DOI:
10.1039/D4MA01046A
(Paper)
Mater. Adv., 2025, Advance Article
One step gamma-ray induced crosslinking and sterilization of electrospun poly(ε-caprolactone)/collagen composite scaffolds
Received
17th October 2024
, Accepted 20th January 2025
First published on 19th February 2025
Abstract
Sterilizing biomaterials before implantation is crucial, but this process can sometimes alter the physical, chemical, and morphological properties of collagen-based biomaterials, potentially leading to weaknesses. To address this issue, we propose a method that combines crosslinking and sterilization of collagen-based composite scaffolds through gamma-ray irradiation. In this study, poly(ε-caprolactone) (PCL)/collagen composite scaffolds were fabricated using an electrospinning technique and exposed to gamma rays at doses ranging from 15 to 45 kGy. The radiation dose was optimized to enhance mechanical properties, which indicated a higher degree of collagen crosslinking. Additionally, we demonstrated that both Gram-positive and Gram-negative bacteria were completely and effectively sterilized during the crosslinking process. In conclusion, gamma-ray irradiation shows great promise as a method for simultaneously inducing crosslinking and sterilization in collagen-based scaffolds, offering substantial potential for biomedical applications.
Introduction
Sterilization is crucial when biomaterials are intended for direct application to the human body, as unsterilized materials can lead to serious side effects such as bacterial infections and inflammation.1,2 Common sterilization methods include ultraviolet (UV) radiation, ethylene oxide (EO) gas, and steam sterilization.3–6 However, these methods often have limitations. UV sterilization typically requires chemical agents, EO sterilization can leave toxic residues, and steam sterilization operates at high temperatures and pressures, which can damage or alter the properties of biomaterials.7–10 These challenges can make achieving uniform and complete sterilization difficult. Gamma-ray sterilization, on the other hand, offers a more effective solution. It works by destroying bacteria through the mutation of bacterial DNA, providing a uniform and thorough sterilization process.11–13 Therefore, gamma rays can effectively sterilize medical and implantable devices, achieving complete sterilization even for large and complex devices due to their high penetration depth.14,15
Gamma rays are a form of high-energy radiation capable of ionizing polymeric biomaterials. This allows gamma rays to modify the properties of polymeric biomaterials, such as inducing crosslinking, functionalization, polymerization, and degradation, without the need for chemical reagents like crosslinkers, initiators, or catalysts. This process is facilitated by radical combination.16,17 Gamma rays can generate free radicals at low binding energy sites, with the extent of this effect being controllable by adjusting the radiation dose and dose rate.18,19 In crosslinking reactions, gamma rays can induce uniform and rapid crosslinking of polymers through radical combination, eliminating the need for crosslinking reagents.20–22 For instance, hydrophilic materials can undergo reactions when immersed in or dissolved in water during radiation exposure. The radiation energy generates H˙ and/or OH˙ radicals from the water, which in turn create radicals at specific sites within the material, particularly at low binding energy sites. These radicals can stabilize by inducing crosslinking through radical recombination, depending on the material's properties.23–25
In this study, we aimed to explore the feasibility of using gamma-ray irradiation to achieve simultaneous crosslinking and sterilization of collagen-based composite biomaterials. We first prepared a composite scaffold composed of poly(ε-caprolactone) (PCL) and collagen through electrospinning. PCL/collagen scaffolds were selected as the model for this study to evaluate the dual functionality of gamma-ray irradiation. Collagen offers excellent biocompatibility and cellular interaction properties, while PCL provides superior mechanical strength and structural stability, forming a complementary combination. These characteristics make PCL/collagen scaffolds highly suitable for biomedical applications, including tissue engineering. Furthermore, gamma-ray irradiation enables simultaneous collagen crosslinking and scaffold sterilization, making this system an ideal platform to assess the potential of gamma rays in enhancing scaffold properties for advanced biomedical applications. This scaffold was then subjected to gamma-ray irradiation at various doses. After treatment, we characterized the gamma-ray-irradiated PCL/collagen scaffolds to assess their degree of crosslinking and mechanical properties. Additionally, we evaluated the sterilization efficacy using Escherichia coli (E. coli) and Staphylococcus aureus (S. aureus) as test organisms.
