DOI:
10.1039/D4NA00763H
(Review Article)
Nanoscale Adv., 2025,
7, 42-59
Carbon dots: synthesis, sensing mechanisms, and potential applications as promising materials for glucose sensors
Received
11th September 2024
, Accepted 7th November 2024
First published on 22nd November 2024
Abstract
The disruption of glucose (Glu) metabolism in the human body can lead to conditions such as diabetes and hyperglycemia. Therefore, accurately determining Glu levels is crucial for clinical diagnosis and other applications. Carbon dots (CDs) are a novel category of carbon nanomaterials that exhibit outstanding optical properties, excellent biocompatibility, high water solubility, low production costs, and straightforward synthesis. Recently, researchers have developed various carbon dot sensors for fast and real-time Glu monitoring. In this context, we provide a comprehensive introduction to Glu and CDs for the first time. We categorize the synthetic methods for CDs and the sensing mechanisms, further classifying the applications of carbon dot probes into single-probe sensing, ratiometric sensing, and visual detection. Finally, we discuss the future development needs for CD-based Glu sensors. This review aims to offer insights into advancing Glu sensors and modern medical treatments.
1. Introduction
Diabetes mellitus is a condition resulting from a defect in insulin secretion, leading to the buildup of Glu. In the year 2000, over 170 million people globally were affected by diabetes mellitus, and it is projected that by 2030, this number will increase to 366 million.1 Glu levels in the human body are crucial indicators of overall health. For individuals with diabetes, it is especially important to monitor these levels on a daily basis.2,3 As a result, there is a pressing need to develop highly sensitive, selective, and reliable methods for Glu detection that can be applied across various fields, including clinical diagnostics, biotechnology, and the food industry.4,5 To date, a variety of methods and devices have been proposed for Glu testing, including electrochemical sensors,6–9 Raman spectroscopy,10,11 chemiluminescence (CL),12,13 electrochemical transistor sensors,14,15 potentiometric sensors,16,17 and fluorescence (FL) sensors.18–22 Among these, FL sensors have gained recognition as a simple, rapid, and convenient tool for Glu detection.
Various fluorescent materials have been utilized for the detection of Glu, including metal–organic frameworks (MOFs),23–26 nanoclusters (NCs),27 and small molecule fluorescent sensors.28,29 However, each of these materials has certain limitations. MOFs struggle with poor solubility, nanoclusters have issues with short-term stability, and small molecule-based sensors suffer from poor selectivity.30 In recent years, CDs have attracted considerable interest across various fields.31–37
As innovative 0-dimensional materials with diameters ranging from 1 to 10 nm, CDs exhibit excellent photostability, good solubility in water, and resistance to photobleaching.38–40 Compared to other fluorescent materials, CDs offer advantages such as low cost, ease of use, mild preparation conditions, low toxicity, biocompatibility, and a wide range of sources.40–42 Consequently, they have been extensively utilized in optoelectronics,34,43,44 catalysis,33,45 biomedical applications,46,47 industrial applications,32 food studies,48 and particularly in analytical chemistry as fluorescent probes.
Additionally, for clinical applications, the optical and surface chemical properties of CDs make them ideal candidates for theranostic uses, including biosensing,49 bioimaging50 and drug delivery,51 both in vitro and in vivo. In diagnostics, fluorescence molecular bioimaging is crucial for early tumor detection, allowing noninvasive, highly sensitive, and specific observation of pathological and physiological processes.52 CDs offer several advantages, such as low cytotoxicity, excellent biocompatibility, stable photoluminescence, easy functionalization, and remarkable resistance to photobleaching.43,53 Due to these benefits, numerous in vivo and in vitro bioimaging applications have been developed recently.54–56 Moreover, CDs are widely utilized in next-generation optical biosensors due to their high sensitivity, low cost, and practicality. These sensors are used not only for elemental and biomarker detection but also for monitoring changes in fluorescence signals at the single-cell level, through mechanisms such as fluorescence enhancement (turn-on) or quenching (off-state).57,58
CDs were first developed in 2004.59 Since then, their simple synthesis and unique properties have led to a substantial increase in publications exploring various precursors and synthesis methods. These methods are generally divided into top-down and bottom-up approaches.
CDs can be synthesized using two primary methods: “top-down” and “bottom-up.” Top-down techniques, such as ultrasonic synthesis and chemical exfoliation, break down larger carbon structures and are suited for large-scale production but often require extended processing times, harsh conditions, and expensive equipment.31,60 On the other hand, “bottom-up” methods, which are typically used for producing CDs, involve constructing CDs from molecular precursors such as Glu and citric acid through processes such as microwave pyrolysis and chemical vapor deposition. These methods provide better control and produce CDs with fewer defects.61,62
In this review, we aim to offer a unique perspective by highlighting recent progress in using CDs for detecting Glu in different samples. We will cover the synthesis of CDs and examine practical applications for Glu detection, including single probe sensing, ratiometric sensing, and visual detection methods in real samples. Additionally, we will briefly address the challenges and future prospects in the development and application of CDs, as illustrated in Fig. 1.
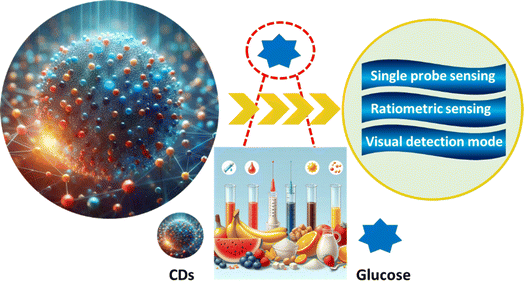 |
| Fig. 1 Summary of CDs and their applications. | |
2. Conditions associated with glucose imbalance
In recent decades, various factors such as shifts in eating habits and physical activity have contributed to an increase in disorders linked to abnormal blood Glu levels. Alongside new discoveries regarding diseases associated with this issue, these developments have driven research to explore innovative methods for Glu monitoring.63,64 The condition linked to abnormal blood Glu levels is known as Diabetes Mellitus (DM), which manifests in several forms: type 1 DM, type 2 DM, Alzheimer's disease, cardiovascular disease and kidney disease.
Type 2 DM is the most prevalent form, accounting for around 90% of all diabetes cases, and the number of patients continues to increase.65 An estimated 374 million people are at increased risk of developing this form of the disease. Type 1 diabetes is more common among children and adolescents, with over 1.1 million young individuals living with it. The two most common types of diabetes stem from either the pancreas's inability to produce insulin (DM1) or reduced tissue sensitivity to insulin (2 DM), both leading to disrupted carbohydrate metabolism. Therefore, monitoring blood Glu levels is crucial.66,67
Similar to Alzheimer's disease (AD), type 2 diabetes mellitus (2 DM) is another widespread condition often linked to obesity and aging. In the U.S., approximately 24 million people exhibit clinical symptoms of 2 DM.68,69 This condition is characterized by elevated blood Glu levels due to increased hepatic Glu production, reduced insulin production by pancreatic β-cells, and insulin resistance, where target cells have a diminished response to insulin as a result of down-regulated expression of insulin receptors (IRs), IGF-1 receptors (IGF-1R), and insulin receptor substrate (IRS) proteins.70 Several clinical studies have found a connection between 2 DM and neurodegenerative disorders, including memory decline. Longitudinal studies have shown that Glu intolerance and impaired insulin secretion are associated with a higher risk of developing dementia or AD.70–72
Diabetes mellitus, being a condition caused by either insufficient insulin or insulin resistance, falls under the field of endocrinology. Compared to non-diabetic individuals, people with diabetes face a significantly higher risk of experiencing cardiovascular events, along with worse outcomes from cardiovascular disease, including a notably higher mortality rate.73 The American Heart Association even refers to diabetes as “a cardiovascular disease”.74 This dual view of diabetes as both an endocrine and systemic vascular disorder is supported by two key factors: (1) the heightened cardiovascular risk related to even early, mild cases of diabetes, and (2) the importance of not only managing blood sugar levels but also identifying and addressing other cardiovascular risk factors to reduce morbidity and mortality in affected patients.75
Diabetic kidney disease (DKD) is a leading cause of both morbidity and mortality in individuals with diabetes mellitus and the primary cause of end-stage renal disease globally.76 The kidney's critical role in Glu homeostasis is now widely understood. For instance, renal gluconeogenesis significantly contributes to overall Glu production in the postabsorptive state. The kidney helps regulate Glu levels by filtering and reabsorbing Glu. Typically, Glu filtered by the glomeruli is fully reabsorbed, but in cases of hyperglycemia or reduced reabsorptive function, glucosuria can occur,77 as illustrated in Fig. 2.
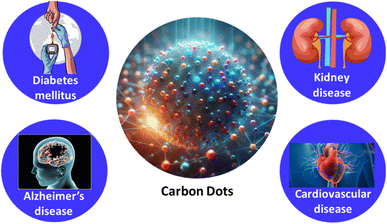 |
| Fig. 2 Common glucose-related diseases. | |
Most current articles on Glu monitoring primarily concentrate on the medical applications of Glu measurement using various sensors. In contrast, there is a scarcity of comprehensive reviews that systematically explore the underlying principles of Glu detection. This review is the first to focus specifically on CDs for Glu detection, opening new avenues for a deeper understanding of this technology and its potential applications.
3. Synthesis methods
Compared to other nanoparticles, CDs offer advantages in their synthesis methods, with multiple options available some of which are environmentally friendly30,78. They can be produced through a “bottom-up” approach79,80 such as using small organic molecules such as glucose81 and urea,82 or even biological materials such as biowaste,60 animal products,83,84 and plant extracts,49 which chemically assemble into nanoparticles.85,86 Alternatively, a “top-down” method involves breaking down larger pure carbon materials such as carbon black,87 graphite oxide,88 carbon nanotubes,89 or graphite into nanoparticles.90,91
The bottom-up method offers several important benefits over the top-down approach,92 including being more eco-friendly93–95 and less time-consuming96 and enabling simple adjustments to the surface state and composition of the CDs.97–99 These benefits make bottom-up methods more prevalent in the literature and contribute to CDs being an ideal choice over other nanoparticle types. In this process, the organic compound is dissolved in a solvent and heated until it experiences dehydration and carbonization.100–102 This method can be accomplished using different techniques, including hydrothermal carbonization,103,104 microwaving,105,106 combustion,107–109 and pyrolysis.110 Each technique has its own pros and cons regarding efficiency, time, cost, and energy consumption, and they produce CDs of different sizes and compositions,111,112 as summarized in Fig. 3.
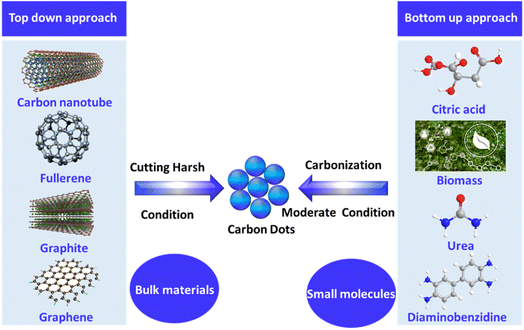 |
| Fig. 3 Methods for synthesizing CDs: top-down versus bottom-up approaches. | |
3.1 Bottom up synthesis methods
The bottom-up approach involves hydrothermal carbonization, solvothermal synthesis, microwaving, ultrasonic synthesis, pyrolysis and combustion.
3.1.1 Hydrothermal and solvothermal synthesis of CDs.
Hydrothermal carbonization is widely used in scientific research and is considered relatively simple and low-cost.113,114 It utilizes non-toxic starting materials to achieve controlled CD formation, enabling straightforward adjustments to their composition.115,116 This method requires a specially designed reaction vessel capable of withstanding the high temperatures necessary for carbonization while trapping emitted vapors to raise the reaction pressure.117–119 Containing the vapors enhances the efficiency of digesting organic materials, and the sample can be heated longer without significant evaporation loss.120 CDs produced through this method typically exhibit high photoluminescence quantum yields;121 however, drawbacks include non-uniform particle sizes, impurities that are difficult to remove, and possible variations in photoluminescence behavior among CDs within the same sample.122,123 In addition to hydrothermal fabrication, the solvothermal method for preparing CDs offers the benefits of being cost-effective and requiring simple equipment.124 Unlike the hydrothermal process, where water is used as the solvent, the solvothermal approach uses one or more solvents in a sealed Teflon-lined steel autoclave.125,126 The mixture of solvent and carbon source undergoes a reaction under high pressure and temperature conditions.127
3.1.2 Microwave-assisted synthesis of CDs.
Microwaves, a form of electromagnetic radiation, can serve as an alternative heating method to ovens used in hydrothermal carbonization. Similar vessels can be employed but made from nonmetallic materials to enhance the digestion efficiency of organic materials.128,129 The main benefit of microwaving is the strong interaction between the carbon source and electromagnetic radiation, which allows for fast and localized heating.130–132 This approach is energy-efficient, eco-friendly, and generally regarded as simpler than many other CD synthesis methods.53,133 Nevertheless, the resulting CDs may have a wide size distribution, and separating them from the solution can be challenging.134,135 As a result, CDs are frequently synthesized using microwave-assisted techniques.
