DOI:
10.1039/D4MD00685B
(Research Article)
RSC Med. Chem., 2025,
16, 373-378
A novel approach for the synthesis of the cyclic lipopeptide globomycin†
Received
3rd September 2024
, Accepted 6th October 2024
First published on 21st October 2024
Abstract
Cyclic lipopeptides (CLiPs) are a highly diverse class of secondary metabolites produced by bacteria and fungi. Examples of CLiPs have been found that possess potent antimicrobial activity against multidrug-resistant Gram-negative bacteria. Globomycin is a 19-membered CLiP that kills both Gram-positive and Gram-negative bacteria through inhibition of lipoprotein signal peptidase II (Lsp). It can only be obtained in small quantities from its Streptomyces producer strain, so there has been much interest in development of synthetic methods to access globomycin and analogues. Globomycin contains an N-terminal anti-α-methyl-β-hydroxy nonanoyl lipid tail, whose hydroxyl group forms an ester with the C-terminal carboxylate. Constructing the anti-arrangement between the α-methyl and β-hydroxy is synthetically challenging and previous globomycin syntheses are not compatible with diversification of the lipid tail after the stereocenters have been installed. Herein, we describe a new approach for the synthesis of globomycin that allows for facile lipid diversification. Using an anti-Evans Aldol condensation, a common intermediate is obtained that allows different “lipid swapping” through Grubbs-catalyzed cross-metathesis. Upon auxiliary cleavage, the resulting lipid can then be utilized in solid-phase peptide synthesis. Given the plethora of lipopeptides that contain β-hydroxy lipids, this method offers a convenient approach for convergent generation of lipopeptide analogues.
Introduction
The ever-increasing threat of antimicrobial resistance (AMR) to the quality and sustainability of healthcare is well documented and it is predicted that by 2050, 10 million people will die annually due to AMR.1–4 Data from two Chinese hospitals in the midst of the COVID-19 pandemic found that secondary bacterial infections were associated with 50% of deaths, while only one survivor suffered from co-pathogenesis.5 These findings highlight the immense threat and opportunism of bacteria to cause both primary and co/secondary infections that increase both morbidity and mortality rates of viral infections.6 A recent global analysis of AMR, published in the Lancet in 2022, further highlights the perilous situation to which human healthcare could plummet, associating 4.95 million deaths to AMR in 2019 alone.7 The dangers of AMR are perpetuated by a growing innovation gap in the discovery of novel classes of antibiotics, only exacerbated by big pharma reducing or removing their antimicrobial R&D programs.3,8,9 Clinical overuse and misuse is another increasing concern as it is reported that between 2000 and 2010, the medicinal use of antibiotics rose by nearly 40%.2,4 Antimicrobial peptides (AMPs) are a diverse class of antimicrobial compounds. As of 2019, there were 34 AMPs in the preclinical stage of testing and 27 in clinical trials. Of those in clinical trials (phases I–III), nearly 80% were cationic in nature, highlighting the effectiveness with which peptides of this nature can target the negatively charged bacterial cell membrane.10
Despite the interest in AMPs as drug candidates, the challenges associated with their industrial production and clinical application, such as cost,11 potential cytotoxicity and instability in vivo,12 have spawned only modest efforts in R&D.13 Making clinically viable AMPs can be problematic, owing to their rapid hydrolysis in vivo, toxicity issues and the cost of synthesis.14 Macrocyclic AMPs can benefit from increased permeability across the bacterial membrane and improved stability against enzymatic hydrolysis.12,15 Murepavadin was a promising peptidomimetic antibacterial candidate for treating Pneumonia; the first of its kind as an outer-membrane protein-targeting