Experimental methods and materials
Materials
Poly(ε-caprolactone) (PCL, DURECT Corporation, Birmingham, AL) and collagen (Elastin Products, Owensville, MO) were purchased. 1,1,1,3,3,3-Hexafluoro-2-propanol (HFIP) and acetic acid were purchased from Sigma-Aldrich (St. Louis, MO, USA). Dulbecco's phosphate-buffered saline (DPBS), fetal bovine serum (FBS), smooth muscle cell growth basal medium (SmBM®), and penicillin and streptomycin (PS) were purchased from GIBCO (Carlsbad, CA, USA). All other reagents and solvents were of analytical grade and used as received.
Gamma-ray irradiation of the electrospun PCL/collagen scaffolds
To prepare the PCL/collagen blend solution, PCL and collagen (1
:
1) were dissolved in HFIP at room temperature to achieve a final concentration of 5% (w/v). For scaffold fabrication, the PCL/collagen solution was processed using an electrospinning setup, which included a high-voltage power supply, an infusion pump, and a rotating collector drum. The solution was loaded into a plastic syringe (Henke Sass Wolf, Germany) equipped with an 18 G stainless-steel needle. The needle was positioned 15 cm from the collector, with a feed rate of 3.0 mL h−1 and an applied voltage of 25 kV. Following electrospinning, the PCL/collagen scaffolds were irradiated with a 60Co source (ACEL type C-1882, Korea Atomic Energy Research Institute, Jeongeup, Republic of Korea) at radiation doses of 15, 25, 35, and 45 kGy (at a rate of 10 kGy h−1) in an EtOH (96
:
4) solution. After irradiation, the electrospun PCL/collagen scaffolds were washed three times with deionized water. The schematic illustration of PCL/collagne composite scaffolds is shown in Fig. 1.
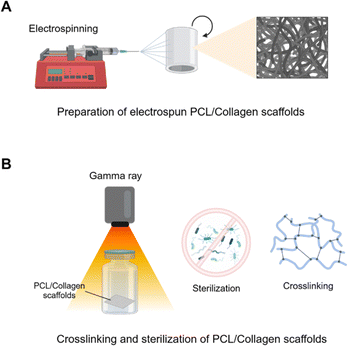 |
| Fig. 1 Schematic illustration of PCL/collagen composite scaffolds. (A) Preparation of PCL/collagen scaffolds using electrospinning. (B) Gamma ray-induced collagen crosslinking and sterilization. | |
Characterization of the gamma-ray-irradiated PCL/collagen scaffolds
The morphologies of both PCL/collagen scaffolds and gamma-ray irradiated PCL/collagen scaffolds were examined using scanning electron microscopy (SEM, JSM–6390, JEOL, Japan) with an electron beam energy of 15 kV and a working distance of 8–10 mm. High-resolution images of all samples were obtained after gold sputter-coating for 60 s. To assess the degree of crosslinking in PCL/collagen scaffolds subjected to different radiation doses, the 2,4,6-trinitrobenzene sulfonic acid (TNBS) assay was conducted. Collagen was dissolved in 0.1 M sodium bicarbonate (pH 8.5) at concentrations ranging from 1.25 to 20 μg mL−1. A 0.01% (w/v) TNBS solution was added to each sample, which was then gently shaken and incubated at 37 °C for 2 h. Subsequently, 10% sodium dodecyl sulfate (SDS) and 1 N HCl were added to each sample, and absorbance was measured at 335 nm. The chemical properties of PCL/collagen scaffolds and gamma-ray irradiated PCL/collagen scaffolds were analyzed using attenuated total reflection-Fourier transformed infrared spectroscopy (ATR-FTIR, Bruker TEMSOR 37, Bruker AXS. INC., Karlsruhe, Germany) over the range 650 to 4000 cm−1 at a resolution of 4 cm−1 averaged over 64 scans.
