DOI:
10.1039/D4MA00896K
(Review Article)
Mater. Adv., 2025,
6, 1578-1607
Therapeutic systems based on natural gut microbiota modulators: the latest advances in the treatment of inflammatory bowel disease
Received
5th September 2024
, Accepted 23rd January 2025
First published on 29th January 2025
Abstract
The gut microbiota plays an indispensable role in maintaining gut health. However, the imbalance of gut microbiota under inflammatory conditions is closely related to the acceleration of inflammatory bowel disease (IBD) progression. Consequently, modulating the gut microbiota is one of the crucial strategies for treating IBD. Naturally sourced phytonutrients, probiotics, prebiotics, gut microbiota metabolites and extracellular vesicles (EVs) exhibit remarkable gut microbiota regulation capabilities through diverse pathways, for instance, exerting anti-inflammatory and antioxidant activities, competing with harmful bacteria for nutrients, selectively promoting the proliferation of beneficial bacteria, regulating immune homeostasis, and so on. Since they are all derived from nature and exhibit excellent gut microbiota modulation capabilities, we define them as “natural gut microbiota modulators” (NGMMs). In recent years, the thriving development of therapeutic systems has illuminated the direction for the advancement of NGMMs. Constructing therapeutic systems based on MGMMs can overcome the challenges they face in treating IBD and significantly enhance their therapeutic efficacy. In this review, We first reviewed the role of gut microbiota in IBD, and then systematically summarized how the therapeutic system based on NGMMs positively influences the intestinal microbiota. Furthermore, we sort out the challenges faced by these therapeutic systems and offer insights into their prospects.
1 Introduction
Inflammatory bowel disease (IBD) is a chronic, non-specific inflammatory disease that includes Crohn's disease (CD) and ulcerative colitis (UC).1 Approximately 7 million people globally are affected by IBD, and over the past three decades, the incidence of IBD has soared in low-income and middle-income countries.2 Clinical symptoms of IBD include abdominal pain, diarrhoea, blood in the stool, and weight loss.3 The pathogenesis of IBD is intricate, involving multiple factors such as genetic factors, environmental factors (including air pollution, geography, etc.), lifestyle factors (for example, smoking and diet), and gut microbiota imbalance.4 The fundamental goal of IBD treatment is to restore intestinal epithelial tissue and reshape intestinal homeostasis. At present, the clinical treatment drugs for IBD primarily consist of aminosalicylates, corticosteroids, immunosuppressants, and so on. These drugs can alleviate inflammation, but their efficacy in restoring the tissue and structure of the intestinal epithelium is limited, and long-term use often results in significant side effects.5,6 Therefore, it is necessary to explore more effective treatment strategies.
The gut microbiota encompasses a complex microbial community, including approximately 160 major bacterial species alongside fungi, parasites, viruses, archaea, and protozoa, all coexisting with the host in an intricate yet stable ecological balance.7–9 In physiological conditions, the gut microbiota serves as an integral part of the intestinal ecosystem, engaging in a mutually beneficial and symbiotic relationship with the host, and extensively participating in various life activities.10 However, under inflammatory conditions, the gut microbiota exhibits a state of dysbiosis, characterized by changes in its composition and metabolic disorders, leading to the impairment of its normal functions and exacerbating the progression of inflammation.11 For example, under the intestinal inflammation condition, the decreased abundance of Faecalibacterium, Phascolarctobacterium, and Clostridia clades IV and XIVa impairs their ability to produce butyrate, utilize hydrogen, and exert other anti-inflammatory properties.12 In addition, due to the disruption of the intestinal mucosal barrier caused by IBD, Clostridium difficile is more likely to colonize and overgrow in the intestine, producing protein toxins that are associated with the destruction of tight junction proteins (TJs), epithelial cell death, and mucosal inflammation.13,14 Gut microbiota dysbiosis is a crucial factor contributing to the disruption of intestinal homeostasis and intestinal epithelial tissue damage. Modulating the gut microbiota is considered a potential target for the treatment of IBD.15
Naturally sourced phytonutrients,16 probiotics,17 prebiotics,11 gut microbiota metabolites,18 and extracellular vesicles (EVs)19 demonstrate remarkable abilities to regulate gut microbiota through various pathways. Phytonutrients can exert anti-inflammatory and antioxidant activities to regulate the intestinal microenvironment, thereby restoring the homeostasis of gut microbiota.20 Supplementing probiotics can inhibit the growth and reproduction of harmful bacteria by competing with them for nutrients, thereby improving the gut microbiota.21 Prebiotics can selectively promote the proliferation of beneficial bacteria in the gut to regulate the gut microbiota.22 Gut microbiota metabolites can function as signaling molecules that impact intestinal immune homeostasis and epithelial barrier function, subsequently leading to the improvement of the gut microbiota.23 EVs can restore the homeostasis of the intestinal microenvironment by directly conducting information transfer with the host's intestinal flora.24 Since phytonutrients, probiotics, prebiotics, gut microbiota metabolites, and EVs all originate from nature and demonstrate remarkable abilities in regulating the gut microbiota, we define them as “natural gut microbiota modulators” (NGMMs), which, due to their wide range of sources and ease of access, confer unique advantages in the treatment of IBD.
However, there are still some issues that arise when using NGMMs to modulate the gut microbiota. The efficacy of phytonutrients is mainly limited by their stability and solubility.25 For probiotics, the complex digestive tract environment and long transit time can affect their survival rate and colonization rate, thereby impacting their efficacy.26 Some prebiotics, due to their rapid fermentation characteristics, may lead to a shortage of fermentable carbohydrates for bacteria in the distal colon.27 Some gut microbiota metabolites have limited efficacy due to their rapid metabolism and excretion in the body.28 Hence, how to solve these problems to make them better play the regulatory role of intestinal flora has become the focus of our attention. The vigorous development of therapeutic systems offers possible solutions.29,30 Modifying or encapsulating drug molecules with chemical or biological materials of different properties to construct therapeutic systems can enhance their bioavailability and ensure better therapeutic efficacy.31–33 From this perspective, they can all be encapsulated and modified to construct therapeutic systems that enable them to better exert their therapeutic effects. In this review, from the perspective of natural sources, we summarize how therapeutic systems based on NGMMs with excellent gut microbiota modulation capabilities address the challenges and limitations they face in practical applications. Furthermore, some therapies related to NGMMs, such as fecal microbiota transplantation (FMT) and synbiotics, have also been summarized. Finally, we will separately summarize and elaborate on the current challenges and prospects of these therapeutic systems.
2 Gut microbiota and IBD
The human gut is home to many microorganisms, which are more than 10 times more numerous than the total number of human cells.34 And the number of bacteria accounts for more than 90% of the intestinal microorganisms. The gut provides a rich nutrient environment for bacteria to grow, so nearly 100 trillion species of bacteria can exist and colonize the gastrointestinal tract of the human body. Healthy intestinal bacteria consist mainly of Firmicutes, Bacteroidetes, Actinobacteria, and Proteobacteria, in which Firmicutes are dominant (50–75%), followed by Bacteroidetes (10–50%), Actinobacteria (1–10%), and Proteobacteria (usually less than 1%). The gut microbiota is mainly composed of these four phyla and is in dynamic equilibrium under healthy conditions, but the gut microbiota exhibits temporal and spatial differences at the genus level and even more subdivided species levels. From the esophagus distally to the rectum, the number of bacteria varies significantly, with about 101 bacteria per gram of content in the esophagus and stomach, and about 1012 bacteria per gram of content in the rectum.35 These gut microbiota can play a significant role in various physiological processes within the host, including substance metabolism, the maintenance of immune homeostasis, and endocrine regulation, thereby influencing the overall gut health of the host.36 The gut microbiota can break down and ferment certain food components that are difficult for the human body to digest, such as cellulose and some complex carbohydrates, converting them into short-chain fatty acids (SCFAs). This not only provides nutrition for intestinal cells, maintains the integrity of the intestinal mucosa, but also contributes to overall energy metabolism.37 The homeostasis of the immune system is crucial for intestinal health. Metabolites derived from gut microbiota influence excessive immune cell responses, including those of T cells, B cells, dendritic cells, and macrophages, to maintain immune homeostasis.38 Furthermore, in situations where nutrients are insufficient, the gut microbiota can compete with pathogenic bacteria for nutrients, thereby restricting the growth and reproduction of the latter and maintaining intestinal health.39 In return, the gut microbiota can obtain the necessary nutrients from the host to sustain their growth and reproduction. Under normal physiological conditions, the host and gut microbiota form a mutually influencing and interdependent relationship.
However, under pathological conditions, the interdependent relationship between the gut microbiota and the host is disrupted, leading to an unhealthy symbiotic state. Extensive tissue inflammation of the intestinal epithelium leads to the loss of the basis for gut microbiota colonization in the intestine, resulting in a dramatic decrease in gut microbiota, and a significant reduction in diversity.40 The dysregulation of gut microbiota is closely related to the occurrence of IBD. Fig. 1 summarizes the gut microbiota associated with the development and progression of IBD, as well as the related molecular mechanisms.41 Due to the decreased abundance of the intestinal symbiotic flora, the loss of competition and antagonism against pathogenic bacteria provides an opportunity for the colonization of pathogens, leading to an overall shift in the gut microbiota characterized by a reduction in beneficial bacteria and an increase in harmful bacteria.42Fusobacterium mainly resides in the oral cavity and intestine. Compared with the healthy control group, the abundance of Fusobacterium in the colonic mucosa of UC patients is higher. Fusobacterium varium can cause colonic mucosal inflammation, exacerbating IBD.43 Furthermore, due to the disruption of redox homeostasis in an inflammatory state, there is a surge in ROS/RNS levels. ROS/RNS provides terminal electron acceptors for anaerobic respiration, leading to a significant proliferation of facultative anaerobes and a decrease in the abundance of obligate anaerobes.44E. coli is a Gram-negative, facultative anaerobic bacterium. Evidence shows that the abundance of Adherent-invasive E. coli (AIEC) strains increases in the inflammatory state, and AIEC can adhere to and penetrate the intestinal mucosa of patients with IBD, thereby inducing inflammation and increasing the permeability of intestinal epithelium.45 The decrease in the abundance of beneficial bacteria and the increase in harmful bacteria within the gut microbiota can lead to metabolic disorders, which are manifested primarily by the reduction of beneficial metabolites and the elevation of harmful metabolites.46,47 The overall level of SCFAs in the intestines of patients with IBD is significantly reduced, mainly attributed to the reduced number of bacteria that produce SCFAs, including Faecalibacterium prausnitzii, Bifidobacterium adolescentis, and Dialister invisus.18,48 Intestinal microbiota can metabolize tryptophan into indole compounds, which are ligands for the aryl hydrocarbon receptor (AhR). These indole metabolites promote the maturation of Treg cells by activating the AhR, thereby maintaining immune homeostasis. Imbalances in intestinal microbiota metabolism can directly lead to a reduction in the production of indole metabolites, disrupting intestinal immune homeostasis and further exacerbating inflammation.49 For example, Clostridium sporogenes not only metabolize Trp to produce indole-3-acetic acid (IAA) and indole-3-propionic acid (IPA), but they also possess the capability to decarboxylate Trp, resulting in the production of the neurotransmitter serotonin.50 However, as a symbiotic bacterium, the abundance of Clostridium sporogenes can be affected by inflammatory states. On the contrary, some “harmful” gut microbiota metabolites, particularly niacin, taurine, and acylcarnitines, are more abundant in patients with IBD.48 And taurine has been identified as an activator of mucosal inflammasomes, capable of exacerbating the progression of inflammation.51 In conclusion, the disruption of gut microbiota is closely related to the development of IBD. Modulating the gut microbiota not only competitively inhibits harmful bacteria by increasing the abundance of beneficial bacteria, thereby reducing the levels of harmful products and inhibiting the spread of inflammation, but also restores the normal function of the gut microbiota to promote the repair of the intestinal epithelial barrier, and maintain immune homeostasis. Therefore, modulating the gut microbiota to restore its homeostasis holds significant importance for the treatment of IBD.
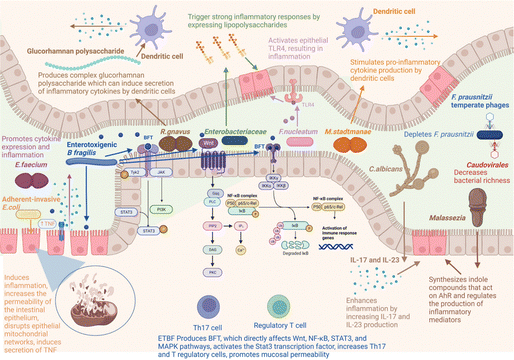 |
| Fig. 1 Microbes involved in inflammatory bowel disease and their molecular mechanisms. B. fragilis, Bacteroides fragilis; E. faecium, Enterococcus faecium; E. coli, Escherichia coli; F. nucleatum, Fusobacterium nucleatum; F. prausnitzii, Faecalibacterium prausnitzii; M. stadtmanae, Methanosphaera stadtmanae; R. gnavus, Ruminococcus gnavus; TLR4, Toll-like receptor 4; BFT, B. fragilis toxin; TNF, tumor necrosis factor; ETBF, enterotoxigenic B. fragilis; AhR, aryl hydrocarbon receptor; IL, interleukin; PLC, phospholipase C; DAG, diacylglycerol; PKC, protein kinase C. Reproduced from reference [H. Pandey, D. Jain, D. W. T. Tang, S. H. Wong and D. Lal, Gut microbiota in pathophysiology, diagnosis, and therapeutics of inflammatory bowel disease, Intest. Res., 2024, 22(1), 15–43] with permission from [Korean Association for the Study of Intestinal Diseases], copyright [2024]. | |
3 Therapeutic systems based on NGMMs
With the continuous exploration of researchers, the NGMMs are found to play a significant role in regulating gut microbiota, and their wide range of sources and ease of access make them promising candidates for the treatment of IBD.16,52,53 Additionally, many articles have reported that therapeutic systems based on NGMMs are capable of addressing challenges encountered in their applications, thereby enhancing their remarkable abilities to regulate gut microbiota. For example, therapeutic systems with gastric acid resistance can carry phytonutrients through the harsh gastric acid environment and release them precisely at the site of colonic inflammation, thereby improving their bioavailability. Probiotics modified with encapsulation materials or through genetic editing techniques can enhance their adhesion to the intestinal wall, thereby improving their survival and colonization rates within the gut. Therapeutic systems with sustained-release functions can carry prebiotics to extend their fermentation time at the site of colonic inflammation. Therapeutic systems with controlled-release functions can carry indole derivatives to prevent their premature absorption in the small intestine. Extracellular vesicles themselves constitute a therapeutic system containing multiple components, capable of providing nutrients to intestinal microbiota to promote their growth and reproduction. In Fig. 2, We summarized the therapeutic systems based on NGMMs and in this section, we will delve into the specifics of NGMMs and the therapeutic systems based on them, how function to modulate gut microbiota for the treatment of IBD.
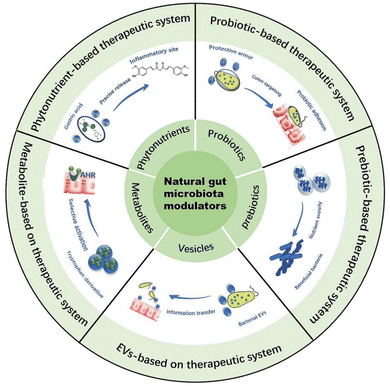 |
| Fig. 2 The therapeutic systems based on natural gut microbiota modulators for IBD treatment. EVs: extracellular vesicles; metabolites: gut microbiota metabolites; the phytonutrients-based therapeutic system enhances their bioavailability and achieves responsive release at sites of intestinal inflammation. The probiotic-based therapeutic system provides armor for probiotics to overcome the gastric acid environment, thereby improving their survival and colonization rates. The prebiotic-based therapeutic system selectively supplies nutrients to beneficial bacteria to promote their proliferation. The extracellular vesicles (EVs)-based therapeutic system facilitates information transfer between the host and gut microbiota to regulate the homeostasis of the gut flora. The gut microbiota metabolites-based therapeutic system selectively activates relevant immune pathways to maintain immune homeostasis. | |
3.1 Phytonutrient-based therapeutic systems
Phytonutrients, also known as nutraceutical plant chemicals, are secondary metabolites accumulated in different parts of plants.16 Based on their unique properties and different structures, phytonutrients are classified into phenolic acids, flavonoids, carotenoids, tocopherols, curcuminoids, and so on.54 Due to their excellent anti-inflammatory,55 antioxidant,56 and immune-modulating activities,57 significant progress has been made in the treatment of IBD by using phytonutrients.58 For example, epigallocatechin-3-gallate (EGCG), which is abundant in tea, can treat IBD by stabilizing mast cells and inhibiting pro-inflammatory cytokines such as IL-6, monocyte chemoattractant protein-1 (MCP-1), and TNF-α through the NF-κB pathway.59,60 With the continuous deepening of research on the treatment of IBD using phytonutrients, many researchers have shifted their focus to the gut microbiota regulating the activity of phytonutrients. In Table 1, we have summarized some phytonutrients that can regulate the gut microbiota. For example, chlorogenic acid (CGA), a common phenolic acid found in coffee and tea, exhibits excellent therapeutic effects on IBD. CGA is capable of reversing the decrease in intestinal microbial diversity caused by dextran sulfate sodium (DSS) and also increasing the relative abundance of Lactobacillus.61 However, the therapeutic effects of phytonutrients are often affected by their stability and bioavailability.62 How to improve the bioavailability and stability of phytonutrients to better exert their gut microbiota-modulating capabilities has become our focus of attention. Based on this, many therapeutic systems have been developed to deliver phytonutrients to the intestinal inflammation site to better exert their therapeutic effects. Next, therapeutic systems will be categorized based on the structures of various phytonutrients, with an elaboration on how they exert their gut microbiota modulating effects.