3.1.3 Ultrasonic synthesis of CDs.
Ultrasonic treatment has been recognized as an effective method for producing various CDs, leading to numerous studies on its application for their synthesis. In this process, carbon precursors, along with acids, alkalis, and other oxidizing agents, are subjected to intense ultrasound waves, causing the carbon particles to break down into nanoscale particles. Continuous cavitation of the molecules occurs during the process. The high energy of the ultrasonic waves eliminates the need for complex post-treatment procedures, enabling the simple synthesis of small-sized CDs.136,137 As a result, the ultrasonic synthesis method triggers the reaction using the thermal effects of cavitation and ultra-high-frequency vibrations. It offers unmatched benefits, including environmental friendliness, cost-effectiveness, strong penetration, and consistent results.138 Therefore, high-performance CDs can be produced by combining ultrasound with other techniques.
3.1.4 Pyrolysis and combustion synthesis of CDs.
Both pyrolysis and combustion involve thermal decomposition methods but differ in atmospheric conditions: pyrolysis occurs in a low-oxygen or an oxygen-free environment, while combustion requires oxygen.107,110,139,140 These techniques may require strong acids or bases to initiate the digestion of the carbon precursor, making them less environmentally friendly.60,141 CDs synthesized via pyrolysis tend to have relatively high photoluminescence QYs, but the process is time-consuming, requires specific setups, and the CDs are difficult to separate from the solution.142,143 Additionally, producing CDs through combustion is regarded as one of the most efficient, low-cost, simple, and rapid one-step methods, relying on the carbonization of precursor molecules. Carbonization is a chemical process where organic materials undergo extended pyrolysis in an inert atmosphere, resulting in solid residues with a higher carbon content.96
3.1.5 Microfluidic synthesis.
The concept of microfluidics was first introduced by Manz in the 1990s.144 With advancements in micromachining, microfluidic technology has rapidly evolved, making significant strides in various fields. Compared to traditional chemical synthesis methods, microfluidic technology offers several distinct advantages.145 Microfluidic systems, known for their precise control over reaction conditions, have attracted the attention of researchers for generating nanomaterials. Previously, studies on quantum dots (QDs) were mostly conducted in batch reactors. Although these reactors were relatively simple, they struggled with uncontrollable reactions and challenges in maintaining consistency across different batches. In contrast, microfluidic-based reactors provide precise control over reaction conditions, such as temperature, pressure, and concentration distribution.146–149 Most bottom-up synthesis methods for QDs involve two key phases: nucleation and growth. These processes can be finely tuned in microreactors to produce QDs with specific sizes, morphologies, and compositions. Furthermore, due to the high sealing efficiency of microreactors, the need for inert gas protection typically required in batch production can often be eliminated in the microfluidic synthesis of many QD types.150 Microfluidic approaches to CDs generally enhance synthesis efficiency, increase quantum yields, and have the potential for large-scale production.
3.2 Top-down method
The top-down approach encompasses techniques such as arc discharge, laser ablation, electrochemical methods, and chemical oxidation.
The arc discharge method involves continuous electrical discharge between the cathode and anode, which generates high temperatures, gradually consuming the anode and forming CDs.151 Since 2004, it has gained popularity as a quick preparation method. However, CDs produced this way have inconsistent particle sizes and uncontrollable morphology, leading to the method being rarely used today.152,153 The laser ablation method employs a high-energy laser as a light source to irradiate carbon materials and produce CDs.154 This method allowed for the specific functionalization of CD surfaces, enhancing their fluorescence properties.155 The chemical oxidation method uses strong oxidizing agents to synthesize CDs. The primary advantage of this method is its ability to enhance the water solubility of CDs, and it remains a widely used technique for this purpose.156 The electrochemical method uses graphite rods as the carbon source to produce CDs through electrochemical treatment.157 In 2012, Shinde and Dhanraj discovered that this method allows control over the size of CDs by adjusting factors such as potential, electrolyte concentration, and reaction temperature. This method is popular for its simplicity, high yield, and production of uniformly sized particles.158
4. Sensing mechanism
In theory, any fluorescence variation such as changes in intensity, anisotropy, wavelength, or lifetime associated with the concentration of various analytes can serve as a basis for sensors. This section provides a concise overview of these sensing mechanisms.
4.1 Förster resonance energy transfer
FRET (Förster Resonance Energy Transfer) involves the nonradiative transfer of energy between an excited donor and an acceptor through long-range dipole–dipole interactions. In 1948, Theodor Förster introduced an equation that quantifies the efficiency of electronic excitation transfer between an energy donor (D) and acceptor (A).159 CDs are frequently utilized as donors. Currently, there are primarily two types of FRET-based CD probes. The first type involves the significant overlap between the emission spectrum of CDs and the absorption spectrum of the target, allowing FRET to occur from CDs to targets when they are at an appropriate distance. This interaction leads to a decrease in the fluorescence intensity of the CDs or a shift in the emission wavelength toward either the blue or red end of the spectrum. In the second scenario, FRET occurs from CDs to the quencher, resulting in reduced fluorescence intensity of the CDs. However, when a target is present, the distance between the CDs and the quencher increases, halting FRET and thereby increasing the fluorescence intensity of the CDs. As illustrated in Fig. 4.
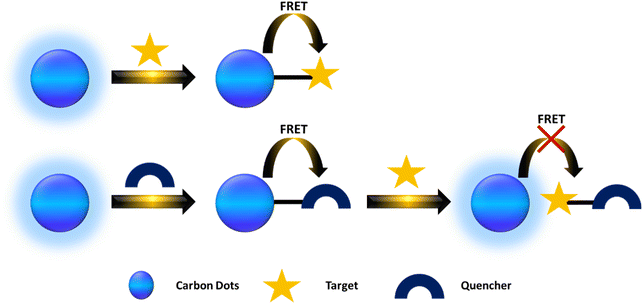 |
| Fig. 4 A scheme describing FRET from the CDs to the quencher and target. | |
4.2 Inner filter effect (IFE)
The fluorescence intensity observed is proportional to the intensity of the excitation light, and the quantum yield is slightly lower than that seen in an infinitely dilute solution. This phenomenon is known as the IFE, which can result in a reduction of the excitation intensity at the observation point or a decrease in the observed fluorescence intensity due to absorption.160
Currently, there are two primary types of IFE-based fluorescent probes. The first type occurs when the absorbance spectrum of the analyte significantly overlaps with the excitation or emission spectrum of CDs, thereby directly inducing the IFE. The second type involves a situation where the absorption spectrum does not align well with the CD spectrum; instead, a component (A) is introduced to react with the analyte to form a product. As illustrated in Fig. 5.
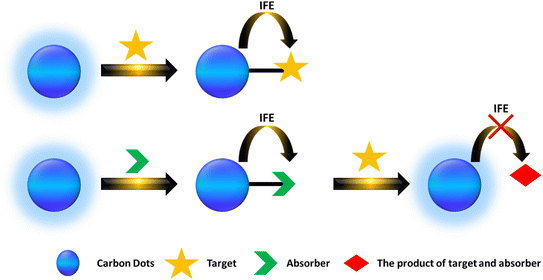 |
| Fig. 5 A scheme showing the IFE from the CDs to the quencher and target. | |
4.3 Photoinduced electron transfer (PET)
PET is a process involving the transfer of electrons between an electron donor (D) and an electron acceptor (A). As illustrated in Fig. 6, there are two forms of PET. When excited using light, the electrons in a fluorophore move from the highest occupied molecular orbital (HOMO) to the lowest unoccupied molecular orbital (LUMO). If an acceptor is present with a HOMO energy higher than that of the fluorophore, electron transfer can occur from the acceptor to the fluorophore, a phenomenon known as a-PET. The change in fluorescence of the fluorophore due to a-PET can serve as a signal for detecting the acceptor or target, as the presence of either reduces a-PET. The second form is called b-PET, in which electron transfer takes place from the fluorophore to the acceptor because the fluorophore's LUMO is higher than that of the acceptor.161 A decrease in fluorophore fluorescence indicates the presence of the acceptor, while an increase in fluorescence can signal the detection of a target that binds to the acceptor, preventing it from accepting the excited electron from the fluorophore.
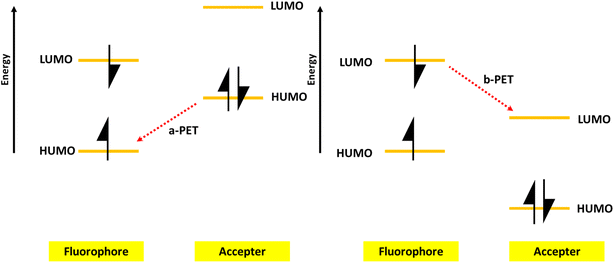 |
| Fig. 6 A scheme showing PET from the CDs to the quencher and target. | |
4.4 Static quenching mechanism
Static quenching occurs when a nonfluorescent ground-state complex is formed through the interaction between CDs and a quencher. When this complex absorbs light, it quickly returns to the ground state without emitting a photon.162 Static quenching exhibits several characteristics: (1) the absorption spectrum may change due to the formation of a ground-state complex. (2) The fluorescence may increase with temperature because of the dissociation of the weakly bound quencher. (3) The fluorescence lifetimes of the CDs remain constant. Because the static quenching mechanism can be easily confirmed, it is frequently utilized for analyte detection.
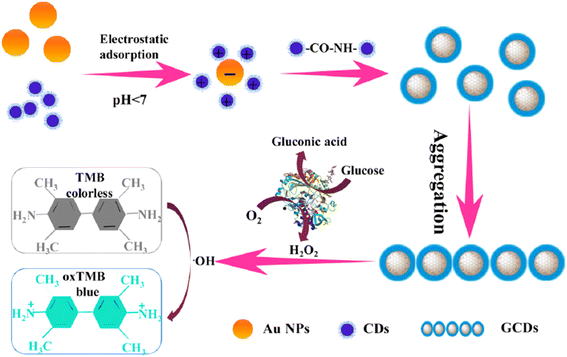 |
| Fig. 7 A schematic illustration for the synthesis of GCDs and detection of Glu with permission. Copyright 2021, Elsevier.167 | |
5. Applications
CDs, owing to their remarkable properties such as exceptional photoluminescence, low toxicity, abundant precursor sources, easy surface functionalization, and excellent biocompatibility, are promising zero-dimensional carbon-based nanomaterials.163,164 They are widely used in bioimaging to visualize cells and tissues, providing a non-toxic alternative for diagnosing diseases. CDs also show great potential in drug delivery systems, where they can be functionalized to target specific tissues, enhancing treatment precision. In biosensing, CDs assist in detecting biomolecules such as glucose and cancer markers, enabling early and accurate diagnoses. Additionally, CDs play a role in cancer therapy through photodynamic and photothermal treatments, helping to target and destroy cancer cells. These unique characteristics make them suitable candidates for use as Glu sensors. This review aims to examine cutting-edge CD-based optical sensors and their sensing capabilities.
5.1 Single probe sensing
In this section, we explore the use of single-probe sensors based on CDs for precise Glu measurement in various samples. These sensors are not only cost-effective but also exhibit low toxicity, making them ideal for practical applications. The sensor system typically consists of CDs or a combination of CDs with other materials, designed to accurately detect and quantify Glu concentrations. This approach leverages the unique properties of CDs, such as strong photoluminescence and ease of surface modification, to enhance sensitivity and reliability in Glu sensing.
Chen et al. developed165 a sensitive and selective Glu sensor using thiol-functionalized CDs (S-CDs). Upon exposure to H2O2, the S-CDs were converted into non-luminous assemblies due to the target-initiated oxidation of –SH groups into –S–S– bonds. Since Glu can be broken down into H2O2 by Glu oxidase, the S-CDs were used to detect Glu with a low detection limit (LOD) of 0.03 μM, a dynamic range of 0.1–50 μM, and high selectivity for distinguishing Glu from other sugars.
