DOI:
10.1039/D4NH00542B
(Review Article)
Nanoscale Horiz., 2025,
10, 681-698
Semiconductor photocatalytic antibacterial materials and their application for bone infection treatment
Received
22nd October 2024
, Accepted 10th January 2025
First published on 14th January 2025
Abstract
Bacterial infection in bone tissue engineering is a severe clinical issue. Traditional antimicrobial methods usually cause problems such as bacterial resistance and biosecurity. Employing semiconductor photocatalytic antibacterial materials is a more controlled and safer strategy, wherein semiconductor photocatalytic materials generate reactive oxygen species under illumination for killing bacteria by destroying their cell membranes, proteins, DNA, etc. In this review, P-type and N-type semiconductor photocatalytic materials and their antibacterial mechanisms are introduced. Type II heterojunctions, P–N heterojunctions, type Z heterojunctions and Schottky junctions have been reported to reduce the recombination of carriers, while element doping, sensitization and up-conversion luminescence expand the photoresponse range. Furthermore, the applications of semiconductor photocatalytic antibacterial materials in bone infection treatment such as osteomyelitis treatment, bone defect repair and dental tissue regeneration are summarized. Finally, the conclusion and future prospects of semiconductor photocatalytic antibacterial materials in bone tissue engineering were analyzed.
1. Introduction
Bone infection caused by traumatic fracture, surgical infection, blood transmission, adjacent tissue infection and immune dysfunction cannot be ignored, and its incidence is as high as 30%.1 These infections often lead to serious complications such as osteomyelitis and osteonecrosis, which affect the quality of life of patients and even threaten their lives. Implant-associated infections, in particular, have become a growing problem with the popularity of orthopedic surgery and artificial joint replacement.2 Implant infection can not only lead to postoperative complications, but also to implant failure, bone damage and even reoperation, and it is thus difficult to treat and require a long course of treatment.3 Since penicillin was discovered in 1928, various antibiotics have been used to cope with bacterial infection, such as vancomycin, tetracycline, and ciprofloxacin.4 For example, Ren et al.5 found that bisphosphonate-bound sitafloxacin combined with vancomycin was effective in clearing methicillin-resistant Staphylococcus aureus (MRSA) infection, reducing bone resorption and biofilm formation from the surrounding implant. However, the abuse of antibiotics generates bacterial resistance, which can lead to the production of super bacteria, and long-term or high doses of antibiotics may cause damage to liver and kidney functions. Therefore, there is an urgent need to find a strategy to replace antibiotics and fight bacterial infection.
To deal with the problem of bacterial infection without causing bacterial resistance, researchers have sought to use other strategies to combat bacteria, such as metal ion release, photothermal therapy (PTT), and antibacterial gas therapy. Shicheng et al.6 prepared Cu-doped bioactive glass using electrophoretic deposition to deal with implant-associated infection, and the released Cu ions could improve the antibacterial effect of the implant through contact killing, immune regulation, and other functions. Although released metal ions have excellent antibacterial effects, they also exhibit a certain cytotoxicity, which limits their application in bone tissue engineering. By contrast, PTT has no cytotoxicity and can be controlled by near-infrared (NIR) light; for example, Zhang et al.7 modified a nucleic acid aptamer on the surface of a polycaprolactone (PCL) nanofiber scaffold to generate mild hyperthermia through NIR light irradiation, thus effectively preventing the proliferation of bacteria in the early stage of bone defect repair. To completely kill bacteria, the temperature of photothermal therapy is usually maintained at >50 °C, but excessive temperature could also cause damage to surrounding healthy tissue. Antibacterial gases are able to diffuse freely into biofilms and exert an antibacterial effect within bacterial cell due to their low molecular weight, but the controllability of the gas release rate and cytotoxicity need further consideration.
As a burgeoning antibacterial strategy, photocatalytic antibacterial effect has attracted increasing attention. Specifically, photocatalytic antibacterial materials can produce electrons and holes because the electron can jump from the valence band (VB) to the conduction band (CB) under illumination. Then, the produced electrons and holes can react with substances such as water and oxygen to produce reactive oxygen species (ROS) with strong redox properties, and the ROS can kill bacteria by destroying the cell membranes, proteins, DNA, and so on. The generation of ROS in photocatalytic antibacterial processes can be precisely controlled by the external illumination, which is a key advantage of this method.8 Once the bacteria are eliminated, the external light source can be turned off, effectively halting the production of ROS and preventing any potential damage to normal, healthy cells.9 This ability to control the activation of ROS based on illumination makes photocatalytic antibacterial treatment a safer, more targeted, and more controlled strategy compared to traditional antimicrobial methods, which may suffer from issues such as toxicity to surrounding tissues and bacterial resistance.10 This control over ROS generation offers a promising approach for localized, selective bacterial eradication without causing harm to the host cells. Semiconductor photocatalytic materials are widely used to combat bacterial infection in bone tissue engineering but only a few articles have summarized their application in bone tissue engineering.
In this review, the research progress on semiconductor photocatalytic antibacterial materials and their application in bone tissue engineering have been presented. It focuses on advancements made during the period 2019–2024, with key topics including semiconductor, photocatalysis, antibacterial, heterojunctions, doping, up-conversion, bone repair, bone scaffolds, tooth implants, and osteomyelitis. The application of semiconductor materials in bone repair has gradually become a hot research direction, and breakthroughs in related technologies have provided new solutions for bone regeneration and repair. This paper will systematically review the advances in these research fields, analyze their challenges and development trends, and provide references for the future application of related technologies. Two types of semiconductor photocatalytic materials are introduced, namely, P-type semiconductor and N-type semiconductor, and the advantages and limitations of each material have been discussed. To decrease the recombination of electrons and holes, some methods that could separate electrons and holes were summarized, such as type II heterojunction, P–N heterojunction, type Z heterojunction and Schottky junction. To increase the utilization of light, some strategies that could expand the light response range have been summarized, such as element doping, sensitization and up-conversion luminescence. Furthermore, the applications of semiconductor photocatalytic antibacterial materials in bone tissue engineering have been discussed, such as osteomyelitis treatment, bone defect repair and dental tissue regeneration. Finally, the prospects and challenges of semiconductor photocatalytic antibacterial materials in bone tissue engineering have been summarized.
2. Semiconductor photocatalytic materials
Bacterial infection in bone tissue engineering has always been a difficult problem. However, the development of semiconductors has provided a new solution for it in recent years. The band structure of a semiconductor typically features a low-energy VB filled with electrons and an empty high-energy CB, and the distance between these two regions is called the band gap width. The band gap in semiconductors is usually discontinuous, and their photocatalytic behavior depends on the specific band structure. When light energy matches or exceeds the band gap, electrons in the VB are excited to the CB, moving to the surface and creating holes in the VB, resulting in a highly active pair of hole–electrons, which can also be called photogenerated carriers. The electrons in the CB react with oxygen molecules (O2) in solution to form the superoxide anion (˙O2−) through the equation e− + O2 → ˙O2−.11 The holes in the VB react with hydroxide ion (OH−) to form the hydroxyl radical (˙OH) (h+ + OH− → ˙OH).12 Besides, ˙O2− can further react with water molecule to form the hydrogen peroxide (H2O2) (˙O2− + H2O → H2O2 + OH−).13 These ROS have strong redox properties, and when they come into contact with bacteria, they can destroy the bacteria cell membrane, proteins and DNA, thus leading to bacterial death. Semiconductor materials can be divided into P-type and N-type semiconductors according to their carriers, and commonly used semiconductor photocatalytic antibacterial materials are shown in Table 1.
