthma Allergy, 2022, 15, 487–504, DOI:10.2147/JAA.S324080.
- E. Fröhlich, Non-Cellular Layers of the Respiratory Tract: Protection against Pathogens and Target for Drug Delivery, Pharmaceutics, 2022, 14(5), 992, DOI:10.3390/pharmaceutics14050992.
- E. Fröhlich and S. Salar-Behzadi, Toxicological Assessment of Inhaled Nanoparticles: Role of in Vivo, Ex Vivo, in Vitro, and in Silico Studies, Int. J. Mol. Sci., 2014, 15(3), 4795–4822, DOI:10.3390/ijms15034795.
- M. R. Lascia, Polonelli, L, Pulm. Drug Delivery, 2018, 1–67 Search PubMed.
- C. Carvalho, N. Daum and C. M. Lehr, Carrier Interactions with the Biological Barriers of the Lung: Advanced in Vitro Models and Challenges for Pulmonary Drug Delivery, Adv. Drug Delivery Rev., 2014, 75, 129–140, DOI:10.1016/j.addr.2014.05.014.
- B. Button, R. C. Boucher and University of North Carolina Virtual Lung Group, Role of Mechanical Stress in Regulating Airway Surface Hydration and Mucus Clearance Rates, Respir. Physiol. Neurobiol., 2008, 163(1–3), 189–201, DOI:10.1016/J.RESP.2008.04.020.
- V. Phatale, K. K. Vaiphei, S. Jha, D. Patil, M. Agrawal and A. Alexander, Overcoming Skin Barriers through Advanced Transdermal Drug Delivery Approaches, J. Controlled Release, 2022, 351, 361–380, DOI:10.1016/j.jconrel.2022.09.025.
- N. Tiwari, E. R. Osorio-Blanco, A. Sonzogni, D. Esporrín-Ubieto, H. Wang and M. Calderón, Nanocarriers for Skin Applications: Where Do We Stand?, Angew. Chem., Int. Ed., 2022, 61(3), e202107960, DOI:10.1002/anie.202107960.
-
M. Vestita, P. Tedeschi and D. Bonamonte, Anatomy and Physiology of the Skin, in Textbook of Plastic and Reconstructive Surgery: Basic Principles and New Perspectives, 2022, pp. 3–13, DOI:10.1007/978-3-030-82335-1_1.
- J. E. Lai-Cheong and J. A. McGrath, Structure and Function of Skin, Hair and Nails, Medicine, 2017, 45(6), 347–351, DOI:10.1016/j.mpmed.2017.03.004.
- C. Gorzelanny, C. Mess, S. W. Schneider, V. Huck and J. M. Brandner, Skin Barriers in Dermal Drug Delivery: Which Barriers Have to Be Overcome and How Can We Measure Them?, Pharmaceutics, 2020, 12(7), 684, DOI:10.3390/pharmaceutics12070684.
- U. Blume-Peytavi and A. Vogt, Human Hair Follicle: Reservoir Function and Selective Targeting, Br. J. Dermatol., 2011, 165(s2), 13–17, DOI:10.1111/j.1365–2133.2011.10572.x.
- A. J. Foster, B. Chouhan, S. L. Regan, H. Rollison, S. Amberntsson, L. C. Andersson, A. Srivastava, M. Darnell, J. Cairns, S. E. Lazic, K. J. Jang, D. B. Petropolis, K. Kodella, J. E. Rubins, D. Williams, G. A. Hamilton, L. Ewart and P. Morgan, Integrated in Vitro Models for Hepatic Safety and Metabolism: Evaluation of a Human Liver-Chip and Liver Spheroid, Arch. Toxicol., 2019, 93(4), 1021–1037, DOI:10.1007/s00204-019-02427-4.
- A. Corsini and M. Bortolini, Drug-Induced Liver Injury: The Role of Drug Metabolism and Transport, J. Clin. Pharmacol., 2013, 53(5), 463–474, DOI:10.1002/jcph.23.
- B. Corrado, V. De Gregorio, G. Imparato, C. Attanasio, F. Urciuolo and P. A. Netti, A Three-Dimensional Microfluidized Liver System to Assess Hepatic Drug Metabolism and Hepatotoxicity, Biotechnol. Bioeng., 2019, 116(5), 1152–1163, DOI:10.1002/BIT.26902.
- E. Trefts, M. Gannon and D. H. Wasserman, The Liver, Curr. Biol., 2017, 27(21), R1147–R1151, DOI:10.1016/j.cub.2017.09.019.
- F. Tasnim, J. Xing, X. Huang, S. Mo, X. Wei, M. H. Tan and H. Yu, Generation of Mature Kupffer Cells from Human Induced Pluripotent Stem Cells, Biomaterials, 2019, 192, 377–391, DOI:10.1016/j.biomaterials.2018.11.016.
-
Y. Mizuguchi, S. Specht, K. Isse, J. G. Lunz and A. J. Demetris, Biliary Epithelial Cells, Molecular pathology of liver diseases, 2011, vol. 5, pp. 27–51, DOI:10.1007/978-1-4419-7107-4_4.
- K. Tsaioun, B. J. Blaauboer and T. Hartung, Evidence-Based Absorption, Distribution, Metabolism, Excretion (ADME) and Its Interplay with Alternative Toxicity Methods, ALTEX, 2016, 33(4), 343–358, DOI:10.14573/altex.1610101.
- J. Poisson, S. Lemoinne, C. Boulanger, F. Durand, R. Moreau, D. Valla and P. E. Rautou, Liver Sinusoidal Endothelial Cells: Physiology and Role in Liver Diseases, J. Hepatol., 2017, 66(1), 212–227, DOI:10.1016/J.JHEP.2016.07.009.
- S. Prill, D. Bavli, G. Levy, E. Ezra, E. Schmälzlin, M. S. Jaeger, M. Schwarz, C. Duschl, M. Cohen and Y. Nahmias, Real-Time Monitoring of Oxygen Uptake in Hepatic Bioreactor Shows CYP450-Independent Mitochondrial Toxicity of Acetaminophen and Amiodarone, Arch. Toxicol., 2016, 90(5), 1181–1191, DOI:10.1007/s00204-015-1537-2.
- P. Mirabelli, L. Coppola and M. Salvatore, Cancer Cell Lines Are Useful Model Systems for Medical Research, Cancers, 2019, 11(8), 1098, DOI:10.3390/cancers11081098.
- V. E. J. M. Palasantzas, I. Tamargo-Rubio, K. Le, J. Slager, C. Wijmenga, I. H. Jonkers, V. Kumar, J. Fu and S. Withoff, IPSC-Derived Organ-on-a-Chip Models for Personalized Human Genetics and Pharmacogenomics Studies, Trends Genet., 2023, 39(4), 268–284, DOI:10.1016/J.TIG.2023.01.002.
- Y. Zhao, S. Landau, S. Okhovatian, C. Liu, R. Xing, Z. Lu, B. Fook, L. Lai, Q. Wu, J. Kieda, K. Cheung, S. Rajasekar, K. Jozani, B. Zhang and M. Radisic, Integrating Organoids and Organ-on-a-Chip Devices, Nat. Rev. Bioeng., 2024, 2(7), 588–608, DOI:10.1038/s44222-024-00207-z.