Water contact angle measurement
To assess the wettability of PCL/collagen scaffolds before and after irradiation, the water contact angle was measured using a Phoenix 300 contact angle analyzer (Surface Electro Optics Co., Suwon, Republic of Korea) following the static sessile drop method. A 10 μL droplet of distilled water was placed on the scaffold surfaces for measurement.
Mechanical testing
The mechanical properties of the PCL/collagen scaffolds were evaluated to determine the degree of crosslinking at various radiation doses ranging from 15 to 45 kGy. A uniaxial load testing machine (Model #5544, Instron Co.) equipped with a 100 N load cell was used, operating at a crosshead speed of 1 mm s−1. The samples were prepared with dimensions of 10 mm in width and 30 mm in length for testing.
Sterility validation of the gamma-ray-irradiated PCL/collagen scaffolds
To validate the effectiveness of gamma-ray irradiation in achieving sterility, Escherichia coli (E. coli, KCOM 1633, a Gram-negative bacterium) and Staphylococcus aureus (S. aureus, KCOM 1333, a Gram-positive bacterium) were prepared. Each bacterial strain was diluted in 1 mL of LB media to achieve a density of 1 × 104 bacteria per mL. The PCL/collagen and gamma-irradiated PCL/collagen scaffolds were then immersed in LB media containing the bacteria and incubated for 18 h at 37 °C. Following incubation, the bacterial samples were exposed to gamma radiation at doses of 15, 25, 35, and 45 kGy (at a dose rate of 10 kGy h−1). After irradiation, 5 μL of the irradiated media was plated onto LB agar and incubated for 18 h at 37 °C.26
In vitro biological evaluation of the gamma-ray-irradiated PCL/collagen scaffolds
Smooth muscle cells (SMCs) were seeded onto PCL/collagen scaffolds at a density of 2 × 104 cells per cm2 and incubated at 37 °C in a 5% CO2 atmosphere. The SMCs were cultured in SmBM® supplemented with 10% FBS and 1% PS. The culture medium was replaced every 2 days, and the cells were maintained for 3 days. To assess cell adhesion, the scaffolds were stained for F-actin and nuclei. After 3 days of culture, cells were fixed in 3.7% paraformaldehyde at room temperature for 15 min, followed by washing with DPBS. SMCs were then permeabilized with DPBS containing 0.1% Triton X-100 at 4 °C for 10 min and blocked with a solution of 5% FBS in DPBS at 37 °C for 1 h. For staining, the scaffolds were incubated at 37 °C for 1 h with rhodamine–phalloidin (1
:
100, Molecular Probes, Eugene, OR) to visualize F-actin and Hoechst 33258 (1
:
1000, Molecular Probes, Eugene, OR) to stain nuclei. Fluorescence images were captured using a fluorescence microscope (DMI3000B, Leica, Germany). To evaluate SMC proliferation on the scaffolds, a cell counting kit-8 (CCK-8, Dojindo Molecular Technologies, Inc., Japan) assay was performed on days 1, 3, and 7. CCK-8 solution (culture medium
:
CCK-8 = 9
:
1) was added to the scaffolds and incubated at 37 °C for 2 h. The absorbance of the solution was measured at 450 nm using a microplate reader (Powerwave XS, Biotek, VT, USA).
Statistical analysis
All tests were performed in triplicate, and data are presented as mean ± standard deviation (SD) unless otherwise specified. Statistical significance was determined using one-way analysis of variance (ANOVA) with Tukey's post-hoc test for multiple comparisons, analyzed with SigmaPlot software (p < 0.05, Systat Software, Inc., San Jose, CA, USA).