Table 1 Commonly used semiconductor photocatalytic antibacterial materials
Type |
Materials |
Band gap (eV) |
Absorption wavelength |
Advantage |
Drawback |
Ref. |
N |
TiO2 |
3.0–3.2 |
Ultraviolet (UV) light |
• Low toxicity |
• Broad band-gap |
14
|
• High biocompatibility, |
• Fast recombination of electron–hole pair |
• High antibacterial activity |
|
ZnO |
3.2–3.7 |
UV light |
• UV resistance |
• Broad band-gap |
15
|
• Good photocatalytic performance |
• Fast recombination of electron–hole pair |
• Antibacterial activity |
|
g-C3N4 |
2.7 |
UV light |
• Biocompatible |
• High electron–hole recombination rate |
16
|
• Non-toxic |
• Insufficient absorption of visible light |
• Low manufacturing cost |
• Low surface area |
Bi2S3 |
1.3–1.7 |
Visible light |
• Visible-light response |
• Broad band gap |
17 and 18
|
• Rapid recombination of holes and electrons |
WO3 |
2.7–2.8 |
UV light |
• Non-toxic and stable in water-based media |
• High recombination of photogenerated carriers |
19 and 20
|
WS2 |
1.9 |
Visible light |
• Large surface area can increase the active site |
• High recombination of photogenerated carriers |
21
|
ZnS |
3.7 |
UV light |
• Antioxidant |
• Broad band-gap |
22
|
• Hydrolytic chemical stability |
• Fast recombination of electron–hole pair |
CdS |
2.4 |
Visible light |
• Strong reducing ability |
• Severe photocorrosion occurs during the photocatalytic reaction |
23
|
SnS2 |
2.0–2.4 |
Visible light |
• High specific surface area and porosity |
• Charge mobility difference |
24
|
P |
Cu2O |
2.0–2.2 |
Visible light |
• Inhibit the recombination of electron–hole pairs |
• Optical instability |
25
|
CuO |
1.2 |
Visible light |
• Low cost |
• Low photocatalytic activity |
26
|
• Narrow band gap |
• Less oxidation activity |
CuS |
1.7–2.2 |
Visible light |
• Narrow band gap |
• Lack of chemical stability |
27
|
• Good light absorption performance in the visible–NIR region |
• Rapid recombination of photogenerated carriers |
|
• Particle aggregation |
MoS2 |
1.78 |
Visible light |
• Good visible light absorption ability |
• A limited number of active edge sites are available |
28
|
SnS |
1.3 |
NIR |
• Narrow band gap |
• Low conductivity and poor capacity retention |
29
|
• High absorption coefficient |
AgBr |
2.57 |
Visible light |
• High photocatalytic efficiency |
• Shielding effect by Ag layers on the surface of silver halide |
30 and 31
|
NiO |
3.3 |
UV light |
• Low-cost and earth-abundant |
• The active sites to the amorphization-induced under-coordinated Ni atoms would result in electron doping in NiO |
32
|
CoO |
2.44–2.6 |
Visible light |
• High activity |
• Lattice distortion (disorder) will produce localized electronic states blocking the carrier migration |
33–35
|
• Relatively narrow bandgap |
• Short lifespan |
2.1. N-Type semiconductor photocatalytic materials
N-Type semiconductors, also known as electronic semiconductors, are semiconductors in which the free electron concentration is significantly higher than the hole concentration.36 The letter “N” comes from the English word “Negative”, meaning negative electricity. In N-type semiconductors, the main carriers involved in the conductance are negatively charged electrons, which come from the doped donor impurity.37 Any semiconductor doped with donor impurities, with donor concentration higher than acceptor concentration, is an N-type semiconductor, as shown in Fig. 1(A). The widely used semiconductor photocatalytic antibacterial materials, such as titanium dioxide (TiO2), zinc oxide (ZnO), graphitic carbon nitride (g-C3N4), bismuth sulfide (Bi2S3), molybdenum oxide (MoO3), and layer double hydroxides (LDHs), are mainly introduced in this paper.
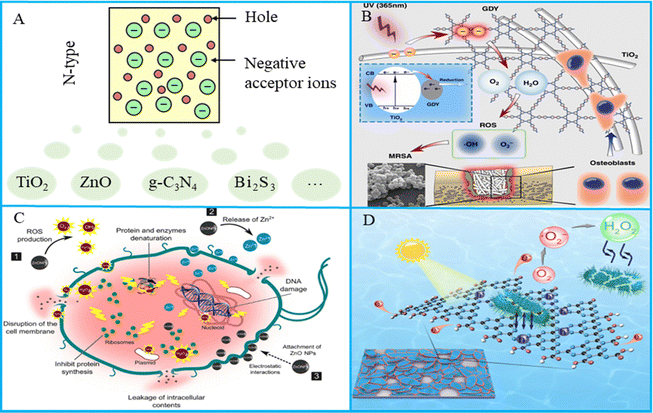 |
| Fig. 1 (A) N-Type semiconductors with electrons as the main photocarriers. (B) Schematic of graphdiyne-modified TiO2 nanofibers with osteoinductive and enhanced photocatalytic antibacterial activities to prevent implant infection. Reproduced with permission.38 Copyright 2020, Springer Nature. (C) Schematic illustration of the antimicrobial mechanism of ZnO NPs against bacterial cells. Reproduced with permission.39 Copyright 2019, Springer Nature. (D) Schematic diagram of indirect bacteria inactivation using H2O2 generated by an edge-functionalized g-C3N4 nanosheet. Reproduced with permission.40 Copyright 2019, Elsevier. | |
TiO2 antibacterial materials have been widely used in medical devices, dental implants and other medical aspects to control pathogenic bacterial infection, as shown in Fig. 1(B). TiO2 has three phases, namely, anatase, rutile, and brookite, and it can respond to UV light. At the same time, it has high antibacterial activity and has been widely used in the field of photocatalysis. Many researchers have studied TiO2 antibacterial materials and their antibacterial effects.41–43 Xiao et al.44 constructed FeS2-sensitized TiO2 materials via a hot-solvent method, and the disinfection efficiency was 95.7% and 92.4% for Escherichia coli (E. coli) and Staphylococcus aureus (S. aureus), respectively. Varnagiris et al.45 deposited TiO2 films on polystyrene magnetic beads by magnetron sputtering technology. Under ultraviolet irradiation, TiO2 films produced many ROS and the results indicated that 94% of E. coli was killed by the films. Additionally, the photocatalytic antibacterial properties of TiO2 were largely influenced by the cell wall structure. For optimal photocatalytic inactivation, direct contact between bacteria and the TiO2 surface is vital as it increases the likelihood of ROS attack. Consequently, photocatalytic antibacterial effectiveness depends on cell wall thickness, cell envelope morphology, and the outer membrane's resistance to ROS from the photocatalyst.46 Besides, the development of TiO2 faces key problems such as wide semiconductor energy band, low quantum efficiency, and inability to produce visible light response, which limits its application in bone tissue engineering. How to improve these shortcomings of semiconductors is the key to using TiO2 photocatalytic antibacterial materials.47
As a multifunctional biosafety material, ZnO has outstanding UV resistance, good photocatalytic properties and antibacterial activity, and has shown broad practical application prospects in research and development, as shown in Fig. 1(C). Some studies have pointed out that the antibacterial effect of ZnO comes from the production of ROS, the release of Zn2+ and cell membrane damage. Many studies clearly show that ZnO nanoparticles could produce ROS, including ˙O2−, H2O2 and hydroxyl radicals (˙OH), which could lead to bacterial death. Moreover, due to the oxidizing ability of Zn2+ released by ZnO, Zn2+ interacts with organic functional groups and attaches to bacterial cells and membrane proteins. It penetrates bacterial cells, disrupting their electron transfer system and hindering protein gene expression. Nonato et al.48 prepared poly-lactic acid (PLA) bone scaffold with ZnO nanofibers, and the results showed that ZnO nanofibers exhibited good antibacterial properties. The specific surface area of ZnO will affect its antibacterial effect, and small size ZnO particles and high specific surface area ZnO have better antibacterial effect.49 Besides, ZnO is a promising antimicrobial agent; however, it only reacts with UV light due to its wide bandgap. In addition, ZnO exhibits rapid electron–hole pairs recombination, which limits its photocatalytic antibacterial efficiency.
g-C3N4 is a commonly used photocatalytic material with good biosafety and excellent photocatalytic effect, and it has been used in many fields such as antibacterial, water decomposition and carbon dioxide decomposition. An example of antibacterial application of g-C3N4 is shown in Fig. 1(D). Liu et al.50 prepared nitrogen-deficient g-C3N4 ultra-thin nanosheets using thermal polymerization technology. The results indicated that the nanosheets could reduce O2 to ˙O2−, which could attack the cell membrane of E. coli. Guo et al.51 prepared acridinium-grafted g-C3N4 nanosheets, which changed the band gap of g-C3N4 from 2.77 eV to 2.12 eV. Molecular dynamics simulation reveals that the nanosheets could insert the bacteria, which facilitated ROS combination with the bacterial cell membrane, and the removed biofilm biomass was 91% after light irradiation. g-C3N4 has the excellent photocatalysis effect similar to TiO2, but the chemical potential of VB is lower than that of ˙OH generation, causing low generation rate of ˙OH, and the antibacterial effect of ˙O2− is lower than ˙OH, which limits its antibacterial application.
Bi2S3 is a layered semiconductor with a narrow band gap of 1.2–1.7 eV.52 It has good photocatalytic stability, low cost, harmless to human body, and is an excellent choice for bone tissue engineering antibacterial materials. Wang et al.50 prepared urchin-shaped Au/Bi2S3 nanomaterials by the hard template method to replace antibiotics and combat superbugs. Au/Bi2S3 core–shell structure improved the separation of electron–hole pairs and increased the efficiency of ROS production. As a result, the bactericidal rate of S. aureus and E. coli reached 100% under NIR irradiation. Wang et al.53 developed a green and reliable antimicrobial method by loading ZnS and Bi2S3 into thermally-sensitive polymer microspheres. The Bi2S3 could be released under the NIR irradiation because the microspheres transformed the absorbed NIR into heat and the thermally-sensitive polymer could be melted when the temperature was above the fusing point, and compared with the photothermal method alone, the killing rates of S. aureus and E. coli were increased remarkably. Although the Bi2S3 can be excited under NIR, the narrow band gap also corresponds to low redox potential, thus limiting the generation of ROS.