Table 1 Phytonutrients with the ability to regulate gut microbiota
Category |
Name |
Alterations of gut microbiota |
Ref. |
Bacteria with increased abundance |
Bacteria with decreased abundance |
Phenolic acids |
Chlorogenic acid |
Ruminococcaceae, Lactobacillaceae, Bacteroidaceaee, Erysipelotrichaceae ↑ |
Firmicutes: Bacteroides, Desulfovibrionaceae ↓ |
63
|
Protocatechuic acid |
Bifidobacterium, Olsenella, Rikenella, Turicibacter, Clostridium_sensu_stricto ↑ |
Lactococcus, Enterorhabdus ↓ |
64
|
Caffeic acid |
Bifidobacterium, Actinobacteria ↑ |
Firmicutes: Bacteroides, Romboutsia, Lactobacillus, Clostridium_sensu_stricto 1 ↓ |
65
|
p-Coumaric acid |
Candidatus saccharimonas, Clostridium sensu stricto, Leuconostoc, Gastranaerophilales↑ |
Morganella, Holdemanella, Fusicatenibacter, Serratia↓ |
66
|
Flavonoids |
Quercetin |
Bacteroides, Bifidobacterium, Lactobacillus, Clostridia ↑ |
Fusobacterium, Enterococcusin↓ |
67
|
Tangeretin |
Lachnospiraceae, Lactobacillaceae ↑ |
Enterobacteriaceae, Alistipes↓ |
68
|
Rutin |
Verrucomicrobia, Roseburia, Bacteroides, Alistipes, Parasutterella, Akkermansia ↑ |
Lachnospiraceae, Ruminococcaceae, Erysipelotrichaceae↓ |
69
|
Luteolin |
Proteobacteria, Lactobacillus, Bifidobacterium, Desulfovibrio ↑ |
Firmicutes to Bacteroides, Actinobacteria↓ |
70
|
Carotenoids |
β-Carotene |
Firmicutes, Actinobacteria, Faecalibacterium, Candidatus stoquefichus, Akkermansia ↑ |
Bacteroidetes, Proteobacteria↓ |
71 and 72
|
Lycopene |
Firmicutes, Actinobacteria, Lactobacillus, Clostridiales, Bifidobacterium, Lachnospiraceae ↑ |
Proteobacteria, Mucispirillum↓ |
73
|
Astaxanthin |
Lactobacillus, Bifidobacteria ↑ |
Enterobacteriaceae spp., Clostridium coccoides ↓ |
74
|
Fucoxanthin |
Lachnospiraceae ↑ |
Bacteroidales, Rikenellaceae↓ |
75
|
Tocopherols |
α-Tocopherol |
Bacteroides acidifaciens, Roseburia ↑ |
Parabacteroides goldsteinii, CL02T12C30↓ |
76
|
γ-Tocopherol |
Bifidobacterium, Roseburia ↑ |
Candidatus Saccharimonas↓ |
77
|
Curcuminoids |
Curcumin |
Bacteroidetes, Muribaculaceae ↑ |
Deinococcus-Thermus, Bacteroides, Ruminococcaceae ↓ |
78
|
3.1.1 Phenolic acids.
Phenolic acids are compounds that contain a large number of phenol rings and carboxyl groups. Dietary phenolic acids have been proven to improve intestinal function by reducing intestinal inflammation and altering gut microbiota.61 Ferulic acid (4-hydroxy-3-methoxycinnamic acid; FA) possesses excellent anti-inflammatory and antioxidant activities and is widely found in symbionts, grasses, grains, fruits, and other sources.79,80 As a hydrophobic small molecule drug, ferulic acid exhibits low bioavailability during delivery through the gastrointestinal tract. Chengke Zhao et al. synthesized lignin-like polymers (FAL) through the free radical polymerization of FA subsequently, the FAL underwent self-assembly to form FAL nanoparticles (FAL NPs). Since the inherent weakly acidic groups in FA remain stable under strongly acidic conditions and can dissociate under alkaline conditions, the pH sensitivity of FAL NPs enables them to withstand the highly acidic gastric environment (pH 0.9–3.5) and ensures precise release of FA at intestinal inflammatory sites, significantly enhancing its bioavailability. After oral administration, FAL NPs can effectively scavenge ROS to reduce intestinal mucosa damage induced by oxidative stress, weaken local immune responses, and ultimately alleviate inflammation. Furthermore, during this process, the gut microbiota is also improved. The Enterobacteriaceae can lead to a reduction in bacterial diversity and enhanced expression of pro-inflammatory cytokines, such as TNF-α. Lactobacillus is a probiotic associated with regulating inflammatory cytokines and inducing regulatory T cells. FALNPs significantly reduced the abundance of Enterobacteriaceae and increased the number of Lactobacillus, thereby promoting the recovery of colonic inflammation.81
3.1.2 Flavonoids.
The flavonoids are a class of 2-phenylchromene compounds that possess a C6–C3–C6 basic carbon skeleton.82 Quercetin (QCT) is a common flavonoid found in fruits, vegetables, and red onions, existing in the forms of glycosides and aglycones. It can reduce inflammation and pyroptosis induced by LPS through the TLR4/NF-κB/NLRP3 pathway.83,84 However, the poor chemical stability of quercetin leads to its low bioavailability. Lechen Wang et al. encapsulated QCT within N-succinyl chitosan (NSC) by crosslinking it with sodium alginate (SA) to construct QCT nanoparticles (QCT NPs). The in vitro release experiment demonstrated that QCT NPs exhibited the highest cumulative release rate in a pH 7.4 medium, with a release amount reaching 77%, whereas in a pH 1.2 medium, the release rate was the lowest, with a release amount only reaching 24%. The carboxyl groups of sodium alginate ensure that the QCT NPs can withstand the acidic environment of the stomach, contracting at low pH and dissolving at higher pH, enabling them to reach the site of intestinal inflammation and release QCT in the weakly alkaline environment which effectively enhances the bioavailability of QCT and significantly improves its therapeutic effects. QCT NPs are capable of reducing the expression of inflammatory cytokines in mice with colitis, alleviating colonic inflammatory infiltration, increasing goblet cell density and mucin proteins, and effectively restoring intestinal epithelial barrier (IEB). Akkermansia muciniphila is a Gram-negative anaerobic mucus-layer-degrading bacterium and its metabolites have a positive regulatory effect on the intestinal immune system.85 After the administration of QCT NPs, the relative abundance of Akkermansia and Lactobacillus increased significantly. Moreover, the levels of short-chain fatty acids were significantly increased after QCT NPs treatment.86
3.1.3 Carotenoids.
Carotenoids are a class of natural liposoluble pigments that possess an isoprenoid-like structure.87 Astaxanthin (AST) as a type of carotenoid, has attracted much attention due to its great potential in the treatment of IBD. However, AST is a highly unsaturated molecule, which leads to its tendency to degrade when exposed to acidic pH, certain enzymes, oxygen, and light, limiting its therapeutic effects.88 Xiumin Zhang et al. modified triphenylphosphine (TPP) with cysteine (Cys) and then assembled it into an intelligent polymeric nanocarrier through an EGCG-mediated Mannich reaction (EGCG-Cys-TPP) for the efficient delivery of AST. EGCG-Cys-TPP can protect the structural stability of astaxanthin during gastrointestinal transport, and after reaching the inflammatory site, the responsiveness of Cys to GSH can ensure the release of AXT. In vitro studies have demonstrated that AXT@EGCG-Cys-TPP exhibits enhanced mitochondrial accumulation tendencies, leading to the effective elimination of reactive oxygen species (ROS) and preservation of optimal mitochondrial membrane potential, which is approximately 1.5 times that of free AST and EGCG. Importantly, in vivo experiments have shown that the colon length of IBD mice treated with these nanocarriers increased by 51.29%, and promoted the polarization of M2 macrophages. The gut microbiota has been greatly improved in mice with DSS-induced colitis after AXT@EGCG-Cys-TPP treatment (Fig. 3).89
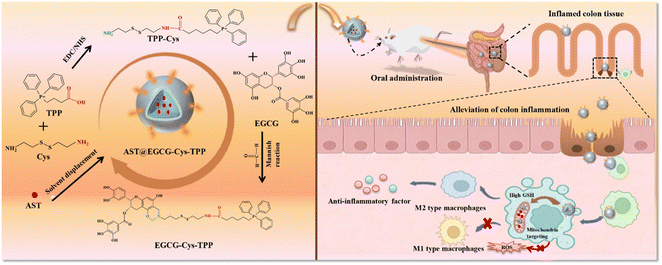 |
| Fig. 3 Schematic illustration of the formation of a dual nutrition nanocarrier oral delivery system for mitochondria targeting and GSH response (left) and its intervention effect on the IBD model (right). Reprinted from X. Zhang, W. Su and Y. Chen et al., Bi-functional astaxanthin macromolecular nanocarriers to alleviate dextran sodium sulfate-induced inflammatory bowel disease, Int. J. Biol. Macromol., 256, 128494, Copyright (2024), with permission from Elsevier. | |
3.1.4 Tocopherols.
Tocopherols are the natural forms of vitamin E, which encompass α-, γ-, and δ-tocopherol (αT, γT, and δT), possessing antioxidant and anti-inflammatory properties.90 These compounds have been demonstrated to exert protective effects on intestinal health. Roseburia intestinalis is an anaerobic, Gram-positive, slightly curved rod-shaped flagellated bacterium that produces butyrate in the colon, which has been proven to prevent intestinal inflammation and maintain energy homeostasis by producing metabolites.91 Supplementation with γ-tocopherol-rich tocopherols (γTmT) significantly reduced the depletion of Roseburia relative abundance induced by DSS. Bacteroides acidifaciens, as a new generation of probiotics, has demonstrated therapeutic effects on IBD.92 The addition of α-tocopherol increased the relative abundance of Bacteroides acidifaciens under DSS conditions.76 α-tocopherol is a lipophilic antioxidant. M. Plaza-Oliver et al. constructed a nanoemulsion as a new oral nanocarrier (NE-ADP) by stabilizing α-tocopherol with ascorbyl-2,6-dipalmitate.93 NE-ADP exhibits good stability in gastrointestinal media, and NE and ADP work synergistically to exert antioxidant effects for the treatment of IBD.
3.1.5 Curcuminoids.
Curcuminoids are a class of chemical compounds known as diarylheptanoids, which primarily encompass curcumin, demethoxycurcumin, and bisdemethoxycurcumin.94 Curcumin (Cur) exhibits strong antioxidant activity and regulatory effect on the gut microbiota, which is mainly manifested in its ability to increase the abundance and diversity of gut microbiota in mice with DSS-induced colitis, as well as its capacity to correct the relative abundance of Bacteroidetes altered by DSS.78 However, the poor solubility of Cur in water, photodegradation, and chemical instability limit its direct application in the treatment of IBD. Jintao Li et al. constructed a metal polyphenol network (MPN) via metal coordination between epigallocatechin gallate (EGCG) and Fe3+ and encapsulated Cur into MPN to generate Cur-MPN. Cur-MPN can effectively scavenge ROS. Then, the Cur-MPN is encapsulated within the yeast microcapsules (YM) to obtain CM@YM CM@YM is capable of protecting Cur-MPN from harsh gastrointestinal environments and enhancing its targeting and retention capabilities in inflamed colons. When orally administered, CM@YM can alleviate DSS-induced colitis by scavenging ROS, reducing pro-inflammatory cytokines, exhibiting both protective and therapeutic effects, thereby restoring barrier function and maintaining intestinal homeostasis. Importantly, CM@YM also regulates the gut microbiota to a beneficial state by improving bacterial diversity, shifting the composition to an anti-inflammatory phenotype, and increasing the content of short-chain fatty acids (SCFAs) such as acetic acid, propionic acid, and butyric acid (Fig. 4).95
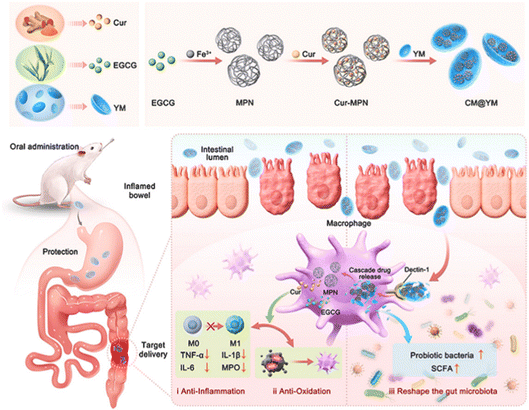 |
| Fig. 4 Schematic illustration of preparation and potential therapeutic mechanisms of CM@YM. Curcumin was self-assembled into the MPN formed by the coordination of EGCG and Fe3+ to form the ROS scavenger Cur-MPN, which was then encapsulated in YM by chemical precipitation to obtain CM@YM. Reprinted from J. T. Li, J. Song and Z. C. Deng et al., Robust reactive oxygen species modulator hitchhiking yeast microcapsules for colitis alleviation by trilogically intestinal microenvironment renovation, Bioact. Mater., 36, 203–220, Copyright (2024), with permission from Elsevier. | |
In summary, phytonutrients hold immense potential in regulating gut microbiota for the treatment of IBD. However, due to their inherent properties, including hydrophobicity, poor chemical stability, susceptibility to oxidation, and photolysis, they cannot fully exert their therapeutic effects and regulatory role on gut microbiota. By constructing a therapeutic system to load phytonutrients, their bioavailability can be greatly enhanced and precise release at the site of pathology ensured, thereby maximizing their therapeutic benefits.
3.2 Probiotic-based therapeutic systems
Minor changes in gut microbiota can trigger fluctuations in intestinal homeostasis, causing a “butterfly effect” and leading to severe inflammatory reactions. Probiotics can be regarded as the “fulcrum of Archimedes”, used to leverage the disrupted state of gut microbiota under inflammatory conditions and improve the composition of gut microbiota.17 FAO (Food and Agriculture Organization of the United Nations) and WHO (World Health Organization) define probiotics as live microorganisms that confer a health benefit on the host when administered in adequate amounts.96 This means that the vitality and quantity of probiotics will determine the extent to which they can influence the gut microbiota. However, in practice, the direct consumption of probiotics often yields suboptimal results due to their low survival and colonization rates in the intestine. This can be attributed to two main factors: physiological and pathological. Physiological factors refer to the fact that before probiotics reach the site of colonic inflammation, they must first pass through the digestive tract.97 The complex physiological environment of the digestive tract and the long duration of passage can both affect the viability of probiotics.98 Pathological factors refer to the loss of mucus layer in the intestinal tract during inflammatory states, which causes probiotics to lose their colonization sites, resulting in a low colonization rate.99 How to effectively enhance the survival rate of probiotics and their colonization rate at the site of intestinal inflammation is a crucial point in probiotic-mediated regulation of gut microbiota. To address this challenge, many researchers have developed therapeutic systems by encapsulating probiotics with protective layers to enhance their survival and colonization rates. We refer to these as exogenously modified probiotic therapeutic systems. Furthermore, the rapid advancement of gene engineering technology has opened up another pathway to enhance the therapeutic efficacy of probiotics by equipping them with new traits through gene editing techniques to cope with complex environmental conditions. We refer to this as the endogenously modified probiotic therapeutic system. In this section, we will provide detailed introductions to these two types of therapeutic systems.
3.2.1 Exogenous modified probiotic therapeutic systems.
Using exogenous materials to modify or encapsulate probiotics to construct a therapeutic system, thereby enhancing their survival ability and colonization rate, is an effective method for treating IBD.100 During the process of exogenous encapsulation, the probiotic, as part of the therapeutic system, is modified to acquire the ability to resist complex lesion environments without compromising its vitality.101 The modification coatings for probiotics encompass metal–polyphenol networks (MPNs), polymers, polysaccharides, proteins, biofilms, and so on. These various modification materials can endow probiotics with different exogenous functions, such as immunoregulation, antioxidant properties, and anti-inflammatory characteristics, paving the way for better colonization of probiotics and enabling them to exert their regulatory role in the intestinal flora.102 We will classify these therapeutic systems based on the modification materials and provide detailed introductions to each. In Table 2, we summarized the encapsulation materials, encapsulation processes, and their impacts on gut microbiota for both probiotic therapeutic systems based on exogenous modification.
Table 2 Probiotic-based therapeutic systems
Classification |
Encapsulation material |
Probiotic/core |
Encapsulation process |
Alterations of gut microbiota |
Ref. |
MPN-modified probiotic therapeutic systems |
Fe3+–tannic acid (Fe–TA) cross-linking network and carboxymethylated β-glucan (mGN) |
ECN |
Fe–TA network is formed through electrostatic interaction on the ECN surface; mGN is deposited on the Fe–TA network by hydrogen bonding |
Bacteroidetes, Bifidobacterium, SCFAs ↑, Escherichia Shigella ↓ |
103
|
Mn2+ and polydopamine |
Lactic acid bacteria (LAB) |
Mn2+ released by LAB mediates the oxidation of dopamine on the surface of LAB |
— |
104
|
Polymer-derived probiotic therapeutic systems |
Nitric oxide (NO)-responsive poly-γ-glutamic acid hydrogel microcapsules (NRPM) |
Lactic acid bacteria (LAB) |
Using a novel droplet-based microfluidic technology, probiotics are embedded into γ-PGA microgels. |
Turicibacter, Acidaminococcaceae, Negativicutes, and Phascolarctobacterium ↑ |
105
|
Polysaccharide-modified probiotic therapeutic systems |
Biocatalytic ferrihydrite nanoparticles and fucoidan-derived protective shield |
ECN |
One-step bioinspired mineralization process and a layer-by-layer electrostatic selfassembly process |
Muribaculaceae, Prevotellaceae UCG-001, Lachnospiraceae NK4A136 ↑, Proteobacteria, Escherichia Shigella ↓ |
106
|
High-molecular-weight hyaluronan (HMW-HA) and Fe3+ and catechins network and |
ECN |
Layer-by-layer coating procedure |
Lactobacillus, Muribaculaceae, Parabacteroides ↑, Desulfovibrio, Clostridium sensu stricto 1, Escherichia Shigella ↓ |
107
|
Hyaluronic acid (HA) |
Lactobacillus acidophilus (Lac) |
Lac solution was incubated with HDP solution for 4 h at a speed of 300 rpm (HDP@Lac) |
Lactobacillus acidophilus, Akkermansia ↑, Desulfovibrionaceae ↓ |
108
|
Dopamine (DOPA) |
|
|
|
|
Phenylboric acid (PA) |
|
|
|
|
Protein-modified probiotic therapeutic systems |
Proanthocyanidins (PAs), mucin (MUC), phosphatidylcholine (PC) and methacrylate modified gelatin porous microgels |
Akkermansia muciniphila (AKK) |
Modifying AKK with proanthocyanidins (PAs), mucin (MUC), and phosphatidylcholine (PC), and then encapsulating it within methacrylate-modified gelatin porous microgels (AKK@GPMGs) |
Lactobacillaceae, Muribaculaceae, Akkermansiaceae ↑, Enterobacteriaceae, Desulfovibrionaceae, Porphyromonadaceae ↓ |
109
|
Ca2+–tannic acid (Fe–TA) cross-linking network and mucin |
ECN |
Layer-by-layer coating with mucin and TA |
Lachnospiraceae NK4A136, Bifidobacterium adolescentis ↑, Escherichia coli ↓ |
110
|
Biofilm-modified probiotic therapeutic systems |
A self-coating biofilm |
Bacillus subtili (BS) |
By cultivating BS on a solid minimal salt-glucose-glutamate (MSgg) agar plate, a self-coating biofilm formed on the surface of the BS |
—
|
111
|
3.2.1.1 MPN-modified prebiotic therapeutic systems.
MPNs are supramolecular network structures consisting of metal ions coordinated to phenolic ligands.112 Due to their unique physicochemical properties and biocompatibility, MPNs have received considerable attention in the biomedical field in recent years. MPNs, capable of forming continuous and stable coatings on various substrate surfaces and exhibiting remarkable defensive and adhesive properties, hold enormous potential for application in the modification of probiotics.113 Polyphenols and metal ions can chelate on the surface of probiotics, forming a protective layer that enhances the survival rate of the probiotics. Furthermore, the phenolic hydroxyl groups in MPNs can covalently bind with proteins at sites of colonic inflammation, thereby strengthening the adhesion of probiotics to the intestinal wall.17Escherichia coli Nissle 1917 a Gram-negative strain, not only exhibits antagonistic effects against a variety of intestinal pathogens but also regulates the secretion of immune factors in vivo, enhancing the host's immune capacity.114 Xie Anqi et al. used Fe3+–tannic acid (Fe–TA) cross-linking network and carboxymethylated β-glucan (mGN) to modify Escherichia coli Nissle 1917 (ECN) (ECN@Fe–TA@mGN). Under the protection of the Fe–TA@mGN “shield”, the survival rate of the armed ECN after simulated gastric juice was 1720 times higher than that of bare EcN. After reaching the colonic inflammatory site, mGN is degraded with the assistance of enzymes secreted by intestinal microorganisms, releasing Fe–TA-modified ECN (ECN@Fe–TA). The Fe–TA network can help ECN adhere to the intestinal inflammatory site, greatly improving the retention rate of ECN at the colonic inflammatory site. The intestinal retention rate of EcN@Fe–TA@mGN reached as high as 47.54 ± 6.06% at 16 hours post-administration, while nearly all of the bare EcN were excreted within 8 hours post-administration. Moreover, EcN@FeTA@mGN is able to positively regulate gut microbiota, thereby alleviating intestinal inflammation (Fig. 5).103
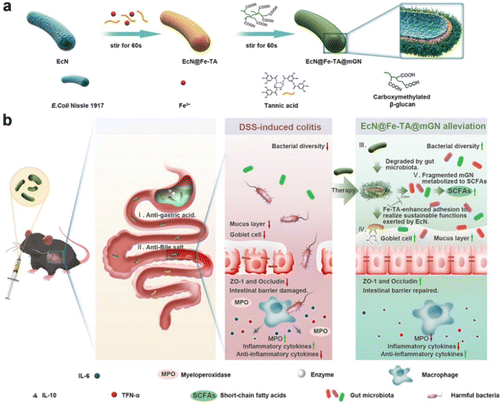 |
| Fig. 5 Schematic illustration of the fabrication of EcN@Fe–TA@mGN and its alleviation process for DSS-induced colitis in mice. (a) Preparation of EcN@Fe–TA@mGN. (b) Probiotics exhibited superior resistance to gastric acids and bile salts after being armed with a Fe–TA@mGN “shield” and remained intact in the upper digestive tract. Reproduced from reference [A. Xie, H. Ji and Z. Liu et al., Modified Prebiotic-Based “Shield” Armed Probiotics with Enhanced Resistance of Gastrointestinal Stresses and Prolonged Intestinal Retention for Synergistic Alleviation of Colitis, ACS Nano, 2023, 17(15), 14775–14791] with permission from [ACS Publictions], copyright [2023]. | |