antibiotic. Unfortunately, its phase III clinical trial was terminated due to unexpected toxicity levels not initially found in healthy subjects.16 Cyclic lipopeptides (CLiPs) are a sub class of AMPs. They are non-ribosomally synthesized, contain a cyclic core, and are N-terminally acylated, offering diverse structures that can kill bacteria via several different avenues.17,18 Our current last-line-of-defence antibiotics for treating Gram-positive infections (daptomycin), and Gram-negative infections (polymyxin), are both CLiPs, highlighting that these compounds still have an important role to play in our fight against AMR. In recent years, there has been a resurgence in CLiP R&D, including the ornicidines,19–22 menaquinone-binding CLiPs23–25 and globomycin.26,27 Globomycin (1) (Fig. 1) was first discovered in 1978 and is produced by Streptomyces strains.28 Its antimicrobial activity against the Gram-negative strain Pseudomonas aeruginosa is due to its coordination and inhibition of lipoprotein signal peptidase II enzyme LspA.29 This enzyme is involved in the posttranslational processing of lipoproteins and is not found in humans, offering a selective drug target.30 Globomycin binds to the aspartic acid catalytic dyad in LspMrs (Lsp from MRSA) via hydrogen bonding from its Ser3 hydroxy group.29 This prevents cleavage of the signal peptide, which is responsible for anchoring the lipopeptide to the cytoplasmic membrane. LspA inhibition blocks the lipoprotein outer-membrane localization (Lol) pathway and results in accumulation of the lipoprotein precursor, prolipoprotein, causing cell death.31
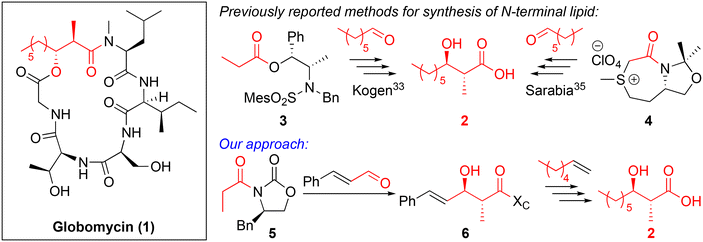 |
| Fig. 1 Structure of globomycin (1) (left), previously reported synthesis of the chiral lipid tail required for globomycin synthesis (top right) and our novel approach for preparation of this chiral lipid tail (bottom). XC = chiral auxiliary. | |
Globomycin is a 19-membered cyclic depsipeptide that contains an N-terminal anti-α-methyl-β-hydroxy nonanoyl lipid tail. The β-hydroxy group is cyclized to the C-terminus through an ester bond. Globomycin is produced in small quantities (10 mg L−1 titre) by its producer strain,28 making total chemical synthesis the only viable option for larger quantities of material. Despite only being a pentapeptide, the total synthesis of globomycin is not trivial. Constructing the anti-α-methyl-β-hydroxy lipid tail is one of the main synthetic challenges. In the first reported total synthesis of globomycin,32,33 Kogen et al. utilized an anti-selective boron-mediated asymmetric aldol reaction (developed by Abiko and Masamune34) for preparation of carboxylic acid 2 (Fig. 1), which was a key intermediate in their solution-phase synthesis of globomycin. This approach provides acid 2 in excellent yields (93%, 94% diastereomeric excess) but chiral auxiliary 3 is very expensive (∼£500 per g, Sigma-Aldrich), or must be prepared in three steps from norepinephrine.34 In 2011, Sarabia et al. used a combination of solid-phase synthesis (peptide chain assembly) and solution-phase synthesis (cyclization step) to synthesize globomycin.35 For construction of acid 2, they employed an asymmetric epoxidation of heptanal using sulfur ylide 4 as a key step. From heptanal, acid 2 was obtained in 42.5% yield over four steps. However, sulfur ylide 4 must also be synthesized, requiring a four step-synthesis from L-methionine (46% overall) that includes two distillations and a recrystallization.36 We rationalized that use of Evans-type auxiliary 5 could allow for a cheaper, more-efficient synthesis of acid 2. Use of an α,β-unsaturated aldehyde in an anti-selective Aldol condensation would yield unsaturated lipid 6 that could then be diversified by “lipid swapping” through Grubbs-catalyzed cross metathesis reactions (Scheme 1). Inspired by previous total syntheses of brevicidine and laterocidine analogues,19,20,37 we aimed to perform the entirety of globomycin synthesis on resin.