Results
Characterization of the electrospun PCL/collagen scaffolds after gamma-ray irradiation
The SEM images show the fiber morphology of electrospun PCL/collagen composite scaffolds subjected to varying doses of gamma-ray irradiation (Fig. 2A). Despite the increase in radiation from 15 kGy to 45 kGy, no significant changes in fiber morphology or diameter were observed, indicating that gamma-ray doses within this range did not induce any morphological changes in the PCL/collagen scaffolds. The fiber diameter of 0, 15, 25, 35, and 45 kGy was 204.0 ± 6.0, 203.0 ± 6.5, 201.3 ± 10.9, 200.0 ± 4.0, and 205.6 ± 6.5 nm. The degree of collagen crosslinking was quantified using the TNBS assay, which confirmed that collagen crosslinking increased proportionally with the gamma-ray radiation dose (Fig. 2B). Specifically, the crosslinking degrees for electrospun PCL/collagen scaffolds irradiated at 15, 25, 35, and 45 kGy were 17.76 ± 6.56%, 36.97 ± 2.39%, 54.20 ± 4.69%, and 78.19 ± 4.79%, respectively. The TNBS assay measures free amine groups, and the results indicated a decrease in free amine content as crosslinking increased, with higher doses of gamma radiation producing higher crosslinking levels. FT-IR spectra of the electrospun PCL/collagen scaffolds after gamma-ray irradiation displayed the characteristic peaks of PCL (CH2, C
O, and C–O) and collagen (amide A, I, II, and III) (Fig. 2C). Even at the highest dose of 45 kGy, these characteristic peaks remained unchanged, demonstrating the structural stability of both PCL and collagen despite increasing radiation doses.
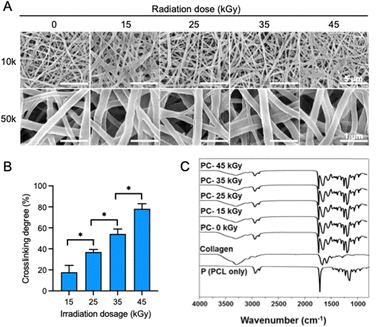 |
| Fig. 2 Electrospun PCL/collagen composite scaffolds following gamma-ray irradiation: (A) SEM images, (B) degrees of crosslinking, (C) FT-IR spectra of scaffolds subjected to radiation doses ranging from 15 to 45 kGy. | |
Water contact angle measurement
Hydrophilicity was evaluated through water contact angle measurements, which confirmed an increase in the hydrophilicity of the scaffolds as the gamma-ray dose increased (Fig. 3). At 10 seconds, the water contact angle of the PCL scaffold alone was 103.79°, reflecting its high hydrophobicity. However, the PCL/collagen composite scaffold exhibited a water contact angle of 46.68°, indicating enhanced hydrophilicity. The water contact angles for the PCL/collagen scaffolds irradiated at 15, 25, 35, and 45 kGy were 26.65°, 14.70°, 13.29°, and 18.04°, respectively. This indicates the presence of oxygen-based functional groups introduced onto the surface of the PCL/collagen composite scaffolds after the gamma-ray irradiation process.
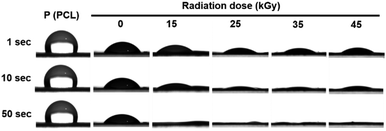 |
| Fig. 3 Water contact angle of PCL/collagen composite scaffolds following gamma-ray irradiation: photographs showing water droplets on the scaffold surfaces at 1, 10, and 50 seconds. | |
Tensile properties of the electrospun PCL/collagen scaffolds
To assess the mechanical properties of electrospun PCL/collagen scaffolds in relation to collagen crosslinking induced by gamma-ray irradiation, tensile properties were measured (Fig. 4). The stress–strain curves of the scaffolds indicated an increase in tensile stress and a corresponding decrease in tensile strain with higher radiation doses (Fig. 4A). The tensile strength of the scaffolds at 15, 25, 35, and 45 kGy was 3.23 ± 0.27, 4.00 ± 0.21, 4.28 ± 0.23, 4.79 ± 0.19, and 6.08 ± 0.23 MPa, respectively (Fig. 4B). This indicates that the tensile strength increased with increasing radiation doses, correlating with enhanced collagen crosslinking. Conversely, the tensile strain decreased as radiation doses increased, indicating that the scaffolds became more brittle at higher doses. The Young's modulus of the scaffolds at 15, 25, 35, and 45 kGy was 0.57 ± 0.09, 0.88 ± 0.19, 1.29 ± 0.09, 2.02 ± 0.14, and 3.16 ± 0.29 MPa, respectively (Fig. 4C). Additionally, the elongation at break values (%) for the scaffolds were 135.81 ± 13.30%, 116.78 ± 7.73%, 86.97 ± 3.10%, 54.93 ± 3.73%, and 34.49 ± 3.24%, respectively (Fig. 4D). These findings confirm that collagen crosslinking, as reflected in the mechanical properties, increased proportionally with the radiation dose.