In this study, a molecularly imprinted electrochemical sensor (MIECS) was developed for Glu detection using a glassy carbon electrode (GCE) modified with hollow nickel nanospheres (HNiNSs), CDs, and chitosan (CS). Under optimal conditions, the sensor exhibited two wide linear ranges for Glu concentrations: 0.03–10 μM and 20–300 μM, with a LOD of 4.6 nM. The sensor was successfully applied to detect Glu in human serum and fermentation samples.166
Gan et al. developed167 Au/CDs(GCDs) for Glu detection using the surface-enhanced Raman scattering (SERS) method, which exhibited enhanced peroxidase-like activity. Based on this property, GCD composites were further utilized to detect Glu, achieving a LOD as low as 5 × 10−7 M, with a working range of 0 to 50 μM, as shown in Fig. 7.
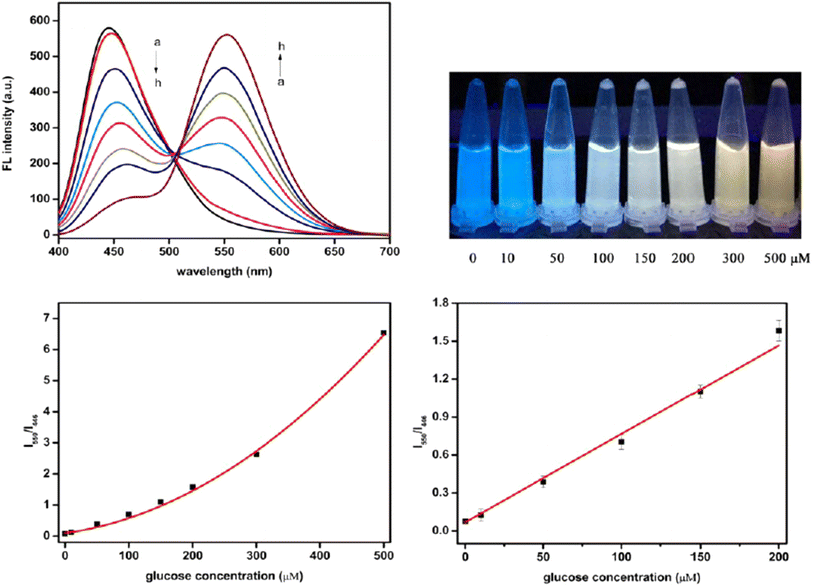 |
| Fig. 8 Visual detection of Glu based on CDs with permission. Copyright 2024, Elsevier.204 | |
Mutuyimana et al. developed168 orange-red emissive CDs whose FL is significantly quenched by hydrogen peroxide. Since the oxidation of Glu by Glu oxidase (GOx) generates H2O2, which quenches the FL through static quenching, a fluorometric method was designed for Glu detection. Under optimal conditions, the method exhibited a linear response for Glu concentrations ranging from 0.5 to 100 μM, with a LOD of 0.33 μM.
Doping CDs with different metal atoms enhances their photophysical properties due to surface passivation. Metal-doped CDs not only modify the photophysical behavior of the CDs but also give them multifunctional characteristics, even though the exact mechanism behind their photoluminescence remains unclear. For example, the synthesized Cu-doped CDs demonstrated peroxidase-like activity that surpassed that of horseradish peroxidase. As a result, the Cu-CD-based CL sensing method was effectively used for sensitive Glu detection, with a low LOD of 0.32 μM and a linear range of 1–48 μM.169
In another study, bifunctional Ag-CDs were synthesized, and a simple, sensitive dual-mode sensing platform utilizing colorimetric and SERS techniques was developed for detecting Glu in body fluids. This method took advantage of the Ag-CDs' strong peroxidase-like and SERS activities. The colorimetric detection of Glu showed a linear range of 50–800 μM with a LOD of 11.30 μM, while the SERS mode exhibited a linear range of 10–800 μM with a LOD of 3.54 μM.170
In this section, we examine in greater detail the most commonly used CDs for visual Glu sensing platforms, as summarized in Table 1.
Table 1 List of selected CDs, probes, real samples, QY, methods, dynamic ranges, and LOD values
Color |
Sample |
QY% |
Method |
Dynamic range |
LOD |
Ref. |
Green CDs |
Serum |
2.74 |
FL enhancement |
0.2–6 μM |
0.12 μM |
171
|
Blue NCDs |
Urine |
— |
Electrochemical |
1 × 10−5 to 5 × 10−3 M |
3.8 × 10−7 |
172
|
Green rGO-PBA |
— |
— |
FL quenching |
0.01–0.35 M |
— |
173
|
Blue Pd-CDs |
Fruit juice |
— |
Colorimetric |
0–100 μM |
0.2 μM |
174
|
Blue CD/V2O5 |
Serum |
— |
Colorimetric |
0.7–300 μM |
0.7 μM |
175
|
Green CDs |
Serum |
71.7 |
FL quenching |
10–240 μM |
0.92 μM |
176
|
Blue CDs |
Serum |
58.7 |
FL quenching |
0.2–20 mM |
0.15 μM |
177
|
CDs |
— |
— |
Colorimetric |
0–500 μM |
87.3 nM |
178
|
Blue Gd-CDs |
Serum |
— |
FL quenching |
0.6–40.0 μM |
0.6 μM |
179
|
Blue CDs |
Serum |
— |
FL quenching |
1–30 mM |
1 mM |
180
|
Blue CDs/MnO2 |
Serum |
21.63 |
FL enhancement |
2–200 μM |
0.83 μM |
181
|
CDs |
Serum |
— |
Colorimetric |
0.010–0.40 mM |
2 μM |
182
|
Blue CDs/AgNPs |
Serum |
— |
FL enhancement |
2–100 μM |
1.39 μM |
183
|
Blue CDs |
Serum |
— |
FL quenching |
0.5–7 mM |
0.058 mM |
184
|
SCDs |
Saliva |
22.63 |
FL quenching |
1–250 μM |
0.57 μM |
185
|
Green CDs |
Milk |
— |
FL quenching |
10–100 μM |
0.686 μM |
186
|
Blue CDs |
Glial cells |
9.91 |
FL quenching |
0.3–15 μM |
0.1 μM |
187
|
CDs |
Serum |
— |
Electrochemical |
1–12 mM |
0.25 mM |
188
|
Blue CDs |
Serum |
— |
FL quenching |
9–900 μM |
1.5 μM |
189
|
Blue CDs |
Serum |
9.06 |
FL quenching |
0–30 mM |
— |
190
|
Blue CDs |
Urine, serum |
— |
FL enhancement |
20–150 μM |
3.9 μM |
191
|
Blue CDs |
Serum |
— |
FL quenching |
5–750 μM |
0.5 μM |
192
|
CDs |
Serum |
— |
Electrochemical |
0.5–40 μM, 50–600 μM |
0.09 μM |
193
|
5.2 Ratiometric sensor
A single probe sensor can be influenced by external factors such as the intensity of excitation light, environmental interference, and probe concentration. These factors may lead to fluctuations in FL intensity, compromising the accuracy and reliability of measurements.22 To address these challenges, ratiometric fluorescent probes have been designed, offering self-calibration through two emission peaks that respond differently to the target analyte. Additionally, ratiometric fluorescent probes allow for visual detection by showing color changes under UV light, enhancing both the convenience and efficiency of the analysis process.194,195 Developing an innovative ratiometric sensing method holds great promise and importance for the easy detection of Glu.
Cho et al. prepared196 a ratiometric FL Glu probe based on CDs, where the signal originated from blue CDs and green rhodamine 6G as the reference signal. A change in probe FL color, from blue to green, was observed as the Glu concentration increased. The probe showed a linear range of 0.1–500 μM with a LOD of 0.04 μM. A stable solid-state probe using a hydrogel film exhibited a similar ratiometric FL color change and sensitivity, with a linear range of 0.5–500 μM and an LOD of 0.08 μM.
In this research, a new Fe-doped CD ratiometric probe was fabricated. This probe consisted of blue doped CDs and 2,3-diaminophenazine (DAP), produced by the oxidation of o-phenylenediamine (OPD) by Fe-CDs in the presence of H2O2, which emitted a yellow signal. The responses can be used to accurately quantify H2O2 and Glu in the ranges of 0–133 μM and 0–300 μM, with LOD of 0.47 μM and 2.5 μM, respectively.197
Wen-Sheng and colleagues developed CDs functionalized with boronic acid. This ratiometric probe, which relies on the boronic acid groups on the CDs' surface, interacts with the cis-diol groups of Glu to form a coordination compound, leading to FL quenching of the C-dots caused by their aggregation. Moreover, the aggregation of the C-dots simultaneously enhanced resonance light scattering (RLS) due to the presence of two pairs of cis-diol groups. As a result, a hybrid ratiometric chemosensor for Glu was designed. Importantly, the inert surface of the CDs allows this probe to measure Glu over a dynamic range of 10 to 2000 μM, with a LOD as low as 10 μM.198
A novel ratiometric FL sensor was developed using carbon quantum dots (CQDs) and o-diaminobenzene (ODB). The sensor was used to detect various concentrations of Glu standard solutions (as shown in Fig. 8). As the Glu concentration increased, the FL peak of CQDs at 446 nm gradually decreased, while the peak of oxidized ODB (oxODB) at 550 nm steadily increased. To quantify the relationship between the FL changes of CQDs and oxODB and Glu concentration, a linear fit was applied to the FL ratio of oxODB to CQDs against the Glu concentration. The FL ratio demonstrated a strong linear correlation with Glu concentrations in the range of 10 to 200 μM, with a LOD of 1.15 μM.199
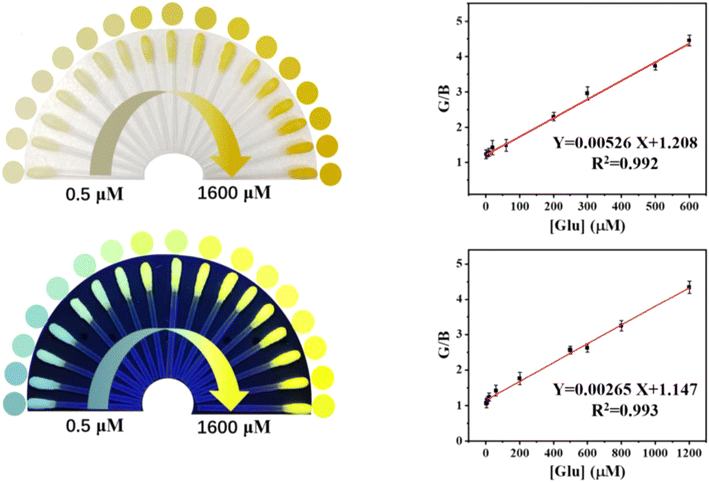 |
| Fig. 9 The ratiometric FL spectra for detecting Glu with permission. Copyright 2021, MDPI.199 | |
A novel fluorescent probe for Glu detection was developed using a platform combining NCDs and CdTe quantum dots (CdTe QDs). This ratiometric probe, based on blue CDs and yellow CdTe QDs, was successfully applied for Glu detection under optimized experimental conditions. The probe demonstrated a wide linear range of 26–900 μM with a LOD of 7.86 μM.200
5.3 Visual detection
Colorimetric sensing is a favored approach for analytical chemists, as it enables swift on-site detection through visible color changes.201 Visual detection has long captivated scholars, and the development of portable optical sensors for qualitative and semi-quantitative analysis of various substances has further drawn attention from researchers in many disciplines. This method allows analytes to be identified without relying on complex external instruments.30 Therefore, creating sensitive and selective methods for visually detecting Glu in various samples is highly important.
In this study, a visual detection method for Glu was developed. Glu was specifically broken down by Glu oxidase (a natural enzyme), generating H2O2, which was then catalyzed by CDs (FeMn/N-CDs, a nanozyme) to accelerate the conversion of o-phenylenediamine (OPD, colorless) into 2,3-diaminophenazine (DAP, yellow). As a result, the absorbance at 450 nm increased with higher Glu concentrations, causing a color change from colorless to yellow. Simultaneously, the FL of FeMn/N-CDs at 450 nm gradually decreased, while the FL of DAP at 550 nm increased, enabling dual-mode detection via both ratiometric FL and colorimetry. The portable swabs created for this purpose had dynamic ranges of 1–600 μM (LOD = 0.37 μM) for colorimetric detection and 4–1200 μM (LOD = 1.19 μM) for fluorometric detection,202 as shown in Fig. 9.