3.2.1.2 Polymer-derived probiotic therapeutic systems.
Polymers are macromolecules composed of many repeating units connected by covalent bonds, which mainly include natural polymers and synthetic polymers.115 As an effective drug delivery tool, polymers have shown wide application and clinical success in various diseases due to their simple synthesis methods, the ability to adjust chemical structures and self-assemble hierarchical structures, as well as good biocompatibility and biodegradability.116 Due to their ability to achieve passive/active targeted delivery, adhesion, mucus penetration, and controlled drug release through their physicochemical properties such as surface charge, surface functional groups, and polymer segments, polymers have emerged as excellent carriers for probiotic delivery.117 For example, poly-γ-glutamic acid (γ-PGA) is a safe and edible polymer that constitutes the mucus in fermented foods. It has been reported to counteract the colonization of harmful microorganisms in the intestine and possesses excellent anti-inflammatory properties, making it an excellent carrier for probiotic delivery.118,119 Rui Wang et al. constructed nitric oxide (NO)-responsive γ-PGA hydrogel microcapsules (NRPM) based on a microfluidic technology platform for encapsulating lactic acid bacteria (LAB). NPRM demonstrates stability in a gastric acid environment. Due to the cytoprotective effects of NRPM, the modified probiotics exhibited higher viability in simulated gastric (89.67%) and intestinal (93.67%) fluid environments, compared to 0% and 61.60% for the free cells, respectively. At sites of colonic inflammation, it rapidly releases LAB in response to NO molecule stimulation, facilitating intestinal mucosal repair and regulating the balance of intestinal flora, ultimately achieving the mitigation of colitis.105
3.2.1.3 Polysaccharide-modified probiotic therapeutic systems.
Polysaccharides are high-molecular-weight carbohydrates very large variety of architecture (linear or branched) and linkages connecting their monomeric units. They are ubiquitous in plants, fungi, microorganisms, algae, and animals.120,121 Evidence suggests that some polysaccharides exhibit good resistance to gastric acid. In the gastric environment, polysaccharides may undergo a slight or significant reduction in molecular weight, potentially due to the cleavage of glycosidic bonds and dispersion of aggregates. However, they are not digested into monosaccharides for absorption and utilization.122 This makes them excellent carriers for the delivery of probiotics through the complex gastrointestinal environment. In addition, some polysaccharides exhibit excellent targeting capabilities towards inflamed areas of the colon, ensuring the precise release of the therapeutic system at the site of the lesion. For example, fucoidan is not only a specific ligand for P-selectin, which is overexpressed in inflamed intestines, but it is also capable of electrostatic binding to positively charged proteins accumulated in damaged intestinal epithelia.123,124 Chen ting et al. coated the nanozyme-loaded ECN (ECN-Fh) with fucoidan (ECN-Fh@F) through a layer-by-layer electrostatic self-assembly process using chitosan. This fucoidan-based shielding further encapsulates the probiotics, imparting them with additional inflammatory colon targeting capabilities. Upon oral administration, the engineered probiotics significantly enhanced the viability and colonization of the inflamed gut (Fig. 6).106 High-molecular-weight hyaluronan (HMW-HA), is a negatively charged glycosaminoglycan biopolymer that specifically binds to the CD44 receptor. Limeng Zhu et al. utilized HMW-HA as a nano-armor for ECN, endowing the probiotics with exceptional resistance to various extreme environmental conditions. In the gastric acid environment, the relative survival rate of EcN@PC-Fe/HA was 80.3%, while the relative survival rate of native EcN was 6.0%. The functionalization of HMW-HA, through its specific positive charges associated with inflammatory lesions and the high expression of CD44 receptors, facilitates the targeted accumulation of probiotics in the diseased regions of the gut. Observed through IVIS, the fluorescence signal of EcN-mCherry@PC-Fe/HA towards inflamed colon was 1.6 times that of EcN-mCherry@PC-Fe. Protected by nano-armor, ECN can effectively enhance the diversity of intestinal microbiota and reduce intestinal pathogens.107 In addition to directly using polysaccharides for probiotic delivery, chemical modification can endow polysaccharides with new properties that make them better suited for probiotic delivery. Qian-Xiao Huang and his colleagues utilized dopamine protected by phenylboric acid to modify hyaluronic acid (HDP) for the delivery of Lactobacillus acidophilus (Lac). HDP can protect Lac from the damaging effects of gastric acid, enhancing its survival rate. In the presence of high levels of ROS, the phenylborate ester groups in Lac@HDP undergo oxidation and cleavage, exposing catechol hydroxyl groups and imparting a robust mucosal adhesion capability. This significantly prolongs the retention time of Lac at the inflammatory site.108
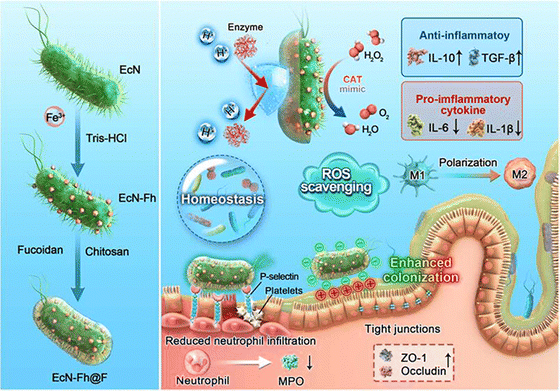 |
| Fig. 6 Schematic illustration of the development of engineered probiotics for multipronged management of IBD and the characterization of EcN-Fh. The in situ growth of biocatalytic Fh NPs on probiotics through a one-step bioinspired mineralization process. The Fh NPs-decorated probiotics are further coated with a fucoidan-derived protective shield to enhance colon delivery. Reproduced from reference [T. Chen, W. Meng and Y. Li et al., Probiotics Armed with In Situ Mineralized Nanocatalysts and Targeted Biocoatings for Multipronged Treatment of Inflammatory Bowel Disease, Nano Lett., 2024, 24(24), 7321–7331] with permission from [ACS Publictions], copyright [2024]. | |
3.2.1.4 Protein-modified probiotic therapeutic systems.
Mucin is an acid-resistant glycoprotein composed of mucopolysaccharides, commonly found in gastrointestinal mucus.125 The acid resistance of mucin, combined with its ability to interact with the intestinal mucus layer through hydrogen bonds, disulfide bonds, and hydrophobic interactions, makes it an excellent material for constructing probiotic therapeutic systems.126Akkermansia muciniphila (AKK) is a probiotic that resides in the mucus layer. Its beneficial activities are primarily exerted through consuming mucin and producing bioactive compounds, which nourish intestinal cells and commensal probiotics.127,128 Yujie Zhang et al. developed an AKK-based therapeutic system, (AKK@GPMGs), which involves modifying AKK successively with proanthocyanidins (PAs), mucin (MUC), and phosphatidylcholine (PC), and then encapsulating it within methacrylate-modified gelatin porous microgels. In this therapeutic system, mucin serves as a sufficient energy source for AKK, promoting its growth and reproduction. Furthermore, AKK@PAs-MUC-PC (AKK@PMP) is released at the inflamed sites in the colon, and the PAs-MUC-PC layer in AKK@PMP exhibits strong adhesion properties towards the inflamed colon sites, ensuring the colonization of AKK in the intestine.109 After treatment with AKK@GPMGs, the mucus thickness of colitis mice increased, intestinal permeability decreased, and goblet cell abundance increased, significantly enhancing the beneficial intestinal microbiota while inhibiting harmful microbiota (Fig. 7).
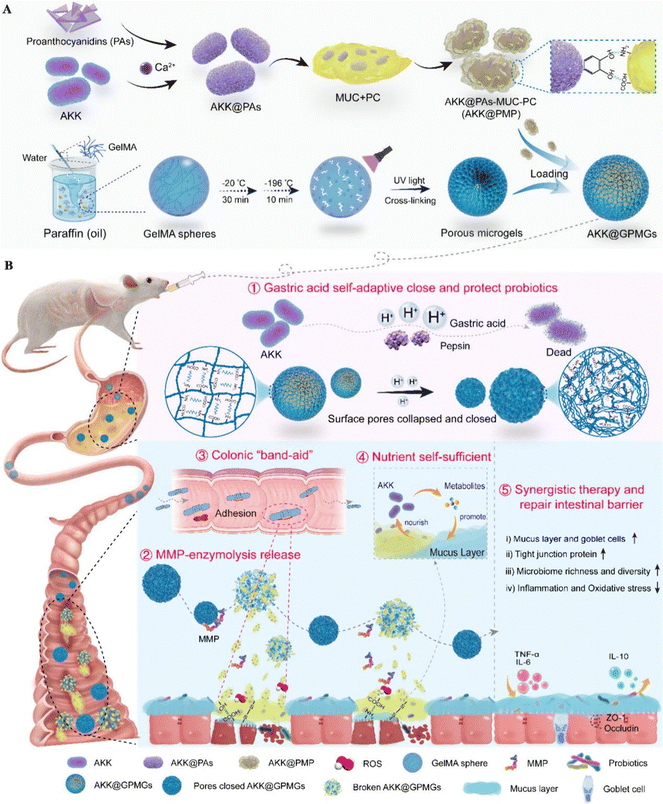 |
| Fig. 7 Gastrointestinal self-adaptive and nutrient self-sufficient Akkermansia muciniphila-gelatin porous microgels (AKK@GPMGs) for synergistic therapy of UC: (A) preparation of AKK@GPMGs; (B) properties and synergistic therapy of AKK@GPMGs. Reproduced from reference [Y. Zhang, Y. Wang and X. Zhang et al., Gastrointestinal Self-Adaptive and Nutrient Self-Sufficient Akkermansia muciniphila-Gelatin Porous Microgels for Synergistic Therapy of Ulcerative Colitis, ACS Nano, 2024, 18(39), 26807–26827] with permission from [ACS Publictions], copyright [2024]. | |
3.2.1.5 Biofilm-modified probiotic therapeutic systems.
Biofilms are protective layers produced by bacteria under extreme conditions to counteract external environmental threats, such as displacement caused by physical forces and elimination due to environmental attacks.129 Biofilms are primarily composed of biomacromolecules, including proteins, polysaccharides, DNA, RNA, peptidoglycan, lipids, and phospholipids.130 For bacteria, the biofilm not only serves as an adhesive that attaches colonies to surfaces and prevents their removal by flowing liquids but also defends against external threats such as antibiotics and the host immune system by preventing penetration.131,132 This means that the biofilm can function as part of a therapeutic system, offering both chemical barrier and physical adhesion properties to protect probiotics as they navigate through the complex gastric environment and colonize at sites of colonic inflammation. Bacillus subtili (BS) is an important probiotic that can work synergistically with other beneficial gastrointestinal bacteria to support digestion, enzyme production, and the health of the immune and digestive systems.133 Under appropriate conditions, BS can secrete large amounts of extracellular polysaccharides and proteins such as TasA and BslA, which collectively form a biofilm.134 Based on this, Xinyue Wang et al. used BS as a probiotic model and cultivated it on solid minimal salts glycerol glutamate plates to stimulate the formation of a robust biofilm on the surface of BS. Biofilm-coated BS demonstrated significantly improved gastrointestinal tolerance and adhesion in mice and pigs. Especially in the pig model, the oral bioavailability of biofilm-coated BS was 125 times higher and the colonization rate was 17 times greater compared to uncoated BS.111
In summary, probiotic therapeutic systems based on exogenous modification can effectively protect probiotics from the damaging effects of gastric acid, thereby enhancing their survival rate. Different modification materials can impart probiotics with improved adhesion and retention time on the intestinal wall, enabling them to better exert their regulatory effects on the intestinal flora.
3.2.2 Endogenous modified probiotic therapeutic systems.
In addition to endowing probiotics with some new characteristics through encapsulation and modification, gene editing technology can also improve the characteristics of probiotics to enhance their stability in complex environments and promote their colonization at intestinal inflammatory sites. The probiotic therapeutic systems constructed based on gene editing technology are referred to as endogenous modified probiotic therapeutic systems. Probiotics possess a vast genome, which provides convenience for their genetic modification. Moreover, from homologous recombination to the first-generation Zinc Finger Nucleases (ZFNs) technology, to the second-generation Transcription Activator-like Effector Nucleases (TALENs) technology, and the more popular third-generation Clustered Regularly Interspaced Short Palindromic Repeats (CRISPR)-associated (CRISPR–Cas) technology in recent years, gene editing technologies have provided more powerful tools for editing and modifying microbial genomes.135 Based on this, genetically engineered probiotics have been designed for the treatment of IBD and we will introduce it in detail through some examples.
Jun Zhou et al. genetically engineered ECN to overexpress CAT and SOD (ECN-pE) to alleviate oxidative damage caused by IBD. To improve the bioavailability of ECN-pE, they used chitosan and sodium alginate to coat ECN-pE (ECN-pE(C/A)2). Such engineered EcN-pE(C/A)2 could be effective in relieving inflammation and removing ROS. It also can regulate the gut microbiota and improve the abundance of Lachnospiraceae_NK4A136 and Odoribacter, which could produce an important short-chain fatty acid, butyrate. Butyrate serves not only as a crucial energy source for colonic epithelial cells but also inhibits the release of inflammatory cytokines and upregulates the expression of TJs.136 eATP is produced by activated immune cells and commensal bacteria and is relevant to IBD.137 On the one hand, eATP activates purinergic receptors to boost pro-inflammatory cytokine production; on the other hand, eATP activates effector T cells, suppresses regulatory T cell (Treg) responses, and promotes enteric neuron apoptosis.138–140 Based on the association of eATP with IBD, Benjamin M. Scott et al. developed yeast-based engineered probiotics that express a human P2Y2 purinergic receptor which greatly increases the eATP sensitivity. Then they linked the activation of this engineered P2Y2 receptor to the secretion of the ATP-degrading enzyme apyrase thus to create engineered yeast probiotics which can sense a pro-inflammatory molecule and generate a proportional self-regulated response aimed at its neutralization. With the presence of eATP, these yeast-based engineered probiotics responsively secrete the CD39-like eATP-degrading enzyme apyrase. Clostridium cluster XIVa is associated with the induction of Treg cells, and it is consistently decreased in patients with IBD. Engineered yeast probiotics show good therapeutic effects on IBD, and the abundance of Lachnospiraceae, which is included in the Clostridium cluster XIVa, in colitis mice treated with engineered yeast probiotics is significantly higher than that in colitis mice without engineered yeast probiotics treatment.141 Sulfate-reducing bacteria (SRBs) present in the colon produce hydrogen sulfide (H2S) from sulfur oxide species extracted from the host and host enzymes detoxify H2S to thiosulfate.142,143 Thiosulfate as a biomarker of IBD, is an appealing target for studying the link between gut sulfur metabolism and inflammation.144 Ting-Ting Fang et al. constructed a thiosulfate-responsive engineered bacteria (E-acgEBE-AvG). E-acgEBE-AvG was composed of three units: a timely reporting unit, a single-base-edited unit, a controllable recorded-cell self-lysis, and a drug release unit. In controllable recorded-cell self-lysis and drug release unit, with the presence of thiosulfate and xylose, lysis E was expressed which led to the lysis of the edited bacteria and immunomodulator AvCystain release.145 Zhen-Ping Zou et al. also developed a kind of “intelligent” whole-cell engineered bacteria, i-ROBOT (intelligent responsive bacteria for diagnosis and therapy) which constitutes a self-tunable drug-secreting system that can respond to levels of the inflammatory marker thiosulfate to drive the release of AvCystatin. Mice with colitis treated with i-ROBOT showed reduced levels of inflammatory factors and restored intestinal epithelial tissue.146 Designing antibodies to reduce the expression of inflammatory cytokines (such as TNF-α) is the most common immunotherapy in IBD.147 Interferon lambda 1 (IFNL1) as a member of the IL-10 superfamily, signals through various pathways involving IL-10 and type I IFNs, making it a promising candidate to investigate the treatment of IBD.148 Based on this, Koon Jiew Chua et al. engineered probiotic EcN (ECN-IFNL1) to produce and secrete a type III interferon, interferon lambda 1 (IFNL1), in response NO. Then, their experiment demonstrated that IFNL1-expressing EcN strains upregulated Foxp3 expression in T cells and thereafter reduced the production of pro-inflammatory cytokines such as IL-13 and IL-33, significantly ameliorating inflammation. In addition, EcN-IFNL1 can also maintain the intestinal epithelial barrier and restore intestinal homeostasis by promoting the expression of TJs.149
In summary, gene editing technology can equip probiotics with new characteristics, such as novel enzymatic activities, new responsiveness, and improved drug-loading capabilities, which not only enhance their stability in complex environments but also significantly broaden their application scope, making them suitable not only for the treatment of IBD but also for its diagnosis.
3.3 Prebiotic-based therapeutic systems
Prebiotics are defined as a substrate that is selectively utilized by host microorganisms conferring a health benefit.150 Currently, prebiotics mainly include polyphenols,151 polysaccharides,152 peptides,153 microalgae154etc. They can provide nutrients for beneficial bacteria in the gut, promoting their growth and reproduction, and also enhancing the levels of beneficial metabolites produced by the gut microbiota.155–157 For example, as a prebiotic, microalgae accumulate substantial fatty acids and other substances beneficial to the growth of intestinal flora, which can be utilized by the intestinal flora to exert a positive regulatory effect on it. However, the efficacy of prebiotics can be limited by their physicochemical properties. For example, the poor oral bioavailability of pure polyphenol prebiotics due to their solubility limits their ability to regulate gut microbiota.151 The rapid fermentation characteristics of polysaccharides in the intestine may lead to a shortage of fermentable carbohydrates for bacteria in the distal colon, affecting the stability of gut microbiota.158 Therefore, it is necessary to take measures to address the challenges faced by prebiotics in the process of exerting their efficacy. Researchers have done a lot of work to address these challenges by constructing therapeutic systems to deliver prebiotics, which is an effective strategy to improve their bioavailability and prolong their action time at the site of colonic inflammation. In addition, the characteristics of prebiotics, such as their resistance to gastric acid and non-hydrolysis by relevant enzymes in the gastrointestinal tract, also make them suitable as carriers for drug delivery.159 For instance, the bioactive microalgae Spirulina platensis (SP) possesses excellent biocompatibility, cost-effectiveness, a large active surface area, remarkable stability, and resistance to gastric acid, making it an ideal natural carrier for drug delivery160 Based on this, we classify prebiotic therapeutic systems into two categories: those aimed at delivering prebiotics and those that utilize prebiotics as carriers, and we will provide detailed introductions to both in this part.