- S. M. Jung and S. Kim, In Vitro Models of the Small Intestine for Studying Intestinal Diseases, Front. Microbiol., 2022, 12, 767038, DOI:10.3389/FMICB.2021.767038/BIBTEX.
- T. Däullary, F. Imdahl, O. Dietrich, L. Hepp, T. Krammer, C. Fey, W. Neuhaus, M. Metzger, J. Vogel, A. J. Westermann, A. E. Saliba and D. Zdzieblo, A Primary Cell-Based in Vitro Model of the Human Small Intestine Reveals Host Olfactomedin 4 Induction in Response to Salmonella Typhimurium Infection, Gut Microbes, 2023, 15(1), 2186109, DOI:10.1080/19490976.2023.2186109.
- J. Zhang, E. Wehrle, M. Rubert and R. Müller, 3D Bioprinting of Human Tissues: Biofabrication, Bioinks, and Bioreactors, Int. J. Mol. Sci., 2021, 22(8), 3971, DOI:10.3390/IJMS22083971.
- T. Messelmani, L. Morisseau, Y. Sakai, C. Legallais, A. Le Goff, E. Leclerc and R. Jellali, Liver Organ-on-Chip Models for Toxicity Studies and Risk Assessment, Lab Chip, 2022, 22(13), 2423–2450, 10.1039/D2LC00307D.
- A. Skardal, J. Aleman, S. Forsythe, S. Rajan, S. Murphy, M. Devarasetty, N. Pourhabibi Zarandi, G. Nzou, R. Wicks, H. Sadri-Ardekani, C. Bishop, S. Soker, A. Hall, T. Shupe and A. Atala, Drug Compound Screening in Single and Integrated Multi-Organoid Body-on-a-Chip Systems, Biofabrication, 2020, 12(2), 025017, DOI:10.1088/1758–5090/AB6D36.
- J. Taelman, M. Diaz and J. Guiu, Human Intestinal Organoids: Promise and Challenge, Front. Cell Dev. Biol., 2022, 10, 854740, DOI:10.3389/FCELL.2022.854740/BIBTEX.
- C. M. Leung, P. de Haan, K. Ronaldson-Bouchard, G. A. Kim, J. Ko, H. S. Rho, Z. Chen, P. Habibovic, N. L. Jeon, S. Takayama, M. L. Shuler, G. Vunjak-Novakovic, O. Frey, E. Verpoorte and Y. C. Toh, A Guide to the Organ-on-a-Chip, Nat. Rev. Methods Primers, 2022, 2(1), 1–29, DOI:10.1038/s43586-022-00118-6.
- P. Schuller, M. Rothbauer, S. R. A. Kratz, G. Höll, P. Taus, M. Schinnerl, J. Genser, N. Bastus, O. H. Moriones, V. Puntes, B. Huppertz, M. Siwetz, H. Wanzenböck and P. Ertl, A Lab-on-a-Chip System with an Embedded Porous Membrane-Based Impedance Biosensor Array for Nanoparticle Risk Assessment on Placental Bewo Trophoblast Cells, Sens. Actuators, B, 2020, 312, 127946, DOI:10.1016/J.SNB.2020.127946.
- S. Jalili-Firoozinezhad, F. S. Gazzaniga, E. L. Calamari, D. M. Camacho, C. W. Fadel, A. Bein, B. Swenor, B. Nestor, M. J. Cronce, A. Tovaglieri, O. Levy, K. E. Gregory, D. T. Breault, J. M. S. Cabral, D. L. Kasper, R. Novak and D. E. Ingber, A Complex Human Gut Microbiome Cultured in an Anaerobic Intestine-on-a-Chip, Nat. Biomed. Eng., 2019, 3(7), 520–531, DOI:10.1038/s41551-019-0397-0.
- N. Panse and P. M. Gerk, The Caco-2 Model: Modifications and Enhancements to Improve Efficiency and Predictive Performance, Int. J. Pharm., 2022, 624, 122004, DOI:10.1016/J.IJPHARM.2022.122004.
- K. D. Boger, A. E. Sheridan, A. L. Ziegler and A. T. Blikslager, Mechanisms and Modeling of Wound Repair in the Intestinal Epithelium, Tissue Barriers, 2023, 11(2), 2087454, DOI:10.1080/21688370.2022.2087454.
- B. Yi, K. Y. Shim, S. K. Ha, J. Han, H. H. Hoang, I. Choi, S. Park and J. H. Sung, Three-Dimensional in Vitro Gut Model on a Villi-Shaped Collagen Scaffold, BioChip J., 2017, 11(3), 219–231, DOI:10.1007/S13206-017-1307-8.
- J. Creff, L. Malaquin and A. Besson, In Vitro Models of Intestinal Epithelium: Toward Bioengineered Systems, J. Tissue Eng., 2021, 12 DOI:10.1177/2041731420985202.
- S. A. Jelinsky, M. Derksen, E. Bauman, C. S. Verissimo, W. T. M. van Dooremalen, J. L. Roos, C. H. Barón, C. Caballero-Franco, B. G. Johnson, M. G. Rooks, J. Pott, B. Oldenburg, R. G. J. Vries, S. F. Boj, M. T. Kasaian, F. Pourfarzad and C. V. Rosadini, Molecular and Functional Characterization of Human Intestinal Organoids and Monolayers for Modeling Epithelial Barrier, Inflammatory Bowel Dis., 2023, 29(2), 195–206, DOI:10.1093/IBD/IZAC212.
- A. Fedi, C. Vitale, G. Ponschin, S. Ayehunie, M. Fato and S. Scaglione, In Vitro Models Replicating the Human Intestinal Epithelium for Absorption and Metabolism Studies: A Systematic Review, J. Controlled Release, 2021, 335, 247–268, DOI:10.1016/J.JCONREL.2021.05.028.
- M. Kasendra, A. Tovaglieri, A. Sontheimer-Phelps, S. Jalili-Firoozinezhad, A. Bein, A. Chalkiadaki, W. Scholl, C. Zhang, H. Rickner, C. A. Richmond, H. Li, D. T. Breault and D. E. Ingber, Development of a Primary Human Small Intestine-on-a-Chip Using Biopsy-Derived Organoids, Sci. Rep., 2018, 8(1), 1–14, DOI:10.1038/s41598-018-21201-7.
- B. Rothen-Rutishauser, M. Gibb, R. He, A. Petri-Fink and C. M. Sayes, Human Lung Cell Models to Study Aerosol Delivery – Considerations for Model Design and Development, Eur. J. Pharm. Sci., 2023, 180, 106337, DOI:10.1016/J.EJPS.2022.106337.
- D. F. Lee, M. I. Lethem and A. B. Lansley, A Comparison of Three Mucus-Secreting Airway Cell Lines(Calu-3, SPOC1 and UNCN3T) for Use as Biopharmaceutical Models of the Nose and Lung, Eur. J. Pharm. Biopharm., 2021, 167, 159–174, DOI:10.1016/j.ejpb.2021.07.016.