Bandi et al. developed203 Fe-doped CDs(FeCDs) with high peroxidase (POD)-like activity, which were immobilized on cellulose nanofibrils (CNFs) to create a composite paper. The POD activity of this paper was assessed through the oxidation of TMB and applied for the colorimetric detection of H2O2 and Glu. CNFs served as a support for the nanozyme, providing reusability, while also adsorbing the chromogen during the reaction, leading to a visible color change, making it suitable as a test strip for portable, on-site detection. A smartphone was used to monitor the color change, simplifying and reducing the cost of the detection process. This method offered linear detection ranges of 10–70 μM and a LOD of 1.73 μM for Glu.
In this study, N-CDs with excellent peroxidase-like activity were synthesized. These N-CDs catalyzed the oxidation of the chromogenic substrate TMB in the presence of H2O2, producing a blue oxidized product (TMBox) with an absorption peak at 654 nm. A smartphone-based colorimetric method was developed to quantitatively detect TMBox, recording the 1/L values (L representing lightness in the HSL color space). Since H2O2 is a byproduct of Glu oxidation in the presence of Glu oxidase (GOx), this method was adapted for sensitive and selective Glu detection, with a LOD of 1.09 μM.204
In this section, we examine in greater detail the most commonly used CDs for visual Glu sensing platforms, as summarized in Table 2.
Table 2 List of selected CDs, probes, real samples, QY, methods, dynamic ranges, and LOD values
Color |
Sample |
QY% |
Method |
Dynamic range |
LOD |
Ref. |
Green CDs |
Human plasma |
29.03 |
Solution assay |
1–10 ppm |
2.53 ppm (13.98 μM) |
205
|
Blue CDs |
Serum and urine |
11 |
Paper platform |
10−6 to 10−5 M |
— |
206
|
Blue CDs |
Saliva |
— |
Paper platform |
10.0 × 10−6, 40.0 × 10−6 M |
2.60 × 10−6 M |
207
|
Green BCNP |
Serum |
46 |
Solution assay |
32 μM to 2 mM |
8 μM |
208
|
Blue Fe-CDs |
Serum |
19.11 |
Solution assay |
— |
— |
209
|
Green R-CDs/B2O3 |
Serum |
6.70 |
Portable test gel |
0–20 mM |
— |
210
|
6. Conclusions and prospects
CDs are gaining significant attention due to their exceptional properties, non-toxic nature, availability, and simple synthesis process. Since their discovery, extensive research has been conducted on CDs, with the current focus shifting towards sustainable development, a critical necessity for this century. This review primarily highlights the latest advancements in the use of CDs for the detection of Glu. To advance this field, we have compiled and emphasized reliable synthetic methods for CDs while highlighting key accomplishments in various sensing techniques that utilize CDs for Glu detection. Despite significant progress, several limitations and challenges still require attention.
Despite the progress made in CD research, many challenges remain. Predicting the photophysical behavior and selectivity of CDs for specific targets, such as Glu, is still difficult, as is optimizing CD synthesis. Additionally, inconsistencies in CD properties and selectivity, even when synthesized from the same source, highlight the need for further investigation. The enhanced efficiencies observed in Glu detection also require more in-depth conceptual analysis.
Moreover, the practical use of CDs remains largely confined to laboratory settings. There is an urgent need to advance this technology for real-time, on-site Glu detection and to develop faster, simpler detection methods for broader applications. Extensive research is essential to overcome these challenges and fully realize the potential of CDs in practical scenarios. Additionally, producing CDs in large yields is still of interest and in demand for real industrial applications, making further research on this important aspect necessary.
In conclusion, CD-based fluorescent probes show significant promise for Glu detection, but further research is needed to refine the design of CDs to improve their efficiency in real-world applications. We hope that this review will inspire greater interest in the development and optimization of CDs, ultimately driving their widespread use in Glu detection in the near future.
Data availability
Data sharing is not applicable to this article as no datasets were generated or analysed during the current study.
Conflicts of interest
There are no conflicts to declare.
Acknowledgements
We extend our deepest appreciation to the Department of Chemistry, College of Science, at the University of Sulaimani and Charmo University for providing the necessary resources and facilities for conducting this research.
References
- S. Wild, G. Roglic, A. Green, R. Sicree and H. King, Global prevalence of diabetes: estimates for the year 2000 and projections for 2030, Diabetes Care, 2004, 27(5), 1047–1053 CrossRef
.
- M. Singh, P. K. Kathuroju and N. Jampana, Polypyrrole based amperometric glucose biosensors, Sens. Actuators, B, 2009, 143(1), 430–443 CrossRef
.
- J. L. Cano Perez, J. Gutiérrez-Gutiérrez, C. Perezcampos Mayoral, E. L. Pérez-Campos, M. del S. Pina Canseco and L. Tepech Carrillo,
et al., Fiber optic sensors: a review for glucose measurement, Biosensors, 2021, 11(3), 61 CrossRef
.
- J. Zhang, J. Ma, S. Zhang, W. Wang and Z. Chen, A highly sensitive nonenzymatic glucose sensor based on CuO nanoparticles decorated carbon spheres, Sens. Actuators, B, 2015, 211, 385–391 CrossRef CAS
.
- R. Reghunath and K. K. Singh, Recent advances in graphene based electrochemical glucose sensor, Nano-Struct. Nano-Objects, 2021, 26, 100750 CrossRef CAS
.
- G. Wang, X. He, L. Wang, A. Gu, Y. Huang and B. Fang,
et al., Non-enzymatic electrochemical sensing of glucose, Microchim. Acta, 2013, 180, 161–186 CrossRef CAS
.
- Q. Dong, H. Ryu and Y. Lei, Metal oxide based non-enzymatic electrochemical sensors for glucose detection, Electrochim. Acta, 2021, 370, 137744 CrossRef CAS
.
- D. Thatikayala, D. Ponnamma, K. K. Sadasivuni, J.-J. Cabibihan, A. K. Al-Ali and R. A. Malik,
et al., Progress of advanced nanomaterials in the non-enzymatic electrochemical sensing of glucose and H2O2, Biosensors, 2020, 10(11), 151 CrossRef CAS
.
- M. S. Mustafa, N. N. Mohammad, F. H. Radha, K. F. Kayani, H. O. Ghareeb and S. J. Mohammed, Eco-friendly spectrophotometric methods for concurrent analysis of phenol, 2-aminophenol, and 4-aminophenol in ternary mixtures and water samples: assessment of environmental sustainability, RSC Adv., 2024, 14(23), 16045–16055 RSC
.
- X. Sun, Glucose detection through surface-enhanced Raman spectroscopy: A review, Anal. Chim. Acta, 2022, 1206, 339226 CrossRef CAS
.
- R. Pandey, S. K. Paidi, T. A. Valdez, C. Zhang, N. Spegazzini and R. R. Dasari,
et al., Noninvasive monitoring of blood glucose with Raman spectroscopy, Acc. Chem. Res., 2017, 50(2), 264–272 CrossRef CAS PubMed
.
- M. Liu, Z. Lin and J.-M. Lin, A review on applications of chemiluminescence detection in food analysis, Anal. Chim. Acta, 2010, 670(1–2), 1–10 CrossRef CAS PubMed
.
- M. Iranifam, Analytical applications of chemiluminescence systems assisted by carbon nanostructures, TrAC, Trends Anal. Chem., 2016, 80, 387–415 CrossRef CAS
.
- L. Kergoat, B. Piro, M. Berggren, G. Horowitz and M.-C. Pham, Advances in organic transistor-based biosensors: from organic electrochemical transistors to electrolyte-gated organic field-effect transistors, Anal. Bioanal. Chem., 2012, 402, 1813–1826 CrossRef CAS PubMed
.
- M. Sophocleous, L. Contat-Rodrigo, E. García-Breijo and J. Georgiou, Organic electrochemical transistors as an emerging platform for bio-sensing applications: a review, IEEE Sens. J., 2020, 21(4), 3977–4006 Search PubMed
.
- M. M. Rahman, A. J. Ahammad, J.-H. Jin, S. J. Ahn and J.-J. Lee, A comprehensive review of glucose biosensors based on nanostructured metal-oxides, Sensors, 2010, 10(5), 4855–4886 CrossRef CAS PubMed
.
- Y. Liu, L. Yang and Y. Cui, Transdermal amperometric biosensors for continuous glucose monitoring in diabetes, Talanta, 2023, 253, 124033 CrossRef CAS
.
- J. C. Pickup, F. Hussain, N. D. Evans, O. J. Rolinski and D. J. S. Birch, Fluorescence-based glucose sensors, Biosens. Bioelectron., 2005, 20(12), 2555–2565 CrossRef CAS
.
- G. P. C. Mello, E. F. C. Simões, D. M. A. Crista, J. M. M. Leitão, L. Pinto da Silva and J. C. G. Esteves da Silva, Glucose sensing by fluorescent nanomaterials, Crit. Rev. Anal. Chem., 2019, 49(6), 542–552 CrossRef CAS
.
- J. C. Pickup, F. Khan, Z.-L. Zhi, J. Coulter and D. J. S. Birch, Fluorescence intensity-and lifetime-based glucose sensing using glucose/galactose-binding protein, J. Diabetes Sci. Technol., 2013, 7(1), 62–71 CrossRef
.
- K. F. Kayani, S. J. Mohammed, N. N. Mohammad, A. M. Abdullah, D. I. Tofiq and M. S. Mustafa,
et al., Sulfur quantum dots for fluorescence sensing in biological and pharmaceutical samples: a review, Materials Advances, 2024, 5, 6351–6367, 10.1039/D4MA00502C
.
- K. F. Kayani, S. J. Mohammed, N. N. Mohammad, G. H. Abdullah, D. A. Kader and N. S. Hamad Mustafa, Ratiometric fluorescence detection of tetracycline in milk and tap water with smartphone assistance for visual pH sensing using innovative dual-emissive phosphorus-doped carbon dots, Food Control, 2024, 164, 110611 CrossRef CAS
.
- M. Adeel, K. Asif, M. M. Rahman, S. Daniele, V. Canzonieri and F. Rizzolio, Glucose detection devices and methods based on metal–organic frameworks and related materials, Adv. Funct. Mater., 2021, 31(52), 2106023 CrossRef CAS
.
- K. F. Kayani and K. M. Omer, A red luminescent europium metal organic framework (Eu-MOF) integrated with a paper strip using smartphone visual detection for determination of folic acid in pharmaceutical formulations, New J. Chem., 2022, 46(17), 8152–8161, 10.1039/D2NJ00601D
.
- K. F. Kayani, N. N. Mohammad, D. A. Kader, S. J. Mohammed, D. A. Shukur and A. H. Alshatteri,
et al., Ratiometric Lanthanide Metal-Organic Frameworks (MOFs) for Smartphone-Assisted Visual Detection of Food Contaminants and Water: A Review, ChemistrySelect, 2023, 8(47), e202303472, DOI:10.1002/slct.202303472
.
- K. F. Kayani, Bimetallic metal–organic frameworks (BMOFs) for dye removal: a review, RSC Adv., 2024, 14(43), 31777–31796, 10.1039/D4RA06626J
.
- J. Zhang, L. Tu, S. Zhao, G. Liu, Y. Wang and Y. Wang,
et al., Fluorescent gold nanoclusters based photoelectrochemical sensors for detection of H2O2 and glucose, Biosens. Bioelectron., 2015, 67, 296–302 CrossRef CAS
.
- J. Chen, J. Ge, L. Zhang, Z. Li and L. Qu, Poly (styrene sulfonate) and Pt bifunctionalized graphene nanosheets as an artificial enzyme to construct a colorimetric chemosensor for highly sensitive glucose detection, Sens. Actuators, B, 2016, 233, 438–444 CrossRef CAS
.
- K. F. Kayani and A. M. Abdullah, Eco-Friendly Fluorescent Detection Method for Cu2+ ions Combined with Smartphone-Integrated Paper Strip Sensors Based on Highly Fluorescent 2-Aminoterephthalic Acid in Milk Samples, J. Food Compos. Anal., 2024, 106577 CrossRef CAS
.
- K. F. Kayani, S. J. Mohammed, D. Ghafoor, M. K. Rahim and H. R. Ahmed, Carbon dot as fluorescence sensor for glutathione in human serum samples: a review, Materials Advances, 2024, 5(11), 4618–4633, 10.1039/D4MA00185K
.