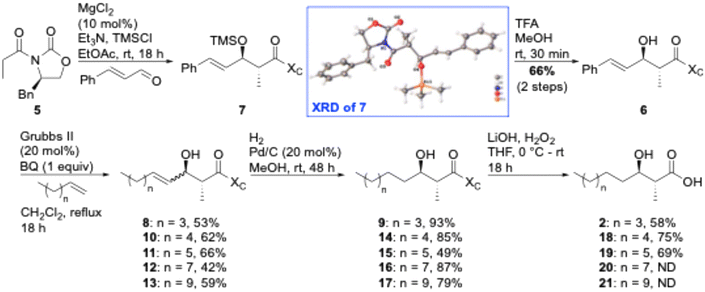 |
| Scheme 1 Novel synthesis of globomycin lipid tail. BQ = benzoquinone; ND = not determined; TFA = trifluoroacetic acid; TMS = trimethylsilyl; XC = chiral auxiliary; XRD = X-ray diffraction. | |
Results and discussion
We have previously utilized acetyl or propanoyl Evans-type auxiliaries in Aldol condensations to prepare the chiral lipids required for solid-phase peptide synthesis (SPPS) of tridecaptins38,39 and cerexins.40 For the synthesis of acid 2, propanoyl Evans auxiliary 5 was chosen as a cheap (∼£15 per g, Sigma-Aldrich) and readily available starting material. To allow lipid diversification after the α and β stereocentres have been set through an Aldol condensation, we required an alkene to be present in the resulting product. To achieve this, Evans previously reported procedure for magnesium-halide catalyzed anti-Aldol reactions of chiral N-acyloxazolidinones41 was used to perform an Aldol condensation between trans-cinnamaldehyde and Evans auxiliary 5 (Scheme 1), yielding enol 6 in 66% yield. The use of TMSCl in this reaction is required for silyation of the intermediate metal aldolate, turning over the metal center and improving diastereoselectivity. Serendipitously, during one synthesis of enol 6 the TMS deprotection step was omitted, resulting in isolation of TMS ether 7, which readily crystallized in column fraction tubes. An X-ray crystal structure of TMS ether 7 was obtained, unambiguously showing that the desired anti-selectivity had been achieved (see ESI†). Next, the use of Grubbs II-catalyzed cross-metathesis (CM), followed by alkene hydrogenation, was investigated to swap out the phenyl group with a pentene chain to give the anti-α-methyl-β-hydroxy nonanoyl lipid tail found in globomycin. We chose the Grubbs II catalyst as it's cheaper than Hoveyda–Grubbs variants. During optimization of the CM step, we found that enolization of the enol starting material 6 and product 8, to yield α-methyl-β-ketones, was a major competing side reaction. This side reaction was almost entirely eliminated by addition of 1 equiv. of benzoquinone. Alkene 8 was isolated as a mixture of cis- and trans-isomers, which were then reduced using H2 over a Pd/C catalyst to provide oxazolidinone 9 in 93% yield. In our initial efforts, we carried the crude CM reaction mixture through to hydrogenation and then performed column chromatography to isolate the desired product. However, reaction mixtures were complex and often resulted in co-elution of side-products with desired products. Therefore, we performed column chromatography after both the CM and hydrogenation steps. Finally, acid 2 was obtained by hydrolysis of oxazolidinone 9. Overall, this approach provides acid 2 in 19% yield over 4 steps. Before using acid 2 in the synthesis of globomycin, we investigated whether alkene 8 could be used as a common intermediate for the synthesis of chiral lipids of varying chain lengths. Gratifying, cross metathesis reactions between a range of linear terminal alkenes and alkene 8 proceeded smoothly to yield metathesis products 10–13, with the general trend being that product yields decreased as the chain length of the alkene increased. Hydrogenation of these alkenes yielded alkanes 14–17, mostly in excellent yields. Auxiliary cleavage proceeded smoothly to yield acids 18 and 19 but as chain length increased it became more difficult to separate the desired acid products from oxazolidinone by product.
Next, we utilized acid 2 in the synthesis of globomycin (1) using the method previously reported by Sarabia et al. (Scheme 2).35 Fmoc-Gly-2CT (22) was first prepared with a loading of 0.5 mmol g−1, and Fmoc SPPS then used to synthesize resin-bound pentapeptide 23. Acylated peptide 24 was then prepared by treatment with two portions of acid 2, DEPC and TBS, Bn-protected pentapeptide 25 was cleaved from resin using 7
:
2
:
1 CH2Cl2
:
AcOH
:
TFA and a Yamaguchi esterification used to prepare cyclic lipopeptide 26. Following sequential treatment with TBAF and H2/Pd(OH)2 to remove the TBS and Bn groups respectively, RP-HPLC purification was performed, providing pure globomycin (1) in 10% overall yield. HPLC co-injection with natural globomycin (Merck: G1424) showed only a single peak, providing confirmation that the synthetic product is identical to natural globomycin.