- N. Sibinovska, S. Žakelj, R. Roškar and K. Kristan, Suitability and Functional Characterization of Two Calu-3 Cell Models for Prediction of Drug Permeability across the Airway Epithelial Barrier, Int. J. Pharm., 2020, 585, 119484, DOI:10.1016/j.ijpharm.2020.119484.
- N. Petpiroon, W. Netkueakul, K. Sukrak, C. Wang, Y. Liang, M. Wang, Y. Liu, Q. Li, R. Kamran, K. Naruse, S. Aueviriyavit and K. Takahashi, Development of Lung Tissue Models and Their Applications, Life Sci., 2023, 334, 122208, DOI:10.1016/J.LFS.2023.122208.
- P. R. Tata, H. Mou, A. Pardo-Saganta, R. Zhao, M. Prabhu, B. M. Law, V. Vinarsky, J. L. Cho, S. Breton, A. Sahay, B. D. Medoff and J. Rajagopal, Dedifferentiation of Committed Epithelial Cells into Stem Cells in Vivo, Nature, 2013, 503(7475), 218–223, DOI:10.1038/nature12777.
- R. E. Rayner, P. Makena, G. L. Prasad and E. Cormet-Boyaka, Optimization of Normal Human Bronchial Epithelial(NHBE) Cell 3D Cultures for in Vitro Lung Model Studies, Sci. Rep., 2019, 9(1), 500, DOI:10.1038/S41598-018-36735-Z.
- A. A. Pezzulo, T. D. Starner, T. E. Scheetz, G. L. Traver, A. E. Tilley, B. G. Harvey, R. G. Crystal, P. B. McCray and J. Zabner, The Air-Liquid Interface and Use of Primary Cell Cultures Are Important to Recapitulate the Transcriptional Profile of in Vivo Airway Epithelia, Am. J. Physiol., 2011, 300(1), 25–31, DOI:10.1152/AJPLUNG.00256.2010.
- P. S. Hiemstra, G. Grootaers, A. M. van der Does, C. A. M. Krul and I. M. Kooter, Human Lung Epithelial Cell Cultures for Analysis of Inhaled Toxicants: Lessons Learned and Future Directions, Toxicol. In Vitro, 2018, 47, 137–146, DOI:10.1016/J.TIV.2017.11.005.
- I. M. Kooter, M. Gröllers-Mulderij, M. Steenhof, E. Duistermaat, F. A. A. van Acker, Y. C. M. Staal, P. C. Tromp, E. Schoen, C. F. Kuper and E. van Someren, Cellular Effects in an In Vitro Human 3D Cellular Airway Model and A549/BEAS-2B In Vitro Cell Cultures Following Air Exposure to Cerium Oxide Particles at an Air–Liquid Interface, Applied In Vitro Toxicology, 2016, 2(1), 56–66, DOI:10.1089/AIVT.2015.0030.
- J. Zavala, B. Obrien, K. Lichtveld, K. G. Sexton, I. Rusyn, I. Jaspers and W. Vizuete, Assessment of Biological Responses of EpiAirway 3-D Cell Constructs versus A549 Cells for Determining Toxicity of Ambient Air Pollution, Inhalation Toxicol., 2016, 28(6), 251–259, DOI:10.3109/08958378.2016.1157227.
- K. Zscheppang, J. Berg, S. Hedtrich, L. Verheyen, D. E. Wagner, N. Suttorp, S. Hippenstiel and A. C. Hocke, Human Pulmonary 3D Models For Translational Research, Biotechnol. J., 2018, 13(1), 1700341, DOI:10.1002/BIOT.201700341.
- A. Doryab, J. Groll, A. Doryab and J. Groll, Biomimetic In Vitro Lung Models: Current Challenges and Future Perspective, Adv. Mater., 2023, 35(13), 2210519, DOI:10.1002/ADMA.202210519.
- D. Huh, B. D. Matthews, A. Mammoto, M. Montoya-Zavala, H. Yuan Hsin and D. E. Ingber, Reconstituting Organ-Level Lung Functions on a Chip, Science, 2010, 328(5986), 1662–1668, DOI:10.1126/science.1188302.
- D. Huh, D. C. Leslie, B. D. Matthews, J. P. Fraser, S. Jurek, G. A. Hamilton, K. S. Thorneloe, M. A. McAlexander and D. E. Ingber, A Human Disease Model of Drug Toxicity–Induced Pulmonary Edema in a Lung-on-a-Chip Microdevice, Sci. Transl. Med., 2012, 4(159), 159ra147, DOI:10.1126/SCITRANSLMED.3004249.
- P. Zamprogno, S. Wüthrich, S. Achenbach, G. Thoma, J. D. Stucki, N. Hobi, N. Schneider-Daum, C. M. Lehr, H. Huwer, T. Geiser, R. A. Schmid and O. T. Guenat, Second-Generation Lung-on-a-Chip with an Array of Stretchable Alveoli Made with a Biological Membrane, Commun. Biol., 2021, 4(1), 168, DOI:10.1038/S42003-021-01695-0.
- M. Humayun, C. W. Chow and E. W. K. Young, Microfluidic Lung Airway-on-a-Chip with Arrayable Suspended Gels for Studying Epithelial and Smooth Muscle Cell Interactions, Lab Chip, 2018, 18(9), 1298–1309, 10.1039/C7LC01357D.
- J. D. Stucki, N. Hobi, A. Galimov, A. O. Stucki, N. Schneider-Daum, C. M. Lehr, H. Huwer, M. Frick, M. Funke-Chambour, T. Geiser and O. T. Guenat, Medium Throughput Breathing Human Primary Cell Alveolus-on-Chip Model, Sci. Rep., 2018, 8(1), 1–13, DOI:10.1038/s41598-018-32523-x.
- A. Moreira, M. Müller, P. F. Costa and Y. Kohl, Advanced In Vitro Lung Models for Drug and Toxicity Screening: The Promising Role of Induced Pluripotent Stem Cells, Adv. Biol., 2022, 6(2), 2101139, DOI:10.1002/ADBI.202101139.
-
S. Yadav, K. Fujimoto, T. Takenaga, S. Takahashi, Y. Muramoto, R. Mikawa, T. Noda and S. Gotoh, Yokokawa Isogenic IPSC-Derived Proximal and Distal Lung-on-Chip Models: Tissue- and Virus-Specific Immune Responses in Human Lungs, bioRxiv, 2023, preprint, DOI:10.1101/2023.11.24.568532.
- M. Schäfer-Korting, U. Bock, W. Diembeck, H. J. Düsing, A. Gamer, E. Haltner-Ukomadu, C. Hoffmann, M. Kaca, H. Kamp, S. Kersen, M. Kietzmann, H. C. Korting, H. U. Krächter, C. M. Lehr, M. Liebsch, A. Mehling, C. Müller-Goymann, F. Netzlaff, F. Niedorf, M. K. Rübbelke, U. Schäfer, E. Schmidt, S. Schreiber, H. Spielmann, A. Vuia and M. Weimer, The Use of Reconstructed Human Epidermis for Skin Absorption Testing: Results of the Validation Study, ATLA, Altern. Lab. Anim., 2008, 36(2), 161–187, DOI:10.1177/026119290803600207.