MoO3, as a typical 2D layer structure material, shows remarkable photocatalytic and antibacterial properties.54 Recently, there has been extensive research on the use of MoO3 nanomaterials for photodynamic or photocatalytic antibacterial applications. Gao et al.55 prepared an oxygen vacancy-mediated bactericidal MoO3, and MoO3 nanosheets were able to activate the adsorbed oxygen production ˙O2−, break the cell membrane of the bacteria attached to the surface, and induce the production of intracellular ROS, resulting in the death of the bacteria, and the antibacterial rate reached 99% in bacterial disinfection of authentic atmosphere. Yang et al.56 anchored MoO3 nanosheets to the surface of carbon nanosheets, which effectively collected NIR light and promoted the transfer of charge carriers, thus greatly enhancing the ability of ˙O2− generation. After 50 min of NIR light treatment, folds and partially merged membranes appeared on the surface of the bacterial cells, and eventually the violent collapse of the cell structure led to the death of the bacteria.
LDHs are an important class of layered materials, which are classified as a type of semiconductor material, known as naturally occurring hydrotalcite compounds, and they have been widely utilized in the fields of photocatalytic antibacterial materials and bone repair.57 Rad et al.58 synthesized FeCuMg and CrCuMg LDHs and compared their sonophotocatalytic activities. The bandgap energies of FeCuMg and CrCuMg LDHs were calculated to be 2.54 eV and 2.41 eV, respectively. At 600 μg mL−1, CrCuMg LDH and FeCuMg LDH reduced the activity of S. aureus by 91.3% and 80.0%, respectively. Mg alloy materials used in biomedicine lack corrosion resistance and antibacterial properties, while LDH surface coating engineering technology is expected to improve their antibacterial effects.59 Zhang et al.60 prepared a plasma electrolytic oxidation coating on Mg alloy and modified it with LDH. After NIR irradiation, the bacterial colony of the LDH group decreased significantly, indicating that the LDH film had good antibacterial activity. Besides, the sample also had good biocompatibility and osteogenic induction.
2.2. P-Type semiconductor photocatalytic materials
Compared with N-type semiconductors, which use electrons as the main carriers, P-type semiconductors, also known as hole-type semiconductors, refer to semiconductors with positively charged holes as the main conductive carrier or impurity semiconductors with hole concentrations much higher than free electron concentrations.61 In P-type semiconductors, holes are the majority charge carriers and free electrons are the minority charge carriers. The holes act as positive charge carriers and lead the conduction process.62 The letter “P” is derived from the first letter of the word “Positive”. In this type of semiconductor, the charge carriers involved in the conductivity are mainly positively charged holes, which come from the acceptor in the semiconductor,63 as shown in Fig. 2(A). In this article, the main introduced P-type semiconductors are CuO, CuS and Mo2S.
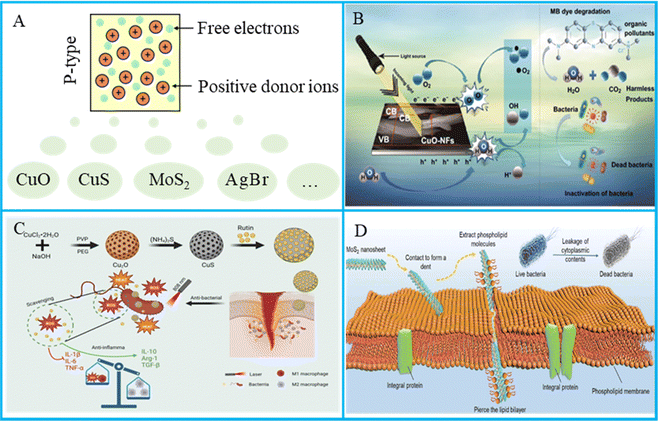 |
| Fig. 2 (A) P-type semiconductors with holes as the main photocarriers. (B) The sustainable performance of CuO in methylene blue degradation and bacterial organisms. Reproduced with permission.64 Copyright 2020, Springer Nature. (C) Synthesis of CuS/rutin and its antibacterial mechanisms. Reproduced with permission.65 Copyright 2024, Elsevier. (D) The physical antibacterial mechanisms between MoS2 nanosheets and bacterial cell membranes. Reproduced with permission.66 Copyright 2022, John Wiley and Sons. | |
CuO is a monoclinic crystalline semiconductor, and in addition to producing ROS, CuO can also release Cu2+ to contact bacteria and destroy bacterial cell membranes;67 an example diagram of the antibacterial use of CuO photocatalysis is shown in Fig. 2(B). Guan et al.68 found that ROS produced by CuO could destroy bacterial cell structure. Several studies have shown that the shape, morphology, and size of CuO nanostructures appear to influence their antimicrobial properties. Siddiqui et al.64 studied the biosynthesis of flower-shaped CuO nanostructures and photocatalytic antibacterial activities, and the obtained results confirmed that the prepared flower-shaped CuO nanostructures showed good antibacterial activity against E. coli (29 ± 2 mm) at a concentration of 100 μg mL−1. George et al.69 focused on the effect of calcination temperatures on the size, size distribution and morphology of the prepared CuO nanoparticles. The results showed that CuO nanoparticles calcined at 800 °C had a smaller size and better antibacterial effect.
CuS nanomaterials can excite a large number of charge carriers, which endows the materials with localized surface plasmon resonance properties in the NIR region, as shown in Fig. 2(C). Zou et al.70 used vancomycin (Van)-loaded CuS nanoparticles (CuSNPs) (CuS@Van) for killing the bacteria. In this study, Van was used not only as a reducing agent acting in tandem with CuSNPs but also as a booster to promote CuS to enter the bacterial interior. It was found that CuS@Van had good antibacterial activity and the antibacterial rate was up to 90%. CuS is also useful for bone tissue engineering. Xu et al.71 used 3D printing technology to prepare PCl bone scaffold, and CuS nanoparticles and polyethylene glycol were added to endow the scaffold with antibacterial properties and elasticity. Under NIR treatment, the scaffold showed good antibacterial and bone regeneration ability.
MoS2 has the greatest potential to fight pathogens because of its layered structure, and the mechanical antibacterial effect is shown in Fig. 2(D). Hu et al.72 modified two-dimensional MoS2 using galactose and focal ligands as starting materials, which had the ability to selectively locate Pseudomonas aeruginosa via polyvalent carbohydrate–lectin interactions. The results showed that ceftazidime antibiotics could be thermally released from two-dimensional MoS2 when NIR and white light irradiated antibiotic-supported materials, causing intracellular oxidative stress and reducing the level of intracellular glutathione (GSH), resulting in an antimicrobial rate of 90%. Some researchers reported an enhancement in its antibacterial effect by changing the size and form of MoS2, and MoS2 quantum dots had better photocatalytic effect than monolayer MoS2. The yield of singlet oxygen by MoS2 quantum dots was higher than that of MoS2 nanosheets and bulk MoS2. Most importantly, reducing the number of layers changed the band gap of MoS2, with values of about 1.1–1.7 eV for different number of layers. The results showed that MoS2 quantum dots had stronger hole and electron redox activity than MoS2 blocks and MoS2 nanosheets.
2.3. Size and morphology
The size and morphology of semiconductor photocatalytic materials have significant influence on the photocatalytic effect.73 The different size and morphology of the material usually affect its specific surface area, and a larger specific surface area can provide more active sites and enhance the efficiency of the photocatalytic reaction.74 The different size and morphology will affect the light absorption characteristics, electron transport rate and selectivity of the catalytic reaction.75 By precisely controlling the size and topography, the band structure of the semiconductor material can also be adjusted to further optimize its response to light. Therefore, optimizing the size and morphology of semiconductor photocatalytic materials is a key factor to improve their photocatalytic efficiency.
From the perspective of size, the influence of the size of semiconductor photocatalytic material on its photocatalytic performance is mainly reflected in the specific surface area and quantum effect.76 As the size decreases, the specific surface area of the material increases, thus providing more active sites for the reaction, which helps to improve the adsorption amount of the reactants and the efficiency of the photocatalytic reaction. Sun et al.77 studied the effect of particle size distribution for the photocatalytic effect of carbon dots, and the smaller particles had significantly enhanced antibacterial activity. Cheng et al.78 constructed MOF/CdS photocatalyst with different specific surface area, and the results indicated that compared with pure CdS and MOF, the MOF/CdS photocatalyst had a larger specific surface area and better photocatalytic effect. In addition, the quantum size effect is particularly significant in nanomaterials. When the material size reaches the nanometer scale, the energy level structure of electrons changes, which can improve the light absorption and electron transition ability so as to improve the efficiency of photocatalytic reaction.79 Kazemi et al.80 synthesized Se nanoparticles by biosynthesis, which exhibited a band gap of 5.12 eV, different from the bulk (5.2 eV) due to the quantum size effect, and showed enhanced photocatalytic antibacterial activity.