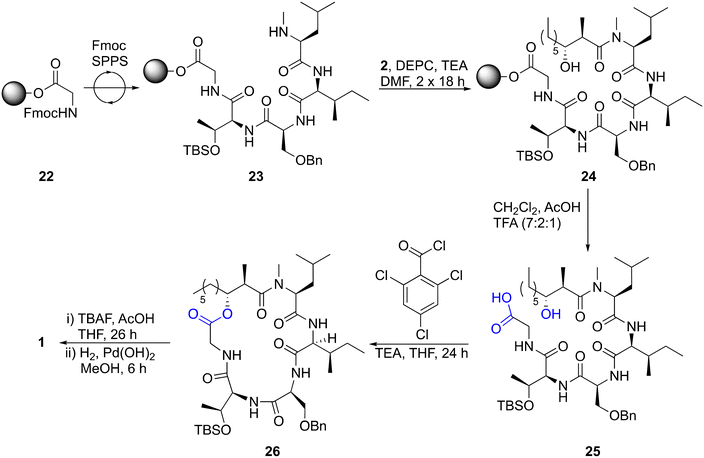 |
| Scheme 2 Synthesis of globomycin (1). Bn = benzyl; DEPC = diethyl cyanophosphonate; DMF = dimethylformamide; Fmoc = fluorenylmethyloxycarbonyl; TBAF = tetrabutylammonium fluoride; TBS = tert-butyldimethylsilyl; TEA = triethylamine; TFA = trifluoroacetic acid. | |
We then embarked on an attempt to perform the total solid-phase synthesis of globomycin. To do this, we envisaged using a similar approach that we previously used to synthesize laterocidine.19,20 This first required immobilization of Fmoc-allo-Thr-OAllyl to an appropriate resin, ideally with a loading of 0.1–0.2 mmol g−1 so as to minimize dimerization during the on-resin cyclization step. A variety of resins were screened for their loading capacity of Fmoc-allo-Thr-OAllyl, including trityl (Trt) chloride, 2-chlorotrityl (2-CT) chloride and brominated Wang resin. However, none were able to anchor the desired residue via its side-chain hydroxy group in loadings high enough for fruitful SPPS. Eventually we identified that 4-methoxybenzhydryl (4-MeO-BH) bromide resin, previously utilized by Ficht et al. in the synthesis of thioester-containing peptides, allowed the desired loadings to be obtained (∼0.2 mmol g−1) (Scheme 3).42 Immobilized Fmoc-allo-Thr-OAllyl 27 was elongated into tetrapeptide 28 using Fmoc SPPS, wherein Fmoc deprotections were completed using 20% 4-methylpiperidine (4-MP) in DMF (2 × 1′, 1 × 5′) and couplings performed using HATU/DIPEA/DMF (1 h). To couple acid 2 on to the secondary amine of tetrapeptide 28, we utilized the method previously reported by Sarabia et al. (DEPC, TEA, DMF, 2 × 18 h), providing acylated tetrapeptide 29.35 Gly-1 was then installed through a Steglich esterification (DIC, DMAP, CH2Cl2, 18 h), and Fmoc and allyl protecting groups sequentially removed. A resin microcleavage was performed and HPLC analysis revealed a mixture of the desired linear precursor 31 (LC-MS, [M + H]+ calcd for C36H67N5O10H 730.5, found 730.3), as well as a side-product in which the glycine ester moiety had been hydrolyzed and allyl group deprotected (LC-MS, [M + H]+ calcd for C34H64N4O9H 673.5, found 673.3). We were unable to ascertain if this occurred due to incomplete Fmoc-Gly-OH coupling in the previous step, hydrolysis during subsequent basic Fmoc deprotections, or during the resin cleavage step. Therefore, it was decided to proceed with screening conditions for the final on-resin macrolactonisation step. Unfortunately, the final on-resin macrolactamization proved extremely challenging. Several conditions were screened, including BOP/DIPEA, DIC/HOBt/DMAP and HATU/DIPEA. Only when HATU was used as a coupling agent was any trace of desired product identified, and even then, the HPLC purification trace was very messy, and product was not present in