- S. H. Mathes, H. Ruffner and U. Graf-Hausner, The Use of Skin Models in Drug Development, Adv. Drug Delivery Rev., 2014, 69–70, 81–102, DOI:10.1016/j.addr.2013.12.006.
- E. Hofmann, A. Schwarz, J. Fink, L.-P. Kamolz and P. Kotzbeck, Modelling the Complexity of Human Skin In Vitro, Biomedicines, 2023, 11(3), 794, DOI:10.3390/biomedicines11030794.
- E. Footner, K. Firipis, E. Liu, C. Baker, P. Foley, R. M. I. Kapsa, E. Pirogova, C. O'Connell and A. Quigley, Layer-by-Layer Analysis of In Vitro Skin Models, ACS Biomater. Sci. Eng., 2023, 9(11), 5933–5952, DOI:10.1021/acsbiomaterials.3c00283.
- E. Footner, K. Firipis, E. Liu, C. Baker, P. Foley, R. M. I. Kapsa, E. Pirogova, C. O'Connell and A. Quigley, Layer-by-Layer Analysis of In Vitro Skin Models, ACS Biomater. Sci. Eng., 2023, 9(11), 5933–5952, DOI:10.1021/acsbiomaterials.3c00283.
- S. Salameh, N. Tissot, K. Cache, J. Lima, I. Suzuki, P. A. Marinho, M. Rielland, J. Soeur, S. Takeuchi, S. Germain and L. Breton, A Perfusable Vascularized Full-Thickness Skin Model for Potential Topical and Systemic Applications, Biofabrication, 2021, 13(3), 035042, DOI:10.1088/1758–5090/abfca8.
- P. Zoio, S. Lopes-Ventura, J. Marto and A. Oliva, Open-Source Human Skin Model with an in Vivo-like Barrier for Drug Testing, ALTEX, 2022, 39(3), 405–418, DOI:10.14573/altex.2111182.
- F. F. Schmidt, S. Nowakowski and P. J. Kluger, Improvement of a Three-Layered in Vitro Skin Model for Topical Application of Irritating Substances, Front. Bioeng. Biotechnol., 2020, 8, 1–11, DOI:10.3389/fbioe.2020.00388.
- B. Huber, A. Link, K. Linke, S. A. Gehrke, M. Winnefeld and P. J. Kluger, Integration of Mature Adipocytes to Build-Up a Functional Three-Layered Full-Skin Equivalent, Tissue Eng., Part C, 2016, 22(8), 756–764, DOI:10.1089/ten.tec.2016.0141.
- J. Kober, A. Gugerell, M. Schmid, L.-P. Kamolz and M. Keck, Generation of a Fibrin Based Three-Layered Skin Substitute, BioMed Res. Int., 2015, 2015(1), 170427, DOI:10.1155/2015/170427.
- Test No. 439: In Vitro Skin Irritation: Reconstructed Human Epidermis Test Method, OECD Guidelines for the Testing of Chemicals, 2021, Section 4, DOI:10.1787/9789264242845-en.
- H. Zhao, Z. Chen, X. Kang, B. Yang, P. Luo, H. Li and Q. He, The Frontline of Alternatives to Animal Testing: Novel in Vitro Skin Model Application in Drug Development and Evaluation, Toxicol. Sci., 2023, 196(2), 152–169, DOI:10.1093/toxsci/kfad093.
- K. Ackermann, S. Lombardi Borgia, H. C. Korting, K. R. Mewes and M. Schäfer-Korting, The Phenion® Full-Thickness Skin Model for Percutaneous Absorption Testing, Skin Pharmacol. Physiol., 2009, 23(2), 105–112, DOI:10.1159/000265681.
- F. F. Schmidt, S. Nowakowski and P. J. Kluger, Improvement of a Three-Layered in Vitro Skin Model for Topical Application of Irritating Substances, Front. Bioeng. Biotechnol., 2020, 8, 1–11, DOI:10.3389/fbioe.2020.00388.
- N. Mori, Y. Morimoto and S. Takeuchi, Skin Integrated with Perfusable Vascular Channels on a Chip, Biomaterials, 2017, 116, 48–56, DOI:10.1016/j.biomaterials.2016.11.031.
- S. Salameh, N. Tissot, K. Cache, J. Lima, I. Suzuki, P. A. Marinho, M. Rielland, J. Soeur, S. Takeuchi, S. Germain and L. Breton, A Perfusable Vascularized Full-Thickness Skin Model for Potential Topical and Systemic
Applications, Biofabrication, 2021, 13(3), 035042, DOI:10.1088/1758-5090/abfca8.
- N. Ismayilzada, C. Tarar, S. R. Dabbagh, B. K. Tokyay, S. A. Dilmani, E. Sokullu, H. E. Abaci and S. Tasoglu, Skin-on-a-Chip Technologies towards Clinical Translation and Commercialization, Biofabrication, 2024, 16(4), 042001, DOI:10.1088/1758-5090/ad5f55.
- B. Ataç, I. Wagner, R. Horland, R. Lauster, U. Marx, A. G. Tonevitsky, R. P. Azar and G. Lindner, Skin and Hair On-a-Chip: In Vitro Skin Models versus Ex Vivo Tissue Maintenance with Dynamic Perfusion, Lab Chip, 2013, 13(18), 3555–3561, 10.1039/C3LC50227A.
- K. Kim, J. Kim, H. Kim and G. Y. Sung, Effect of α-Lipoic Acid on the Development of Human Skin Equivalents Using a Pumpless Skin-on-a-Chip Model, Int. J. Mol. Sci., 2021, 22(4), 2160, DOI:10.3390/ijms22042160.
- H. E. Abaci, K. Gledhill, Z. Guo, A. M. Christiano and M. L. Shuler, Pumpless Microfluidic Platform for Drug Testing on Human Skin Equivalents, Lab Chip, 2015, 15(3), 882–888, 10.1039/C4LC00999A.
- Z.-X. Hong, S.-T. Zhu, H. Li, J.-Z. Luo, Y. Yang, Y. An, X. Wang and K. Wang, Bioengineered Skin Organoids: From Development to Applications, Mil. Med. Res., 2023, 10(1), 40, DOI:10.1186/s40779-023-00475-7.
- K. S. Vellonen, M. Malinen, E. Mannermaa, A. Subrizi, E. Toropainen, Y. R. Lou, H. Kidron, M. Yliperttula and A. Urtti, A Critical Assessment of in Vitro Tissue Models for ADME and Drug Delivery, J. Controlled Release, 2014, 190, 94–114, DOI:10.1016/j.jconrel.2014.06.044.
- C. C. Bell, D. F. G. Hendriks, S. M. L. Moro, E. Ellis, J. Walsh, A. Renblom, L. Fredriksson Puigvert, A. C. A. Dankers, F. Jacobs, J. Snoeys, R. L. Sison-Young, R. E. Jenkins, Å. Nordling, S. Mkrtchian, B. K. Park, N. R. Kitteringham, C. E. P. Goldring, V. M. Lauschke and M. Ingelman-Sundberg, Characterization of Primary Human Hepatocyte Spheroids as a Model System for Drug-Induced Liver Injury, Liver Function and Disease, Sci. Rep., 2016, 6, 1–13, DOI:10.1038/srep25187.