3.3.1 Therapeutic systems for prebiotic delivery.
The hydrophobicity of polyphenol prebiotics is the main reason for their poor bioavailability. The therapeutic systems based on PLGA have particular advantages in delivering hydrophobic drugs, as they can further improve the solubility of these drugs and exhibit high encapsulation efficiency.161 Xiangji Yan et al. have developed a P-selectin binding peptide-decorated poly lactic-co-glycolic acid nanoparticle (PBP-PLGA-NP) as a carrier to load hydrophobic polyphenol prebiotic resveratrol. The PBP-PLGA-NP delivery platform significantly enhances the bioavailability of resveratrol, and through colon targeting, ensures its precise release at the site of inflammation, effectively alleviating intestinal inflammation and improving gut microbiota.162 Polysaccharide prebiotics exert their therapeutic effects primarily through fermentation by gut microbiota, therefore, the efficacy of polysaccharide prebiotics is closely related to their retention time in the intestine. Yeast β-glucan isolated from Saccharomyces cerevisiae is a typical polysaccharide prebiotic fermented by gut microbiota, which can selectively promote the growth of probiotics.163,164 Fan Yang et al. encapsulated derivatives of β-glucan within a polydopamine coating (PDA) through self-assembly. PDA, rich in catechol groups, can effectively adhere to the colonic epithelium, thereby prolonging the retention time of β-glucan at the site of colonic inflammation in the intestine and enhancing the prebiotic activity to improve the gut microbiota.165 In addition, gel exhibits excellent colonic adhesion due to its unique physical and chemical properties, and is capable of effectively prolonging the retention time of drugs. Inulin, a typical polysaccharide prebiotic fermented by gut microbiota, can increase the diversity and abundance of commensal bacteria.166 Zhuangzhuang Zhang et al. developed an inulin gel and used it to form a macroporous composite material with nanoneedles to improve inflammation and regulate intestinal flora. After oral administration, the macroporous composite material exhibited good intestinal adhesion, greatly increasing the retention time of inulin in the intestine (Fig. 8).167
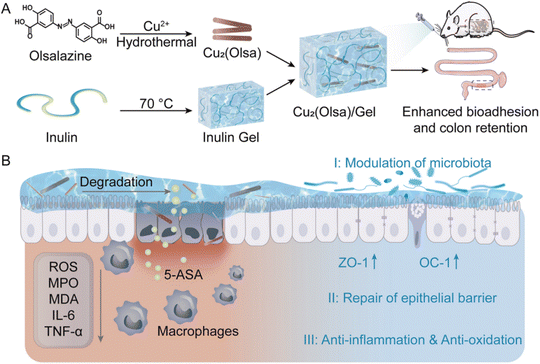 |
| Fig. 8 Schematic illustration of Cu2(Olsa) and inulin gel composite for IBD treatment by restoring intestinal homeostasis. (A) Preparation of Cu2(Olsa)/Gel. (B) The proposed therapeutic mechanisms of Cu2(Olsa)/Gel. Reprinted from Z. Zhang, Y. Pan and Z. Guo et al., An olsalazine nanoneedle-embedded inulin hydrogel reshapes intestinal homeostasis in inflammatory bowel disease, Bioact. Mater., 33, 71–84, Copyright (2024), with permission from Elsevier. | |
3.3.2 Therapeutic systems composed of prebiotic.
Some prebiotics possess inherent abilities such as flexible structure, good biocompatibility, controlled release behavior, adjustable degradation kinetics, and protective capabilities, making them excellent carriers for drug delivery.168 Inulin can not only be used as an active substance for delivery but also serves as an excellent carrier due to its chemical modifiability and gastric acid resistance. Qijuan Sun et al. grafted 4-aminothiophenol (ATP) onto carboxymethyl inulin (CMI) to create a nanocarrier for loading budesonide. The ATP modification transformed the inulin into an amphiphilic polymer, effectively addressing the poor water solubility of budesonide and significantly enhancing its bioavailability (Fig. 9).169 Microalgae are photosynthetic microorganisms rich in protein, polysaccharides, minerals, and various vitamins, which can exert a positive influence on the gut microbiota.170,171 The special structures of some microalgae can ensure that drugs pass through the complex gastrointestinal environment, protecting the drugs from successfully reaching the colon and exerting their therapeutic effects. Danni Zhong et al. constructed a therapeutic system based on bioactive microalgae SP for loading curcumin to treat ulcerative colitis. The gastric acid resistance and stability of SP ensure the smooth delivery of curcumin to the site of colonic inflammation. Subsequently, SP is captured by intestinal villi, gradually degraded, and releases curcumin, thereby achieving an ideal drug distribution in the intestine without causing adverse reactions.172 Pectin is a natural and food-grade polysaccharide prebiotic obtained from the primary cell wall of plants, which can overcome the instability of the treatment system in the stomach.173 Rhein (RH) has good anti-inflammatory effects, but its oral bioavailability is poor due to its limited solubility.174 Ruifeng Luo et al. have developed a nanocarrier formed by modifying lactoferrin with calcium pectinate (CP) and hyaluronic acid (HA), which is used to load RH to improve its bioavailability. CP layer makes CP/HA/RH-NPs more stable and protects RH from destruction in the gastrointestinal environment (Fig. 10).175 In addition, some prebiotics can also be used to achieve controlled drug release due to their good responsiveness to the intestinal microenvironment. Alginate is an anionic polysaccharide with good biocompatibility and biodegradability. Due to its ionizable carboxylic acid groups, it can undergo reversible protonation and deprotonation in different pH environments to achieve responsive drug release.176 Xue Di Zhang and his colleagues modified alginate with polypropylene sulfide to load AST (AST@RS-PPS). After 180 minutes of UV irradiation, the retention rate of AXT in AST@RS-PPS was 58.46 ± 0.25%, which was 5.24 times higher than that of free AXT. After a 6-hour treatment in a 60 °C water bath, the retention rate of AXT in AST@RS-PPS was 78.94 ± 1.44%, representing 4.6 times that of free AXT. This modified RS-PPS protects the structural stability of AST. In addition, the results of animal experiments indicate that AST@RS-PPS can significantly alleviate colitis, protect the integrity of colonic tissue, restore the expression of tight junction proteins ZO-1 and occludin, and increase the abundance of beneficial bacteria. Compared to the control group and DSS group, the RS@PPS group significantly upregulated the abundance of Lachnospiraceae_NKA136 and Clostridia. The increase in the abundance of Lachnospiraceae_NKA136 and Lachnospiraceae is beneficial for the alleviation of colitis and the production of short-chain fatty acids (Fig. 11).177
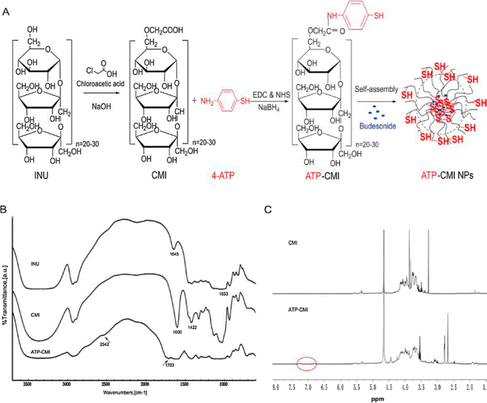 |
| Fig. 9 (A) Synthetic procedure of CMI, ATP-CMI conjugate and ATP-CMI NPs. (B) FT-IR spectrums of INU, CMI, and ATP-CMI conjugate. (C) 1H NMR spectra of CMI and ATP-CMI conjugate in D2O. Reprinted from Q. Sun, L. Luan and M. Arif et al., Redox-sensitive nanoparticles based on 4-aminothiophenol-carboxymethyl inulin conjugate for budesonide delivery in inflammatory bowel diseases, Carbohydr. Polym., 189, 352–359, Copyright (2018), with permission from Elsevier. | |
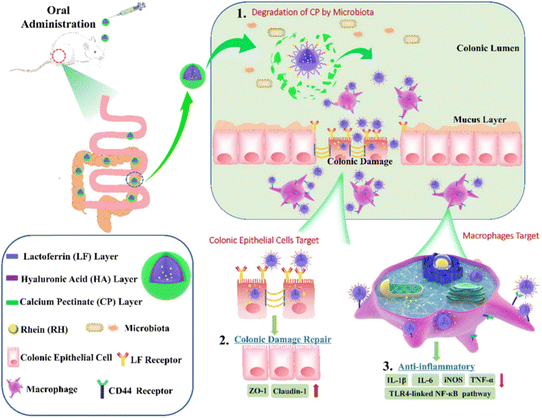 |
| Fig. 10 Schematic illustration of using CP/HA/RH-NPs in the treatment of UC in mice. (1) Schematic illustration of the passage of orally administered CP/HA/RH-NPs through GIT. CP could protect CP/HA/RH-NPs from passing through the stomach and small intestine, and further release HA/RH-NPs into the colonic lumen due to its degradation. (2) Schematic illustration of enhancing the effects of RH in repairing intestinal damage by adjusting ZO-1 and Claudin-1 expression in the UC mice model by colonic epithelial cell target. (3) Schematic illustration of targeting macrophage could effectively promote RH's anti-inflammatory effect through the TLR4/MyD88/NF-κB pathway in in vivo anti-UC therapeutic efficacy. Reprinted from R. Luo, M. Lin and C. Fu et al., Calcium pectinate and hyaluronic acid modified lactoferrin nanoparticles loaded rhein with dual-targeting for ulcerative colitis treatment, Carbohydr. Polym., 263, 117998, Copyright (2021), with permission from Elsevier. | |
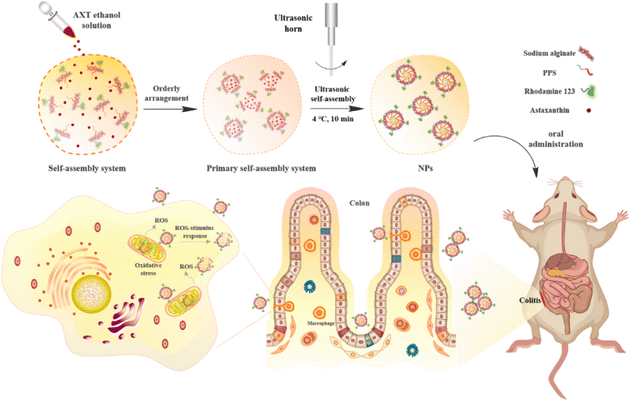 |
| Fig. 11 Preparation of ROS/pH dual-responsive AXT-loaded nanoparticles and their application in the treatment of colitis. Reprinted from X. Zhang, X. Zhao and Z. Hua et al., ROS-triggered self-disintegrating and pH-responsive astaxanthin nanoparticles for regulating the intestinal barrier and colitis, Biomaterials, 292, 121937, Copyright (2023), with permission from Elsevier. | |
In summary, by constructing therapeutic systems that deliver prebiotics to sites of intestinal inflammation, their retention time in the intestine can be extended, maximizing their ability to modulate the intestinal microbiota. Additionally, utilizing prebiotics as encapsulating materials to deliver other drug molecules can effectively enhance the bioavailability of these drug molecules, significantly improving their therapeutic efficacy.
3.4 Gut microbiota metabolites-based therapeutic systems
The gut microbiota metabolites are one of the primary modes of interaction between the gut microbiota and the host.178 The gut microbiota metabolites mainly include SCFAs, tryptophan derivatives, and secondary bile acids.179 In a healthy state, gut microbiota metabolites contribute to maintaining the host's basic functions, including facilitating the absorption of nutrients, promoting the growth and development of IECs, and maintaining immune homeostasis.180 However, in an inflammatory state, the metabolism of gut microbiota becomes disrupted, causing a decrease in the levels of these “beneficial” metabolic products, which further exacerbates the inflammation by losing the positive regulatory effects on the intestinal microenvironment.181 With the gradual deepening of research into gut microbiota and its metabolites, researchers have found that directly supplementing gut microbiota metabolites can effectively reverse the disrupted state of the gut microbiota, restore the positive regulatory effects of gut microbiota metabolites on the intestinal microenvironment, and inhibit the further spread of intestinal inflammation. For example, supplementing with SCFAs can regulate the recognition of the innate immune system, thereby inhibiting the progression of intestinal inflammation.182 However, therapies based on gut microbiota metabolites are often limited by bioavailability, preventing them from maximizing their therapeutic efficacy. An effective means to enhance the therapeutic efficacy is to construct a therapeutic system that carries gut microbiota metabolites for precise release at the site of the lesion. In this section, we will separately introduce SCFAs, tryptophan derivatives, and secondary bile acids, along with the therapeutic effects of therapeutic systems based on these gut microbiota metabolites for IBD.
3.4.1 Short-chain fatty acids.
SCFAs are carboxylic acids containing 1–6 carbon atoms, primarily produced by the anaerobic fermentation of dietary fiber.183 They provide energy for epithelial cells to maintain the epithelial barrier and participate in maintaining intestinal immune homeostasis.132 Butyrate, as a representative of SCFAs, has been clearly shown to regulate the gut microbiota by increasing the abundance of beneficial bacteria in DSS-induced colitis mice.184 However, butyrate can be rapidly metabolized in the body, resulting in a short half-life, which limits its ability to fully exert its therapeutic effects.28 XXi Fan et al. employed poly(ethylene glycol)-block-polymethylmethacrylate as the backbone and covalently attached butyrate to this backbone through disulfide bonds, subsequently forming the polymer nanoparticle (PSBA). With a butyrate content as high as 22%, PSBA achieved the responsive release of butyrate at the site of colonic inflammation, significantly enhancing its bioavailability (Fig. 12).185
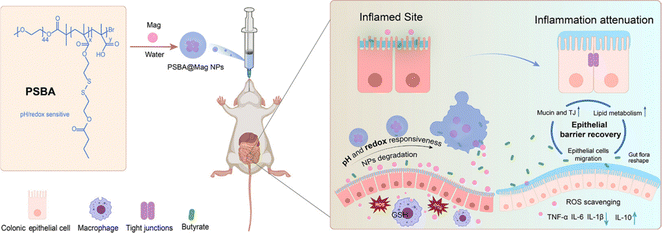 |
| Fig. 12 Magnolol-incorporated pH and redox dual responsive butyrate-based polymeric nanoparticles (PSBA@Mag) as an oral nanomedicine to treat inflammatory bowel disease by improving epithelial barrier repair and inflammation mitigation. Reproduced from reference [X. Fan, Z. Z. Zhang and W. X. Gao et al., An Engineered Butyrate-Derived Polymer Nanoplatform as a Mucosa-Healing Enhancer Potentiates the Therapeutic Effect of Magnolol in Inflammatory Bowel Disease, ACS Nano, 2023, 18(1), 229–244] with permission from [ACS Publications], copyright [2023]. | |
3.4.2 Tryptophan derivatives.
Tryptophan can be metabolized by the gut microbiota into indole derivatives (such as indole-3 acetic acid, indole-3 propionic acid, etc.),186 which can play an immunomodulatory role by activating AHR receptors, thereby alleviating inflammation and regulating gut microbiota.187 Indole-3 propionic acid (IPA), a tryptophan derivative with anti-inflammatory properties, can reduce the expression of pro-inflammatory factors in colitis mice and increase the abundance of beneficial bacteria to treat IBD.188 However, the poor oral administration efficiency limits its therapeutic effectiveness. Keli Yang et al. ensured the successful encapsulation of IPA in prebiotic microcapsules composed of alginate and resistant starch (RS) through an electrostatically driven microfluidics platform (IPA@MC), significantly enhancing the bioavailability of IPA. Additionally, IPA@MC demonstrated excellent capability in modulating gut microbiota (Fig. 13).189 Indole-3-acetic acid (IAA), as an important AHR receptor agonist among tryptophan derivatives, can regulate the gut microbiota under inflammatory conditions by modulating immune homeostasis.190 However, since indole-3-acetic acid (IAA) is absorbed in the upper digestive tract, especially in the small intestine, the direct oral administration of IAA results in low bioavailability. To effectively deliver IAA to the site of colonic inflammation, Yingying Song et al. synthesized HAMSIAA by connecting IAA to high amylose maize starch (HAMS) through esterification. HAMSIAA can protect IAA from the stimulated gastric acid environment to reach the inflammatory site, where HAMS is metabolized by intestinal flora into short-chain fatty acids, while IAA alleviates intestinal inflammation by activating AHR receptors.191
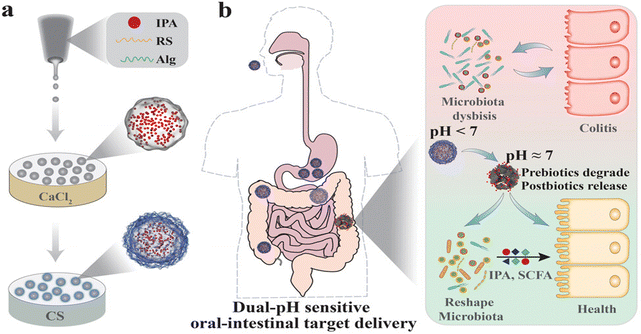 |
| Fig. 13 Schematic illustration of the prebiotics encapsulating postbiotic microcapsules (IPA@MC) for preventing and treating colitis. (a) The microfluidic electrospray procedures for generating IPA encapsulating Alg/RS microcapsules further coated by chitosan. (b) The dual-pH sensitive core–shell structure allows a slow drug release in the acid condition of the upper gastrointestinal tract and a rapid drug release in the neutral condition of the lower gastrointestinal tract to exert the protective effects against colitis via modulating gut microbiota. Alg: alginate; RS: resistant starch; CS: chitosan; IPA: indole-3-propionic acid. Reproduced from reference [K. Yang, X. Wang, R. Huang, H. Wang, P. Lan and Y. Zhao, Prebiotics and Postbiotics Synergistic Delivery Microcapsules from Microfluidics for Treating Colitis, Adv. Sci., 2022, 9(16)] with permission from [Wiley], copyright [2022]. | |
3.4.3 Secondary bile acids.
Bile acids (BAs) include primary bile acids (PBAs) and secondary bile acids (SBAs). PBAs, synthesized from cholesterol in the liver, are modified by gut microbiota into SBAs.192 SBAs primarily include lithocholic acid (LCA), deoxycholic acid (DCA), and ursodeoxycholic acid (UDCA), among others. They can function as signaling molecules, interacting with membrane receptors such as Takeda G protein-coupled receptor 5 (TGR5), nuclear receptors, and their downstream metabolic enzyme transporters to influence the dynamics of the microbiome and regulate various inflammatory pathways.193 Studies have shown that the levels of SBAs are closely related to the development of IBD. Yang et al. found that the levels of certain SBAs in the feces of UC patients were significantly lower than those in healthy individuals.194 The decrease in SBAs levels can affect the expression of TJs and the renewal of intestinal stem cells, leading to damage to the intestinal barrier and subsequently exacerbating IBD.195 Therefore, the treatment of IBD may be achieved. By supplementing SBAs to activate the relevant key signaling pathways. For instance, LCA can enhance anti-inflammatory effects by modulating the TGR5-cAMP-PKA pathway, thereby preventing the activation of the NLRP3 inflammasome. Stimulation of TGR5 with LCA can trigger PKA kinase activity, leading to the activation of the TGR5-cAMP-PKA pathway, which subsequently results in the phosphorylation and ubiquitination of NLRP3.196 However, to date, the therapeutic systems based on SBAs still require development and refinement. This may be because the mechanisms of action of BAs in clinical studies are still under exploration. Several studies have demonstrated the therapeutic effect of SBAs in the treatment of IBD. This therapeutic effect relies on the activation of relevant signaling pathways and the expression of bile acid receptors, such as FXR, GPBAR1, PXR, VDR, and RORγt.197 However, agonists targeting specific bile acid receptors have yet to be developed, and the therapeutic potential of SBAs-based therapies remains understudied. The mechanism of action of SBAs in clinical studies is still being explored, which points the way forward for our future research and the development of therapeutic systems.