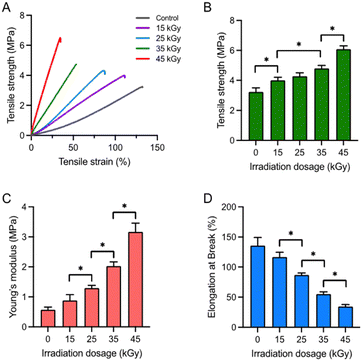 |
| Fig. 4 Tensile properties of PCL/collagen composite scaffolds following gamma-ray irradiation: (A) stress–strain curve, (B) ultimate tensile strength, (C) Young's modulus, and (D) elongation at break (%). | |
Sterility validation of the gamma-ray-irradiated PCL/collagen scaffolds
The sterility of the electrospun PCL/collagen scaffolds following gamma-ray irradiation was evaluated using E. coli and S. aureus (Fig. 5). Initially, each bacterial strain was incubated with the scaffolds for 24 h. After irradiation, the bacteria were cultured in LB medium for an additional 18 h at 37 °C to assess the sterilization effectiveness. Bacterial growth was observed in the non-irradiated scaffolds, while no bacterial presence was detected in the gamma-ray-irradiated scaffolds. This indicates that sterilization was fully achieved even at a low dose of 15 kGy and was maintained up to 45 kGy.
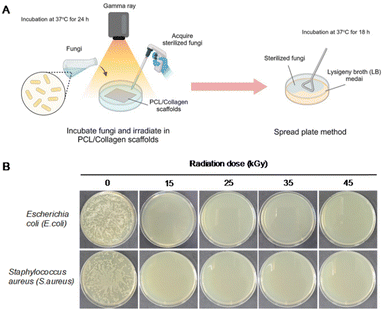 |
| Fig. 5 Sterilization assay of PCL/collagen composite scaffolds following gamma-ray irradiation using Escherichia coli (E. coli) and Staphylococcus aureus (S. aureus): (A) schematic illustration of the spread plate method for the sterilization assay, and (B) optical images showing bacterial growth at different radiation doses. | |
In vitro biological evaluation of the gamma-ray-irradiated PCL/collagen scaffolds
To evaluate the biological properties of the scaffolds, cell adhesion and proliferation assays were conducted on the gamma-ray irradiated PCL/collagen scaffolds using SMCs. Fig. 6A presents F-actin-stained fluorescence images of SMCs after 3 days of incubation on scaffolds irradiated at varying doses (15, 25, 35, and 45 kGy). The results demonstrate strong cell adhesion across all groups. Fig. 6B shows the proliferation of SMCs as measured by the CCK-8 assay over a 7-day period, with a marked increase in proliferation observed from day 1 to day 7. Notably, the gamma-ray irradiated scaffolds exhibited higher cell proliferation compared to non-irradiated and PCL-only scaffolds, likely due to enhanced surface wettability.