From the perspective of morphology, one-dimensional nanostructures (such as nanowires or nanorods) or two-dimensional nanostructures (such as nanosheets or less lamellar material) can provide more reaction sites and enhance the adsorption capacity of the material due to their larger surface exposure. Liu et al.81 developed an NIR-activated heterostructure catalyst (MXene/Co nanowires) consisting of two-dimensional MXene and one-dimensional Co nanowires for acting against bacteria in drug-free therapy. Heterostructure coatings on orthopedic implants could achieve high antimicrobial efficacy of more than 90% within 20 min. Besides, the nanostructures can also damage bacterial cell membrane by mechanical damage. Zhang et al.82 constructed TiO2 nanorod arrays in the surface of implant, which could kill the bacteria not only through ROS and heat under NIR but also through the effect of mechanical puncture. Metasurface engineering is a promising strategy for fighting bacterial infections in Ti-based implants. Yang et al.83 produced metasurface with strong NIR response antibacterial activity on Ti alloy implants through a newly invented alkali–acid bidirectional hydrothermal method based on topochemical transformation. The antimicrobial rates of the metasurface-treated Ti against E. coli and S. aureus were 96.88% and 97.56%, respectively. The powerful nanostructure-induced effect provided a good way to improve the antibacterial effect and bone regeneration effect of implants.
Semiconductor photocatalytic antibacterial materials have good application prospect in bone tissue engineering, but there are some key problems such as broad semiconductor energy bandwidth, causing failure to produce visible light response and fast electron–hole pair recombination, causing low quantum efficiency. Thus, studies on improving photocatalytic properties are particularly important. Methods to improve the photocatalytic properties mainly include promoting the separation of photogenerated carriers and expanding the light response range.
3. Separation of photogenerated carriers
As mentioned earlier, photocatalytic materials are able to generate electron–hole pairs under light and further generate ROS. However, these electrons and holes do not remain separated forever, and most of the transitioned electrons return to the VB and undergo a recombination process, releasing energy usually in the form of light or heat, resulting in photogenic carriers unable to participate in an effective photocatalytic reaction.84 Recombination can reduce the photocatalytic efficiency of semiconductor materials, especially in applications such as photocatalytic degradation and antibacterial, where electrons and holes must be effectively separated to generate ROS or drive charge transport.85 To solve the problem of fast recombination of photogenerated carriers, suitable heterojunctions are usually constructed between semiconductors. It is considered to be a useful strategy for promoting the separation of photogenerated electron–hole pairs and enhancing the photocatalytic activity. According to the different carrier separation mechanisms and coupling materials of various hybrid heterojunctions, semiconductor photocatalysts can be divided into different heterojunctions. In this section, we mainly introduce several commonly used types of heterojunctions and their applications in the field of photocatalytic antibacterial materials, including Schottky junction, type II heterojunction, P–N heterojunction and type Z heterojunction.
3.1. Schottky junction
Semiconductors are able to work with conductive substances such as GO and precious metal nanoparticles to transfer the generated electrons through good electrical conductivity, which is able to reduce the recombination of electrons and holes. At the same time, due to the difference in Fermi energy levels, Schottky barriers, also known as Schottky junction (Fig. 3(A)), are generated between them, which can prevent electron reflux, effectively realize the separation of electrons and holes, and improve the photocatalytic efficiency. Ma et al.86 constructed an oxygen vacancy-embedded LDH/Ti3C2Tx MXene 2D/2D sheet-like Schottky heterostructure, which had improved photocatalytic activity. Wang et al.87 encapsulated colloidal semiconductor quantum dots into an MOF matrix using a simple ligation-assisted self-assembly method, which showed excellent antimicrobial properties against E. coli (7.99 lg reduction in 60 min) and S. aureus (5.23 lg reduction in 120 min) under visible light irradiation. Although semiconductor cooperation with conductive substances is cost-effective and has high optical stability and optical catalytic efficiency, the preparation method is complicated and there are problems such as precious metal shedding and biosecurity, which need to be further studied.
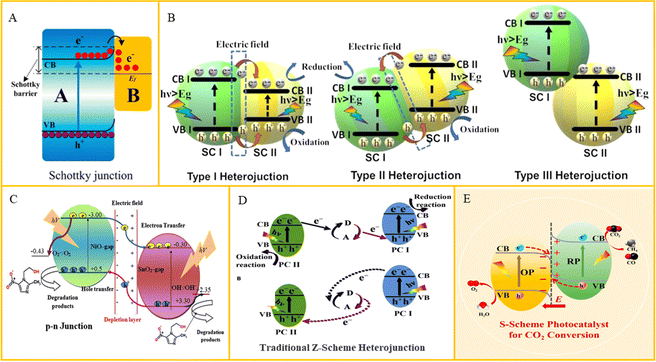 |
| Fig. 3 (A) Schematic of charge transfer in the Schottky junction. Reproduced with permission.88 Copyright 2020, MDPI. (B) Schematic of charge distribution in type I, type II and type III heterojunctions. Reproduced with permission.89 Copyright 2023, Elsevier. (C) Schematic of carrier production and charge separation in an NiO–SnO2 system. Reproduced with permission.90 Copyright 2017, Elsevier. (D) Charge transfer route in a traditional Z-scheme heterojunction. Reproduced with permission.91 Copyright 2020, Elsevier. (E) Schematic of the charge transfer route in an S-scheme heterojunction. Reproduced with permission.92 Copyright 2023, Elsevier. | |
3.2. Type II heterojunction
Heterojunctions are usually defined as an interface between two regions of different semiconductors with different band structures, and they can produce an interfacial band arrangement. According to the difference in the CB and VB energy levels of two semiconductors, the heterojunction formed can be divided into three types, namely, type I (transversal gap), type II (staggered gap) and Type III (broken gap), as shown in Fig. 3(B). For the type II heterojunction, both the VB and CB of semiconductor A are higher than that of semiconductor B. As a result, the photogenerated carriers can be separated because the excited electrons will be easily transferred. Yang et al.93 constructed a core–shell interface electric field at the interface between metal organic framework (MOF) and two-dimensional MoS2 nanosheets by in situ growth of MoS2 on the surface of MOF, which it exhibited an antimicrobial efficiency of 99.73% and 99.58% against S. aureus and E. coli within 20 min. The problem of the type II heterojunction photocatalyst is that the redox reaction is carried out on the semiconductor with low redox potential, which inhibits its redox ability. At the same time, the electrostatic repulsion of electrons and holes also prevents their migration between semiconductors.
3.3. P–N heterojunction
P–N heterojunction is constructed with a P type heterojunction and an N type heterojunction, where the generation of additional electric fields at the interface can improve the electrons transfer (Fig. 3(C)). Li et al.94 prepared Cu2O–TiO2 photocatalytic composites with the homogeneous distribution of P–N heterostructures by supercritical solvothermal method. Holes were the main active substances that reduced water to ˙OH, while the Cu2O–TiO2 interaction caused K+ leakage. The antibacterial effect of several common pathogens, such as Pseudomonas aeruginosa, E. coli and S. aureus, was almost 100%. Ma et al.95 prepared Cu1.96S/g-MnO2 P–N heterojunction, and the photoluminescence strength of Cu1.96S/g-MnO2 nanocomposites was low, which further indicated that P–N heterojunction significantly inhibited the recombination of charge pairs. However, P–N heterojunction is prone to photoinactivation under light irradiation, especially in an oxidizing environment, such as oxygen in the air, which will induce the oxidation of the heterojunction surface, resulting in reduced catalytic activity.
3.4. Type Z heterojunction
The above heterojunctions have the problem that the redox reaction is carried out on the semiconductor with low redox potential. Type Z photocatalysts can be divided into indirect type Z and direct type Z (type S) photocatalysts. The indirect Z heterojunction consists of two photocatalyst semiconductors and an intermediate A/D. Under photoexcitation, the intermediate A/D causes electrons to transfer indirectly from the CB of one semiconductor to the VB of another. Specifically, the photoelectrons reduce A to D, and subsequent holes oxidize D to A (Fig. 3(D)). The electrons are concentrated in semiconductor I and the holes are concentrated in semiconductor I, both of which have good redox ability. This system inhibits the recombination of electrons and holes, improves the redox capacity, and sacrifices part of the carrier to promote charge separation.96 For direct type Z photocatalysts, two different semiconductors without the intermediate are needed, as shown in Fig. 3(E). The photogenerated electrons migrate directly from the CB of one semiconductor to the VB of another, leaving electrons and holes at a high redox potential.97 Zhao et al.98 prepared an organic–inorganic S-scheme heterojunction by carboxyl-modified polythiophene-based linear conjugated polymer and TiO2, where under white light irradiation for 20 min, the heterojunction materials showed significant antibacterial properties, killing 3.86 × 107 cfu mL−1 MRSA. Jiang et al.99 developed a Z-scheme heterojunction composed of single Ag atoms-anchored polymeric carbon nitride (Ag–PCN) and SnO2−x, where the synergy between the single Ag atoms and the Z-scheme heterojunction initiated a cascade electron transfer from SnO2−x to Ag–PCN and then to O2 adsorbed on Ag. A type Z photocatalytic system can not only reduce electron–hole recombination but also maintain excellent redox ability. However, most synthetic artificial type Z photocatalytic systems usually have precious metals or redox pairs, which will bring great difficulties to their practical application.