In summary, by constructing therapeutic systems to deliver metabolites of gut microbiota, can effectively enhance their bioavailability and better leverage the regulatory effects of gut microbiota.
3.5 Extracellular vesicles-based therapeutic systems
Extracellular vesicles (EVs) are nanometer-sized lipid bilayer-delimited particles secreted by almost all types of cells, including bacteria, mammals, and plants, and are considered to be mediators of intercellular communication.198 Based on their sources, EVs can be classified into bacterial extracellular vesicles (BEVs), animal extracellular vesicles (AEVs), and plant extracellular vesicles (PEVs). The size, composition, and cargo of EVs from different sources vary, enabling them to play unique roles in various biological processes that regulate the function and composition of the gut microbiota. PEVs are capable of carrying natural product molecules with excellent bioactivity, which exert immunoregulatory effects, facilitate tissue repair, and improve the growth environment of the gut microbiota.199 AEVs can target specific signaling pathways to reduce inflammation, strengthen tight junctions, and inhibit NF-κB-mediated apoptosis.200 BEVs undertake the task of signal communication between bacteria and among bacteria, as well as between bacteria and their hosts. Consequently, they can carry relevant functional molecules to directly impact the gut microbiota and influence its abundance.201 These three types of EVs can themselves serve as a therapeutic system, exerting significant effects on maintaining homeostasis, regulating immune responses, as well as the composition and function of the gut microbiota. Additionally, their excellent biocompatibility and low immunogenicity make them ideal biomaterials for drug delivery. In this section, we will provide a detailed introduction to these three types of EVs and their therapeutic systems.
3.5.1 Bacterial extracellular vesicles.
Bacterial extracellular vesicles (BEVs) are spherical nanostructures composed of a lipid bilayer secreted by bacteria, with a size range of 20 to 300 nm. By delivering these encapsulated bioactive molecules, BEVs interact with other microorganisms, host cells, and the host immune system, thereby influencing the microbial ecosystem and participating in the preservation of intestinal barrier function.198 In a healthy state, the secretion of BEVs is a natural phenomenon that plays a role in maintaining intestinal homeostasis. However, when IBD occurs, the BEVs secreted by the microbiota are disrupted, failing to provide normal protection to the intestine, thereby allowing the development of intestinal dysbiosis and its potential pathogenic consequences. Therefore, supplementing BEVs secreted by probiotics can effectively regulate the intestinal microbiota and treat IBD. The regulatory role of BEVs on the gut microbiota is primarily achieved through two mechanisms, the first of which is stimulating the restoration of the host's IEB. The EVs produced by Escherichia coli Nissle 1917 are capable of attaching to receptors on the surface of IECs, triggering the nucleotide-binding oligomerization domain 1 (NOD1) signaling pathway to enhance the expression of TJs, thereby restoring the IEB.202 Another mechanism is to foster symbiotic growth or, conversely, restrict the proliferation of pathogenic microorganisms through direct interaction with other bacteria. For instance, direct oral administration of EVs from Akkermansia muciniphila can directly promote the growth of probiotics and inhibit the proliferation of pathogenic bacteria. Hwa Seung Han et al. employed a tangential flow filtration system to isolate Roseburia intestinalis-derived EVs with a diameter of 76 nm from Roseburia intestinalis. They demonstrated that orally administered Roseburia intestinalis-derived EVs could effectively accumulate in inflamed colonic tissue and increase the abundance of Bifidobacteria (Fig. 14).203
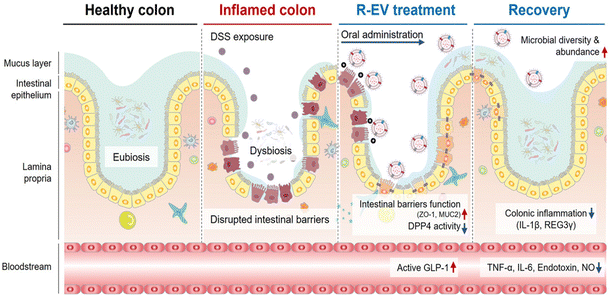 |
| Fig. 14 Schematic illustration of Roseburia-derived extracellular vesicles (R-EVs) as a critical mediator for DSS-induced IBD treatment. R-EVs exert amicable alterations in dysbiosis, reduce the inflammatory response, and restore the intestinal barrier in IBD. Reproduced from reference [H. S. Han, S. Hwang and S. Y. Choi et al., Roseburia intestinalis-derived extracellular vesicles ameliorate colitis by modulating intestinal barrier, microbiome, and inflammatory responses, J. Extracell. Vesicles, 2024, 13(8)] with permission from [Wiley], copyright [2024]. | |
3.5.2 Plant extracellular vesicles.
Plant extracellular vesicles (PEVs) are spherical lipid nanostructures secreted by plants, with a size range of 30 to 500 nm. As natural nanoparticles containing lipids, proteins, nucleic acids, and secondary metabolites, PEVs can exert anti-inflammatory activity and regulate gut microbiota by transporting these functional substances to receptor cells to mediate intercellular communication. A study has demonstrated that EVs derived from ginger can treat colitis by inhibiting the spread of inflammation, reducing the expression of proinflammatory cytokines, as well as increasing the levels of anti-inflammatory cytokines such as IL-10 and IL-22, thereby promoting the restoration of the IEB.204 EVs derived from grapefruit can be ingested by intestinal macrophages and inhibit the production of IL-1β and TNF-α in these macrophages by upregulating the expression of heme oxygenase-1 (HO-1), thereby improving dextran sulfate sodium (DSS)-induced colitis in mice. PEVs derived from tea have been shown to directly exert a positive influence on the diversity of the gut microbiota. These tea-derived PEVs have been identified to increase the ratio of Bacteroidetes/Firmicutes and enhance the overall abundance of fecal bacteria.205 In addition, PEVs have tremendous potential to serve as sustainable, green, and efficient nanocarriers for drug delivery.199 However, their application is still hindered by shortcomings such as low targeting efficiency and poor homogeneity. By integrating PEVs into a holistic therapeutic system, functionalizing them through surface modification and cargo loading, and enhancing their binding to specific target cells, their range of applications can be significantly expanded. For example, modifying PEVs through the insertion of polyethylene glycol (PEG)-lipids can prolong their circulation time in the blood, improve their stability, and effectively deliver them to the site of disease through enhanced permeability and retention (EPR) effects.206 Curcumin, which possesses potent anti-inflammatory activity, was encapsulated into tomato-derived EVs through direct incubation, ultrasonic treatment, and extrusion. In this therapeutic system, the tomato-derived EVs served as carriers for the transport of curcumin, significantly enhancing its anti-inflammatory activity.207
3.5.3 Animal extracellular vesicles.
Animal extracellular vesicles (AEVs) are spherical lipid nanostructures secreted by animals, with a size range of 30 to 1500 nm. The sources of AEVs include animal milk, IECs, immune cells, various bone marrow mesenchymal stem cells (MSCs), and others.208 AEVs from different cellular sources can exert positive regulatory effects on intestinal microbiota in various ways. EVs derived from raw milk can directly impact the gut microbiota by increasing the abundance of “beneficial” microorganisms such as Akkermansia, Muribaculum, and Turicibacter, while simultaneously decreasing the levels of the “harmful” bacterium Desulfovibrio.209 EVs produced by IECs exhibit TGF-β1-dependent immunosuppressive activity, and they induce regulatory T cells and immunosuppressive dendritic cells in an epithelial cell adhesion molecule-dependent manner to treat IBD.210 EVs derived from MSCs can inhibit the M1 polarization of macrophages and reduce the levels of inflammatory cytokines, thereby remodeling the intestinal microenvironment.211 Furthermore, as a therapeutic system, the phospholipid membrane structure of AEVs provides support for subsequent targeted modifications. By anchoring galactose to the surface of extracellular vesicles (EVs) loaded with IL-10 and secreted from genetically engineered mammalian cells (Gal-IL10-EVs), Jingang Liu et al. have endowed the EVs with the ability to target galactose-type lectins of macrophages on the surface of macrophages. The further modification of Gal-IL10-EVs with chitosan/alginate (C/A) granted them the ability to endure harsh gastric environments.212 Min Nie et al. have developed EVs derived from MSCs that carry IL-27 (MSCIL-27 EVs). Subsequently, they encapsulated the MSCIL-27 EVs into dopamine methacrylamide-modified hydrogels, enabling the MSCIL-27 EVs to achieve the desired sustained-release effect and effective wet adhesion properties. The therapeutic system based on MSCIL-27 EVs can firmly anchor onto the colonic surface, reduce inflammatory responses, and repair damaged barriers to treat IBD (Fig. 15).213
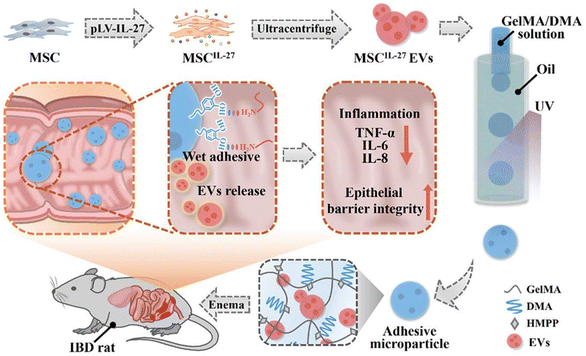 |
| Fig. 15 Schematics of the fabrication and application of the wet-adhesive microparticles with MSCIL-27 EVs loaded for IBD treatment. The MSCIL-27 EVs prepared via lentivirus-mediated gene transfection technology and ultracentrifuge were encapsulated into DMA-modified GelMA through a microfluidic method. Reproduced from reference [M. Nie, D. Huang, G. Chen, Y. Zhao and L. Sun, Bioadhesive Microcarriers Encapsulated with IL-27 High Expressive MSC Extracellular Vesicles for Inflammatory Bowel Disease Treatment, Adv. Sci., 2023, 10(32)] with permission from [Wiley], copyright [2023]. | |
In summary, EVs from different biological sources, as a therapeutic system, can regulate intestinal microbiota through various means. Surface modification of EVs enables them to overcome the gastric acid environment and achieve better targeting, thereby enhancing their therapeutic efficacy in treating IBD.
4 Other therapies related to NGMMs
In the preceding text, we summarized the impact of therapeutic systems based on NGMMs on the gut microbiota. Fecal microbiota transplantation (FMT) and synbiotics are traditional therapies that utilize microorganisms or active substances of natural origin with the ability to regulate intestinal microbiota for the treatment of IBD. Therefore, they are closely related to NGMMs. In this section, FMT and synbiotics are classified as therapies related to NGMMs, and introductions to them are provided.
4.1 Fecal microbiota transplantation
FMT is a therapy that involves collecting feces or fecal matter from a healthy donor and introducing it into the gastrointestinal tract of a patient to correct the ecological imbalance and restore health.214 The gut microbiota composition of IBD patients undergoes profound alterations, resulting in a severe disruption of the intestinal ecology. Consequently, FMT, which exerts its therapeutic effect by altering the gut microbiota composition, emerges as a promising treatment for IBD.215 Vaughn et al. investigated the therapeutic effect of FMT on CD and found that FMT increased the gut microbiota diversity in CD patients after treatment. In this study, clinical responses improved in 11 out of 19 patients (58%).216 Kunde et al. administered FMT via enema once daily for five consecutive days to nine patients with UC. Among these patients, seven experienced symptomatic relief just one week after the treatment.217 However, many studies have also reported severe side effects associated with FMT therapy in the treatment of IBD. Common side effects following FMT include fever and gastrointestinal symptoms (abdominal discomfort, flatulence, diarrhea, constipation, and vomiting).218 The potential reason for such outcomes may be that exposure to the new microbiota can provoke a disproportionate immune response in the host towards the transplanted microbiota. The immune response of the host to FMT may lead to an exacerbation of IBD disease activity and further side effects.219 Furthermore, the clinical administration of FMT via enema significantly reduces patient compliance, which to some extent limits its application. To address these issues, Shuang Zhen et al. constructed alginate microspheres for the encapsulation of fecal microbiota. They found that this therapeutic system exhibited controlled release capabilities, capable of protecting the fecal microbiota from the effects of gastric acid and digestive enzymes, thereby effectively treating IBD.220
4.2 Synbiotics
Synbiotics is a therapy that combines probiotics and prebiotics, exerting synergistic beneficial effects on the host's health.53 Specifically, this synergistic effect refers to the fact that probiotics can directly regulate the balance of the intestinal ecology and increase the abundance of beneficial bacteria, while prebiotics provide energy for the growth and reproduction of probiotics, thereby enhancing their activity.221 Using synbiotics to regulate gut microbiota for the treatment of IBD has emerged as a promising approach. Based on the mechanism by which synbiotics exert their effects, Kolida and Gibson have categorized synbiotics into two types: complementary synbiotics and synergistic synbiotics.222 Among the synbiotics commonly used for the treatment of colitis, the most prevalent is the complementary synbiotic, which is defined as a mixture of probiotics and prebiotics that are selected to function individually to improve IBD. For instance, Steed et al. found that a mixture of inulin and Bifidobacterium longum (FOS) can effectively improve the abundance of gut microbiota in patients with CD.223 In this case, inulin and Bifidobacterium serve as a complementary synbiotic. However, a limitation of this therapy is that the prebiotic does not selectively promote the growth of the supplemented probiotic. Instead, the presence of the prebiotic increases the overall abundance of gut microbiota rather than specifically targeting the supplemented probiotic. Therefore, there is a need for a method to enhance the efficacy of multiple probiotics and prebiotic species in combination with complementary synbiotics. Synergistic synbiotics provide a direction for overcoming the limitations of synbiotic therapy. Synergistic synbiotics refer to the supplementation of prebiotics that selectively stimulate the growth and reproduction of the supplemented probiotics, thereby facilitating the colonization of the supplemented probiotics in the intestine.224 For instance, Yujie Zhang has developed a therapeutic system utilizing multifunctional inulin/trans-ferulic acid/silk protein nanoparticles to load Bifidobacterium. In this therapeutic system, inulin serves as a prebiotic, promoting the rapid proliferation of Bifidobacterium and enabling it to exert its effects within a short period of time.225 In this therapeutic system, inulin and Bifidobacterium function in a synergistic way. The presence of inulin selectively promotes the colonization of Bifidobacterium in the intestine, enabling it to exert its effects more effectively.
In summary, FMT and synbiotics have shown great potential in treating IBD by regulating the gut microbiota. However, the toxic and side effects of FMT and the low synergism of complementary synbiotics have limited their further application. Nevertheless, therapeutic systems based on these two therapies can effectively address their limitations in the application process, helping them to maximize their therapeutic efficacy.
5 Conclusions and prospects
NGMMs, due to their widespread sources, ease of access, and excellent ability to regulate gut microbiota, have made significant progress in the treatment of IBD. However, their application is hindered by their inherent chemical properties or the complex environment of the gut. By constructing therapeutic systems based on NGMMs, it is possible to overcome the limitations of solubility, half-life, the harsh gastric environment, and the complex inflammatory gut environment, thereby enhancing their ability to regulate gut microbiota. However, this does not mean that they are perfect, nor can they permanently resolve all the issues encountered in the treatment of IBD through modulation of the gut microbiota. Next, we will separately introduce the current issues and suggest the focus of future work for each of them.
(1) Safety: the majority of validations concerning the regulatory effects of phytonutrients on gut microbiota rely heavily on animal models, and their safety has yet to be clinically verified. Although probiotics are currently used in clinical treatment, there have been reports of potential side effects such as infections and allergic reactions. For instance, Akkermansia muciniphila benefits the host by degrading mucin in the mucus layer, but the degradation of mucin may exacerbate IBD. Alterations in specific genes of genetically engineered probiotics may accumulate during their growth and reproduction, potentially evolving into superbugs. Large doses of prebiotics exert an osmotic effect in the intestinal lumen and undergo fermentation in the colon, which may cause gaseousness and bloating. Although gut microbiota metabolites can effectively regulate the gut microbiota, they may also have adverse effects on the human body, and determining the appropriate dosage of these metabolites poses a difficult challenge.
(2) Molecular mechanisms: the interaction between phytonutrients and gut microbiota still requires further research, particularly in areas such as how phytonutrients exert their regulatory effects on gut microbiota, how gut microbiota metabolize phytonutrients, and what effects their metabolites have on the host. Additionally, although articles have reported the positive regulatory role of SBAs in IBD, the molecular mechanisms underlying their function still require further exploration to unlock their therapeutic potential and lay the foundation for the development of SBA-based therapeutic systems.
(3) Preparation process: for NGMMs in compound form, including phytonutrients, prebiotics, and gut microbiota metabolites, their solubility in water is poor. Therefore, during the encapsulation process of constructing therapeutic systems, they need to be dissolved in organic solvents. However, completely removing these organic solvents during subsequent preparation steps often poses a challenge. Residual organic solvents may potentially impact the therapeutic process and even cause toxic or adverse effects on patients. For probiotic-based therapeutic systems, the current main encapsulation methods include extrusion and self-assembly. The extrusion process can affect the vitality of probiotics, while the self-assembly, generally conducted under relatively mild conditions, will not affect the vitality of probiotics. However, this encapsulation method relies on electrostatic interactions and may face the risk of leakage in the complex digestive tract environment after oral administration.
(4) Individual variability: different IBD patients have varying compositions of gut microbiota and varying severities of the disease. As a result, they may have different reactions to the same probiotics and prebiotics. Even for the same patient, their reactions may vary at different stages of IBD.
To fully leverage the immense potential of NGMMs and their therapeutic systems in treating IBD, it is imperative to conduct in-depth exploration and practical application targeting the aforementioned issues of safety, individual differences, drug dependence, manufacturing processes, and encapsulation efficiency in the future. Here are some possible solutions and research priorities.
(1) Conduct in-depth clinical research to verify safety: for phytonutrients that have demonstrated therapeutic effects, the research focus should gradually shift from animal models to the clinical field. This necessitates conducting more extensive and in-depth clinical trials to comprehensively evaluate their safety across different populations, such as healthy individuals and patients with IBD. As for probiotics and prebiotics, given their existing clinical applications, it is imperative that we carefully screen and identify potential allergens before patients adopt these therapies. Furthermore, pharmacodynamic experiments regarding NGMMs should also be conducted to determine the selectivity and appropriate dosage of NGMMs for different populations.
(2) More mechanistic studies need to be conducted: specifically, further research is needed on the interaction between phytonutrients and gut microbiota, how phytonutrients exert their regulatory effects on gut microbiota and the impact of downstream signaling pathways triggered by the activation of bile acid receptors by SBAs on inflammation and gut microbiota metabolism.
(3) Optimization of the preparation process: for hydrophobic NGMMs, in the process of constructing therapeutic systems, preference should be given to low-toxicity, non-toxic, or biocompatible organic solvents such as ethanol and glycerin, to reduce potential threats to patient health. Rigorous toxicity assessments should be conducted on the solvents used during the preparation process to ensure that the solvent residue levels are within safe limits. For probiotic-based therapeutic systems, since encapsulation strategies based on physical extrusion and electrostatic interactions may lead to decreased probiotic activity and leakage of probiotics, our future research focus should shift towards chemical modification and biological modification under mild conditions. Encapsulating probiotics through chemical forces or relying on metabolic processes of probiotics (such as biofilm formation and self-oxidation of surfaces) can better maintain their viability and improve encapsulation efficiency. Furthermore, from a technical perspective, the emergence of novel encapsulation technologies such as microfluidic technology offers brand-new options for achieving efficient and safe encapsulation of probiotics.
(4) Developing personalized treatment plans: due to the varying compositions of intestinal microbiota and metabolic characteristics among different patients, it is crucial to develop personalized treatment plans. This involves selecting appropriate types and doses of NGMMs based on the specific circumstances of each patient. During the treatment process, it is essential to regularly monitor changes in the patient's intestinal microbiota, health status, and any potential side effects, to promptly adjust the treatment plan as necessary.