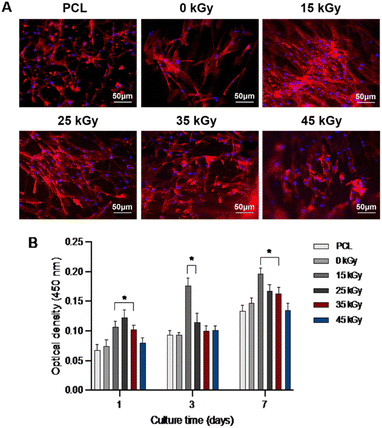 |
| Fig. 6 Cell adhesion and proliferation on PCL/collagen composite scaffolds following gamma-ray irradiation: (A) fluorescence images of F-actin staining after seeding smooth muscle cells (SMCs) on the scaffolds, and (B) cell proliferation assessed using the CCK-8 assay. | |
Discussion
We aimed to demonstrate that gamma-ray irradiation can be effectively applied to PCL/collagen composite scaffolds for simultaneous collagen crosslinking and sterilization. While most gamma-ray-based studies focus on inducing a single reaction per irradiation, there is a noticeable gap in research exploring the potential for initiating multiple reactions through gamma-ray exposure. In a previous study, we successfully prepared and optimized polypyrrole (PPy)/polyvinylpyrrolidone (PVP) hydrogels using gamma rays, achieving both crosslinking and polymerization simultaneously.25 In this study, we present the potential for applying gamma-ray irradiation to induce both collagen crosslinking and sterilization in PCL/collagen scaffolds concurrently. To effectively induce collagen crosslinking, a solution of EtOH
:
diH2O (96
:
4 ratio) was used. Non-crosslinked collagen may alter its physical properties upon exposure to water, so we employed a solution primarily composed of ethanol (96%) to stabilize the collagen-based scaffolds. The addition of 4% diH2O was intended to facilitate a radical reaction induced by gamma-ray irradiation. Previous studies on collagen crosslinking using radiation have been limited, but it has been reported that collagen can be crosslinked through radical recombination reactions.27,28 Hydrophilic substances can effectively undergo reactions when immersed in water. The mechanism involves the formation of radicals, specifically H˙ and OH˙, from water molecules exposed to gamma rays.29–31 Although collagen crosslinking via radiation can be influenced by factors such as the radiation source, dose, and dose rate, controlling this process is challenging due to collagen's inherent sensitivity to radiation.32 However, in this study, we successfully regulated the crosslinking degree of the PCL/collagen scaffolds by adjusting the irradiation dose. The results demonstrated that tensile strength increased as the radiation dose was elevated from 15 to 45 kGy, indicating that higher radiation doses facilitated more extensive collagen crosslinking. Simultaneously, we evaluated the sterilization effect using both Gram-negative (E. coli) and Gram-positive bacteria (S. aureus). The rationale for using gamma rays to achieve both crosslinking and sterilization was to facilitate the immediate application of biomaterials to the human body. In this study, gamma-ray irradiation at doses of 15–25 kGy was found to be sufficient for achieving complete sterilization and enhancing both the mechanical and biological properties of the PCL/collagen scaffolds. These doses are optimal for applications such as medical devices and general biomaterial uses where balanced mechanical performance and biocompatibility are critical. On the other hand, higher doses, exceeding 35 kGy, provided greater crosslinking stability, which is advantageous for advanced tissue engineering applications requiring robust structural integrity. These findings demonstrate the versatility of gamma-ray irradiation in tailoring scaffold properties for specific biomedical applications.26 The enhanced biological performance observed in gamma-ray-irradiated PCL/collagen scaffolds aligns with prior research on electrospun nanofibrous scaffolds, which demonstrated their efficacy in wound healing and antibacterial applications.33,34 These findings further support the potential of gamma-ray irradiation to improve the functionality of nanofibrous scaffolds for biomedical applications, such as tissue engineering and wound healing. Traditional sterilization processes, conducted after the development of biomaterials, can alter their physical and/or chemical properties depending on their characteristics. To circumvent this issue, we employed gamma-ray irradiation to induce collagen crosslinking while simultaneously sterilizing the materials. This study confirmed effective sterilization at radiation doses ranging from 15 to 45 kGy. Typically, gamma rays are used to sterilize medical devices at a standard dose of 25 kGy.12 we found that complete sterilization was achieved even at a lower dose of 15 kGy. These findings demonstrate that it is possible to simultaneously achieve crosslinking and sterilization of the PCL/collagen composite scaffolds using gamma-ray irradiation.