4. Expansion of light response range
In addition to the problem of photogenerated electron and hole recombination, the semiconductor photocatalyst also have the problem of low light utilization. Most semiconductor photocatalysts have a wide band gap and can only absorb UV light and some visible light, but UV light only accounts for a small part of the sunlight. Therefore, it is very important to enlarge the light absorption range of semiconductor photocatalysts. This paper mainly introduces the methods to expand the light response range of semiconductor photocatalysts, including element doping, sensitization and up-conversion luminescence.
4.1. Element doping
Element doping can change the band structure of a semiconductor and generate visible light excitation and photoelectron transition. The doping of elements will affect the outermost orbital of the semiconductor, and metal ions or non-metal ions can enter the band gap as an intermediate stage. Also, the variant phase will narrow the band gap and cause the redshift of the absorption wavelength, further expanding the light wave response range and generating visible light response. On the other hand, the doping of elements causes the covalent bond between the atoms inside the semiconductor to break, resulting in certain defects in the crystal and formation of oxygen vacancies. A low concentration of oxygen vacancies can strengthen the transport of carriers and capture of photoelectrons, inhibit electron–hole recombination, and increase the visible light response. At present, element doping mainly includes metal and non-metal element doping. Metals have semiconductor-like properties, and their addition can strengthen the band gap structure of the semiconductor (Fig. 4(A)), increase the oxygen vacancy, which acts as an electron trap, and help to enhance the charge separation of photoactivity, thus improving the photocatalytic efficiency.100 Although ion doping can improve the ROS production of semiconductors, the biocompatibility of metal ions needs to be further studied. Doped non-metallic elements can improve the relative stability of semiconductors with improved optical quantum efficiency due to their stable valence bond structure and unique charge distribution (Fig. 4(B)). Moreover, non-metallic doping can cause a redshift of optical absorption edge and show the effect of visible light absorption. Common doped non-metallic elements are N, C, F, B, and S.101 However, the influence of specific gravity and doping amount of different nonmetallic elements on the visible light response range of semiconductors needs to be further confirmed.
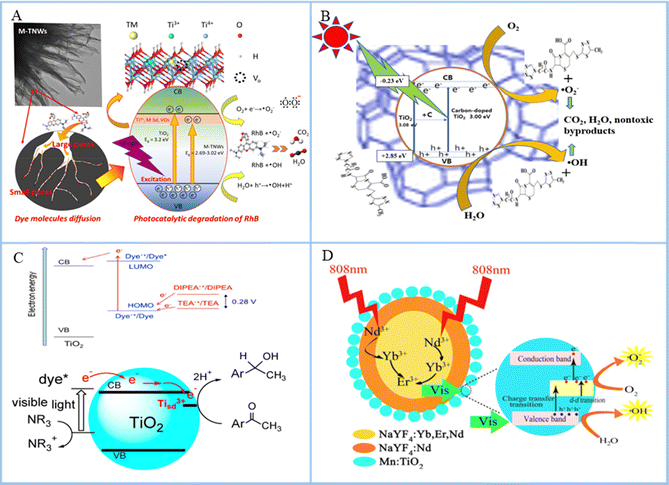 |
| Fig. 4 (A) Schematic of the proposed reaction mechanisms of the photocatalytic degradation of RhB by transition-metal doped titanate nanowire photocatalysts. Reproduced with permission.102 Copyright 2022, Elsevier. (B) Mechanisms of pollutant degradation using a zeolite carbon-doped TiO2 nanocomposite under sunlight. Reproduced with permission.103 Copyright 2023, Springer Nature. (C) Energetics and electron transfer processes and degradation of acetophenone derivatives occurring on dye-sensitized TiO2. Reproduced with permission.104 Copyright 2015, MDPI. (D) Schematic of the working mechanisms for the Yb/Er/Nd@Nd@Mn:TiO2 photocatalyst under 808 nm NIR irradiation. Reproduced with permission.105 Copyright 2019, American Chemical Society. | |
4.2. Sensitization
The addition of sensitizers can broaden the semiconductor absorbance spectrum, which can produce a response to visible light or even NIR light. Sensitizers will be absorbed on the surface of TiO2 with a narrow band gap and higher CB position than TiO2. When photoexcited, optical quantum is absorbed to produce electronic transition, the chemical potential of the excited state is more negative than that of the CB, and the excited electron transfer is injected into the CB of TiO2. The electrons in the CB will be transferred to the surface to participate in the photocatalytic reaction, thus expanding the wavelength response range of TiO2 (Fig. 4(C)). Yang et al.106 studied the design process of chlorophyll-sensitized TiO2. They used a one-step synthesis method to extract chlorophyll from spinach and dope it into TiO2 by heating the reactor. The results showed that TiO2 doped with chlorophyll possessed two different absorption bands near 407 and 668 nm, indicating that the chlorophyll-sensitized TiO2 had wide absorption from UV to visible light region. Wei et al.107 synthesized CdSe quantum dot (QDs)-sensitized TiO2 photoanode on fluorine-doped tin oxide-coated glass by molten salt-assisted self-assembly and constructed an efficient photoelectric chemical sensor to detect rutin. It was found that CdSe could transfer photoexcited electrons to the CB of TiO2 and improve the photoelectric activity of TiO2. In conclusion, the addition of sensitizer to TiO2 could broaden the spectral range of TiO2, generate visible light response, and improve the optical quantum efficiency. However, due to the different components and types of sensitizers and catalytic antibacterial materials, the mechanism of action in the process of photocatalytic antibacterial effect is more complicated and needs to be further studied.
4.3. Up-conversion luminescence
Many semiconductors cannot be excited by NIR light, and the UV light that excites them and part of the visible light used in bone tissue engineering can damage normal human tissue. In addition, their penetration is not as good as NIR light and cannot reach the inside of bone tissue. Up-conversion materials are those with anti-Stokes properties, which can convert infrared light to shorter wavelengths of light (Fig. 4(D)). The most widely used material with the highest light conversion efficiency is NaYF4. Zhang et al.108 synthesized an up-conversion photocatalytic system (UCNPs-CPZ-PVP) using a β-carboxylic phthalocyanine (CPZ) molecule, up-conversion nanoparticles (LiYF4:Yb/Er) and polyethylpyrrolidone (PVP). The UCNPs-CPZ-PVP could absorb NIR and release ROS, and it had good bacteriostatic effect on S. aureus and E. coli. However, photoconversion materials are often difficult to biodegrade, which hinders their application in bone tissue engineering.
5. Application in bone tissue engineering
Semiconductor photocatalytic antibacterial materials have been used in bone tissue engineering, including osteomyelitis treatment, bone defect repair and dental tissue regeneration. Some examples of the application in bone tissue engineering are shown in Table 2.