NGMMs have demonstrated significant regulatory capabilities for gut microbiota, and their natural origin and ease of access grant them a unique advantage in the treatment of IBD. The development of therapeutic systems based on NGMMs can maximize their efficacy and overcome the limitations of using NGMMs in IBD treatment. In the future, the therapeutic system based on NGMMs should continue to evolve towards greater safety and efficacy. The current limitations faced by NGMMs will be addressed with deeper research into their mechanisms and the development of novel encapsulation technologies. The therapeutic systems based on NGMMs hold promise as a novel option for treating IBD through modulation of the gut microbiota.
Data availability
No primary research results, software or code have been included and no new data were generated or analysed as part of this review.
Conflicts of interest
There are no conflicts to declare.
Acknowledgements
This work was supported financially by the Fundamental Research Funds for the Central Universities (no. xzy012023097). The authors thank the Instrument Analysis Center of Xi’an Jiaotong University for the help and funding (subsidy fund for the operation of large instruments and equipment, no. YB2024227) on the characterization.
References
- S. C. Ng, H. Y. Shi and N. Hamidi,
et al., Worldwide incidence and prevalence of inflammatory bowel disease in the 21st century: a systematic review of population-based studies, Lancet, 2017, 390(10114), 2769–2778 CrossRef PubMed.
- J. Burisch, M. Zhao and S. Odes,
et al., The cost of inflammatory bowel disease in high-income settings: a Lancet Gastroenterology & Hepatology Commission, Lancet Gastroenterol. Hepatol., 2023, 8(5), 458–492 CrossRef CAS PubMed.
- H. R. Guo, H. Guo and Y. Xie,
et al., Mo3Se4 nanoparticle with ROS scavenging and multi-enzyme activity for the treatment of DSS-induced colitis in mice, Redox Biol., 2022, 56, 102441 CrossRef CAS PubMed.
- Y. Shan, M. Lee and E. B. Chang, The Gut Microbiome and Inflammatory Bowel Diseases, Annu. Rev. Med., 2022, 73, 455–468 CrossRef CAS PubMed.
- B. Bressler, J. K. Marshall and C. N. Bernstein,
et al., Toronto Ulcerative Colitis Consensus Group. Clinical Practice Guidelines for the Medical Management of Nonhospitalized Ulcerative Colitis: The Toronto Consensus, Gastroenterology, 2015, 148(5), 1035–1058.e3 CrossRef PubMed.
- A. Stallmach, S. Hagel and T. Bruns, Adverse effects of biologics used for treating IBD, Best Pract. Res., Clin. Gastroenterol., 2010, 24(2), 167–182 CrossRef CAS PubMed.
- F. Sommer and F. Backhed, The gut microbiota – masters of host development and physiology, Nat. Rev. Microbiol., 2013, 11(4), 227–238 CrossRef CAS PubMed.
- K. L. Adair and A. E. Douglas, Making a microbiome: the many determinants of host-associated microbial community composition, Curr. Opin. Microbiol., 2017, 35, 23–29 CrossRef PubMed.
- P. B. Eckburg, E. M. Bik and C. N. Bernstein,
et al., Diversity of the human intestinal microbial flora, Science, 2005, 308(5728), 1635–1638 CrossRef PubMed.
- K. L. Glassner, B. P. Abraham and E. M. M. Quigley, The microbiome and inflammatory bowel disease, J. Allergy Clin. Immunol., 2020, 145(1), 16–27 CrossRef CAS PubMed.
- B. Xia, X. N. Liu and Z. Q. Li,
et al., The effects of microbiota-targeted approaches in inflammatory bowel disease: probiotics, probiotic foods, and prebiotics, Curr. Opin. Food Sci., 2023, 49, 100956 CrossRef.
- C. Rodriguez, E. Romero and L. Garrido-Sanchez,
et al., Microbiota insights in Clostridium difficile infection and inflammatory bowel disease, Gut Microbes, 2020, 12(1), 1725220 CrossRef CAS PubMed.
- M. J. Tang, C. H. Wang and Y. Xia,
et al., Clostridioides difficile infection in inflammatory bowel disease: a clinical review, Expert Rev. Anti-Infect. Ther., 2024, 22(5), 297–306 CrossRef CAS PubMed.
- K. E. Orrell and R. A. Melnyk, Large Clostridial Toxins: Mechanisms and Roles in Disease, Microbiol. Mol. Biol. Rev., 2021, 85(3), e0006421 CrossRef PubMed.
- J. Ge, B. Jia and Y. Wang,
et al., DNA Nanostructures Treat Inflammatory Bowel Disease through ROS Scavenging and Gut Microbiota Modulation, Adv. Funct. Mater., 2024, 34(38), 202402781 CrossRef.
- L. Wang, M. Gao and G. Kang,
et al., The Potential Role of Phytonutrients Flavonoids Influencing Gut Microbiota in the Prophylaxis and Treatment of Inflammatory Bowel Disease, Front. Nutr., 2021, 8, 798038 CrossRef PubMed.
- Y. Li, Y. Wang and Y. Li,
et al., Dress me an outfit: advanced probiotics hybrid systems for intelligent IBD therapy, Crit. Rev. Food Sci. Nutr., 2024, 1–24 Search PubMed.
- S. Deleu, K. Machiels and J. Raes,
et al., Short chain fatty acids and its producing organisms: an overlooked therapy for IBD?, Ebiomedicine, 2021, 66, 103293 CrossRef CAS PubMed.
- X. Liang, N. Dai and K. Sheng,
et al., Gut bacterial extracellular vesicles: important players in regulating intestinal microenvironment, Gut Microbes, 2022, 14(1), 2134689 CrossRef PubMed.
- M. Zhang, X. Zhang and J. Zhu,
et al., Bidirectional interaction of nobiletin and gut microbiota in mice fed with a high-fat diet, Food Funct., 2021, 12(8), 3516–3526 RSC.
- Y. Xia, Y. Chen and G. Wang,
et al., Lactobacillus plantarum AR113 alleviates DSS-induced colitis by regulating the TLR4/MyD88/NF-κB pathway and gut microbiota composition, J. Funct. Foods, 2020, 67, 103854 CrossRef CAS.
- L. Pan, T. Fu and H. Cheng,
et al., Polysaccharide from edible alga Gloiopeltis furcata attenuates intestinal mucosal damage by therapeutically remodeling the interactions between gut microbiota and mucin O-glycans, Carbohydr. Polym., 2022, 278, 118921 CrossRef CAS PubMed.
- M. Li, Y. Ding and J. Wei,
et al., Gut microbiota metabolite indole-3-acetic acid maintains intestinal epithelial homeostasis through mucin sulfation, Gut Microbes, 2024, 16(1), 2377576 CrossRef PubMed.
- Y. Olivo-Martinez, S. Martinez-Ruiz and C. Cordero-Alday,
et al., Modulation of Serotonin-Related Genes by Extracellular Vesicles of the Probiotic Escherichia coli Nissle 1917 in the Interleukin-1β-Induced Inflammation Model of Intestinal Epithelial Cells, Int. J. Mol. Sci., 2024, 25(10), 5338 CrossRef CAS PubMed.
- M. Lesjak, I. Beara and N. Simin,
et al., Antioxidant and anti-inflammatory activities of quercetin and its derivatives, J. Funct. Foods, 2018, 40, 68–75 CrossRef CAS.
- R. C. Vazquez-Juarez, J. A. Kuriakose and D. A. Rasko,
et al., CadA Negatively Regulates Escherichia coli O157:H7 Adherence and Intestinal Colonization, Infect. Immun., 2008, 76(11), 5072–5081 CrossRef CAS PubMed.
- T. M. Cantu-Jungles, G. E. do Nascimento and X. Zhang,
et al., Soluble xyloglucan generates bigger bacterial community shifts than pectic polymers during in vitro fecal fermentation, Carbohydr. Polym., 2019, 206, 389–395 CrossRef PubMed.
- L. Serpe, R. Canaparo and M. Daperno,
et al., Solid lipid nanoparticles as anti-inflammatory drug delivery system in a human inflammatory bowel disease whole-blood model, Eur. J. Pharm. Sci., 2010, 39(5), 428–436 CrossRef CAS PubMed.
- R. Yadav, V. Kumar and M. Baweja,
et al., Gene editing and genetic engineering approaches for advanced probiotics: a review, Crit. Rev. Food Sci. Nutr., 2018, 58(10), 1735–1746 CrossRef CAS PubMed.
- Y. Zhou, X. Feng and H. Xu,
et al., The application of natural product-delivering micro/nano systems in the treatment of inflammatory bowel disease, J. Mater. Chem. B, 2023, 11(2), 244–260 RSC.
- R. Chen, X. Lin and Q. Wang,
et al., Dual-targeting celecoxib nanoparticles protect intestinal epithelium and regulate macrophage polarization for ulcerative colitis treatment, Chem. Eng. J., 2023, 452, 139445 CrossRef CAS.
- W. Fu, L. Xu and Z. Chen,
et al., Recent advances on emerging nanomaterials for diagnosis and treatment of inflammatory bowel disease, J. Controlled Release, 2023, 363, 149–179 CrossRef CAS PubMed.
- Z. Chu, W. Wang and W. Zheng,
et al., Biomaterials with cancer cell-specific cytotoxicity: challenges and perspectives, Chem. Soc. Rev., 2024, 53(17), 8847–8877 RSC.
- S. Yuan, K.-S. Wang and H. Meng,
et al., The gut microbes in inflammatory bowel disease: future novel target option for pharmacotherapy, Biomed. Pharmacother., 2023, 165, 114893 CrossRef CAS PubMed.
- A. M. O'Hara and F. Shanahan, The gut flora as a forgotten organ, EMBO Rep., 2006, 7(7), 688–693 CrossRef PubMed.
- L. Chen and J. Wang, Gut microbiota and inflammatory bowel disease, Wires Mech. Disease, 2022, 14(2), e1540 CrossRef CAS PubMed.
- D. Parada Venegas, M. K. De la Fuente and G. Landskron,
et al., Short Chain Fatty Acids (SCFAs)-Mediated Gut Epithelial and Immune Regulation and Its Relevance for Inflammatory Bowel Diseases, Front. Immunol., 2019, 10, 277 CrossRef PubMed.
- W. Yang and Y. Cong, Gut microbiota-derived metabolites in the regulation of host immune responses and immune-related inflammatory diseases, Cell. Mol. Immunol., 2021, 18(4), 866–877 CrossRef CAS PubMed.
- G. Cammarota, G. Ianiro and R. Cianci,
et al., The involvement of gut microbiota in inflammatory bowel disease pathogenesis: potential for therapy, Pharmacol. Ther., 2015, 149, 191–212 CrossRef CAS PubMed.
- E. A. Franzosa, A. Sirota-Madi and J. Avila-Pacheco,
et al., Gut microbiome structure and metabolic activity in inflammatory bowel disease, Nat. Microbiol., 2019, 4(5), 898 CrossRef CAS PubMed.
- H. Pandey, D. Jain and D. W. T. Tang,
et al., Gut microbiota in pathophysiology, diagnosis, and therapeutics of inflammatory bowel disease, Intest. Res., 2024, 22(1), 15–43 CrossRef PubMed.
- N. Kamada, G. Chen and G. Nunez, Harnessing pathogen-commensal relations, Nat. Med., 2012, 18(8), 1190–1191 CrossRef CAS PubMed.
- S. Liu, W. J. Zhao and P. Lan,
et al., The microbiome in inflammatory bowel diseases: from pathogenesis to therapy, Protein Cell, 2021, 12(5), 331–345 CrossRef PubMed.
- Q. Ren, S. Sun and X.-D. Zhang, Redox-active nanoparticles for inflammatory bowel disease, Nano Res., 2021, 14(8), 2535–2557 CrossRef CAS.
- M. Martinez-Medina and L. J. Garcia-Gil,
Escherichia coli in chronic inflammatory bowel diseases: an update on adherent invasive Escherichia coli pathogenicity, World J. Gastrointest. Pathophysiol., 2014, 5(3), 213–227 CrossRef PubMed.
- P. He, L. Yu and F. Tian,
et al., Dietary Patterns and Gut Microbiota: The Crucial Actors in Inflammatory Bowel Disease, Adv. Nutr., 2022, 13(5), 1628–1651 CrossRef CAS PubMed.
- T. S. Postler and S. Ghosh, Understanding the Holobiont: How Microbial Metabolites Affect Human Health and Shape the Immune System, Cell Metab., 2017, 26(1), 110–130 CrossRef CAS PubMed.
- J. Lloyd-Price, C. Arze and A. N. Ananthakrishnan,
et al., Multi-omics of the gut microbial ecosystem in inflammatory bowel diseases, Nature, 2019, 569(7758), 655–662 CrossRef CAS PubMed.
- M. Sun, N. Ma and T. He,
et al., Tryptophan (Trp) modulates gut homeostasis via aryl hydrocarbon receptor (AhR), Crit. Rev. Food Sci. Nutr., 2020, 60(10), 1760–1768 CrossRef CAS PubMed.
- A. Agus, J. Planchais and H. Sokol, Gut Microbiota Regulation of Tryptophan Metabolism in Health and Disease, Cell Host Microbe, 2018, 23(6), 716–724 CrossRef CAS PubMed.
- M. Levy, C. A. Thaiss and D. Zeevi,
et al., Microbiota-Modulated Metabolites Shape the Intestinal Microenvironment by Regulating NLRP6 Inflammasome Signaling, Cell, 2015, 163(6), 1428–1443 CrossRef CAS PubMed.
- S. Razavi, S. Janfaza and N. Tasnim,
et al., Microencapsulating polymers for probiotics delivery systems: preparation, characterization, and applications, Food Hydrocolloids, 2021, 120, 106882 CrossRef CAS.
- M. K. Yadav, I. Kumari and B. Singh,
et al., Probiotics, prebiotics and synbiotics: safe options for next-generation therapeutics, Appl. Microbiol. Biotechnol., 2022, 106(2), 505–521 CrossRef CAS PubMed.
- S. Putta, N. S. Yarla and K. E. Kumar,
et al., Preventive and Therapeutic Potentials of Anthocyanins in Diabetes and Associated Complications, Curr. Med. Chem., 2018, 25(39), 5347–5371 CrossRef CAS PubMed.
- I. N. Beara, M. M. Lesjak and D. Z. Orcic,
et al., Comparative analysis of phenolic profile, antioxidant, anti-inflammatory and cytotoxic activity of two closely-related Plantain species: Plantago altissima L. and Plantago lanceolata L, LWT, 2012, 47(1), 64–70 CrossRef CAS.
- H. Higashino, A. Karatsu and T. Masuda, Catalytic Antioxidant Activity of Two Diterpenoid Polyphenols of Rosemary, Carnosol, and Isorosmanol, against Lipid Oxidation in the Presence of Cysteine Thiol, J. Agric. Food Chem., 2024, 72(4), 2193–2201 CrossRef CAS PubMed.
- J.-C. Xue, S. Yuan and X.-T. Hou,
et al., Natural products modulate NLRP3 in ulcerative colitis, Front. Pharmacol., 2023, 14, 1265825 CrossRef CAS PubMed.
- Y. Feng, M. Pan and R. Li,
et al., Recent developments and new directions in the use of natural products for the treatment of inflammatory bowel disease, Phytomedicine, 2024, 132, 155812 CrossRef CAS PubMed.
- A. G. Geagea, M. Rizzo and A. Eid,
et al., Tea Catechins Induce Crosstalk between Signaling Pathways and Stabilize Mast Cells in Ulcerative Colitis, J. Biol. Regul. Homeostatic Agents, 2017, 31(4), 865–877 CAS.
- Y. Du, H. Ding and K. Vanarsa,
et al., Low Dose Epigallocatechin Gallate Alleviates Experimental Colitis by Subduing Inflammatory Cells and Cytokines, and Improving Intestinal Permeability, Nutrients, 2019, 11(8), 1743 CrossRef CAS PubMed.
- D. Han, Y. Wu and D. Lu,
et al., Polyphenol-rich diet mediates interplay between macrophage-neutrophil and gut microbiota to alleviate intestinal inflammation, Cell Death Dis., 2023, 14(10), 656 CrossRef CAS PubMed.
- M. Hu, Y. Y. Huang and X. Q. Du,
et al., The synergistic effect of epigallocatechin-3-gallate and quercetin co-loaded hydrogel beads on inflammatory bowel disease, Food Funct., 2023, 14(10), 4539–4551 RSC.
- Z. Wang, K.-L. Lam and J. Hu,
et al., Chlorogenic acid alleviates obesity and modulates gut microbiota in high-fat-fed mice, Food Sci. Nutr., 2019, 7(2), 579–588 CrossRef CAS PubMed.
- H. F. Ding, J. H. Liu and Z. X. Chen,
et al., Protocatechuic acid alleviates TMAO-aggravated atherosclerosis via mitigating inflammation, regulating lipid metabolism, and reshaping gut microbiota, Food Funct., 2024, 15(2), 881–893 RSC.
- W. Fang, M. Jin and W. Qi,
et al., Caffeic acid combined with arabinoxylan or β-glucan attenuates diet-induced obesity in mice via modulation of gut microbiota and metabolites, Int. J. Biol. Macromol., 2024, 268, 131683 CrossRef CAS PubMed.
- B. Cao, M.-N. Zeng and F.-X. Hao,
et al., P-coumaric acid ameliorates Aβ25-35-induced brain damage in mice by modulating gut microbiota and serum metabolites, Biomed. Pharmacother., 2023, 168, 115825 CrossRef CAS PubMed.
- R. Lin, M. Piao and Y. Song, Dietary Quercetin Increases Colonic Microbial Diversity and Attenuates Colitis Severity in Citrobacter rodentium-Infected Mice, Front. Microbiol., 2019, 10, 1092 CrossRef PubMed.
- B. Chen, J. K. Luo and Y. H. Han,
et al., Dietary Tangeretin Alleviated Dextran Sulfate Sodium-Induced Colitis in Mice via Inhibiting Inflammatory Response, Restoring Intestinal Barrier Function, and Modulating Gut Microbiota, J. Agric. Food Chem., 2021, 69(27), 7663–7674 CrossRef CAS PubMed.
- X. Yan, Y. Zhai and W. Zhou,
et al., Intestinal Flora Mediates Antiobesity Effect of Rutin in High-Fat-Diet Mice, Mol. Nutr. Food Res., 2022, 66(14), 2100948 CrossRef CAS PubMed.
- X. Liu, R. Z. Sun and Z. Z. Li,
et al., Luteolin alleviates non-alcoholic fatty liver disease in rats via restoration of intestinal mucosal barrier damage and microbiota imbalance involving in gut-liver axis, Arch. Biochem. Biophys., 2021, 711, 109019 CrossRef CAS PubMed.
- X. Yang, Z. He and R. Hu,
et al., Dietary β-Carotene on Postpartum Uterine Recovery in Mice: Crosstalk Between Gut Microbiota and Inflammation, Front. Immunol., 2021, 12, 744425 CrossRef CAS PubMed.
- L. Zhu, Y. Song and H. Liu,
et al., Gut microbiota regulation and anti-inflammatory effect of β-carotene in dextran sulfate sodium-stimulated ulcerative colitis in rats, J. Food Sci., 2021, 86(5), 2118–2130 CrossRef CAS PubMed.
- B. Zhao, J. Wu and J. Li,
et al., Lycopene Alleviates DSS-Induced Colitis and Behavioral Disorders via Mediating Microbes-Gut-Brain Axis Balance, J. Agric. Food Chem., 2020, 68(13), 3963–3975 CrossRef CAS PubMed.