- S. D. Collins, G. Yuen, T. Tu, M. A. Budzinska, K. Spring, K. Bryant and N. A. Shackel, In Vitro Models of the Liver: Disease Modeling, Drug Discovery and Clinical Applications, Hepatocell. Carcinoma, 2019, 47–67, DOI:10.15586/hepatocellularcarcinoma.2019.
- C. Eilenberger, M. Rothbauer, E. K. Ehmoser, P. Ertl and S. Küpcü, Effect of Spheroidal Age on Sorafenib Diffusivity and Toxicity in a 3D HepG2 Spheroid Model, Sci. Rep., 2019, 9(1), 1–11, DOI:10.1038/s41598-019-41273-3.
- J. W. Yang, D. Khorsandi, L. Trabucco, M. Ahmed, A. Khademhosseini, M. R. Dokmeci, J. Y. Ye and V. Jucaud, Liver-on-a-Chip Integrated with Label-Free Optical Biosensors for Rapid and Continuous Monitoring of Drug-Induced Toxicity, Small, 2024, 2403560, DOI:10.1002/SMLL.202403560.
- Y. Wang, H. Wang, P. Deng, W. Chen, Y. Guo, T. Tao and J. Qin, In Situ Differentiation and Generation of Functional Liver Organoids from Human IPSCs in a 3D Perfusable Chip System, Lab Chip, 2018, 18(23), 3606–3616, 10.1039/C8LC00869H.
- L. Ewart, A. Apostolou, S. A. Briggs, C. V. Carman, J. T. Chaff, A. R. Heng, S. Jadalannagari, J. Janardhanan, K. J. Jang, S. R. Joshipura, M. M. Kadam, M. Kanellias, V. J. Kujala, G. Kulkarni, C. Y. Le, C. Lucchesi, D. V. Manatakis, K. K. Maniar, M. E. Quinn, J. S. Ravan, A. C. Rizos, J. F. K. Sauld, J. D. Sliz, W. Tien-Street, D. R. Trinidad, J. Velez, M. Wendell, O. Irrechukwu, P. K. Mahalingaiah, D. E. Ingber and J. W. Scannell, Levner, D. Performance Assessment and Economic Analysis of a Human Liver-Chip for Predictive Toxicology, Commun. Med., 2022, 2(1), 1–16, DOI:10.1038/s43856-022-00209-1.
- K. M. Bircsak, R. DeBiasio, M. Miedel, A. Alsebahi, R. Reddinger, A. Saleh, T. Shun, L. A. Vernetti and A. Gough, A 3D Microfluidic Liver Model for High Throughput Compound Toxicity Screening in the OrganoPlate®, Toxicology, 2021, 450, 152667, DOI:10.1016/J.TOX.2020.152667.
- E. Moradi, S. Jalili-Firoozinezhad and M. Solati-Hashjin, Microfluidic Organ-on-a-Chip Models of Human Liver Tissue, Acta Biomater., 2020, 116, 67–83, DOI:10.1016/J.ACTBIO.2020.08.041.
- M. Lucchetti, K. Oluwasegun Aina, L. Grandmougin, C. Jäger, P. Pérez Escriva, E. Letellier, A. S. Mosig, P. Wilmes, M. Lucchetti, L. Grandmougin, C. Jäger, P. Wilmes, K. O. Aina, A. S. Mosig, P. Pérez Escriva and E. Letellier, An Organ-on-Chip Platform for Simulating Drug Metabolism Along the Gut–Liver Axis, Adv. Healthcare Mater., 2024, 2303943, DOI:10.1002/ADHM.202303943.
- N. Milani, N. Parrott, D. Ortiz Franyuti, P. Godoy, A. Galetin, M. Gertz and S. Fowler, Application of a Gut–Liver-on-a-Chip Device and Mechanistic Modelling to the Quantitative in Vitro Pharmacokinetic Study of Mycophenolate Mofetil, Lab Chip, 2022, 22(15), 2853–2868, 10.1039/D2LC00276K.
- C. W. McAleer, C. J. Long, D. Elbrecht, T. Sasserath, L. R. Bridges, J. W. Rumsey, C. Martin, M. Schnepper, Y. Wang, F. Schuler, A. B. Roth, C. Funk, M. L. Shuler and J. J. Hickman, Multi-Organ System for the Evaluation of Efficacy and off-Target Toxicity of Anticancer Therapeutics, Sci. Transl. Med., 2019, 11(497), eaav1386, DOI:10.1126/SCITRANSLMED.AAV1386.
- N. Tsamandouras, W. L. K. Chen, C. D. Edington, C. L. Stokes, L. G. Griffith and M. Cirit, Integrated Gut and Liver Microphysiological Systems for Quantitative In Vitro Pharmacokinetic Studies, AAPS J., 2017, 19(5), 1499–1512, DOI:10.1208/S12248-017-0122-4/FIGURES/8.
- T. Bricks, P. Paullier, A. Legendre, M. J. Fleury, P. Zeller, F. Merlier, P. M. Anton and E. Leclerc, Development of a New Microfluidic Platform Integrating Co-Cultures of Intestinal and Liver Cell Lines, Toxicol. In Vitro, 2014, 28(5), 885–895, DOI:10.1016/J.TIV.2014.02.005.
- J. M. Prot, L. Maciel, T. Bricks, F. Merlier, J. Cotton, P. Paullier, F. Y. Bois and E. Leclerc, First Pass Intestinal and Liver Metabolism of Paracetamol in a Microfluidic Platform Coupled with a Mathematical Modeling as a Means of Evaluating ADME Processes in Humans, Biotechnol. Bioeng., 2014, 111(10), 2027–2040, DOI:10.1002/BIT.25232.
- M. B. Esch, H. Ueno, D. R. Applegate and M. L. Shuler, Modular, Pumpless Body-on-a-Chip Platform for the Co-Culture of GI Tract Epithelium and 3D Primary Liver Tissue, Lab Chip, 2016, 16(14), 2719–2729, 10.1039/C6LC00461J.
- M. Wang, Y. Sasaki, R. Sakagami, T. Minamikawa, M. Tsuda, R. Ueno, S. Deguchi, R. Negoro, K. So, Y. Higuchi, R. Yokokawa, K. Takayama and F. Yamashita, Perfluoropolyether-Based Gut-Liver-on-a-Chip for the Evaluation of First-Pass Metabolism and Oral Bioavailability of Drugs, ACS Biomater. Sci. Eng., 2024, 10, 4635–4644, DOI:10.1021/ACSBIOMATERIALS.4C00605.
- I. Wagner, E.-M. Materne, S. Brincker, U. Süßbier, C. Frädrich, M. Busek, F. Sonntag, D. A. Sakharov, E. V. Trushkin, A. G. Tonevitsky, R. Lauster and U. Marx, A Dynamic Multi-Organ-Chip for Long-Term Cultivation and Substance Testing Proven by 3D Human Liver and Skin Tissue Co-Culture, Lab Chip, 2013, 13(18), 3538–3547, 10.1039/C3LC50234A.