Table 2 Application of semiconductor photocatalytic antibacterial materials in bone tissue engineering
Application |
Materials |
Bacteria or cells |
Efficiency |
Antibacterial substance |
Function |
Ref. |
Osteosarcoma |
TiO2 |
S. aureus
|
98.7% |
• NO |
Ablate the tumor and eliminate the biofilm |
109
|
• ROS |
Implant |
S-TiO2 |
S. aureus
|
99.995% |
• PTT |
Effective antibacterial activity and accelerated bone integration in vivo |
110
|
• ROS |
Implant |
TiO2 |
MRSA |
98% |
• ROS |
Prevents implant infection and promotes bone tissue regeneration |
38
|
Dental implant |
TiO2 |
Streptococcus sanguis
|
— |
• ROS |
Reduce the biofilm on the surface of dental implants |
111
|
Bone scaffold |
ZnO |
E. coli
|
99.9% |
• ROS |
Excellent photocatalytic antibacterial ability and good cytocompatibility for cell activity and proliferation |
112
|
Implant |
Bi2S3 |
S. aureus
|
99.45% |
• Ag+ |
NIR light effectively eliminates biofilms in a short time |
113
|
|
• ROS |
Osteosarcoma |
TiO2 |
Osteosarcoma cell |
100% |
• ROS |
Ablating osteosarcoma and promoting osteogenic differentiation of cells |
114
|
• PTT |
Osteosarcoma |
ZnO |
Osteosarcoma cell |
48% |
• Zn2+ |
Good antibacterial activity and good cytocompatibility |
115
|
• Fe3+ |
• ROS |
Osteomyelitis |
MoS2 |
MRSA |
99.58% |
• Zn2+ |
Treat MRSA infection osteomyelitis and promote bone growth |
116
|
• ROS |
Arthritis |
CeO2 |
Synovial cells |
67.2% |
• PTT |
Synovial cell proliferation and gradual elimination of bone erosion |
117
|
• ROS |
Osteomyelitis |
MoS2 |
S. aureus
|
— |
• ROS |
Treatment of osteomyelitis caused by S. aureus |
118
|
Osteomyelitis |
BaTiO3 |
S. aureus
|
99% |
• ROS |
Removes pathogens and enhances osteoblast adhesion and differentiation |
119
|
Bone scaffold |
TiO2 |
S. aureus
|
100% |
• ROS |
Enhanced mitochondrial activity and proliferation without inducing additional intracellular ROS of osteoblasts and promoted osseointegration in infected rat tibias |
120
|
Periodontitis |
Copper tannic acid coordination |
Porphyromonas gingivalis
|
98.51% |
• ROS |
Antibacterial, antiplaque properties, and the expression of osteogenetic genes successively |
121
|
Actinomyces viscosus
|
97.17% |
S. aureus
|
93.13% |
E. coli
|
90.51% |
Osteomyelitis |
ZnO |
S. aureus
|
74.18% |
• Zn2+ |
Excellent antibacterial activity and enhanced bone regeneration |
122
|
E. coli
|
63.02% |
• ROS |
Dental implants |
ZnO, TiO2 |
Oral pathogens |
75% |
• Zn2+ |
Higher capability for enhancing bone regeneration, antibiosis, and osseointegration |
123
|
• ROS |
Bone scaffold |
TiO2 |
S. aureus
|
75% |
• ROS |
Synergistic antibacterial effect of ROS and PTT |
124
|
MoS2 |
• PTT |
Implant |
TiO2 |
E. coli
|
100% |
• ROS |
Rapid bactericidal method with high biosafety based on multiple synergistic antimicrobial methods |
82
|
S. aureus
|
99.9% |
• PTT |
Implant |
TiO2/GO |
Streptococcus mutans
|
— |
• ROS |
Excellent antibacterial activity and minimal inflammatory response |
125
|
• PTT |
Implant |
Cus/GO |
S. aureus
|
— |
• ROS |
Endowing powerful antibacterial and bone-promoting abilities for implant-associated infections |
126
|
• PTT |
Bone scaffold |
ZnO |
E. coli
|
87.0% |
• Zn2+ |
High cell compatibility and antimicrobial rate |
127
|
S. aureus
|
88.4% |
• ROS |
5.1. Osteomyelitis treatment
Bacterial infection due to trauma or surgery is a major cause of osteomyelitis, and severe osteomyelitis can lead to osteoporosis and pathological fractures. At present, the clinical treatment of osteomyelitis mainly relies on anti-infective drugs and surgical intervention, but drug therapy takes many weeks and is prone to relapse, while surgical debridement may aggravate bone loss. Semiconductor photocatalysis technology provides a new strategy for the treatment of osteomyelitis, such as the use of ROS produced by photocatalysis to inhibit bacterial growth; some examples are shown in Fig. 5. Li et al.128 prepared Zn-doped C3N4 by two high-temperature combustion processes aimed at osteomyelitis caused by bacterial infection. The results demonstrated that after secondary calcination, the incorporation of Zn2+ into C3N4 improved its electronic structure and promoted interfacial polarization and electron transfer. Under microwave irradiation, the resulting plasma collided with oxygen, producing 1O2 and ˙O2−, and the eradication rate of S. aureus reached 99.8% within 15 min. In addition, the released Zn2+ promoted the polarization of macrophages, further enhancing bone repair.
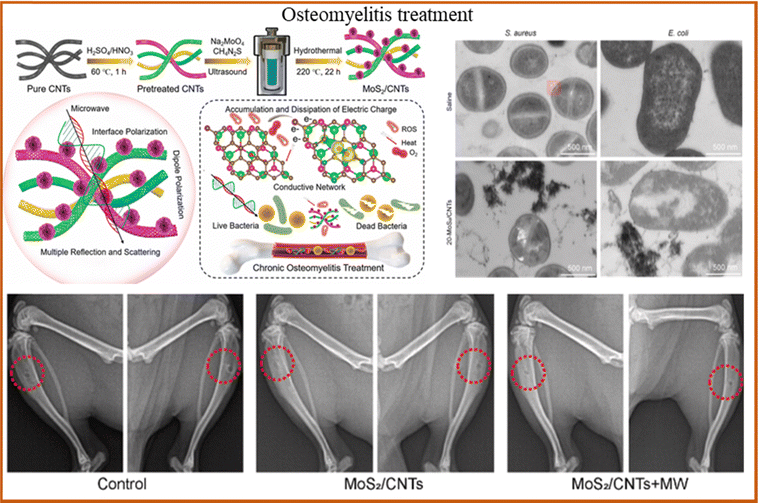 |
| Fig. 5 MoS2/CNTs heterojunctions generate heat and ROS under light, enabling treatment of deep tissue infections. TEM images of S. aureus and E. coli co-culture samples, and X-ray images of the tibias of the mice. Reproduced with permission.129 Copyright 2024, Elsevier. | |
Jin et al.130 used MoS2/Fe3O4 to treat S. aureus infectious osteomyelitis. The experimental results indicated that the magnetic coordination between ROS produced by MoS2/Fe3O4 and Fe3O4 increased the thermal sensitivity and permeability of bacterial cell membrane, prevented bacteria from spreading through the blood, targeted bacteria and killed them in situ. Wu et al.129 prepared core–shell heterojunction ZnO/Ag2S nanoparticles by a wet chemical method for the treatment of early bacterial infection in osteomyelitis. The experimental results showed that 99.9% of E. coli and S. aureus could be eradicated after 10 min of irradiation when the material concentration was 100 μg mL−1. In addition, the release of Zn ions showed good osteogenic effect. However, existing research shows that the recurrence rate of osteomyelitis is still high, most treatment methods are still in the experimental stage, and few have been applied in clinical practice. In the future, the targeted treatment strategy of minimally invasive injection may be an important development direction of osteomyelitis treatment.
5.2. Bone defect repair
Bone scaffold is an effective means to treat bone defect, but it can easily lead to implant-related infection during implantation, resulting in implantation failure and secondary damage to patients.131–144 Therefore, improving the antimicrobial performance of bone scaffold is an effective strategy to deal with implant infection. For example, the antibacterial effect of bone scaffold is enhanced by introducing antibacterial components or surface modifications into the material (Fig. 6). Ullah et al.145 indicated that Ca and P-doped ZnO nanorods were grown on Ti implant by hydrothermal method to deal with the antibacterial effect of the implant and promote the balance between bones. The results showed that the samples exhibited excellent antibacterial effects against E. coli and S. aureus and improved the inflammatory response around the implant.
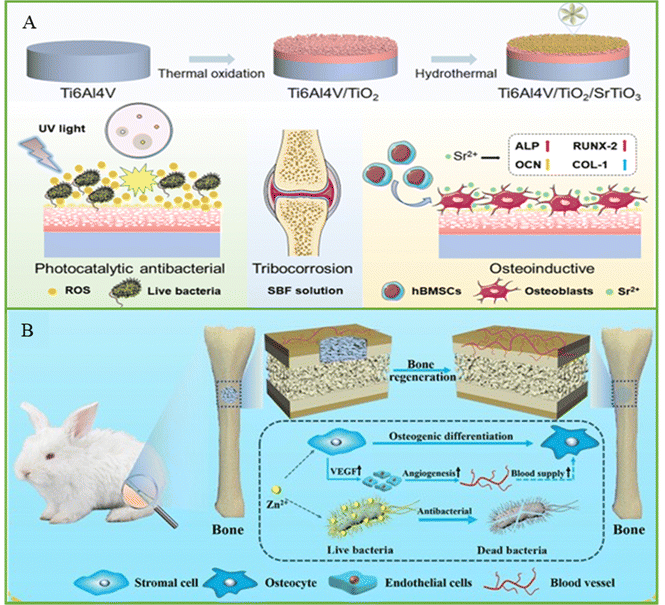 |
| Fig. 6 (A) Schematic of the TiO2/SrTiO3 coating with photocatalytic antibacterial and osteogenesis properties, and the colony of E. coli on a plate after incubating with different samples under dark and UV light. Reproduced with permission.146 Copyright 2022, Elsevier. (B) Schematic of a bone implant with antibacterial and accelerated bone reconstruction functions. Reproduced with permission.147 Copyright 2023, Springer Nature. | |
Wang et al.38 synthesized graphitic acetylene/TiO2 nanofibers by electrostatic assembly to prevent implant bacterial infection. The nanofibers displayed excellent photocatalytic properties and produced a large amount of ROS, which induced bacterial cell wall perforation, cell membrane leakage, and eventually bacterial death. The antibacterial experiment indicated that the antibacterial rate of nanofibers against MRSA reached 98%. In addition, the results of the section of the femur tissue indicated that the nanofiber group had a higher antibacterial effect and bone-inducing ability than the other groups. Lu et al.148 prepared chitosan nanofiber membrane modified with fucoidan and CuS for the prevention of bone infection. The results showed that the antibacterial activity of nanofibers against S. aureus and E. coli reached 99% under light and had good biocompatibility. At present, manufacturing technology has provided assistance for the development of bone scaffolds,149–155 but the antibacterial research on bone scaffolds is still mainly in the theoretical and experimental stages, and the intensity damage of scaffolds and potential damage to human body caused by the light required for photocatalytic antibacterial treatment need to be further evaluated.