sufficient quantities to isolate. In our previous synthesis of globomycin analogues in which the ester was replaced with amides or alkenes,27 we also found that on-resin cyclization steps were messy and low-yielding, suggesting these problems are amino acid sequence dependent. The results are in stark-contrast to on-resin cyclization of brevicidine or laterocidine, which proceed quantitatively with minimal side products.20
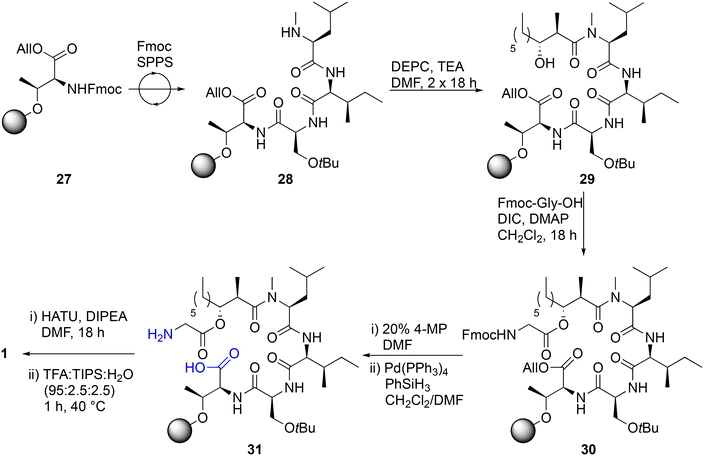 |
| Scheme 3 Unsuccessful total solid-phase synthesis of globomycin (1). All = allyl; Alloc = allyloxycarbonyl; DEPC = diethyl cyanophosphonate; DIC = diisopropylcarbodiimide; DIPEA = diisopropylethylamine; DMAP = 4-dimethylaminopyridine; DMF = dimethylformamide; Fmoc = fluorenylmethyloxycarbonyl; PyBOP = (1H-1,2,3-benzotriazol-1-yloxy)-tris(pyrrolidino)-phosphonium hexafluorophosphate; TEA = triethylamine; TIPS = triisopropylsilane. | |
In summary, we have developed a new synthetic route to the antimicrobial peptide globomycin. Using an anti-Evans Aldol condensation, we obtained a common intermediate that allows for efficient lipid swapping via Grubbs-catalyzed cross-metathesis reactions. This approach addresses the synthetic challenges of constructing the anti-arrangement between the α-methyl and β-hydroxy groups and enables a streamlined synthesis of globomycin analogues with varied lipid tails. Although our investigations into a total solid-phase synthesis of globomycin did not surpass existing methods, they provided valuable insights that may prove useful in the future development of new methods to prepare globomycin. Future syntheses may also be able to incorporate chiral cinnamyl precursor 6 into globomycin, allowing late-stage lipid swapping. Overall, this approach offers a flexible and efficient framework for the synthesis of chiral lipid variants of antimicrobial peptides, which could be used in developing new antibiotic candidates.
Data availability
The data supporting this article have been included as part of the ESI.† Crystallographic data for compound 7 was deposited at the CCDC on Sept 3rd 2024 (deposition number 2381556) and the URL for this is: https://www.ccdc.cam.ac.uk/structures/Search?Ccdcid=2381556&DatabaseToSearch=Published.
Conflicts of interest
There are no conflicts of interest to declare.
Acknowledgements
We thank the School of Chemistry and Chemical Engineering's Analytical Services and Environmental Projects Division at Queen's University Belfast for their assistance with mass spectrometry. Financial support was provided by the Engineering and Physical Sciences Research Council (T01783X/1).
Notes and references
- R. P. Bax, R. Anderson, J. Crew, P. Fletcher, T. Johnson, E. Kaplan, B. Knaus, K. Kristinsson, M. Malek and L. Strandberg, Nat. Med., 1998, 4, 545–546 CrossRef CAS.