- I. Maschmeyer, T. Hasenberg, A. Jaenicke, M. Lindner, A. K. Lorenz, J. Zech, L. A. Garbe, F. Sonntag, P. Hayden, S. Ayehunie, R. Lauster, U. Marx and E. M. Materne, Chip-Based Human Liver-Intestine and Liver-Skin Co-Cultures--A First Step toward Systemic Repeated Dose Substance Testing in Vitro, Eur. J. Pharm. Biopharm., 2015, 95(Pt A), 77–87, DOI:10.1016/J.EJPB.2015.03.002.
- R. S. N. Tavares, T. P. Tao, I. Maschmeyer, S. S. Maria-Engler, M. Schäfer-Korting, A. Winter, C. Zoschke, R. Lauster, U. Marx and L. R. Gaspar, Toxicity of Topically Applied Drugs beyond Skin Irritation: Static Skin Model vs. Two Organs-on-a-Chip, Int. J. Pharm., 2020, 589, 119788, DOI:10.1016/j.ijpharm.2020.119788.
- J. Kühnl, T. P. Tao, K. Brandmair, S. Gerlach, T. Rings, U. Müller-Vieira, J. Przibilla, C. Genies, C. Jaques-Jamin, A. Schepky, U. Marx, N. J. Hewitt and I. Maschmeyer, Characterization of Application Scenario-Dependent Pharmacokinetics and Pharmacodynamic Properties of Permethrin and Hyperforin in a Dynamic Skin and Liver Multi-Organ-Chip Model, Toxicology, 2021, 448, 152637, DOI:10.1016/j.tox.2020.152637.
- K. Brandmair, T.-P. Tao, S. Gerlach, J. Przibilla, A. Schepky, U. Marx, N. J. Hewitt, J. Kühnl and I. Maschmeyer, Suitability of Different Reconstructed Human Skin Models in the Skin and Liver Chip2 Microphysiological Model to Investigate the Kinetics and First-Pass Skin Metabolism of the Hair Dye, 4-Amino-2-Hydroxytoluene, J. Appl. Toxicol., 2024, 44(3), 333–343, DOI:10.1002/jat.4542.
- T.-P. Tao, K. Brandmair, S. Gerlach, J. Przibilla, A. Schepky, U. Marx, N. J. Hewitt, I. Maschmeyer and J. Kühnl, Application of a Skin and Liver Chip2 Microphysiological Model to Investigate the Route-Dependent Toxicokinetics and Toxicodynamics of Consumer-Relevant Doses of Genistein, J. Appl. Toxicol., 2024, 44(2), 287–300, DOI:10.1002/jat.4540.
- J. S. Lee, J. Kim, B. Cui, S. K. Kim, S.-A. Cho, S. An and S.-W. Cho, Hybrid Skin Chips for Toxicological Evaluation of Chemical Drugs and Cosmetic Compounds, Lab Chip, 2022, 22(2), 343–353, 10.1039/D1LC00550B.
- K. Schimek, S. Frentzel, K. Luettich, D. Bovard, I. Rütschle, L. Boden, F. Rambo, H. Erfurth, E. M. Dehne, A. Winter, U. Marx and J. Hoeng, Human Multi-Organ Chip Co-Culture of Bronchial Lung Culture and Liver Spheroids for Substance Exposure Studies, Sci. Rep., 2020, 10(1), 1–13, DOI:10.1038/s41598-020-64219-6.
- D. Bovard, A. Sandoz, K. Luettich, S. Frentzel, A. Iskandar, D. Marescotti, K. Trivedi, E. Guedj, Q. Dutertre, M. C. Peitsch and J. Hoeng, A Lung/Liver-on-a-Chip Platform for Acute and Chronic Toxicity Studies, Lab Chip, 2018, 18(24), 3814–3829, 10.1039/C8LC01029C.
- P. G. Miller, C. Y. Chen, Y. I. Wang, E. Gao and M. L. Shuler, Multiorgan Microfluidic Platform with Breathable Lung Chamber for Inhalation or Intravenous Drug Screening and Development, Biotechnol. Bioeng., 2020, 117(2), 486–497, DOI:10.1002/BIT.27188.
- K. Shinha, W. Nihei, T. Ono, R. Nakazato and H. Kimura, A Pharmacokinetic-Pharmacodynamic Model Based on Multi-Organ-on-a-Chip for Drug-Drug Interaction Studies, Biomicrofluidics, 2020, 14(4) DOI:10.1063/5.0011545.
- J. R. Coppeta, M. J. Mescher, B. C. Isenberg, A. J. Spencer, E. S. Kim, A. R. Lever, T. J. Mulhern, R. Prantil-Baun, J. C. Comolli and J. T. Borenstein, A Portable and Reconfigurable Multi-Organ Platform for Drug Development with Onboard Microfluidic Flow Control, Lab Chip, 2016, 17(1), 134–144, 10.1039/C6LC01236A.
- L. Boeri, L. Izzo, L. Sardelli, M. Tunesi, D. Albani and C. Giordano, Advanced Organ-on-a-Chip Devices to Investigate Liver Multi-Organ Communication: Focus on Gut, Microbiota and Brain, Bioengineering, 2019, 6(4), 91, DOI:10.3390/BIOENGINEERING6040091.
- T. Bricks, P. Paullier, A. Legendre, M. J. Fleury, P. Zeller, F. Merlier, P. M. Anton and E. Leclerc, Development of a New Microfluidic Platform Integrating Co-Cultures of Intestinal and Liver Cell Lines, Toxicol. In Vitro, 2014, 28(5), 885–895, DOI:10.1016/J.TIV.2014.02.005.
- J. M. Prot, L. Maciel, T. Bricks, F. Merlier, J. Cotton, P. Paullier, F. Y. Bois and E. Leclerc, First Pass Intestinal and Liver Metabolism of Paracetamol in a Microfluidic Platform Coupled with a Mathematical Modeling as a Means of Evaluating ADME Processes in Humans, Biotechnol. Bioeng., 2014, 111(10), 2027–2040, DOI:10.1002/BIT.25232.
- M. B. Esch, H. Ueno, D. R. Applegate and M. L. Shuler, Modular, Pumpless Body-on-a-Chip Platform for the Co-Culture of GI Tract Epithelium and 3D Primary Liver Tissue, Lab Chip, 2016, 16(14), 2719–2729, 10.1039/C6LC00461J.
- I. Maschmeyer, T. Hasenberg, A. Jaenicke, M. Lindner, A. K. Lorenz, J. Zech, L. A. Garbe, F. Sonntag, P. Hayden, S. Ayehunie, R. Lauster, U. Marx and E. M. Materne, Chip-Based Human Liver-Intestine and Liver-Skin Co-Cultures--A First Step toward Systemic Repeated Dose Substance Testing in Vitro, Eur. J. Pharm. Biopharm., 2015, 95(Pt A), 77–87, DOI:10.1016/J.EJPB.2015.03.002.
- I. Wagner, E.-M. Materne, S. Brincker, U. Süßbier, C. Frädrich, M. Busek, F. Sonntag, D. A. Sakharov, E. V. Trushkin, A. G. Tonevitsky, R. Lauster and U. Marx, A Dynamic Multi-Organ-Chip for Long-Term Cultivation and Substance Testing Proven by 3D Human Liver and Skin Tissue Co-Culture, Lab Chip, 2013, 13(18), 3538–3547, 10.1039/C3LC50234A.