5.3. Dental tissue regeneration
Tooth loss due to aging, periodontitis, dental caries and other factors seriously affect people's quality of life. Thus, dental implantation becomes an effective means to solve this problem. However, dental implantation surgery is prone to contact with a variety of bacteria, which can adhere to the surface of the implant, causing peri-implant inflammation. Currently, most dental implants are made of ceramic or metal materials such as Ti and its alloys, zirconia, but these materials do not have antibacterial properties on their own. In order to improve the antimicrobial properties of dental implants, researchers have made many explorations (Fig. 7). For example, the production of a TiO2 oxide layer on the surface of Ti implant by anodization is considered to be an effective way to deal with peri-implant inflammation. Heloisa et al.156 developed TiO2 films with different crystal phases by magnetron sputtering deposition, which showed good antibacterial activity on oral multi-species biofilm models. It is a technology that has the potential to reduce biofilms on the surface of implant abutment, and its photocatalytic effect plays a positive role in the treatment of oral biofilm-related diseases.
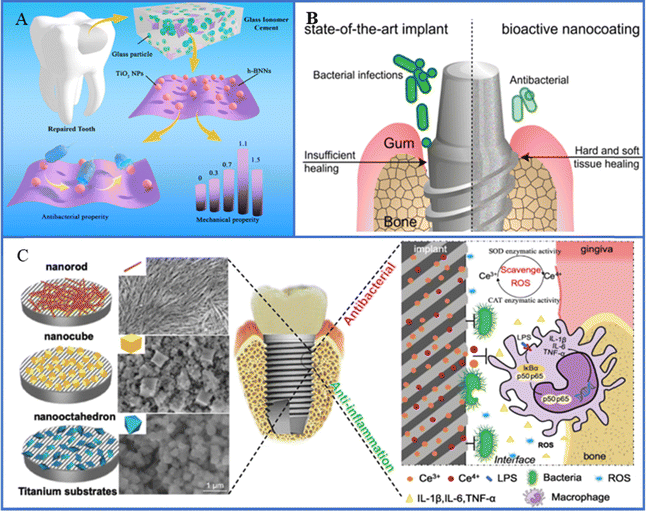 |
| Fig. 7 (A) Schematic of synthesis and performance evaluation of hexagonal boron nitride nanosheet/TiO2 composites. Reproduced with permission.157 Copyright 2022, Frontiers Media. (B) Schematic diagram of versatile antimicrobial nano-architected implant coatings for hard and soft tissue healing. Reproduced with permission.18 Copyright 2021, American Chemical Society. (C) Schematic illustration of implant surface modified by nano-CeO2 for antibacterial and anti-inflammatory properties. Reproduced with permission. Copyright 2019, Elsevier. | |
Chen et al.158 designed ZnO/TiO2 heterojunction nanofilms with photoresponsive properties on the surface of Ti implant to solve the problem of insufficient anti-infection ability of implanted teeth. The nanothin films enhanced the photothermal and photoelectric properties of the implant under NIR irradiation by reducing the band gap and improving the interfacial charge transfer efficiency. Experiments indicated that the implant exhibited excellent broad-spectrum antibacterial effect against three dental pathogens (Porphyromonas gingivalis, Clostridium nucleatum and S. aureus) by destroying the bacterial membrane and increasing the production of intracellular ROS with a bactericidal rate of more than 99.4%. However, the antibacterial strategy of dental implants has not been widely used in clinical practice, and the artificial antibacterial model cannot fully reproduce the complex environment of human implants. Meanwhile, the biocompatibility of antibacterial materials still needs to be further verified. The future direction of development is to develop materials that meet the requirements of dental implant with antibacterial function, facilitate the integration of the implant with the surrounding tissue, and provide personalized solutions for different patients.
6. Conclusions and future prospects
This article summarized the semiconductor materials used for photocatalytic antibacterial treatment, the strategies for improving photocatalytic properties, and their applications in bone tissue engineering. According to the difference in their carriers, semiconductors can be divided into P-type and N-type, and several commonly used photocatalytic semiconductor materials are introduced, such as TiO2, ZnO, and g-C3N4. In order to further improve the antibacterial effect, the efficiency of photocatalysis is improved by reducing the recombination of carriers and expanding the photoresponse range, and the specific methods are summarized such as construction of heterojunction, element doping, and up-conversion luminescence. Finally, the research on the application of semiconductor photocatalytic antimicrobial treatment in bone tissue engineering is summarized, including osteomyelitis treatment, bone defect repair and dental tissue regeneration.
Although semiconductor photocatalytic materials have been used to combat bacterial infection in bone tissue engineering, their clinical applications are still limited, mainly due to the following unresolved issues. Firstly, the biocompatibility of the material is key, but the ROS produced by photocatalysis may damage normal cells, which is not conducive to bone repair, and the clearance of nanomaterials in vivo is also an obstacle.159 Secondly, the lack of standardized models to evaluate the effectiveness of these materials, especially antimicrobial properties and long-term properties, makes comparisons with different bacteria and conditions difficult.160 Thirdly, cumbersome regulatory requirements further complicate the journey from lab to market. To achieve efficient clinical translation, academia, industry and regulators need to work closely together. In recent years, photocatalytic therapy has been transformed into a synergistic therapy by combining with other antimicrobial approaches, bringing the expected benefits, but increasing the composition adjustment and pharmacological complexity, which in turn increases the difficulty of approval.159–162 To overcome these challenges, technologies such as materials genomics, artificial intelligence and machine learning can help drive development, while interdisciplinary collaboration is also key to accelerating the transformation of antimicrobial phototherapy. Semiconductor photocatalytic antimicrobial technology has great potential in the field of bone repair from basic research to clinical application, and future research should focus on the design of multi-functional semiconductor materials with both efficient photocatalytic performance and excellent biocompatibility to meet the needs of bone repair. In addition, research on bone repair and implants should focus on how to design semiconductor materials with durable antibacterial properties and avoid toxic effects on host cells. Through these technological breakthroughs, semiconductor photocatalytic antimicrobial technology can play an important role in the field of bone repair, promoting its transformation from laboratory research to clinical application.
Data availability
Raw data are available from the corresponding author upon reasonable request.
Conflicts of interest
There are no conflicts to declare.
Acknowledgements
This work was supported by the following funds: (1) The Natural Science Foundation of China (52275393, 52475362); (2) National Key Research and Development Program of China (grant no. 2023YFB4605800); (3) Jiangxi Provincial Natural Science Foundation of China (20224ACB204013); (4) The Project of State Key Laboratory of Precision Manufacturing for Extreme Service Performance; (5) Natural Science Foundation of Hunan Province (2023JJ30461); (6) The Science Foundation of Hunan University of Chinese Medicine (Z2023JBGS04); (7) The Fundamental Research Funds for the Central Universities of Central South University (2024ZZTS0325).
References
- Y. Zhao, J. Li, L. Liu, Y. Wang, Y. Ju, C. Zeng, Z. Lu, D. Xie and J. Guo, Adv. Healthcare Mater., 2023, 12, 2300303 Search PubMed.
- D. Huo, F. Wang, F. Yang, T. Lin, Q. Zhong, S.-P. Deng, J. Zhang, S. Tan and L. Huang, J. Mater. Sci. Technol., 2024, 179, 208–223 Search PubMed.
- Z. Yu, Z. Wang, Y. Chen, Y. Wang, L. Tang, Y. Xi, K. Lai, Q. Zhang, S. Li, D. Xu, A. Tian, M. Wu, Y. Wang, G. Yang, C. Gao and T. Huang, Biomaterials, 2025, 313, 122772 Search PubMed.
- B. Fang, P. Qiu, C. Xia, D. Cai, C. Zhao, Y. Chen, H. Wang, S. Liu, H. Cheng, Z. Tang, B. Wang, S. Fan and X. Lin, Biomaterials, 2021, 268, 120603 Search PubMed.