- X. Sun and Y. Lei, Fluorescent carbon dots and their sensing applications, TrAC, Trends Anal. Chem., 2017, 89, 163–180 CrossRef CAS
.
- C. G. Malar, S. Muthulingam, M. Murugesan, G. Srinivasan and R. Sankar, A comprehensive review of the importance of thermal activation in the production of carbon dots and the potential for their use in the bioenergy industry, J. Therm. Anal. Calorim., 2023, 148(2), 505–516 CrossRef CAS
.
- R. Manjupriya and S. M. Roopan, Carbon dots-based catalyst for various organic transformations, J. Mater. Sci., 2021, 56, 17369–17410 CrossRef CAS
.
- X. Li, M. Rui, J. Song, Z. Shen and H. Zeng, Carbon and graphene quantum dots for optoelectronic and energy devices: a review, Adv. Funct. Mater., 2015, 25(31), 4929–4947 CrossRef CAS
.
- H. Wu, H. Xu, Y. Shi, T. Yuan, T. Meng and Y. Zhang,
et al., Recent Advance in Carbon Dots: From Properties to Applications, Chin. J. Chem., 2021, 39(5), 1364–1388 CrossRef CAS
.
- S. Li, W. Su, H. Wu, T. Yuan, C. Yuan and J. Liu,
et al., Targeted tumour theranostics in mice via carbon quantum dots structurally mimicking large amino acids, Nat. Biomed. Eng., 2020, 4(7), 704–716 CrossRef CAS PubMed
.
- H. Xu, J. Chang, H. Wu, H. Wang, W. Xie and Y. Li,
et al., Carbon dots with guanidinium and amino acid functional groups for targeted small interfering RNA delivery toward tumor gene therapy, Small, 2023, 19(31), 2207204 CrossRef CAS
.
- K. F. Kayani and C. N. Abdullah, A Dual-Mode Detection Sensor Based on Nitrogen-Doped Carbon Dots for Visual Detection of Fe(III) and Ascorbic Acid via a Smartphone, J. Fluoresc., 2024 DOI:10.1007/s10895-024-03604-0
.
- K. F. Kayani, M. K. Rahim, S. J. Mohammed, H. R. Ahmed, M. S. Mustafa and S. B. Aziz, Recent Progress in Folic Acid Detection Based on Fluorescent Carbon Dots as Sensors: A Review, J. Fluoresc., 2024 DOI:10.1007/s10895-024-03728-3
.
- K. F. Kayani, O. B. A. Shatery, M. S. Mustafa, A. H. Alshatteri, S. J. Mohammed and S. B. Aziz, Environmentally sustainable synthesis of whey-based carbon dots for ferric ion detection in human serum and water samples: evaluating the greenness of the method, RSC Adv., 2024, 14(8), 5012–5021 RSC
.
- O. B. A. Shatery, K. F. Kayani, M. S. Mustafa and S. J. Mohammed, Rational design for enhancing sensitivity and robustness of a probe via encapsulation of carbon dots into a zeolitic imidazolate framework-8 for quantification of tetracycline in milk with greenness evaluation, Res. Chem. Intermed., 2024, 2291–2306, DOI:10.1007/s11164-024-05271-z
.
- S. J. Mohammed, F. E. Hawaiz, S. B. Aziz and S. H. Al-Jaf, Organic soluble nitrogen-doped carbon dots (ONCDs) to reduce the optical band gap of PVC polymer: Breakthrough in polymer composites with improved optical properties, Opt. Mater., 2024, 149, 115014 CrossRef CAS
.
- S. J. Mohammed, M. K. Sidiq, H. H. Najmuldeen, K. F. Kayani, D. A. Kader and S. B. Aziz, A Comprehensive Review on Nitrogen-Doped Carbon Dots for Antibacterial Applications, J. Environ. Chem. Eng., 2024, 12(6), 114444 CrossRef CAS
. https://www.sciencedirect.com/science/article/pii/S2213343724025752.
- E. A. Stepanidenko, E. V. Ushakova, A. V. Fedorov and A. L. Rogach, Applications of carbon dots in optoelectronics, Nanomaterials, 2021, 11(2), 1–20 CrossRef
.
- C. T. Tracey, D. O. Shavronskaya, H. Yang, P. V. Krivoshapkin and E. F. Krivoshapkina, Heterogeneous carbon dot catalysts for biodiesel production: A mini review, Fuel, 2024, 362, 130882 CrossRef CAS
.
- M. Behi, L. Gholami, S. Naficy, S. Palomba and F. Dehghani, Carbon dots: A novel platform for biomedical applications, Nanoscale Adv., 2022, 4(2), 353–376 RSC
.
- K. Ghosal and A. Ghosh, Carbon dots: The next generation platform for biomedical applications, Mater. Sci. Eng., C, 2019, 96, 887–903 CrossRef CAS PubMed
.
- N. Oladzadabbasabadi, M. A. Dheyab, A. M. Nafchi, M. Ghasemlou, E. P. Ivanova and B. Adhikari, Turning food waste into value-added carbon dots for sustainable food packaging application: a review, Adv. Colloid Interface Sci., 2023, 103020 CrossRef CAS PubMed
.
- M. Zulfajri, H. N. Abdelhamid, S. Sudewi, S. Dayalan, A. Rasool and A. Habib,
et al., Plant part-derived carbon dots for biosensing, Biosensors, 2020, 10(6), 1–37 CrossRef
.
- B. Wang, H. Cai, G. I. N. Waterhouse, X. Qu, B. Yang and S. Lu, Carbon Dots in Bioimaging, Biosensing and Therapeutics: A Comprehensive Review, Small Sci., 2022, 2(6), 2200012 CrossRef CAS
.
- J. Mohammed, P. K. Desu, J. R. Namratha and G. K. Rao, Applications of Carbon Dots (CDs) in Drug Delivery, Adv. Pharmacol. Pharm., 2023, 11(1), 36–45 CAS
.
- H. Lu, W. Li, H. Dong and M. Wei, Graphene quantum dots for optical bioimaging, Small, 2019, 15(36), 1902136 CrossRef
.
- T. N. J. I. Edison, R. Atchudan, M. G. Sethuraman, J. J. Shim and Y. R. Lee, Microwave assisted green synthesis of fluorescent N-doped carbon dots: Cytotoxicity and bio-imaging applications, J. Photochem. Photobiol., B, 2016, 161, 154–161, DOI:10.1016/j.jphotobiol.2016.05.017
.
- J. Wang, P. Zhang, C. Huang, G. Liu, K. C.-F. Leung and Y. X. J. Wáng, High performance photoluminescent carbon dots for in vitro and in vivo bioimaging: effect of nitrogen doping ratios, Langmuir, 2015, 31(29), 8063–8073 CrossRef CAS PubMed
.
- Z. Hallaji, Z. Bagheri, M. Oroujlo, M. Nemati, Z. Tavassoli and B. Ranjbar, An insight into the potentials of carbon dots for in vitro live-cell imaging: recent progress, challenges, and prospects, Microchim. Acta, 2022, 189(5), 190 CrossRef CAS PubMed
.
- C. He, X. Lin, Y. Mei, Y. Luo, M. Yang and Y. Kuang,
et al., Recent advances in carbon dots for in vitro/vivo fluorescent bioimaging: A mini-review, Front. Chem., 2022, 10, 905475 CrossRef CAS PubMed
.
- W. Su, H. Wu, H. Xu, Y. Zhang, Y. Li and X. Li,
et al., Carbon dots: a booming material for biomedical applications, Mater. Chem. Front., 2020, 4(3), 821–836 RSC
.
- D. Yang, H. Li, Q. Li, K. Li, F. Xiao and Y. Yang, Highly selective histamine assay via SERS: based on the signal enhancement of carbon dots and the fluorescence quenching of gold nanoparticles, Sens. Actuators, B, 2022, 350, 130866 CrossRef CAS
.
- X. Xu, R. Ray, Y. Gu, H. J. Ploehn, L. Gearheart and K. Raker,
et al., Electrophoretic analysis and purification of fluorescent single-walled carbon nanotube fragments, J. Am. Chem. Soc., 2004, 126(40), 12736–12737 CrossRef CAS PubMed
.
- M. Jorns and D. Pappas, A review of fluorescent carbon dots, their synthesis, physical and chemical characteristics, and applications, Nanomaterials, 2021, 11, 1448 CrossRef CAS PubMed
.
- L. Cui, X. Ren, M. Sun, H. Liu and L. Xia, Carbon dots: Synthesis, properties and applications, Nanomaterials, 2021, 11(12), 3419 CrossRef CAS PubMed
.
- M. Bacon, S. J. Bradley and T. Nann, Graphene quantum dots, Part. Part. Syst. Charact., 2014, 31(4), 415–428 CrossRef CAS
.
- H.-C. Wang and A.-R. Lee, Recent developments in blood glucose sensors, J. Food Drug Anal., 2015, 23(2), 191–200 CrossRef CAS PubMed
.
- N. S. Oliver, C. Toumazou, A. E. G. Cass and D. G. Johnston, Glucose sensors: a review of current and emerging technology, Diabetic Med., 2009, 26(3), 197–210 CrossRef CAS
.
- R. A. DeFronzo, E. Ferrannini, L. Groop, R. R. Henry, W. H. Herman and J. J. Holst,
et al., Type 2 diabetes mellitus, Nat. Rev. Dis. Prim., 2015, 1(1), 1–22 Search PubMed
.
- K. Fiedorova, M. Augustynek, J. Kubicek, P. Kudrna and D. Bibbo, Review of present method of glucose from human blood and body fluids assessment, Biosens. Bioelectron., 2022, 211, 114348 CrossRef CAS
.
-
E. Ginter and V. Simko, Type 2 diabetes mellitus, pandemic in 21st century, Diabetes An Old Dis a New Insight, 2013, pp. 42–50 Search PubMed
.
- R. A. L. De Sousa, A. R. Harmer, D. A. Freitas, V. A. Mendonça, A. C. R. Lacerda and H. R. Leite, An update on potential links between type 2 diabetes mellitus and Alzheimer's disease, Mol. Biol. Rep., 2020, 47, 6347–6356 CrossRef CAS PubMed
.
- K. Akter, E. A. Lanza, S. A. Martin, N. Myronyuk, M. Rua and R. B. Raffa, Diabetes mellitus and Alzheimer's disease: shared pathology and treatment?, Br. J. Clin. Pharmacol., 2011, 71(3), 365–376 CrossRef CAS
.
- J. A. Luchsinger, M.-X. Tang, S. Shea and R. Mayeux, Hyperinsulinemia and risk of Alzheimer disease, Neurology, 2004, 63(7), 1187–1192 CrossRef PubMed
.
- X. Li, D. Song and S. X. Leng, Link between type 2 diabetes and Alzheimer's disease: from epidemiology to mechanism and treatment, Clin. Interventions Aging, 2015, 549–560 CrossRef
.
- E. Ronnemaa, B. Zethelius, J. Sundelof, J. Sundstrom, M. Degerman-Gunnarsson and C. Berne,
et al., Impaired insulin secretion increases the risk of Alzheimer disease, Neurology, 2008, 71(14), 1065–1071 CrossRef CAS
.
- R. W. Nesto, Correlation between cardiovascular disease and diabetes mellitus: current concepts, Am. J. Med., 2004, 116(5), 11–22 CrossRef PubMed
.
- J. B. Buse, H. N. Ginsberg, G. L. Bakris, N. G. Clark, F. Costa and R. Eckel,
et al., Primary prevention of cardiovascular diseases in people with diabetes mellitus: a scientific statement from the American Heart Association and the American Diabetes Association, Circulation, 2007, 115(1), 114–126 CrossRef PubMed
.
- A. M. Wägner, A. Martínez-Rubio, J. Ordonez-Llanos and A. Pérez-Pérez, Diabetes mellitus and cardiovascular disease, Eur. J. Intern. Med., 2002, 13(1), 15–30 CrossRef PubMed
.
- Y.-C. Lin, Y.-H. Chang, S.-Y. Yang, K.-D. Wu and T.-S. Chu, Update of pathophysiology and management of diabetic kidney disease, J. Formosan Med. Assoc., 2018, 117(8), 662–675 CrossRef CAS PubMed
.
- O. Marsenic, Glucose control by the kidney: an emerging target in diabetes, Am. J. Kidney Dis., 2009, 53(5), 875–883 CrossRef CAS
.