- J. Hübner, M. Raschke, I. Rütschle, S. Gräßle, T. Hasenberg, K. Schirrmann, A. Lorenz, S. Schnurre, R. Lauster, I. Maschmeyer, T. Steger-Hartmann and U. Marx, Simultaneous Evaluation of Anti-EGFR-Induced Tumour and Adverse Skin Effects in a Microfluidic Human 3D Co-Culture Model, Sci. Rep., 2018, 8(1), 15010, DOI:10.1038/s41598-018-33462-3.
- J. Theobald, M. A. Abu el Maaty, N. Kusterer, B. Wetterauer, M. Wink, X. Cheng and S. Wölfl, In Vitro Metabolic Activation of Vitamin D3 by Using a Multi-Compartment Microfluidic Liver-Kidney Organ on Chip Platform, Sci. Rep., 2019, 9(1), 1–11, DOI:10.1038/s41598-019-40851-9.
- Q. Huang, T. Yang, Y. Song, W. Sun, J. Xu, Y. Cheng, R. Yin, L. Zhu, M. Zhang, L. Ma, H. Li and H. Zhang, A Three-Dimensional(3D) Liver–Kidney on a Chip with a Biomimicking Circulating System for Drug Safety Evaluation, Lab Chip, 2024, 24(6), 1715–1726, 10.1039/D3LC00980G.
- P. Mamoshina, B. Rodriguez and A. Bueno-Orovio, Toward a Broader View of Mechanisms of Drug Cardiotoxicity, Cell Rep. Med., 2021, 2(3), 100216, DOI:10.1016/J.XCRM.2021.100216.
- Y. Baert, I. Ruetschle, W. Cools, A. Oehme, A. Lorenz, U. Marx, E. Goossens and I. Maschmeyer, A Multi-Organ-Chip Co-Culture of Liver and Testis Equivalents: A First Step toward a Systemic Male Reprotoxicity Model, Hum. Reprod., 2020, 35(5), 1029–1044, DOI:10.1093/HUMREP/DEAA057.
- C. Oleaga, A. Riu, S. Rothemund, A. Lavado, C. W. McAleer, C. J. Long, K. Persaud, N. S. Narasimhan, M. Tran, J. Roles, C. A. Carmona-Moran, T. Sasserath, D. H. Elbrecht, L. Kumanchik, L. R. Bridges, C. Martin, M. T. Schnepper, G. Ekman, M. Jackson, Y. I. Wang, R. Note, J. Langer, S. Teissier and J. J. Hickman, Investigation of the Effect of Hepatic Metabolism on Off-Target Cardiotoxicity in a Multi-Organ Human-on-a-Chip System, Biomaterials, 2018, 182, 176–190, DOI:10.1016/J.BIOMATERIALS.2018.07.062.
- C. P. P. de Mello, C. Carmona-Moran, C. W. McAleer, J. Perez, E. A. Coln, C. J. Long, C. Oleaga, A. Riu, R. Note, S. Teissier, J. Langer and J. J. Hickman, Microphysiological Heart–Liver Body-on-a-Chip System with a Skin Mimic for Evaluating Topical Drug Delivery, Lab Chip, 2020, 20(4), 749–759, 10.1039/C9LC00861F.
- F. Yin, X. Zhang, L. Wang, Y. Wang, Y. Zhu, Z. Li, T. Tao, W. Chen, H. Yu and J. Qin, HiPSC-Derived Multi-Organoids-on-Chip System for Safety Assessment of Antidepressant Drugs, Lab Chip, 2021, 21(3), 571–581, 10.1039/D0LC00921K.
- A. Skardal, S. V. Murphy, M. Devarasetty, I. Mead, H. W. Kang, Y. J. Seol, Y. S. Zhang, S. R. Shin, L. Zhao, J. Aleman, A. R. Hall, T. D. Shupe, A. Kleensang, M. R. Dokmeci, S. Jin Lee, J. D. Jackson, J. J. Yoo, T. Hartung, A. Khademhosseini, S. Soker, C. E. Bishop and A. Atala, Multi-Tissue Interactions in an Integrated Three-Tissue Organ-on-a-Chip Platform, Sci. Rep., 2017, 7(1), 1–16, DOI:10.1038/s41598-017-08879-x.
- K. Ronaldson-Bouchard, D. Teles, K. Yeager, D. N. Tavakol, Y. Zhao, A. Chramiec, S. Tagore, M. Summers, S. Stylianos, M. Tamargo, B. M. Lee, S. P. Halligan, E. H. Abaci, Z. Guo, J. Jacków, A. Pappalardo, J. Shih, R. K. Soni, S. Sonar, C. German, A. M. Christiano, A. Califano, K. K. Hirschi, C. S. Chen, A. Przekwas and G. Vunjak-Novakovic, A Multi-Organ Chip with Matured Tissue Niches Linked by Vascular Flow, Nat. Biomed. Eng., 2022, 6(4), 351–371, DOI:10.1038/s41551-022-00882-6.
- A. Herland, B. M. Maoz, D. Das, M. R. Somayaji, R. Prantil-Baun, R. Novak, M. Cronce, T. Huffstater, S. S. F. Jeanty, M. Ingram, A. Chalkiadaki, D. Benson Chou, S. Marquez, A. Delahanty, S. Jalili-Firoozinezhad, Y. Milton, A. Sontheimer-Phelps, B. Swenor, O. Levy, K. K. Parker, A. Przekwas and D. E. Ingber, Quantitative Prediction of Human Pharmacokinetic Responses to Drugs via Fluidically Coupled Vascularized Organ Chips, Nat. Biomed. Eng., 2020, 4(4), 421–436, DOI:10.1038/s41551-019-0498-9.
- C. W. McAleer, C. J. Long, D. Elbrecht, T. Sasserath, L. R. Bridges, J. W. Rumsey, C. Martin, M. Schnepper, Y. Wang, F. Schuler, A. B. Roth, C. Funk, M. L. Shuler and J. J. Hickman, Multi-Organ System for the Evaluation of Efficacy and off-Target Toxicity of Anticancer Therapeutics, Sci. Transl. Med., 2019, 11(497), eaav1386, DOI:10.1126/SCITRANSLMED.AAV1386.
- C. Oleaga, C. Bernabini, A. S. T. Smith, B. Srinivasan, M. Jackson, W. Mclamb, V. Platt, R. Bridges, Y. Cai, N. Santhanam, B. Berry, S. Najjar, N. Akanda, X. Guo, C. Martin, G. Ekman, M. B. Esch, J. Langer, G. Ouedraogo, J. Cotovio, L. Breton, M. L. Shuler and J. J. Hickman, Multi-Organ Toxicity Demonstration in a Functional Human in Vitro System Composed of Four Organs, Sci. Rep., 2015, 6(1), 20030, DOI:10.1038/srep20030.
- T. Satoh, S. Sugiura, K. Shin, R. Onuki-Nagasaki, S. Ishida, K. Kikuchi, M. Kakiki and T. Kanamori, A Multi-Throughput Multi-Organ-on-a-Chip System on a Plate Formatted Pneumatic Pressure-Driven Medium Circulation Platform, Lab Chip, 2017, 18(1), 115–125, 10.1039/C7LC00952F.