Conclusion
We successfully developed a one-step process for gamma-ray-induced crosslinking and sterilization of electrospun PCL/collagen composite scaffolds. Gamma-ray irradiation at doses ranging from 15 to 45 kGy did not alter the fiber morphology or diameter of the scaffolds but effectively induced collagen crosslinking, thereby enhancing their tensile mechanical properties. Additionally, the process ensured complete sterilization of the scaffolds. The gamma-ray treatment also improved surface wettability, leading to enhanced cell adhesion and proliferation. This study demonstrates that gamma-ray-induced crosslinking and sterilization is a valuable and efficient approach for preparing collagen-based scaffolds for various biomedical applications.
Data availability
All relevant data supporting the findings of this study are fully included within the main article and its supplementary information. The numerical data used in the analysis are explicitly presented in the manuscript, ensuring full transparency and reproducibility of the research. Should any further clarification regarding the data be required, we would be happy to provide additional details upon request.
Conflicts of interest
There are no conflicts to declare.
Acknowledgements
This work was supported by the Korea Atomic Energy Research Institute (KAERI) Institutional Programs (No. 271000733 and 2710007330).
Notes and references
- J. M. Anderson, A. Rodriguez and D. T. Chang, Semin. Immunol., 2008, 20, 86–100 CrossRef CAS PubMed.
- E. Mariani, G. Lisignoli, R. M. Borzì and L. Pulsatelli, Int. J. Mol. Sci., 2019, 20, 636–677 CrossRef CAS PubMed.
- C. A. Rickert, M. G. Bauer, J. C. Hoffmeister and O. Lieleg, Adv. Mater. Interfaces, 2022, 9, 2101716 CrossRef CAS.
- B. Bharti, H. Li, Z. Ren, R. Zhu and Z. Zhu, Chemosphere, 2022, 308, 136404 CrossRef CAS.
- A. Igual-Munoz, J.-L. Genilloud, B. M. Jolles and S. Mischler, Bioengineering, 2023, 10, 749 CrossRef CAS.
- N. P. Tipnis and D. J. Burgess, Int. J. Pharm., 2018, 544, 455–460 CrossRef CAS PubMed.
- I. Mehta, H.-Y. Hsueh, S. Taghipour, W. Li and S. Saeedi, Rob. Auton. Syst., 2023, 161, 104332 CrossRef PubMed.
- J. Koivunen and H. Heinonen-Tanski, Water Res., 2005, 39, 1519–1526 CrossRef CAS PubMed.
- G. C. C. Mendes, T. R. S. Brandão and C. L. M. Silva, Am. J. Infect. Control, 2007, 35, 574–581 CrossRef PubMed.
- W. J. Rogers, in Sterilisation of Biomaterials and Medical Devices, ed. S. Lerouge and A. Simmons, Woodhead Publishing, 2012, pp. 20–55 Search PubMed.
- A. Trampuz, K. E. Piper, J. M. Steckelberg and R. Patel, J. Med. Microbiol., 2006, 55, 1271–1275 CrossRef CAS PubMed.
- C. R. Harrell, V. Djonov, C. Fellabaum and V. Volarevic, Int. J. Med. Sci., 2018, 15, 274–279 CrossRef CAS PubMed.
- S. Shahi, R. Khorvash, M. Goli, S. M. Ranjbaran, A. Najarian and A. Mohammadi Nafchi, Food Sci. Nutr., 2021, 9, 5883–5896 CrossRef CAS PubMed.
- R. Singh, D. Singh and A. Singh, World J. Radiol., 2016, 8, 355–369 CrossRef PubMed.
- R. E. Harrington, T. Guda, B. Lambert and J. Martin, in Biomaterials Science, ed. W. R. Wagner, S. E. Sakiyama-Elbert, G. Zhang and M. J. Yaszemski, Academic Press, 4th edn, 2020, pp. 1431–1446 Search PubMed.