- A. Coates, Y. Hu, R. Bax and C. Page, Nat. Rev. Drug Discovery, 2002, 1, 895–910 CrossRef CAS.
- H. W. Boucher, G. H. Talbot, D. K. Benjamin, Jr., J. Bradley, R. J. Guidos, R. N. Jones, B. E. Murray, R. A. Bonomo and D. Gilbert, Clin. Infect. Dis., 2013, 56, 1685–1694 CrossRef PubMed.
-
J. O'Neill, Antimicrobial Resistance: Tackling a Crisis for the Health and Wealth of Nations, Review on Antimicrobial Resistance, 2014 Search PubMed.
- F. Zhou, T. Yu, R. Du, G. Fan, Y. Liu, Z. Liu, J. Xiang, Y. Wang, B. Song, X. Gu, L. Guan, Y. Wei, H. Li, X. Wu, J. Xu, S. Tu, Y. Zhang, H. Chen and B. Cao, Lancet, 2020, 395, 1054–1062 CrossRef CAS PubMed.
- D. E. Morris, D. W. Cleary and S. C. Clarke, Front. Microbiol., 2017, 8, 1041 CrossRef PubMed.
- C. J. L. Murray, K. S. Ikuta, F. Sharara, L. Swetschinski, G. Robles Aguilar, A. Gray, C. Han, C. Bisignano, P. Rao, E. Wool, S. C. Johnson, A. J. Browne, M. G. Chipeta, F. Fell, S. Hackett, G. Haines-Woodhouse, B. H. Kashef Hamadani, E. A. P. Kumaran, B. McManigal, S. Achalapong, R. Agarwal, S. Akech, S. Albertson, J. Amuasi, J. Andrews, A. Aravkin, E. Ashley, F.-X. Babin, F. Bailey, S. Baker, B. Basnyat, A. Bekker, R. Bender, J. A. Berkley, A. Bethou, J. Bielicki, S. Boonkasidecha, J. Bukosia, C. Carvalheiro, C. Castañeda-Orjuela, V. Chansamouth, S. Chaurasia, S. Chiurchiù, F. Chowdhury, R. Clotaire Donatien, A. J. Cook, B. Cooper, T. R. Cressey, E. Criollo-Mora, M. Cunningham, S. Darboe, N. P. J. Day, M. De Luca, K. Dokova, A. Dramowski, S. J. Dunachie, T. Duong Bich, T. Eckmanns, D. Eibach, A. Emami, N. Feasey, N. Fisher-Pearson, K. Forrest, C. Garcia, D. Garrett, P. Gastmeier, A. Z. Giref, R. C. Greer, V. Gupta, S. Haller, A. Haselbeck, S. I. Hay, M. Holm, S. Hopkins, Y. Hsia, K. C. Iregbu, J. Jacobs, D. Jarovsky, F. Javanmardi, A. W. J. Jenney, M. Khorana, S. Khusuwan, N. Kissoon, E. Kobeissi, T. Kostyanev, F. Krapp, R. Krumkamp, A. Kumar, H. H. Kyu, C. Lim, K. Lim, D. Limmathurotsakul, M. J. Loftus, M. Lunn, J. Ma, A. Manoharan, F. Marks, J. May, M. Mayxay, N. Mturi, T. Munera-Huertas, P. Musicha, L. A. Musila, M. M. Mussi-Pinhata, R. N. Naidu, T. Nakamura, R. Nanavati, S. Nangia, P. Newton, C. Ngoun, A. Novotney, D. Nwakanma, C. W. Obiero, T. J. Ochoa, A. Olivas-Martinez, P. Olliaro, E. Ooko, E. Ortiz-Brizuela, P. Ounchanum, G. D. Pak, J. L. Paredes, A. Y. Peleg, C. Perrone, T. Phe, K. Phommasone, N. Plakkal, A. Ponce-de-Leon, M. Raad, T. Ramdin, S. Rattanavong, A. Riddell, T. Roberts, J. V. Robotham, A. Roca, V. D. Rosenthal, K. E. Rudd, N. Russell, H. S. Sader, W. Saengchan, J. Schnall, J. A. G. Scott, S. Seekaew, M. Sharland, M. Shivamallappa, J. Sifuentes-Osornio, A. J. Simpson, N. Steenkeste, A. J. Stewardson, T. Stoeva, N. Tasak, A. Thaiprakong, G. Thwaites, C. Tigoi, C. Turner, P. Turner, H. R. van Doorn, S. Velaphi, A. Vongpradith, M. Vongsouvath, H. Vu, T. Walsh, J. L. Walson, S. Waner, T. Wangrangsimakul, P. Wannapinij, T. Wozniak, T. E. M. W. Young Sharma, K. C. Yu, P. Zheng, B. Sartorius, A. D. Lopez, A. Stergachis, C. Moore, C. Dolecek and M. Naghavi, Lancet, 2022, 399, 629–655 Search PubMed.