- L. Zhang, W. Cao and Y. Gao,
et al., Astaxanthin (ATX) enhances the intestinal mucosal functions in immunodeficient mice, Food Funct., 2020, 11(4), 3371–3381 RSC.
- M. Terasaki, A. Kubota and H. Kojima,
et al., Fucoxanthin and Colorectal Cancer Prevention, Cancers, 2021, 13(10), 2379 CrossRef CAS PubMed.
- K. Y. Liu, C. H. Nakatsu and Y. Jones-Hall,
et al., Vitamin E alpha- and gamma-tocopherol mitigate colitis, protect intestinal barrier function and modulate the gut microbiota in mice, Free Radical Biol. Med., 2021, 163, 180–189 CrossRef CAS PubMed.
- K. Y. Liu, Q. Y. Wang and C. H. Nakatsu,
et al., Combining gamma-tocopherol and aspirin synergistically suppresses colitis-associated colon tumorigenesis and modulates the gut microbiota in mice, and inhibits the growth of human colon cancer cells, Eur. J. Pharmacol., 2023, 946, 175656 CrossRef CAS PubMed.
- F. Zhang, Y. L. Zhou and H. T. Chen,
et al., Curcumin Alleviates DSS-Induced Anxiety-Like Behaviors via the Microbial-Brain-Gut Axis, Oxid. Med. Cell. Longevity, 2022, 2022, 6244757 Search PubMed.
- N. Kumar and V. Pruthi, Potential applications of ferulic acid from natural sources, Biotechnol. Rep., 2014, 4, 86–93 CrossRef PubMed.
- R. Kaur, A. Sood and D. K. Lang,
et al., Natural Products as Sources
of Multitarget Compounds: Advances in the Development of Ferulic Acid as Multitarget Therapeutic, Curr. Top. Med. Chem., 2022, 22(5), 347–365 CrossRef CAS PubMed.
- C. Zhao, J. Yang and M. Chen,
et al., Synthetic Lignin-Derived Therapeutic Nano Reagent as Intestinal pH-Sensitive Drug Carriers Capable of Bypassing the Gastric Acid Environment for Colitis Treatment, ACS Nano, 2023, 17(1), 811–824 CrossRef CAS PubMed.
- H. Sakurama, S. Kishino and Y. Uchibori,
et al., β-Glucuronidase from Lactobacillus brevis useful for baicalin hydrolysis belongs to glycoside hydrolase family 30, Appl. Microbiol. Biotechnol., 2014, 98(9), 4021–4032 CrossRef CAS PubMed.
- S. Hu, M. Zhao and W. Li,
et al., Preclinical evidence for quercetin against inflammatory bowel disease: a meta-analysis and systematic review, Inflammopharmacology, 2022, 30(6), 2035–2050 CrossRef CAS PubMed.
- H.-X. Zhang, Y.-Y. Li and Z.-J. Liu,
et al., Quercetin effectively improves LPS-induced intestinal inflammation, pyroptosis, and disruption of the barrier function through the TLR4/NF-κB/NLRP3 signaling pathway in vivo and in vitro, Food Nutr. Res., 2022, 66, 8948 CAS.
- V. F. Rodrigues, J. Elias-Oliveira and I. S. Pereira,
et al., Akkermansia muciniphila and Gut Immune System: A Good Friendship That Attenuates Inflammatory Bowel Disease, Obesity, and Diabetes, Front. Immunol., 2022, 13, 934695 CrossRef CAS PubMed.
- L. C. Wang, R. R. Fu and Y. Meng,
et al., pH Sensitive Quercetin Nanoparticles Ameliorate DSS-Induced Colitis in Mice by Colon-Specific Delivery, Mol. Nutr. Food Res., 2024, 68(2), e2300051 CrossRef PubMed.
- M. Bernabeu, S. M. T. Gharibzahedi and A. A. Ganaie,
et al., The potential modulation of gut microbiota and oxidative stress by dietary carotenoid pigments, Crit. Rev. Food Sci. Nutr., 2024, 64(33), 12555–12573 CrossRef CAS PubMed.
- C. Liu, S. Zhang and D. J. McClements,
et al., Design of Astaxanthin-Loaded Core-Shell Nanoparticles Consisting of Chitosan Oligosaccharides and Poly(lactic-co-glycolic acid): Enhancement of Water Solubility, Stability, and Bioavailability, J. Agric. Food Chem., 2019, 67(18), 5113–5121 CrossRef CAS PubMed.
- X. Zhang, W. Su and Y. Chen,
et al., Bi-functional astaxanthin macromolecular nanocarriers to alleviate dextran sodium sulfate-induced inflammatory bowel disease, Int. J. Biol. Macromol., 2024, 256, 128494 CrossRef CAS PubMed.
- Q. Jiang, Natural forms of vitamin E: metabolism, antioxidant, and anti-inflammatory activities and their role in disease prevention and therapy, Free Radical Biol. Med., 2014, 72, 76–90 CrossRef CAS PubMed.
- K. Nie, K. Ma and W. Luo,
et al., Roseburia intestinalis: A Beneficial Gut Organism From the Discoveries in Genus and Species, Front. Cell. Infect. Microbiol., 2021, 11, 757718 CrossRef CAS PubMed.
- C. Zheng, Y. Zhong and J. Xie,
et al., Bacteroides acidifaciens and its derived extracellular vesicles improve DSS-induced colitis, Front. Microbiol., 2023, 14, 1304232 CrossRef PubMed.
- M. Plaza-Oliver, A. Beloqui and M. J. Santander-Ortega,
et al., Ascorbyl-dipalmitate-stabilised nanoemulsions as a potential localised treatment of inflammatory bowel diseases, Int. J. Pharm., 2020, 586, 119533 CrossRef CAS PubMed.
- S. Misra, P. Pandey and H. N. Mishra, Novel approaches for co-encapsulation of probiotic bacteria with bioactive compounds, their health benefits and functional food product development: a review, Trends Food Sci. Technol., 2021, 109, 340–351 CrossRef CAS.
- J. T. Li, J. Song and Z. C. Deng,
et al., Robust reactive oxygen species modulator hitchhiking yeast microcapsules for colitis alleviation by trilogically intestinal microenvironment renovation, Bioact. Mater., 2024, 36, 203–220 CAS.
- C. Hill, F. Guarner and G. Reid,
et al., The International Scientific Association for Probiotics and Prebiotics consensus statement on the scope and appropriate use of the term probiotic, Nat. Rev. Gastroenterol. Hepatol., 2014, 11(8), 506–514 CrossRef PubMed.
- D. K. Govindarajan, N. Viswalingam and Y. Meganathan,
et al., Adherence patterns of Escherichia coli in the intestine and its role in pathogenesis, Med. Microecol., 2020, 5, 100025 CrossRef.
- M. Yao, J. Xie, H. Du and D. J. McClements,
et al., Progress in microencapsulation of probiotics: a review, Compr. Rev. Food Sci. Food Saf., 2020, 19(2), 857–874 CrossRef PubMed.
- J.-F. Sicard, G. Le Bihan and P. Vogeleer,
et al., Interactions of Intestinal Bacteria with Components of the Intestinal Mucus, Front. Cell. Infect. Microbiol., 2017, 7, 387 CrossRef PubMed.
- S. Asgari, A. Pourjavadi and T. R. Licht,
et al., Polymeric carriers for enhanced delivery of probiotics, Adv. Drug Delivery Rev., 2020, 161, 1–21 CrossRef PubMed.
- L. Kan, Z. Zheng and W. Fu,
et al., Recent progress on engineered micro/nanomaterials mediated modulation of gut microbiota for treating inflammatory bowel disease, J. Controlled Release, 2024, 370, 43–65 CrossRef CAS PubMed.
- M. Han, W. Lei and J. Liang,
et al., The single-cell modification strategies for probiotics delivery in inflammatory bowel disease: a review, Carbohydr. Polym., 2024, 324, 121472 CrossRef CAS PubMed.
- A. Xie, H. Ji and Z. Liu,
et al., Modified Prebiotic-Based “Shield” Armed Probiotics with Enhanced Resistance of Gastrointestinal Stresses and Prolonged Intestinal Retention for Synergistic Alleviation of Colitis, ACS Nano, 2023, 17(15), 14775–14791 CrossRef CAS PubMed.
- F. Centurion, S. Merhebi and M. Baharfar,
et al., Cell-Mediated Biointerfacial Phenolic Assembly for Probiotic Nano Encapsulation, Adv. Funct. Mater., 2022, 32(26), 2200775 CrossRef CAS.
- R. Wang, K. Guo and W. Zhang,
et al., Poly-γ-Glutamic Acid Microgel-Encapsulated Probiotics with Gastric Acid Resistance and Smart Inflammatory Factor Targeted Delivery Performance to Ameliorate Colitis, Adv. Funct. Mater., 2022, 32(26), 2113034 CrossRef CAS.
- T. Chen, W. Meng and Y. Li,
et al., Probiotics Armed with In Situ Mineralized Nanocatalysts and Targeted Biocoatings for Multipronged Treatment of Inflammatory Bowel Disease, Nano Lett., 2024, 24(24), 7321–7331 CrossRef CAS PubMed.
- L. M. Zhu, T. T. Yu and W. C. Wang,
et al., Responsively Degradable Nanoarmor-Assisted Super Resistance and Stable Colonization of Probiotics for Enhanced Inflammation-Targeted Delivery, Adv. Mater., 2024, 36(18), e2308728 CrossRef PubMed.
- Q.-X. Huang, J.-L. Liang and C.-H. Yang,
et al., Stimulation-responsive mucoadhesive probiotics for inflammatory bowel disease treatment by scavenging reactive oxygen species and regulating gut microbiota, Biomaterials, 2023, 301, 122274 CrossRef CAS PubMed.
- Y. Zhang, Y. Wang and X. Zhang,
et al., Gastrointestinal Self-Adaptive and Nutrient Self-Sufficient Akkermansia muciniphila-Gelatin Porous Microgels for Synergistic Therapy of Ulcerative Colitis, ACS Nano, 2024, 18(39), 26807–26827 CrossRef CAS PubMed.
- X. Y. Yang, J. L. Yang and Z. H. Ye,
et al., Physiologically Inspired Mucin Coated Escherichia coli Nissle 1917 Enhances Biotherapy by Regulating the Pathological Microenvironment to Improve Intestinal Colonization, ACS Nano, 2022, 16(3), 4041–4058 CrossRef CAS PubMed.
- X. Wang, Z. Cao and M. Zhang,
et al., Bioinspired oral delivery of gut microbiota by self-coating with biofilms, Sci. Adv., 2020, 6(26), eabb1952 CrossRef CAS PubMed.
- H. Ejima, J. J. Richardson and F. Caruso, Metal-phenolic networks as a versatile platform to engineer nanomaterials and biointerfaces, Nano Today, 2017, 12, 136–148 CrossRef CAS.
- W. Xie, Z. Guo and L. Zhao,
et al., Metal-phenolic networks: facile assembled complexes for cancer theranostics, Theranostics, 2021, 11(13), 6407–6426 CrossRef CAS PubMed.
- Z. Zhao, S. Xu and W. Zhang,
et al., Probiotic Escherichia coli NISSLE 1917 for inflammatory bowel disease applications, Food Funct., 2022, 13(11), 5914–5924 RSC.
- M. Sohail, Mudassir and M. U. Minhas,
et al., Natural and synthetic polymer-based smart biomaterials for management of ulcerative colitis: a review of recent developments and future prospects, Drug Delivery Transl. Res., 2019, 9(2), 595–614 CrossRef CAS PubMed.
- H. Li, J. Sun and H. Zhu,
et al., Recent advances in development of dendriticpolymer-basednanomedicines for cancer diagnosis, Wiley Interdiscip. Rev.: Nanomed. Nanobiotechnol., 2021, 13(2), e1670 CAS.
- Z. Tu, Y. Zhong and H. Hu,
et al., Design of therapeutic biomaterials to control inflammation, Nat. Rev. Mater., 2022, 7(7), 557–574 CrossRef CAS PubMed.
- M. Davaatseren, J. T. Hwang and J. H. Park,
et al., Poly-γ-Glutamic Acid Attenuates Angiogenesis and Inflammation in Experimental Colitis, Mediators Inflammation, 2013, 2013, 982383 Search PubMed.
- M. Tamura, C. Hoshi and Y. Kimura,
et al., Effects of γ-Polyglutamic Acid on the Cecal Microbiota and Visceral Fat in KK-AY/TaJcl Male Mice, Food Sci. Technol. Res., 2018, 24(1), 151–157 CrossRef CAS.
- F. Seidi, R. Jenjob and T. Phakkeeree,
et al., Saccharides, oligosaccharides, and polysaccharides nanoparticles for biomedical applications, J. Controlled Release, 2018, 284, 188–212 CrossRef CAS PubMed.
- W. Yang, P. Zhao and X. Li,
et al., The potential roles of natural plant polysaccharides in inflammatory bowel disease: a review, Carbohydr. Polym., 2022, 277, 118821 CrossRef CAS PubMed.
- M. Yu, B. Xiao and X. Hao,
et al., Pumpkin polysaccharide preparation, simulated gastrointestinal digestion, and in vivo biodistribution, Int. J. Biol. Macromol., 2019, 36(18), 1293–1303 CrossRef PubMed.
- S. Zhang, J. Ermann and M. D. Succi,
et al., An inflammation-targeting hydrogel for local drug delivery in inflammatory bowel disease, Sci. Trans. Med., 2015, 7(300), 300ra128 Search PubMed.
- D. E. Tylawsky, H. Kiguchi and J. Vaynshteyn,
et al., P-selectin-targeted nanocarriers induce active crossing of the blood-brain barrier via caveolin-1-dependent transcytosis, Nat. Mater., 2023, 22(3), 391–399 CrossRef CAS PubMed.
- G. Petrou and T. Crouzier, Mucins as multifunctional building blocks of biomaterials, Biomater. Sci., 2018, 6(9), 2282–2297 RSC.
- B. Demouveaux, V. Gouyer and F. Gottrand,
et al., Gel-forming mucin interactome drives mucus viscoelasticity, Adv. Colloid Interface Sci., 2018, 252, 69–82 CrossRef CAS PubMed.
- L. E. Davey, P. N. Malkus and M. Villa,
et al., A genetic system for Akkermansia muciniphila reveals a role for mucin foraging in gut colonization and host sterol biosynthesis gene expression, Nat. Microbiol., 2023, 8(8), 1450–1467 CrossRef CAS PubMed.
- J. Yan, L. Sheng and H. Li,
Akkermansia muciniphila: is it the Holy Grail for ameliorating metabolic diseases?, Gut Microbes, 2021, 13(1), 1984104 CrossRef PubMed.
- M. Tallawi, M. Opitz and O. Lieleg, Modulation of the mechanical properties of bacterial biofilms in response to environmental challenges, Biomater. Sci., 2017, 5(5), 887–900 RSC.
- W. Yin, Y. Wang and L. Liu,
et al., Biofilms: The Microbial “Protective Clothing” in Extreme Environments, Int. J. Mol. Sci., 2019, 20(14), 3423 CrossRef CAS PubMed.
- H.-C. Flemming, J. Wingender and U. Szewzyk,
et al., Biofilms: an emergent form of bacterial life, Nat. Rev. Microbiol., 2016, 14(9), 563–575 CrossRef CAS PubMed.
- J. Yan and B. L. Bassler, Surviving as a Community: Antibiotic Tolerance and Persistence in Bacterial Biofilms, Cell Host Microbe, 2019, 26(1), 15–21 CrossRef CAS PubMed.
- H. A. Hong, L. H. Duc and S. M. Cutting, The use of bacterial spore formers as probiotics, FEMS Microbiol. Rev., 2005, 29(4), 813–835 CrossRef CAS PubMed.
- B. Mielich-Suess and D. Lopez, Molecular mechanisms involved in Bacillus subtilis biofilm formation, Environ. Microbiol., 2015, 17(3), 555–565 CrossRef PubMed.
- J. H. Ma, Y. H. Lyu and X. Liu,
et al., Engineered probiotics, Microb. Cell Fact., 2022, 21(1), 72 CrossRef PubMed.
- J. Zhou, M. Y. Li and Q. F. Chen,
et al., Programmable probiotics modulate inflammation and gut microbiota for inflammatory bowel disease treatment after effective oral delivery, Nat. Commun., 2022, 13(1), 3432 CrossRef CAS PubMed.
- M. Idzko, D. Ferrari and H. K. Eltzschig, Nucleotide signalling during inflammation, Nature, 2014, 509(7500), 310–317 CrossRef CAS PubMed.
- K. Atarashi, J. Nishimura and T. Shima,
et al., ATP drives lamina propria T(H)17 cell differentiation, Nature, 2008, 455(7214), 808–812 CrossRef CAS PubMed.
- B. D. Gulbransen, M. Bashashati and S. A. Hirota,
et al., Activation of neuronal P2X7 receptor-pannexin-1 mediates death of enteric neurons during colitis, Nat. Med., 2012, 18(4), 600–604 CrossRef CAS PubMed.
- M. C. Takenaka, S. Robson and F. J. Quintana, Regulation of the T Cell Response by CD39, Trends Immunol., 2016, 37(7), 427–439 CrossRef CAS PubMed.
- B. M. Scott, C. Gutierrez-Vazquez and L. M. Sanmarco,
et al., Self-tunable engineered yeast probiotics for the treatment of inflammatory bowel disease, Nat. Med., 2021, 27(7), 1212–1222 CrossRef CAS PubMed.
- V. Vitvitsky, P. K. Yadav and A. Kurthen,
et al., Sulfide Oxidation by a Noncanonical Pathway in Red Blood Cells Generates Thiosulfate and Polysulfides, J. Biol. Chem., 2015, 290(13), 8310–8320 CrossRef CAS PubMed.
- F. Blachier, A.-M. Davila and S. Mimoun,
et al., Luminal sulfide and large intestine mucosa: friend or foe?, Amino Acids, 2010, 39(2), 335–347 CrossRef CAS PubMed.
- K. N. M. Daeffler, J. D. Galley and R. U. Sheth,
et al., Engineering bacterial thiosulfate and tetrathionate sensors for detecting gut inflammation, Mol. Syst. Biol., 2017, 13(4), 923 CrossRef PubMed.
- T.-T. Fang, Z.-P. Zou and Y. Zhou,
et al., Prebiotics-Controlled Disposable Engineered Bacteria for Intestinal Diseases, ACS Synth. Biol., 2022, 11(9), 3004–3014 CrossRef CAS PubMed.
- Z. P. Zou, Y. Du and T. T. Fang,
et al., Biomarker-responsive engineered probiotic diagnoses, records, and ameliorates inflammatory bowel disease in mice, Cell Host Microbe, 2023, 31(2), 199–212 CrossRef CAS PubMed.
- A. Holmer and S. Singh, Overall and comparative safety of biologic and immunosuppressive therapy in inflammatory bowel diseases, Expert Rev. Clin. Immunol., 2019, 15(9), 969–979 CrossRef CAS PubMed.
- S. Commins, J. W. Steinke and L. Borish, The extended IL-10 superfamily: IL-10, IL-19, IL-20, IL-22, IL-24, IL-26, IL-28, and IL-29, J. Allergy Clin. Immunol., 2008, 121(5), 1108–1111 CrossRef CAS PubMed.
- K. J. Chua, H. Ling and I. Y. Hwang,
et al., An Engineered Probiotic Produces a Type III Interferon IFNL1 and Reduces Inflammations in in vitro Inflammatory Bowel Disease Models, ACS Biomater. Sci. Eng., 2022, 9(9), 5123–5135 CrossRef PubMed.
- G. R. Gibson, R. Hutkins and M. E. Sanders,
et al., The International Scientific Association for Probiotics and Prebiotics (ISAPP) consensus statement on the definition and scope of prebiotics, Nat. Rev. Gastroenterol. Hepatol., 2017, 14(8), 491–502 CrossRef PubMed.