- S. J. Mohammed, F. E. Hawaiz, S. B. Aziz and S. H Al-Jaf, Organic soluble nitrogen-doped carbon dots (ONCDs) to reduce the optical band gap of PVC polymer: Breakthrough in polymer composites with improved optical properties, Opt. Mater., 2024, 149(July 2023), 1–16 Search PubMed
.
- Y. Choi, Y. Choi, O. H. Kwon and B. S. Kim, Carbon Dots: Bottom-Up Syntheses, Properties, and Light-Harvesting Applications, Chem.–Asian J., 2018, 13(6), 586–598 CrossRef CAS PubMed
.
- D. M. A. Crista, J. C. G. E. da Silva and L. P. da Silva, Evaluation of different bottom-up routes for the fabrication of carbon dots, Nanomaterials, 2020, 10(7), 1–15 CrossRef PubMed
.
- A. Piasek, J. Pulit-Prociak, M. Zielina and M. Banach, Fluorescence of D-Glucose-Derived Carbon Dots: Effect of Process Parameters, J. Fluoresc., 2023, 34(4), 1693–1705 CrossRef PubMed
.
- R. Sendão, M. del V. M. de Yuso, M. Algarra, J. C. G. Esteves da Silva and L. Pinto da Silva, Comparative life cycle assessment of bottom-up synthesis routes for carbon dots derived from citric acid and urea, J. Cleaner Prod., 2020, 254, 1–10 CrossRef
.
- B. P. de Oliveira and F. O. M. da Silva Abreu, Carbon quantum dots synthesis from waste and by-products: Perspectives and challenges, Mater. Lett., 2021, 282, 128764 CrossRef CAS
.
- M. Kumari, G. R. Chaudhary, S. Chaudhary, A. Umar, S. Akbar and S. Baskoutas, Bio-Derived Fluorescent Carbon Dots: Synthesis, Properties and Applications, Molecules, 2022, 27(16), 5329 CrossRef CAS PubMed
.
- J. Vinoth Kumar, G. Kavitha, R. Arulmozhi, V. Arul, S. Singaravadivel and N. Abirami, Green Sources Derived Carbon Dots for Multifaceted Applications, J. Fluoresc., 2021, 31(4), 915–932 CrossRef CAS
.
- X. Zhang, M. Jiang, N. Niu, Z. Chen, S. Li and S. Liu,
et al., Natural-Product-Derived Carbon Dots: From Natural Products to Functional Materials, ChemSusChem, 2018, 11(1), 11–24 CrossRef
.
- R. B. González-González, L. T. González, M. Madou, C. Leyva-Porras, S. O. Martinez-Chapa and A. Mendoza, Synthesis, Purification, and Characterization of Carbon Dots from Non-Activated and Activated Pyrolytic Carbon Black, Nanomaterials, 2022, 12(3), 298 CrossRef PubMed
.
- Q. Wang, H. Zheng, Y. Long, L. Zhang, M. Gao and W. Bai, Microwave-hydrothermal synthesis of fluorescent carbon dots from graphite oxide, Carbon, 2011, 49(9), 3134–3140 CrossRef CAS
.
- D. B. Shinde and V. K. Pillai, Electrochemical preparation of luminescent graphene quantum dots from multiwalled carbon nanotubes, Chem.–Eur. J., 2012, 18(39), 12522–12528 CrossRef CAS
.
- J. Y. Lee, P. K. Mehta, S. Subedi and K.-H. Lee, Development of ratiometric fluorescent probes based on peptides for sensing Pb2+ in aquatic environments and human serum, Spectrochim. Acta, Part A, 2023, 294, 122502 CrossRef CAS
.
- J. Joseph and A. Anappara, White-Light Emitting Carbon Dots Prepared By the, ChemPhysChem, 2017, 292–298 CrossRef CAS
.
- F. Bruno, A. Sciortino, G. Buscarino, M. L. Soriano, Á. Ríos and M. Cannas,
et al., A comparative study of top-down and bottom-up carbon nanodots and their interaction with mercury ions, Nanomaterials, 2021, 11(5), 1265 CrossRef CAS
.
- N. Ullal, K. Muthamma and D. Sunil, Carbon dots from eco-friendly precursors for optical sensing application: an up-to-date review, Chem. Pap., 2022, 76, 6097–6127 CrossRef CAS
.
- H. Shabbir, T. Tokarski, D. Ungor and M. Wojnicki, Eco friendly synthesis of carbon dot by hydrothermal method for metal ions salt identification, Materials, 2021, 14(24), 1–13 CrossRef PubMed
.
- A. Shafi, S. Bano, S. Sabir, M. Zain Khan and M. Muzibur Rahman, Eco-Friendly Fluorescent Carbon Nanodots: Characteristics and Potential Applications, Carbon-Based Mater. Environ. Prot. Rem., 2020, 1–16 CAS
.
- A. Sharma and J. Das, Small molecules derived carbon dots: Synthesis and applications in sensing, catalysis, imaging, and biomedicine, J. Nanobiotechnol., 2019, 17(1), 1–24 CrossRef CAS PubMed
.
- F. T. Wang, L. N. Wang, J. Xu, K. J. Huang and X. Wu, Synthesis and modification of carbon dots for advanced biosensing application, Analyst, 2021, 146(14), 4418–4435 RSC
.
- F. Yan, Y. Jiang, X. Sun, Z. Bai, Y. Zhang and X. Zhou, Surface modification and chemical functionalization of carbon dots: a review, Microchim. Acta, 2018, 185(9), 424 CrossRef PubMed
.
- R. Cheng, Y. Xiang, R. Guo, L. Li, G. Zou and C. Fu,
et al., Structure and Interface Modification of Carbon Dots for Electrochemical Energy Application, Small, 2021, 17(40), 1–31 CrossRef PubMed
.
- M. L. Bhaisare, A. Talib, M. S. Khan, S. Pandey and H. F. Wu, Synthesis of fluorescent carbon dots via microwave carbonization of citric acid in presence of tetraoctylammonium ion, and their application to cellular bioimaging, Microchim. Acta, 2015, 182(13–14), 2173–2181 CrossRef CAS
.
- H. Ding, J. S. Wei, P. Zhang, Z. Y. Zhou, Q. Y. Gao and H. M. Xiong, Solvent-Controlled Synthesis of Highly Luminescent Carbon Dots with a Wide Color Gamut and Narrowed Emission Peak Widths, Small, 2018, 14(22), 1–10 CrossRef
.
- L. Fang, M. Wu, C. Huang, Z. Liu, J. Liang and H. Zhang, Industrializable synthesis of narrow-dispersed carbon dots achieved by microwave-assisted selective carbonization of surfactants and their applications as fluorescent nano-additives, J. Mater. Chem. A, 2020, 8(40), 21317–21326 RSC
.
- Y. Li, F. Liu, J. Cai, X. Huang, L. Lin and Y. Lin,
et al., Nitrogen and sulfur co-doped carbon dots synthesis via one step hydrothermal carbonization of green alga and their multifunctional applications, Microchem. J., 2019, 147(March), 1038–1047 CrossRef CAS
.
- R. Atchudan, S. Perumal, T. N. J. I. Edison, A. K. Sundramoorthy, R. Vinodh and S. Sangaraju,
et al., Natural Nitrogen-Doped Carbon Dots Obtained from Hydrothermal Carbonization of Chebulic Myrobalan and Their Sensing Ability toward Heavy Metal Ions, Sensors, 2023, 23(2), 787 CrossRef CAS
.
- T. V. De Medeiros, J. Manioudakis, F. Noun, J. R. Macairan, F. Victoria and R. Naccache, Microwave-assisted synthesis of carbon dots and their applications, J. Mater. Chem. C, 2019, 7(24), 7175–7195 RSC
.
- M. P. Romero, F. Alves, M. D. Stringasci, H. H. Buzzá, H. Ciol and N. M. Inada,
et al., One-Pot Microwave-Assisted Synthesis of Carbon Dots and in vivo and in vitro Antimicrobial Photodynamic Applications, Front. Microbiol., 2021, 12(June), 1–13 Search PubMed
.
- S. Zhang, L. Zhang, L. Huang, G. Zheng, P. Zhang and Y. Jin,
et al., Study on the fluorescence properties of carbon dots prepared via combustion process, J. Lumin., 2019, 206, 608–612 CrossRef CAS
.
- M. C. Rong, K. X. Zhang, Y. R. Wang and X. Chen, The synthesis of B, N-carbon dots by a combustion method and the application of fluorescence detection for Cu2+, Chin. Chem. Lett., 2017, 28(5), 1119–1124 CrossRef CAS
.
- K. Zouhri, L. J. Snyder, M. McFarland, P. O. Laubie, K. A. S. Fernando and C. E. Bunker, Combustion-Induced Endothermic Process in Carbon Dots Synthesized on Magnetite Nanoparticle Substrate, Crystals, 2024, 14(6), 1–14 CrossRef
.
- X. W. Tan, A. N. B. Romainor, S. F. Chin and S. M. Ng, Carbon dots production via pyrolysis of sago waste as potential probe for metal ions sensing, J. Anal. Appl. Pyrolysis, 2014, 105, 157–165 CrossRef CAS
.
- L. Guo, L. Li, M. Liu, Q. Wan, J. Tian and Q. Huang,
et al., Bottom-up preparation of nitrogen doped carbon quantum dots with green emission under microwave-assisted hydrothermal treatment and their biological imaging, Mater. Sci. Eng., C, 2018, 84(October), 60–66 CrossRef CAS PubMed
.
- Z. L. Wu, Z. X. Liu and Y. H. Yuan, Carbon dots: Materials, synthesis, properties and approaches to long-wavelength and multicolor emission, J. Mater. Chem. B, 2017, 5(21), 3794–3809 RSC
.
- T. Shen, Q. Wang, Z. Guo, J. Kuang and W. Cao, Hydrothermal synthesis of carbon quantum dots using different precursors and their combination with TiO2 for enhanced photocatalytic activity, Ceram. Int., 2018, 44(10), 11828–11834 CrossRef CAS
.
- Y. Wang, X. Chang, N. Jing and Y. Zhang, Hydrothermal synthesis of carbon quantum dots as fluorescent probes for the sensitive and rapid detection of picric acid, Anal. Methods, 2018, 10(23), 2775–2784 RSC
.
- J. Y. Li, Y. Liu, Q. W. Shu, J. M. Liang, F. Zhang and X. P. Chen,
et al., One-pot hydrothermal synthesis of carbon dots with efficient up- and down-converted photoluminescence for the sensitive detection of morin in a dual-readout assay, Langmuir, 2017, 33(4), 1043–1050 CrossRef CAS
.
- A. E. Tomskaya, M. N. Egorova, A. N. Kapitonov, D. V. Nikolaev, V. I. Popov and A. L. Fedorov,
et al., Synthesis of Luminescent N-Doped Carbon Dots by Hydrothermal Treatment, Phys. Status Solidi B, 2018, 255(1), 1–5 CrossRef
.
- O. B. A. Shatery and K. M. Omer, Selectivity Enhancement for Uric Acid Detection via In Situ Preparation of Blue Emissive Carbon Dots Entrapped in Chromium Metal-Organic Frameworks, ACS Omega, 2022, 7(19), 16576–16583 CrossRef CAS
.
- B. Wang, J. Yu, L. Sui, S. Zhu, Z. Tang and B. Yang,
et al., Rational Design of Multi-Color-Emissive Carbon Dots in a Single Reaction System by Hydrothermal, Adv. Sci., 2021, 8(1), 2001453 CrossRef CAS
.
- L. Lin, Y. Wang, Y. Xiao and W. Liu, Hydrothermal synthesis of carbon dots codoped with nitrogen and phosphorus as a turn-on fluorescent probe for cadmium(II), Microchim. Acta, 2019, 186(3), 5–11 Search PubMed
.
- Y. Liu, W. Li, P. Wu, C. Ma, X. Wu and M. Xu,
et al., Hydrothermal synthesis of nitrogen and boron co-doped carbon quantum dots for application in acetone and dopamine sensors and multicolor cellular imaging, Sens. Actuators, B, 2019, 281, 34–43 CrossRef CAS
.
- S. Arulkumar, S. Parthiban, A. Goswami, R. S. Varma and M. Naushad, Highly-sensitive electrocatalytic determination for toxic phenols based on coupled cMWCNT/cyclodextrin edge-functionalized graphene composite, Mater. Today: Proc., 2016, 318, 99–108 Search PubMed
.