- A. T. Naikwadi, B. K. Sharma, K. D. Bhatt and P. A. Mahanwar, Front. Chem., 2022, 10, 837111 CrossRef CAS PubMed.
- W. Ngwa, F. Boateng, R. Kumar, D. J. Irvine, S. Formenti, T. Ngoma, C. Herskind, M. R. Veldwijk, G. L. Hildenbrand, M. Hausmann, F. Wenz and J. Hesser, Int. J. Radiat. Oncol., Biol., Phys., 2017, 97, 624–637 CrossRef CAS PubMed.
- L. E. Feinendegen, M. Pollycove and C. A. Sondhaus, Nonlinearity Biol., Toxicol., Med., 2004, 2, 143–171 CAS.
- C. Betlazar, R. J. Middleton, R. B. Banati and G.-J. Liu, Redox Biol., 2016, 9, 144–156 CrossRef CAS.
- M. A. Raza, J.-O. Jeong and S. H. Park, Front. Mater., 2021, 8, 769436 CrossRef.
- J.-O. Jeong, S.-I. Jeong, Y.-M. Lim and J.-S. Park, Materials, 2022, 15, 8642 CrossRef CAS.
- J.-O. Jeong, J.-S. Park, E. J. Kim, S.-I. Jeong, J. Y. Lee and Y.-M. Lim, Int. J. Mol. Sci., 2020, 21, 187 CrossRef CAS PubMed.
- J. A. LaVerne, Radiat. Res., 2000, 153, 196–200 CrossRef CAS.
- J. Ma, F. Wang and M. Mostafavi, Molecules, 2018, 23, 244 CrossRef PubMed.
- J.-O. Jeong, J.-S. Park, Y.-A. Kim, S.-J. Yang, S.-I. Jeong, J.-Y. Lee and Y.-M. Lim, Polymers, 2020, 12, 111 CrossRef CAS PubMed.
- S. Kim, J.-O. Jeong, S. Lee, J.-S. Park, H.-J. Gwon, S. I. Jeong, J. G. Hardy, Y.-M. Lim and J. Y. Lee, Sci. Rep., 2018, 8, 3721 CrossRef PubMed.
- M. Abdelmouleh, M. Amin, M. Lalande, T. Schlathölter and J.-C. Poully, Phys. Chem. Chem. Phys., 2023, 25, 29249–29256 RSC.
- X. Liao, L. Li and B. Shi, J. Radioanal. Nucl. Chem., 2004, 260, 619–625 CrossRef CAS.
- X. Zhang, L. Xu, X. Huang, S. Wei and M. Zhai, J. Biomed. Mater. Res., Part A, 2012, 100A, 2960–2969 CrossRef CAS PubMed.
- F. Cataldo, O. Ursini, E. Lilla and G. Angelini, J. Radioanal. Nucl. Chem., 2008, 275, 125–131 CrossRef CAS.
- M. Demeter, M. Virgolici, C. Vancea, A. Scarisoreanu, M. G. A. Kaya and V. Meltzer, Radiat. Phys. Chem., 2017, 131, 51–59 CrossRef CAS.
- D. T. Cheung, N. Perelman, D. Tong and M. E. Nimni, J. Biomed. Mater. Res., 1990, 24, 581–589 CrossRef CAS PubMed.
- F. S. Abadehie, A. H. Dehkordi, M. Zafari, M. Bagheri, S. Yousefiasl, S. Pourmotabed, L. Mahmoodnia, M. Validi, M. Ashrafizadeh and E. N. Zare, Eng. Regener., 2021, 2, 219–226 Search PubMed.
- M. R. Islam, M. A. Karim, M. N. Islam and M. S. Islam, Eng. Regener., 2021, 2, 82–90 Search PubMed.
|
This journal is © The Royal Society of Chemistry 2025 |
Click here to see how this site uses Cookies. View our privacy policy here.