- U. Theuretzbacher, K. Outterson, A. Engel and A. Karlén, Nat. Rev. Microbiol., 2020, 18, 275–285 CrossRef.
- L. L. Silver, Clin. Microbiol. Rev., 2011, 24, 71–109 CrossRef CAS PubMed.
- H. B. Koo and J. Seo, Pept. Sci., 2019, 111, e24122 Search PubMed.
- R. D. Ballantine, C. E. McCallion, E. Nassour, S. Tokajian and S. A. Cochrane, MedChemComm, 2019, 10, 484–487 RSC.
- R. D. Ballantine, Y.-X. Li, P.-Y. Qian and S. A. Cochrane, Chem. Commun., 2018, 54, 10634–10637 RSC.
- G. S. Dijksteel, M. M. W. Ulrich, E. Middelkoop and B. Boekema, Front. Microbiol., 2021, 12, 616979 CrossRef PubMed.
- R. Kuppusamy, M. Willcox, D. S. Black and N. Kumar, Antibiotics, 2019, 8, 44 CrossRef CAS PubMed.
- T. Rezai, B. Yu, G. L. Millhauser, M. P. Jacobson and R. S. Lokey, J. Am. Chem. Soc., 2006, 128, 2510–2511 CrossRef CAS PubMed.
- A. Wach, K. Dembowsky and G. E. Dale, Antimicrob. Agents Chemother., 2018, 62, e02355-17 CrossRef.
- J. Wang, X. Dou, J. Song, Y. Lyu, X. Zhu, L. Xu, W. Li and A. Shan, Med. Res. Rev., 2019, 39, 831–859 CrossRef CAS.
- S. A. Cochrane and J. C. Vederas, Med. Res. Rev., 2016, 36, 4–31 CrossRef CAS PubMed.
- K. Al Ayed, R. D. Ballantine, M. Hoekstra, S. J. Bann, C. M. J. Wesseling, A. T. Bakker, Z. Zhong, Y. X. Li, N. C. Brüchle, M. van der Stelt, S. A. Cochrane and N. I. Martin, Chem. Sci., 2022, 13, 3563–3570 Search PubMed.
- R. D. Ballantine, K. Al Ayed, S. J. Bann, M. Hoekstra, N. I. Martin and S. A. Cochrane, RSC Med. Chem., 2022, 13, 1640–1643 Search PubMed.
- R. D. Ballantine, K. Al Ayed, S. J. Bann, M. Hoekstra, N. I. Martin and S. A. Cochrane, J. Med. Chem., 2023, 66, 6002–6009 Search PubMed.
- K. Al Ayed, D. Zamarbide Losada, N. V. Machushynets, B. Terlouw, S. S. Elsayed, J. Schill, V. Trebosc, M. Pieren, M. H. Medema, G. P. van Wezel and N. I. Martin, ACS Infect. Dis., 2023, 9, 739–748 CrossRef CAS.
- H. Itoh, K. Tokumoto, T. Kaji, A. Paudel, S. Panthee, H. Hamamoto, K. Sekimizu and M. Inoue, Nat. Commun., 2019, 10, 2992 Search PubMed.
- D. Chen, L. Tian, K. H. L. Po, S. Chen and X. Li, Bioorg. Med. Chem., 2020, 28, 115677 Search PubMed.
- L. Li, B. Koirala, Y. Hernandez, L. W. MacIntyre, M. A. Ternei, R. Russo and S. F. Brady, Nat. Microbiol., 2022, 7, 120–131 Search PubMed.