- H. K. M. Ng, G. K. Lim and C. P. Leo, Comparison between hydrothermal and microwave-assisted synthesis of carbon dots from biowaste and chemical for heavy metal detection: A review, Microchem. J., 2021, 165(August 2020), 106116 CrossRef CAS
.
- J. Zheng, Y. Wang, F. Zhang, Y. Yang, X. Liu and K. Guo,
et al., Microwave-assisted hydrothermal synthesis of solid-state carbon dots with intensive emission for white light-emitting devices, J. Mater. Chem. C, 2017, 5(32), 8105–8111 RSC
.
- P. Zhao, X. Li, G. Baryshnikov, B. Wu, H. Ågren and J. Zhang,
et al., One-step solvothermal synthesis of high-emissive amphiphilic carbon dots: Via rigidity derivation, Chem. Sci., 2018, 9(5), 1323–1329 RSC
.
- D. Zhao, X. Liu, Z. Zhang, R. Zhang, L. Liao and X. Xiao,
et al., Synthesis of multicolor carbon dots based on solvent control and its application in the detection of crystal violet, Nanomaterials, 2019, 9(11), 1556 CrossRef CAS PubMed
.
- B. Ju, H. Nie, X. G. Zhang, Q. Chen, X. Guo and Z. Xing,
et al., Inorganic Salt Incorporated Solvothermal Synthesis of Multicolor Carbon Dots, Emission Mechanism, and Antibacterial Study, ACS Appl. Nano Mater., 2018, 1(11), 6131–6138 CrossRef CAS
.
- T. Geng, C. Liu, G. Xiao, S. Lu and B. Zou, Advances in the application of high pressure in carbon dots, Mater. Chem. Front., 2019, 3(12), 2617–2626 RSC
.
- Q. Liu, N. Zhang, H. Shi, W. Ji, X. Guo and W. Yuan,
et al., One-step microwave synthesis of carbon dots for highly sensitive and selective detection of copper ions in aqueous solution, New J. Chem., 2018, 42(4), 3097–3101 RSC
.
- C. Zhao, X. Li, C. Cheng and Y. Yang, Green and microwave-assisted synthesis of carbon dots and application for visual detection of cobalt(II) ions and pH sensing, Microchem. J., 2019, 147(January), 183–190 CrossRef CAS
.
- M. Jorns, S. Strickland, M. Mullins and D. Pappas, Improved citric acid-derived carbon dots synthesis through microwave-based heating in a hydrothermal pressure vessel, RSC Adv., 2022, 12(50), 32401–32414 RSC
.
- R. Tabaraki and N. Sadeghinejad, Microwave assisted synthesis of doped carbon dots and their application as green and simple turn off–on fluorescent sensor for mercury (II) and iodide in environmental samples, Ecotoxicol. Environ. Saf., 2018, 153(January), 101–106 CrossRef CAS PubMed
.
- Y. Zhang, X. Liu, Y. Fan, X. Guo, L. Zhou and Y. Lv,
et al., One-step microwave synthesis of N-doped hydroxyl-functionalized carbon dots with ultra-high fluorescence quantum yields, Nanoscale, 2016, 8(33), 15281–15287 RSC
.
- R. K. Singh, R. Kumar, D. P. Singh, R. Savu and S. A. Moshkalev, Progress in microwave-assisted
synthesis of quantum dots (graphene/carbon/semiconducting) for bioapplications: a review, Mater. Today Chem., 2019, 12, 282–314 CrossRef CAS
.
- B. Wang, H. Song, X. Qu, J. Chang, B. Yang and S. Lu, Carbon dots as a new class of nanomedicines: Opportunities and challenges, Coord. Chem. Rev., 2021, 442, 214010 CrossRef CAS
.
- F. Huo, P. G. Karmaker, Y. Liu, B. Zhao and X. Yang, Preparation and Biomedical Applications of Multicolor Carbon Dots: Recent Advances and Future Challenges, Part. Part. Syst. Charact., 2020, 37(4), 1–20 CrossRef
.
- J. Xu, K. Cui, T. Gong, J. Zhang, Z. Zhai and L. Hou, Ultrasonic-Assisted Synthesis of N-Doped, Multicolor Carbon Dots toward Fluorescent Inks, Fluorescence Sensors, and Logic Gate Operations, Nanomaterials, 2022, 12, 312 CrossRef CAS
.
- Z. Ma, H. Ming, H. Huang, Y. Liu and Z. Kang, One-step ultrasonic synthesis of fluorescent N-doped carbon dots from glucose and their visible-light sensitive photocatalytic ability, New J. Chem., 2012, 36(4), 861–864 RSC
.
- X. Lin, M. Xiong, J. Zhang, C. He, X. Ma and H. Zhang,
et al., Carbon dots based on natural resources: Synthesis and applications in sensors, Microchem. J., 2021, 160, 105604, DOI:10.1016/j.microc.2020.105604
.
- C. S. Stan, C. Albu, A. Coroaba, M. Popa and D. Sutiman, One step synthesis of fluorescent carbon dots through pyrolysis of N-hydroxysuccinimide, J. Mater. Chem. C, 2015, 3(4), 789–795 RSC
.
- X. Sun, X. Jin, W. Pan, E. Guo, W. Liu and D. Li,
et al., Highly luminescent carbon dots synthesized by microwave-assisted pyrolysis and evaluation of their toxicity to Physa acuta, J. Nanosci. Nanotechnol., 2016, 16(1), 648–653 CrossRef CAS PubMed
.
- Y. Wang, Y. Zhu, S. Yu and C. Jiang, Fluorescent carbon dots: Rational synthesis, tunable optical properties and analytical applications, RSC Adv., 2017, 7(65), 40973–40989 RSC
.
- P. K. Sarswat and M. L. Free, Light emitting diodes based on carbon dots derived from food, beverage, and combustion wastes, Phys. Chem. Chem. Phys., 2015, 17(41), 27642–27652 RSC
.
- S. Sahana, A. Gautam, R. Singh and S. Chandel, A recent update on development, synthesis methods, properties and application of natural products derived carbon dots, Nat. Prod. Bioprospect., 2023, 13(1), 51 CrossRef PubMed
.
- A. Manz, J. C. Fettinger, E. Verpoorte, H. Lüdi, H. M. Widmer and D. J. Harrison, Micromachining of monocrystalline silicon and glass for chemical analysis systems A look into next century’s technology or just a fashionable craze?, TrAC, Trends Anal. Chem., 1991, 10, 144–149 CrossRef CAS
.
- G.-X. Li, Q. Li, R. Cheng and S. Chen, Synthesis of quantum dots based on microfluidic technology, Curr. Opin. Chem. Eng., 2020, 29, 34–41 CrossRef
.
- Y. Cheng, S. Da Ling, Y. Geng, Y. Wang and J. Xu, Microfluidic synthesis of quantum dots and their applications in bio-sensing and bio-imaging, Nanoscale Adv., 2021, 3(8), 2180–2195 RSC
.
- G. Li, C. Liu, X. Zhang, P. Zhai, X. Lai and W. Jiang, Low temperature synthesis of carbon dots in microfluidic chip and their application for sensing cefquinome residues in milk, Biosens. Bioelectron., 2023, 228, 115187 CrossRef CAS PubMed
.
- L. Chen, C. Wang, C. Liu and S. Chen, Facile access to fabricate carbon dots and perspective of large-scale applications, Small, 2023, 19(31), 2206671 CrossRef CAS PubMed
.
- Y. Chen, A. Shen, J. Guo, L. Zhu, G. Li and Y. Qin,
et al., Continuous productions of highly fluorescent carbon dots and enriched polymer nanofibers via microfluidic techniques, Chem. Eng. J., 2023, 471, 144444 CrossRef CAS
.
- I. Lignos, R. Maceiczyk and A. J. deMello, Microfluidic technology: uncovering the mechanisms of nanocrystal nucleation and growth, Acc. Chem. Res., 2017, 50(5), 1248–1257 CrossRef CAS PubMed
.
- X. Li, L. Yu, M. He, C. Chen, Z. Yu and S. Jiang,
et al., Review on carbon dots: Synthesis and application in biology field, BMEMat, 2023, 1(4), e12045 CrossRef
.
- L. Cui, X. Ren, M. Sun, H. Liu and L. Xia, Carbon dots: Synthesis, properties and applications, Nanomaterials, 2021, 11(12), 1–38 CrossRef PubMed
.
- N. Arora and N. N. Sharma, Arc discharge synthesis of carbon nanotubes: Comprehensive review, Diamond Relat. Mater., 2014, 50, 135–150 CrossRef CAS
.
- A. Kaczmarek, J. Hoffman, J. Morgiel, T. Mościcki, L. Stobiński and Z. Szymański,
et al., Luminescent carbon dots synthesized by the laser ablation of graphite in polyethylenimine and ethylenediamine, Materials, 2021, 14(4), 1–13 CrossRef PubMed
.
- Isnaeni, M. Y. Hanna, A. A. Pambudi and F. H. Murdaka, Influence of ablation wavelength and time on optical properties of laser ablated carbon dots, AIP Conf. Proc., 2017, 1801, 1 CrossRef
.
- A. R. Sadrolhosseini, G. Krishnan, S. Shafie, S. A. Rashid and S. W. Harun, Optical and Photoacoustic Properties of Laser-Ablated Silver Nanoparticles in a Carbon Dots Solution, Molecules, 2020, 25(24), 5798 CrossRef CAS PubMed
.
- S. Campuzano, P. Yáñez-Sedeño and J. M. Pingarrón, Carbon dots and graphene quantum dots in electrochemical biosensing, Nanomaterials, 2019, 9(4), 1–18 CrossRef PubMed
.
- D. Rocco, V. G. Moldoveanu, M. Feroci, M. Bortolami and F. Vetica, Electrochemical Synthesis of Carbon Quantum Dots, ChemElectroChem, 2023, 10(3), 202201104 CrossRef PubMed
.
- T. Förster, Zwischenmolekulare energiewanderung und fluoreszenz, Ann. Phys., 1948, 437(1–2), 55–75 CrossRef
.
- X. Sun and Y. Lei, Fluorescent carbon dots and their sensing applications, TrAC, Trends Anal. Chem., 2017, 89, 163–180, DOI:10.1016/j.trac.2017.02.001
.
- J. Hu, Y. Sun, A. A. Aryee, L. Qu, K. Zhang and Z. Li, Mechanisms for carbon dots-based chemosensing, biosensing, and bioimaging: A review, Anal. Chim. Acta, 2022, 1209, 338885 CrossRef CAS PubMed
.
- F. Zu, F. Yan, Z. Bai, J. Xu, Y. Wang and Y. Huang,
et al., The quenching of the fluorescence of carbon dots: a review on mechanisms and applications, Microchim. Acta, 2017, 184, 1899–1914 CrossRef CAS
.
- S. Munusamy, T. R. Mandlimath, P. Swetha, A. G. Al-Sehemi, M. Pannipara and S. Koppala,
et al., Nitrogen-doped carbon dots: Recent developments in its fluorescent sensor applications, Environ. Res., 2023, 231, 116046 CrossRef CAS PubMed
.
- M. Batool, H. M. Junaid, S. Tabassum, F. Kanwal, K. Abid and Z. Fatima,
et al., Metal Ion Detection by Carbon Dots—A Review, Crit. Rev. Anal. Chem., 2022, 52(4), 756–767, DOI:10.1080/10408347.2020.1824117
.
- J. Chen, J. Yan, B. Dou, Q. Feng, X. Miao and P. Wang, Aggregatable thiol-functionalized carbon dots-based fluorescence strategy for highly sensitive detection of glucose based on target-initiated catalytic oxidation, Sens. Actuators, B, 2021, 330, 129325 CrossRef CAS
.
- H. Wu, W. Zheng, Y. Jiang, J. Xu and F. Qiu, Construction of a selective non-enzymatic electrochemical sensor based on hollow nickel nanospheres/carbon dots–chitosan and molecularly imprinted polymer film for the detection of glucose, New J. Chem., 2021, 45(46), 21676–21683 RSC
.
- H. Gan, W. Han, Z. Fu and L. Wang, The chain-like Au/carbon dots nanocomposites with peroxidase-like activity and their application for glucose detection, Colloids Surf., B, 2021, 199, 111553 CrossRef CAS PubMed
.
- F. P. Mutuyimana, J. Liu, M. Na, S. Nsanzamahoro, Z. Rao and H. Chen,
et al., Synthesis of orange-red emissive carbon dots for fluorometric enzymatic determination of glucose, Microchim. Acta, 2018, 185, 1–10 CrossRef CAS PubMed
.