- C. D. Edington, W. L. K. Chen, E. Geishecker, T. Kassis, L. R. Soenksen, B. M. Bhushan, D. Freake, J. Kirschner, C. Maass, N. Tsamandouras, J. Valdez, C. D. Cook, T. Parent, S. Snyder, J. Yu, E. Suter, M. Shockley, J. Velazquez, J. J. Velazquez, L. Stockdale, J. P. Papps, I. Lee, N. Vann, M. Gamboa, M. E. Labarge, Z. Zhong, X. Wang, L. A. Boyer, D. A. Lauffenburger, R. L. Carrier, C. Communal, S. R. Tannenbaum, C. L. Stokes, D. J. Hughes, G. Rohatgi, D. L. Trumper and M. Cirit, Griffith, L. G. Interconnected Microphysiological Systems for Quantitative Biology and Pharmacology Studies, Sci. Rep., 2018, 8(1), 1–18, DOI:10.1038/s41598-018-22749-0.
- Q. Ramadan, R. S. Fardous, R. Hazaymeh, S. Alshmmari and M. Zourob, Pharmacokinetics-On-a-Chip: In Vitro Microphysiological Models for Emulating of Drugs ADME, Adv. Biol., 2021, 5(9), 2100775, DOI:10.1002/ADBI.202100775.
- S. Fowler, W. L. K. Chen, D. B. Duignan, A. Gupta, N. Hariparsad, J. R. Kenny, W. G. Lai, J. Liras, J. A. Phillips and J. Gan, Microphysiological Systems for ADME-Related Applications: Current Status and Recommendations for System Development and Characterization, Lab Chip, 2020, 20(3), 446–467, 10.1039/C9LC00857H.
- C. Ma, Y. Peng, H. Li and W. Chen, Organ-on-a-Chip: A New Paradigm for Drug Development, Trends Pharmacol. Sci., 2021, 42(2), 119–133, DOI:10.1016/J.TIPS.2020.11.009.
- J. Wu, H. Fang, J. Zhang and S. Yan, Modular Microfluidics for Life Sciences, J. Nanobiotechnol., 2023, 21(1), 85, DOI:10.1186/S12951-023-01846-X.
- X. Lai, M. Yang, H. Wu and D. Li, Modular Microfluidics: Current Status and Future Prospects, Micromachines, 2022, 13(8), 1363, DOI:10.3390/MI13081363.
- L. J. Y. Ong, T. Ching, L. H. Chong, S. Arora, H. Li, M. Hashimoto, R. Dasgupta, P. K. Yuen and Y. C. Toh, Self-Aligning Tetris-Like (TILE) Modular Microfluidic Platform for Mimicking Multi-Organ Interactions, Lab Chip, 2019, 19(13), 2178–2191, 10.1039/C9LC00160C.
- D. J. Carvalho, A. M. Kip, A. Tegel, M. Stich, C. Krause, M. Romitti, C. Branca, B. Verhoeven, S. Costagliola, L. Moroni and S. Giselbrecht, A Modular Microfluidic Organoid Platform Using LEGO-Like Bricks, Adv. Healthcare Mater., 2024, 13(13), 2303444, DOI:10.1002/ADHM.202303444.
- I. Koh and M. Hagiwara, Modular Tissue-in-a-CUBE Platform to Model Blood-Brain Barrier (BBB) and Brain Interaction, Commun. Biol., 2024, 7(1), 1–13, DOI:10.1038/s42003-024-05857-8.
- Q. Sun, J. Pei, Q. Li, K. Niu and X. Wang, Reusable Standardized Universal Interface Module (RSUIM) for Generic Organ-on-a-Chip Applications, Micromachines, 2019, 10(12), 849, DOI:10.3390/MI10120849.
- Y. Hou, X. Ai, L. Zhao, Z. Gao, Y. Wang, Y. Lu, P. Tu and Y. Jiang, An Integrated Biomimetic Array Chip for High-Throughput Co-Culture of Liver and Tumor Microtissues for Advanced Anticancer Bioactivity Screening, Lab Chip, 2020, 20(14), 2482–2494, 10.1039/D0LC00288G.
- R. Novak, M. Ingram, S. Marquez, D. Das, A. Delahanty, A. Herland, B. M. Maoz, S. S. F. Jeanty, M. R. Somayaji, M. Burt, E. Calamari, A. Chalkiadaki, A. Cho, Y. Choe, D. B. Chou, M. Cronce, S. Dauth, T. Divic, J. Fernandez-Alcon, T. Ferrante, J. Ferrier, E. A. FitzGerald, R. Fleming, S. Jalili-Firoozinezhad, T. Grevesse, J. A. Goss, T. Hamkins-Indik, O. Henry, C. Hinojosa, T. Huffstater, K. J. Jang, V. Kujala, L. Leng, R. Mannix, Y. Milton, J. Nawroth, B. A. Nestor, C. F. Ng, B. O'Connor, T. E. Park, H. Sanchez, J. Sliz, A. Sontheimer-Phelps, B. Swenor, G. Thompson, G. J. Touloumes, Z. Tranchemontagne, N. Wen, M. Yadid, A. Bahinski, G. A. Hamilton, D. Levner, O. Levy, A. Przekwas, R. Prantil-Baun, K. K. Parker and D. E. Ingber, Robotic Fluidic Coupling and Interrogation of Multiple Vascularized Organ Chips, Nat. Biomed. Eng., 2020, 4(4), 407–420, DOI:10.1038/s41551-019-0497-x.
- H. Azizgolshani, J. R. Coppeta, E. M. Vedula, E. E. Marr, B. P. Cain, R. J. Luu, M. P. Lech, S. H. Kann, T. J. Mulhern, V. Tandon, K. Tan, N. J. Haroutunian, P. Keegan, M. Rogers, A. L. Gard, K. B. Baldwin, J. C. de Souza, B. C. Hoefler, S. S. Bale, L. B. Kratchman, A. Zorn, A. Patterson, E. S. Kim, T. A. Petrie, E. L. Wiellette, C. Williams, B. C. Isenberg and J. L. Charest, High-Throughput Organ-on-Chip Platform with Integrated Programmable Fluid Flow and Real-Time Sensing for Complex Tissue Models in Drug Development Workflows, Lab Chip, 2021, 21(8), 1454–1474, 10.1039/D1LC00067E.
- M. T. Rogers, A. L. Gard, R. Gaibler, T. J. Mulhern, R. Strelnikov, H. Azizgolshani, B. P. Cain, B. C. Isenberg, N. J. Haroutunian, N. E. Raustad, P. M. Keegan, M. P. Lech, L. Tomlinson, J. T. Borenstein, J. L. Charest and C. Williams, A High-Throughput Microfluidic Bilayer Co-Culture Platform to Study Endothelial-Pericyte Interactions, Sci. Rep., 2021, 11(1), 1–14, DOI:10.1038/s41598-021-90833-z.
Footnote |
† Equally contributing authors. |
|
This journal is © The Royal Society of Chemistry 2025 |
Click here to see how this site uses Cookies. View our privacy policy here.