- K. Garland, H. Pantua, M. G. Braun, D. J. Burdick, G. M. Castanedo, Y. C. Chen, Y. X. Cheng, J. Cheong, B. Daniels, G. Deshmukh, Y. Fu, P. Gibbons, S. L. Gloor, R. Hua, S. Labadie, X. Liu, R. Pastor, C. Stivala, M. Xu, Y. Xu, H. Zheng, S. B. Kapadia and E. J. Hanan, Bioorg. Med. Chem. Lett., 2020, 30, 127419 Search PubMed.
- T. W. Craven, M. D. Nolan, J. Bailey, S. Olatunji, S. J. Bann, K. Bowen, N. Ostrovitsa, T. M. Da Costa, R. D. Ballantine, D. Weichert, P. M. Levine, L. J. Stewart, G. Bhardwaj, J. A. Geoghegan, S. A. Cochrane, E. M. Scanlan, M. Caffrey and D. Baker, ACS Chem. Biol., 2024, 19, 1125–1130 CrossRef CAS PubMed.
- M. Inukai, M. Nakajima, M. Osawa, T. Haneishi and M. Arai, J. Antibiot., 1978, 31, 421–425 Search PubMed.
- L. Vogeley, T. El Arnaout, J. Bailey, P. J. Stansfeld, C. Boland and M. Caffrey, Science, 2016, 351, 876–880 CrossRef CAS PubMed.
- M. Paetzel, A. Karla, N. C. Strynadka and R. E. Dalbey, Chem. Rev., 2002, 102, 4549–4580 Search PubMed.
- T. Kiho, M. Nakayama, K. Yasuda, S. Miyakoshi, M. Inukai and H. Kogen, Bioorg. Med. Chem., 2004, 12, 337–361 CrossRef CAS PubMed.
- H. Kogen, T. Kiho, M. Nakayama, Y. Furukawa, T. Kinoshita and M. Inukai, J. Am. Chem. Soc., 2000, 122, 10214–10215 Search PubMed.
- T. Kiho, M. Nakayama and H. Kogen, Tetrahedron, 2003, 59, 1685–1697 Search PubMed.
- A. Abiko, J.-F. Liu and S. Masamune, J. Am. Chem. Soc., 1997, 119, 2586–2587 Search PubMed.
- F. Sarabia, S. Chammaa and C. García-Ruiz, J. Org. Chem., 2011, 76, 2132–2144 Search PubMed.
- F. Sarabia, S. Chammaa, M. García-Castro and F. Martín-Gálvez, Chem. Commun., 2009, 38, 5763–5765 Search PubMed.
- V. J. Thombare, J. D. Swarbrick, M. A. K. Azad, Y. Zhu, J. Lu, H. Y. Yu, H. Wickremasinghe, X. He, M. Bandiatmakur, R. Li, P. J. Bergen, T. Velkov, J. Wang, K. D. Roberts, J. Li and N. A. Patil, ACS Cent. Sci., 2024, 10, 1703–1717 CrossRef CAS PubMed.
- C. T. Lohans, M. J. van Belkum, S. A. Cochrane, Z. Huang, C. S. Sit, L. M. McMullen and J. C. Vederas, ChemBioChem, 2014, 15, 243–249 CrossRef CAS PubMed.
- S. A. Cochrane, C. T. Lohans, M. J. van Belkum, M. A. Bels and J. C. Vederas, Org. Biomol. Chem., 2015, 13, 6073–6081 RSC.
- S. A. Cochrane, R. R. Surgenor, K. M. W. Khey and J. C. Vederas, Org. Lett., 2015, 17, 5428–5431 CrossRef CAS.
- D. A. Evans, J. S. Tedrow, J. T. Shaw and C. W. Downey, J. Am. Chem. Soc., 2002, 124, 392–393 CrossRef CAS PubMed.
- S. Ficht, R. J. Payne, R. T. Guy and C.-H. Wong, Chem. – Eur. J., 2008, 14, 3620–3629 CrossRef CAS PubMed.
|
This journal is © The Royal Society of Chemistry 2025 |
Click here to see how this site uses Cookies. View our privacy policy here.