- Y. Duan, Y. Huang, S. Chen, W. Zuo and B. Shi, Cu-doped carbon dots as catalysts for the chemiluminescence detection of glucose, ACS Omega, 2019, 4(6), 9911–9917 CrossRef CAS PubMed
.
- L. Haiyang, L. Guantong, Z. Nan, Y. Zhanye, J. Xinge and Z. Bing,
et al., Ag-carbon dots with peroxidase-like activity for colorimetric and SERS dual mode detection of glucose and glutathione, Talanta, 2024, 273, 125898 CrossRef PubMed
.
- Y. Hu, J. Wen, D. Li, Y. Li, M. Alheshibri and M. Zhang,
et al., Carbon dots-based fluorescence enhanced probe for the determination of glucose, Spectrochim. Acta, Part A, 2023, 303, 123149 CrossRef CAS PubMed
.
- Z. Lu, Y. Zhang, M. Sun, P. Zou, X. Wang and Y. Wang,
et al., N-doped carbon dots regulate porous hollow nickel-cobalt sulfide: high-performance electrode materials in supercapacitor and enzymeless glucose sensor, J. Power Sources, 2021, 516, 230685 CrossRef CAS
.
- J. Zhou, R. Wang, W. Su, L. Zhang, A. Li and T. Jiao, Efficient detection of glucose by graphene-based non-enzymatic sensing material based on carbon dot, Colloids Surf., A, 2022, 647, 129122 CrossRef CAS
.
- S. Zhuo, J. Fang, C. Zhu and J. Du, Preparation of palladium/carbon dot composites as efficient peroxidase mimics for H 2 O 2 and glucose assay, Anal. Bioanal. Chem., 2020, 412, 963–972 CrossRef CAS PubMed
.
- F. Honarasa, F. H. Kamshoori, S. Fathi and Z. Motamedifar, Carbon dots on V 2 O 5 nanowires are a viable peroxidase mimic for colorimetric determination of hydrogen peroxide and glucose, Microchim. Acta, 2019, 186, 1–7 CrossRef CAS
.
- D. Chao, J. Chen, Q. Dong, W. Wu, D. Qi and S. Dong, Ultrastable and ultrasensitive pH-switchable carbon dots with high quantum yield for water quality identification, glucose detection, and two starch-based solid-state fluorescence materials, Nano Res., 2020, 13, 3012–3018 CrossRef CAS
.
- J. Li, X. Li, R. Weng, T. Qiang and X. Wang, Glucose assay based on a fluorescent multi-hydroxyl carbon dots reversible assembly with phenylboronic acid brush grafted magnetic nanoparticles, Sens. Actuators, B, 2020, 304, 127349 CrossRef CAS
.
- P.-H. Hsiao and C.-Y. Chen, Insights for realizing ultrasensitive colorimetric detection of glucose based on carbon/silver core/shell nanodots, ACS Appl. Bio Mater., 2019, 2(6), 2528–2538 CrossRef CAS PubMed
.
- M. Hu, J. Qi, J. Ruan and G. Shen, Highly sensitive detection of glucose by a, J. Biomed. Nanotechnol., 2018, 14(6), 1117–1124 CrossRef CAS PubMed
.
- A. S. Krishna, P. A. Nair, C. Radhakumary and K. Sreenivasan, Carbon dot based non enzymatic approach for the detection and estimation of glucose in blood serum, Mater. Res. Express, 2016, 3(5), 55001 CrossRef
.
- Y. Li, X. Li, H. Tan and Z.-Z. Huang, A turn-on fluorescent assay for glucose detection based on carbon dots/manganese dioxide assembly, Microchem. J., 2020, 158, 105266 CrossRef CAS
.
- Y. Long, X. Wang, D. Shen and H. Zheng, Detection of glucose based on the peroxidase-like activity of reduced state carbon dots, Talanta, 2016, 159, 122–126 CrossRef CAS
.
- J.-L. Ma, B.-C. Yin, X. Wu and B.-C. Ye, Simple and cost-effective glucose detection based on carbon nanodots supported on silver nanoparticles, Anal. Chem., 2017, 89(2), 1323–1328 CrossRef CAS PubMed
.
- S. Muhammad, G. Xu, F. Wei, Y. Cen, M. R. Younis and X. Xu,
et al., Simultaneous determination of insulin and glucose in human serum based on dual emissive fluorescent nano-aptasensor of carbon dots and CdTe/CdS/ZnS quantum dots, Sens. Actuators, B, 2019, 292, 321–330 CrossRef CAS
.
- H. O. Othman, R. O. Hassan and A. T. Faizullah, A newly synthesized boronic acid-functionalized sulfur-doped carbon dot chemosensor as a molecular probe for glucose sensing, Microchem. J., 2021, 163, 105919 CrossRef CAS
.
- Y. Wei, L. Li, C. Ma, Y. Wu, C. Zhu and H. Gao,
et al., Phenolic Hydroxyl Group–Carbon Dots as a Fluorescent Probe for the Detection of Hydrogen Peroxide and Glucose in Milk, J. Appl. Spectrosc., 2022, 89(2), 272–280 CrossRef CAS
.
- W. Zhao, M. Zhang, L. Zhang, X. Deng, Y. Wang and Y. Chen,
et al., Carbon Dots with Antioxidant Capacity for Detecting Glucose by Fluorescence and Repairing High-Glucose Damaged Glial Cells, J. Fluoresc., 2024, 1–12 Search PubMed
.
- H. Ji, F. Zhou, J. Gu, C. Shu, K. Xi and X. Jia, Nitrogen-doped carbon dots as a new substrate for sensitive glucose determination, Sensors, 2016, 16(5), 630 CrossRef
.
- P. Shen and Y. Xia, Synthesis-modification integration: one-step fabrication of boronic acid functionalized carbon dots for fluorescent blood sugar sensing, Anal. Chem., 2014, 86(11), 5323–5329 CrossRef CAS PubMed
.
- H. Wang, J. Yi, D. Velado, Y. Yu and S. Zhou, Immobilization of carbon dots in molecularly imprinted microgels for optical sensing of glucose at physiological pH, ACS Appl. Mater. Interfaces, 2015, 7(29), 15735–15745 CrossRef CAS PubMed
.
- Y. Wang, C. Han, L. Yu, J. Wu, Y. Min and J. Tan,
et al., Etching-controlled suppression of fluorescence resonance energy transfer between nitrogen-doped carbon dots and Ag nanoprisms for glucose assay and diabetes diagnosis, Spectrochim. Acta, Part A, 2020, 242, 118713 CrossRef CAS PubMed
.
- X. Zhou, X. Gao, M. Liu, C. Wang and F. Chu, A poly (5-indolylboronic acid) based molecular imprint doped with carbon dots for fluorometric determination of glucose, Microchim. Acta, 2017, 184, 4175–4181 CrossRef CAS
.
- W. Zheng, H. Wu, Y. Jiang, J. Xu, X. Li and W. Zhang,
et al., A molecularly-imprinted-electrochemical-sensor modified with nano-carbon-dots with high sensitivity and selectivity for rapid determination of glucose, Anal. Biochem., 2018, 555, 42–49 CrossRef CAS
.
- X. Liu, H. Zhang, Z. Song, L. Guo, F. Fu and Y. Wu, A ratiometric nanoprobe for biosensing based on green fluorescent graphitic carbon nitride nanosheets as an internal reference and quenching platform, Biosens. Bioelectron., 2019, 129(October 2018), 118–123, DOI:10.1016/j.bios.2019.01.032
.
- A. Bigdeli, F. Ghasemi, S. Abbasi-Moayed, M. Shahrajabian, N. Fahimi-Kashani and S. Jafarinejad,
et al., Ratiometric fluorescent nanoprobes for visual detection: Design principles and recent advances - A review, Anal. Chim. Acta, 2019, 1079, 30–58 CrossRef CAS PubMed
.
- M.-J. Cho and S.-Y. Park, Carbon-dot-based ratiometric fluorescence glucose biosensor, Sens. Actuators, B, 2019, 282, 719–729 CrossRef CAS
.
- D. Zhu, S. Zhuo, C. Zhu, P. Zhang and W. Shen, Synthesis of catalytically active peroxidase-like Fe-doped carbon dots and application in ratiometric fluorescence detection of hydrogen peroxide and glucose, Anal. Methods, 2019, 11(20), 2663–2668 RSC
.
- W.-S. Zou, C.-H. Ye, Y.-Q. Wang, W.-H. Li and X.-H. Huang, A hybrid ratiometric probe for glucose detection based on synchronous responses to fluorescence quenching and resonance light scattering enhancement of boronic acid functionalized carbon dots, Sens. Actuators, B, 2018, 271, 54–63 CrossRef CAS
.
- H. Zhai, Y. Bai, J. Qin and F. Feng, Colorimetric
and ratiometric fluorescence dual-mode sensing of glucose based on carbon quantum dots and potential UV/fluorescence of o-diaminobenzene, Sensors, 2019, 19(3), 674 CrossRef PubMed
.
- H. Zhao, X. Yuan, X. Yang, F. Bai, C. Mao and L. Zhao, Nitrogen-doped carbon dot and CdTe quantum dot dual-color multifunctional fluorescent sensing platform: sensing behavior and glucose and pH detection, Inorg. Chem., 2021, 60(20), 15485–15496 CrossRef CAS PubMed
.
- H. M. Junaid, M. Batool, F. W. Harun, M. S. Akhter and N. Shabbir, Naked eye chemosensing of anions by Schiff bases, Crit. Rev. Anal. Chem., 2022, 52(3), 463–480 CrossRef CAS PubMed
.
- Z. Liao, J. Zhong, X. Tang, Z. Peng, P. Xu and P. Qiu, Smartphone-assisted portable swabs for blood glucose management: A point-of-use assay for dual-mode visual detection based on bifunctional carbon dots, Talanta, 2024, 278, 126545 CrossRef CAS PubMed
.
- R. Bandi, M. Alle, C.-W. Park, S.-Y. Han, G.-J. Kwon and N.-H. Kim,
et al., Cellulose nanofibrils/carbon dots composite nanopapers for the smartphone-based colorimetric detection of hydrogen peroxide and glucose, Sens. Actuators, B, 2021, 330, 129330 CrossRef CAS
.
- K. Su, G. Xiang, C. Cui, X. Jiang, Y. Sun and W. Zhao,
et al., Smartphone-based colorimetric determination of glucose in food samples based on the intrinsic peroxidase-like activity of nitrogen-doped carbon dots obtained from locusts, Arabian J. Chem., 2023, 16(3), 104538 CrossRef CAS
.
- A. Mandal, S. Sappati, A. Karmakar, S. Pradhan, I. Gabriel and S. Varanasi, Smartphone-Assisted and Optical Quantification of Copper and Glucose Using Palm Wine-Tailored Carbon Dots and Their Multiple Logic Gate Application, ACS Biomater. Sci. Eng., 2024, 5362–5380 CrossRef CAS PubMed
.
- E. L. Rossini, M. I. Milani and H. R. Pezza, Green synthesis of fluorescent carbon dots for determination of glucose in biofluids using a paper platform, Talanta, 2019, 201, 503–510 CrossRef CAS PubMed
.
- E. L. Rossini, M. I. Milani, L. S. Lima and H. R. Pezza, Paper microfluidic device using carbon dots to detect glucose and lactate in saliva samples, Spectrochim. Acta, Part A, 2021, 248, 119285 CrossRef CAS PubMed
.
- N. Alizadeh, A. Salimi and R. Hallaj, A strategy for visual optical determination of glucose based on a smartphone device using fluorescent boron-doped carbon nanoparticles as a light-up probe, Microchim. Acta, 2020, 187, 1–10 CrossRef PubMed
.
- R. Zhang, L. Liu, W. Li, X. Luo and F. Wu, Luminescent carbon dots with excellent peroxidase mimicking property for fluorometric and colorimetric detection of glucose, Colloids Surf., B, 2023, 222, 113125 CrossRef CAS PubMed
.
- Q. Yu, J. Jiang, Z. Chen, C. Han, X. Zhang and S. Yang,
et al., A multilevel fluorometric biosensor based on boric acid embedded in carbon dots to detect intracellular and serum glucose, Sens. Actuators, B, 2022, 350, 130898 CrossRef CAS
.
|
This journal is © The Royal Society of Chemistry 2025 |
Click here to see how this site uses Cookies. View our privacy policy here.