- S. M. Aravind, S. Wichienchot and R. Tsao,
et al., Role of dietary polyphenols on gut microbiota, their metabolites and health benefits, Food Res. Int., 2021, 142, 110189 CrossRef PubMed.
- C. Zhang, X. Pi and X. Li,
et al., Edible herbal source-derived polysaccharides as potential prebiotics: composition, structure, gut microbiota regulation, and its related health effects, Food Chem., 2024, 458, 140267 CrossRef CAS PubMed.
- B. Ren, K. Yue and Y. Zhang,
et al., Collagen-derived peptides as prebiotics to improve gut health, Curr. Opin. Food Sci., 2024, 55, 101123 CrossRef CAS.
- V. Kiron, M. Hayes and D. Avni, Inflammatory bowel disease-A peek into the bacterial community shift and algae-based ‘biotic’ approach to combat the disease, Trends Food Sci. Technol., 2022, 129, 210–220 CrossRef CAS.
- F. Turroni, M. Ventura and L. F. Butto,
et al., Molecular dialogue between the human gut microbiota and the host: a Lactobacillus and Bifidobacterium perspective, Cell. Mol. Life Sci., 2014, 71(2), 183–203 CrossRef CAS PubMed.
- S. A. Elbadri, M. S. Fathi and A. E. A. Hamid,
et al., The effect of Lactobacillus acidophilus as a probiotic against Pseudomonas aeruginosa growth and biofilm formation, Novel Res. Microbiol. J., 2019, 3(4), 428–439 CrossRef.
- E. A. Sawin, T. J. De Wolfe and B. Aktas,
et al., Glycomacropeptide is a prebiotic that reduces Desulfovibrio bacteria, increases cecal short-chain fatty acids, and is anti-inflammatory in mice, Am. J. Physiol.: Gastrointest. Liver Physiol., 2015, 309(7), G590–G601 CrossRef CAS PubMed.
- T. M. Cantu-Jungles, G. E. do Nascimento and X. W. Zhang,
et al., Soluble xyloglucan generates bigger bacterial community shifts than pectic polymers during in vitro fecal fermentation, Carbohydr. Polym., 2019, 206, 389–395 CrossRef PubMed.
- Y. Ren, L. Nie and C. Luo,
et al., Advancement in Therapeutic Intervention of Prebiotic-Based Nanoparticles for Colonic Diseases, Int. J. Nanomed., 2022, 17, 6639–6654 CrossRef CAS PubMed.
- X. Yan, Q. Zhou and J. Yu,
et al., Magnetite Nanostructured Porous Hollow Helical Microswimmers for Targeted Delivery, Adv. Funct. Mater., 2015, 25(33), 5333–5342 CrossRef CAS.
- G. Ling, P. Zhang and W. Zhang,
et al., Development of novel self-assembled DS-PLGA hybrid nanoparticles for improving oral bioavailability of vincristine sulfate by P-gp inhibition, J. Controlled Release, 2010, 148(2), 241–248 CrossRef CAS PubMed.
- X. J. Yan, C. H. Yang and M. Yang,
et al., All-in-one theranostic nano-platform based on polymer nanoparticles for BRET/FRET-initiated bioluminescence imaging and synergistically anti-inflammatory therapy for ulcerative colitis, J. Nanobiotechnol., 2022, 20(1), 99 CrossRef CAS PubMed.
- J. Xu, Q. Ma and Y. Zhang,
et al., Yeast-derived nanoparticles remodel the immunosuppressive microenvironment in tumor and tumor-draining lymph nodes to suppress tumor growth, Nat. Commun., 2022, 13(1), 110 CrossRef CAS PubMed.
- Y. Cao, Y. Sun and S. Zou,
et al., Yeast β-Glucan Suppresses the Chronic Inflammation and Improves the Microenvironment in Adipose Tissues of ob/ob Mice, J. Agric. Food Chem., 2018, 66(3), 621–629 CrossRef CAS PubMed.
- F. Yang, Y. Su and C. Yan,
et al., Attenuation of inflammatory bowel disease by oral administration of mucoadhesive polydopamine-coated yeast β-glucan via ROS scavenging and gut microbiota regulation, J. Nanobiotechnol., 2024, 22(1), 166 CrossRef CAS PubMed.
- S. Roy and S. Dhaneshwar, Role of prebiotics, probiotics, and synbiotics in management of inflammatory bowel disease: current perspectives, World J. Gastroenterol., 2023, 29(14), 2078–2100 CrossRef CAS PubMed.
- Z. Zhang, Y. Pan and Z. Guo,
et al., An olsalazine nanoneedle-embedded inulin hydrogel reshapes intestinal homeostasis in inflammatory bowel disease, Bioact. Mater., 2024, 33, 71–84 CAS.
- W. Akram, V. Pandey and R. Sharma,
et al., Inulin: unveiling its potential as a multifaceted biopolymer in prebiotics, drug delivery, and therapeutics, Int. J. Biol. Macromol., 2024, 259, 129131 CrossRef CAS PubMed.
- Q. Sun, L. Luan and M. Arif,
et al., Redox-sensitive nanoparticles based on 4-aminothiophenol-carboxymethyl inulin conjugate for budesonide delivery in inflammatory bowel diseases, Carbohydr. Polym., 2018, 189, 352–359 CrossRef CAS PubMed.
- A. Kusmayadi, Y. K. Leong and H.-W. Yen,
et al., Microalgae as sustainable food and feed sources for animals and humans – Biotechnological and environmental aspects, Chemosphere, 2021, 271, 129800 CrossRef CAS PubMed.
- F. Khavari, M. Saidijam and M. Taheri,
et al., Microalgae: therapeutic potentials and applications, Mol. Biol. Rep., 2021, 48(5), 4757–4765 CrossRef CAS PubMed.
- D. N. Zhong, D. X. Zhang and W. Chen,
et al., Orally deliverable strategy based on microalgal biomass for intestinal disease treatment, Sci. Adv., 2021, 7(48), eabi9265 CrossRef CAS PubMed.
- O. Yuliarti, Z. X. Lau and L. Wee,
et al., Enhancing the stability of oil-in-water emulsion using pectin-lactoferrin complexes, Int. J. Biol. Macromol., 2019, 139, 421–430 CrossRef CAS PubMed.
- Y. Wei, X. Luo and J. Guan,
et al., Biodegradable nanoparticles for improved kidney bioavailability of rhein: preparation, characterization, plasma, and kidney pharmacokinetics, Drug Dev. Ind. Pharm., 2017, 43(11), 1885–1891 CrossRef CAS PubMed.
- R. Luo, M. Lin and C. Fu,
et al., Calcium pectinate and hyaluronic acid modified lactoferrin nanoparticles loaded rhein with dual-targeting for ulcerative colitis treatment, Carbohydr. Polym., 2021, 263, 117998 CrossRef CAS PubMed.
- M. A. Oshi, J. Lee and J. Kim,
et al., pH-Responsive Alginate-Based Microparticles for Colon-Targeted Delivery of Pure Cyclosporine A Crystals to Treat Ulcerative Colitis, Pharmaceutics, 2021, 13(9), 1412 CrossRef CAS PubMed.
- X. Zhang, X. Zhao and Z. Hua,
et al., ROS-triggered self-disintegrating
and pH-responsive astaxanthin nanoparticles for regulating the intestinal barrier and colitis, Biomaterials, 2023, 292, 121937 CrossRef CAS PubMed.
- A. Lavelle and H. Sokol, Gut microbiota-derived metabolites as key actors in inflammatory bowel disease, Nat. Rev. Gastroenterol. Hepatol., 2020, 17(4), 223–237 CrossRef PubMed.
- D. Zhang, J. Liu and H. Cheng,
et al., Interactions between polysaccharides and gut microbiota: a metabolomic and microbial review, Food Res. Int., 2022, 160, 111653 CrossRef CAS PubMed.
- J. Liu, Y. Z. Tan and H. Cheng,
et al., Functions of Gut Microbiota Metabolites, Current Status and Future Perspectives, Aging Dis., 2022, 13(4), 1106–1126 CrossRef PubMed.
- Y. Pan, H. Zhang and M. Li,
et al., Novel approaches in IBD therapy: targeting the gut microbiota-bile acid axis, Gut Microbes, 2024, 16(1), 2356284 CrossRef PubMed.
- Z. Zhang, H. Zhang and T. Chen,
et al., Regulatory role of short-chain fatty acids in inflammatory bowel disease, Cell Commun. Signaling, 2022, 20(1), 64 CrossRef PubMed.
- B. Dalile, L. Van Oudenhove and B. Vervliet,
et al., The role of short-chain fatty acids in microbiota-gut-brain communication, Nat. Rev. Gastroenterol. Hepatol., 2019, 16(8), 461–478 CrossRef PubMed.
- J. H. Wu, H. Huang and L. N. Wang,
et al., A tailored series of engineered yeasts for the cell-dependent treatment of inflammatory bowel disease by rational butyrate supplementation, Gut Microbes, 2024, 16(1), 2316575 CrossRef PubMed.
- X. Fan, Z. Z. Zhang and W. X. Gao,
et al., An Engineered Butyrate-Derived Polymer Nanoplatform as a Mucosa-Healing Enhancer Potentiates the Therapeutic Effect of Magnolol in Inflammatory Bowel Disease, ACS Nano, 2023, 18(1), 229–244 CrossRef PubMed.
- M. Ala, Tryptophan metabolites modulate inflammatory bowel disease and colorectal cancer by affecting immune system, Int. Rev. Immunol., 2022, 41(3), 326–345 CrossRef CAS PubMed.
- F. Dong and G. H. Perdew, The aryl hydrocarbon receptor as a mediator of host-microbiota interplay, Gut Microbes, 2020, 12(1), 1859812 CrossRef PubMed.
- Y. Fu, H. Gao and X. Hou,
et al., Pretreatment with IPA ameliorates colitis in mice: colon transcriptome and fecal 16S amplicon profiling, Front. Immunol., 2022, 13, 1014881 CrossRef CAS PubMed.
- K. Yang, X. Wang and R. Huang,
et al., Prebiotics and Postbiotics Synergistic Delivery Microcapsules from Microfluidics for Treating Colitis, Adv. Sci., 2022, 9(16), e2104089 CrossRef PubMed.
- J. L. Shi, P. Du and Q. G. Xie,
et al., Protective effects of tryptophan-catabolizing Lactobacillus plantarum KLDS 1.0386 against dextran sodium sulfate-induced colitis in mice, Food Funct., 2020, 11(12), 10736–10747 RSC.
- Y. Y. Song, X. Y. Qu and M. Guo,
et al., Indole acetylated high-amylose maize starch: synthesis, characterization and application for amelioration of colitis, Carbohydr. Polym., 2023, 302, 120425 CrossRef CAS PubMed.
- A. Wahlstrom, S. I. Sayin and H.-U. Marschall,
et al., Intestinal Crosstalk between Bile Acids and Microbiota and Its Impact on Host Metabolism, Cell Metab., 2016, 24(1), 41–50 CrossRef PubMed.
- M. L. Chen, K. Takeda and M. S. Sundrud, Emerging roles of bile acids in mucosal immunity and inflammation, Mucosal Immunol., 2019, 12(4), 851–861 CrossRef CAS PubMed.
- Z.-H. Yang, F. Liu and X.-R. Zhu,
et al., Altered profiles of fecal bile acids correlate with gut microbiota and inflammatory responses in patients with ulcerative colitis, World J. Gastroenterol., 2021, 27(24), 3609–3629 CrossRef CAS PubMed.
- Y. Ma, H. Yang and X. Wang,
et al., Bile acids as signaling molecules in inflammatory bowel disease: implications for treatment strategies, J. Ethnopharmacol., 2025, 337, 118968 CrossRef CAS PubMed.
- C. Guo, S. Xie and Z. Chi,
et al., Bile Acids Control Inflammation and Metabolic Disorder through Inhibition of NLRP3 Inflammasome, Immunity, 2016, 45(4), 802–816 CrossRef CAS PubMed.
- S. Fiorucci, A. Carino and M. Baldoni,
et al., Bile Acid Signaling in Inflammatory Bowel Diseases, Dig. Dis. Sci., 2021, 66(3), 674–693 CrossRef CAS PubMed.
- J. Huang, X. Wang and Z. Wang,
et al., Extracellular vesicles as a novel mediator of interkingdom communication, Cytokine Growth Factor Rev., 2023, 73, 173–184 CrossRef CAS PubMed.
- M. Shao, X. Jin and S. Chen,
et al., Plant-derived extracellular vesicles-a novel clinical anti-inflammatory drug carrier worthy of investigation, Biomed. Pharmacother., 2023, 169, 115904 CrossRef CAS PubMed.
- M.-M. Guo, K. Zhang and J.-H. Zhang, Human Breast Milk-Derived Exosomal miR-148a-3p Protects Against Necrotizing Enterocolitis by Regulating p53 and Sirtuin 1, Inflammation, 2022, 45(3), 1254–1268 CrossRef CAS PubMed.
- T. Teame, A. Wang and M. Xie,
et al., Paraprobiotics and Postbiotics of Probiotic Lactobacilli, Their Positive Effects on the Host and Action Mechanisms: A Review, Front. Nutr., 2020, 7, 570344 CrossRef PubMed.
- Y. Olivo-Martinez, S. Martinez-Ruiz and C. Cordero,
et al., Extracellular Vesicles of the Probiotic Escherichia coli Nissle 1917 Reduce PepT1 Levels in IL-1β-Treated Caco-2 Cells via Upregulation of miR-193a-3p, Nutrients, 2024, 16(16), 2719 CrossRef CAS PubMed.
- H. S. Han, S. Hwang and S. Y. Choi,
et al., Roseburia intestinalis-derived extracellular vesicles ameliorate colitis by modulating intestinal barrier, microbiome, and inflammatory responses, J. Extracell. Vesicles, 2024, 13(8), e12487 CrossRef CAS PubMed.
- M. Zhang, E. Viennois and M. Prasad,
et al., Edible ginger-derived nanoparticles: a novel therapeutic approach for the prevention and treatment of inflammatory bowel disease and colitis-associated cancer, Biomaterials, 2016, 101, 321–340 CrossRef CAS PubMed.
- Q. Chen, M. Zu and H. Gong,
et al., Tea leaf-derived exosome-like nanotherapeutics retard breast tumor growth by pro-apoptosis and microbiota modulation, J. Nanobiotechnol., 2023, 21(1), 6 CrossRef CAS PubMed.
- D. Sasaki, K. Kusamori and M. Nishikawa, Delivery of Corn-Derived Nanoparticles with Anticancer Activity to Tumor Tissues by Modification with Polyethylene Glycol for Cancer Therapy, Pharm. Res., 2023, 40(4), 917–926 CrossRef CAS PubMed.
- R. Mammadova, S. Maggio and I. Fiume,
et al., Protein Biocargo and Anti-Inflammatory Effect of Tomato Fruit-Derived Nanovesicles Separated by Density Gradient Ultracentrifugation and Loaded with Curcumin, Pharmaceutics, 2023, 15(2), 333 CrossRef CAS PubMed.
- L. Chen, Q. Ou and X. Kou, Extracellular vesicles and their indispensable roles in pathogenesis and treatment of inflammatory bowel disease: a comprehensive review, Life Sci., 2023, 327, 121830 CrossRef CAS PubMed.
- C. Du, S. Quan and X. Nan,
et al., Effects of oral milk extracellular vesicles on the gut microbiome and serum metabolome in mice, Food Funct., 2021, 12(21), 10938–10949 RSC.
- L. Jiang, Y. Shen and D. Guo,
et al., EpCAM-dependent extracellular vesicles from intestinal epithelial cells maintain intestinal tract immune balance, Nat. Commun., 2016, 7, 13045 CrossRef CAS PubMed.
- M. Arabpour, A. Saghazadeh and N. Rezaei, Anti-inflammatory and M2 macrophage polarization-promoting effect of mesenchymal stem cell-derived exosomes, Int. Immunopharmacol., 2021, 97, 107823 CrossRef CAS PubMed.
- J. Liu, H. Ren and C. Zhang,
et al., Orally-Delivered, Cytokine-Engineered Extracellular Vesicles for Targeted Treatment of Inflammatory Bowel Disease, Small, 2023, 19(50), e2304023 CrossRef PubMed.
- M. Nie, D. Huang and G. Chen,
et al., Bioadhesive Microcarriers Encapsulated with IL-27 High Expressive MSC Extracellular Vesicles for Inflammatory Bowel Disease Treatment, Adv. Sci., 2023, 10(32), e2303349 CrossRef PubMed.
- T. Sunkara, P. Rawla and A. Ofosu,
et al., Fecal microbiota transplant – a new frontier in inflammatory bowel disease, J. Inflammation Res., 2018, 11, 321–328 CrossRef CAS PubMed.
- A. R. Weingarden and B. P. Vaughn, Intestinal microbiota, fecal microbiota transplantation, and inflammatory bowel disease, Gut Microbes, 2017, 8(3), 238–252 CrossRef PubMed.
- B. P. Vaughn, T. Vatanen and J. R. Allegretti,
et al., Increased Intestinal Microbial Diversity Following Fecal Microbiota Transplant for Active Crohn's Disease, Inflammatory Bowel Dis., 2016, 22(9), 2182–2190 CrossRef PubMed.
- S. Kunde, A. Pham and S. Bonczyk,
et al., Safety, Tolerability, and Clinical Response After Fecal Transplantation in Children and Young Adults With Ulcerative Colitis, J. Pediatr. Gastroenterol. Nutr., 2013, 56(6), 597–601 CrossRef PubMed.
- S. Angelberger, W. Reinisch and A. Makristathis,
et al., Temporal Bacterial Community Dynamics Vary Among Ulcerative Colitis Patients After Fecal Microbiota Transplantation, Am. J. Gastroenterol., 2013, 108(10), 1620–1630 CrossRef CAS PubMed.
- T. Qazi, T. Amaratunga and E. L. Barnes,
et al., The risk of inflammatory bowel disease flares after fecal microbiota transplantation: systematic review and meta-analysis, Gut Microbes, 2017, 8(6), 574–588 CrossRef PubMed.
- S. Zhen, C. Zhao and X. Zhao,
et al., Faecal Microbiota Microsphere Contributed to Relieving Gut Barrier Damage in Colitis, Macromol. Res., 2020, 28(6), 644–649 CrossRef CAS.
- S. Lee, S.-P. Choi and H.-J. Choi,
et al., A comprehensive review of synbiotics: an emerging paradigm in health promotion and disease management, World J. Microbiol. Biotechnol., 2024, 40(9), 280 CrossRef PubMed.
-
S. Kolida and G. R. Gibson, Synbiotics in Health and Disease, in Annual Review of Food Science and Technology, ed. M. P. Doyle and T. R. Klaenhammer, 2011, vol. 2, pp. 373–393 Search PubMed.
- H. Steed, G. T. Macfarlane and K. L. Blackett,
et al., Clinical trial: the microbiological and immunological effects of synbiotic consumption – a randomized double-blind placebo-controlled study in active Crohn's disease, Aliment. Pharmacol. Ther., 2010, 32(7), 872–883 CrossRef CAS PubMed.
- D. F. Gomez Quintero, C. R. Kok and R. Hutkins, The Future of Synbiotics: Rational Formulation and Design, Front. Microbiol., 2022, 13, 919725 CrossRef PubMed.
- Y. J. Zhang, X. J. Zhang and L. X. Lv,
et al., Versatile inulin/trans-ferulic acid/silk sericin nanoparticles-nourished probiotic complex with prolonged intestinal retention for synergistic therapy of inflammatory bowel disease, Carbohydr. Polym., 2025, 350, 123063 CrossRef CAS PubMed.
|
This journal is © The Royal Society of Chemistry 2025 |
Click here to see how this site uses Cookies. View our privacy policy here.