- Y. Ren, J. Weeks, T. Xue, J. Rainbolt, K. L. de Mesy Bentley, Y. Shu, Y. Liu, E. Masters, P. Cherian, C. E. McKenna, J. Neighbors, F. H. Ebetino, E. M. Schwarz, S. Sun and C. Xie, Bone Res., 2023, 11, 51 Search PubMed.
- S. Huo, Z. Lyu, X. Su, F. Wang, J. Liu, S. Liu, X. Liu, X. Bao, J. Zhang, K. Zheng and G. Xu, Composites, Part B, 2023, 253, 110521 Search PubMed.
- X. Zhang, Q. Li, L. Li, J. Ouyang, T. Wang, J. Chen, X. Hu, Y. Ao, D. Qin, L. Zhang, J. Xue, J. Cheng and W. Tao, ACS Nano, 2023, 17, 6466–6479 Search PubMed.
- D. Mo, M. Pan, W. Chen, Q. Liu, Y. Yu, L. Yuan, Y. Yang, H. Deng, M. Wang and Z. Qian, Adv. Funct. Mater., 2024, 34, 2313569 Search PubMed.
- L. Zhou, Y. Deng, Y. Ren, H. L. Poon, W. Y. Chu, H. Wang and Y. K. Chan, Chem. Eng. J., 2024, 482, 148978 Search PubMed.
- Q. Yu, C. Wang, X. Zhang, H. Chen, M. X. Wu and M. Lu, ACS Nano, 2024, 18, 14085–14122 Search PubMed.
- X. Wang, X. Wan, X. Qin, C. Chen, X. Qian, Y. Guo, Q. Xu, W.-B. Cai, H. Yang and K. Jiang, ACS Catal., 2022, 12, 9437–9445 Search PubMed.
- Y. Liu, M. Wang, B. Zhang, D. Yan and X. Xiang, ACS Catal., 2022, 12, 6946–6957 Search PubMed.
- Z. Zhang, J. Fan, J. Du and X. Peng, Coord. Chem. Rev., 2021, 427, 213575 Search PubMed.
- F. Dalanta, T. D. Kusworo and N. Aryanti, Appl. Catal., B, 2022, 316, 121576 Search PubMed.
- A. Ali, J. Lee, K. Kim, H. Oh and G.-C. Yi, Adv. Healthcare Mater., 2024, 13, 2304140 Search PubMed.
- U. M. Dankawu, H. Y. Hafeez, C. E. Ndikilar, J. Mohammed, A. B. Suleiman and A. S. Shuaibu, Int. J. Hydrogen Energy, 2024, 67, 1218–1242 Search PubMed.
- T. O. Ajiboye and D. C. Onwudiwe, Results Chem., 2021, 3, 100151 Search PubMed.
- M. T. Matter, L. Maliqi, K. Keevend, S. Guimond, J. Ng, E. Armagan, M. Rottmar and I. K. Herrmann, ACS Appl. Mater. Interfaces, 2021, 13, 33300–33310 Search PubMed.
- J. Murillo-Sierra, A. Hernández-Ramírez, L. Hinojosa-Reyes and J. Guzmán-Mar, Chem. Eng. J. Adv., 2021, 5, 100070 Search PubMed.
- P. Shandilya, S. Sambyal, R. Sharma, P. Mandyal and B. Fang, J. Hazard. Mater., 2022, 428, 128218 Search PubMed.
- Z. Thiehmed, A. Shakoor and T. Altahtamouni, Catalysts, 2021, 11, 1283 Search PubMed.
- H. Moon, M. S. Goh, M. Cha, U.-S. Kim, H. S. Whang, N. Son and M. Kang, Appl. Surf. Sci., 2022, 606, 154787 Search PubMed.
- Z. Meng, J. Zhang, C. Jiang, C. Trapalis, L. Zhang and J. Yu, Small, 2024, 20, 2308952 Search PubMed.
- K. Sharma, S. Patial, P. Singh, A. A. P. Khan, V. Saini, A. K. Nadda, C. M. Hussain, V.-H. Nguyen, C. C. Nguyen and T. B. H. Nguyen, Sol. Energy, 2022, 231, 546–565 Search PubMed.
- J.-C. Kao, J.-P. Chou, P.-J. Chen and Y.-C. Lo, Mater. Today Phys., 2023, 38, 101218 Search PubMed.
- P. Raizada, A. Sudhaik, S. Patial, V. Hasija, A. A. P. Khan, P. Singh, S. Gautam, M. Kaur and V.-H. Nguyen, Arabian J. Chem., 2020, 13, 8424–8457 Search PubMed.
- L. Isac, C. Cazan, L. Andronic and A. Enesca, Catalysts, 2022, 12, 1135 Search PubMed.
- Y. Jing, K. Liang, N. S. Muir, H. Zhou, Z. Li, J. M. Palasz, J. Sorbie, C. Wang, S. K. Cushing, C. P. Kubiak, Z. Sofer, S. Li and W. Xiong, Angew. Chem., Int. Ed., 2024, 63, e202405123 Search PubMed.
- Y. Zi, J. Zhu, L. Hu, M. Wang and W. Huang, Small. Sci., 2022, 2, 2100098 Search PubMed.
- L. Che, J. Pan, K. Cai, Y. Cong and S.-W. Lv, Sep. Purif. Technol., 2023, 315, 123708 Search PubMed.
- Y. Duan, X. Zhu, Q. Luo, L. Wang, Z. Li and D. Wang, J. Catal., 2021, 400, 160–165 Search PubMed.
- M. Amarnath and K. Gurunathan, J. Alloys Compd., 2021, 857, 157584 Search PubMed.
- Z. Lin, C. Du, B. Yan and G. Yang, J. Catal., 2019, 372, 299–310 Search PubMed.
- J. Chu, G. Sun, X. Han, X. Chen, J. Wang, W. Hu, I. Waluyo, A. Hunt, Y. Du, B. Song and P. Xu, Nanoscale, 2019, 11, 15633–15640 Search PubMed.
- S. Zhu, W. Liao, M. Zhang and S. Liang, Chem. Eng. J., 2019, 361, 461–469 Search PubMed.
- H. Sun, X. Guo and A. Facchetti, Chem, 2020, 6, 1310–1326 Search PubMed.
- D. Yuan, W. Liu and X. Zhu, Chem. Soc. Rev., 2023, 52, 3842–3872 Search PubMed.
- R. Wang, M. Shi, F. Xu, Y. Qiu, P. Zhang, K. Shen, Q. Zhao, J. Yu and Y. Zhang, Nat. Commun., 2020, 11, 4465 Search PubMed.
- H. Mohd Yusof, R. Mohamad, U. H. Zaidan and N. A. Abdul Rahman, J. Anim. Sci. Biotechnol., 2019, 10, 57 Search PubMed.
- Z. Teng, N. Yang, H. Lv, S. Wang, M. Hu, C. Wang, D. Wang and G. Wang, Chem, 2019, 5, 664–680 Search PubMed.
- Z. Xu, J. Wu, B. Lovely, Y. Li, M. Ponder, K. Waterman, Y.-T. Kim, D. Shuai, Y. Yin and H. Huang, J. Hazard. Mater., 2024, 480, 136296 Search PubMed.
- N. Thakur, N. Thakur, A. Kumar, V. K. Thakur, S. Kalia, V. Arya, A. Kumar, S. Kumar and G. Z. Kyzas, Sci. Total Environ., 2024, 914, 169815 Search PubMed.
- Y.-h Lyu, F. Wei, T. Zhang, L. Luo, Y. Pan, X. Yang, H. Yu and S. Zhou, J. Alloys Compd., 2021, 876, 160016 Search PubMed.
- C. Xiao, G. Dong, T. Liu, L. Wang and C. Zhu, Sep. Purif. Technol., 2024, 347, 127673 Search PubMed.
- S. Varnagiris, M. Urbonavicius, S. Sakalauskaite, R. Daugelavicius, L. Pranevicius, M. Lelis and D. Milcius, Sci. Total Environ., 2020, 720, 137600 Search PubMed.
- R. Wang, R. Liu, S. Luo, J. Wu, D. Zhang, T. Yue, J. Sun, C. Zhang, L. Zhu and J. Wang, Chem. Eng. J., 2021, 421, 129596 Search PubMed.
- J. Wang, Z. Wang, W. Wang, Y. Wang, X. Hu, J. Liu, X. Gong, W. Miao, L. Ding and X. Li, Nanoscale, 2022, 14, 6709–6734 Search PubMed.
- R. C. Nonato, L. H. Mei, B. C. Bonse, C. V. Leal, C. E. Levy, F. A. Oliveira, C. Delarmelina, M. C. Duarte and A. R. Morales, Polym. Eng. Sci., 2022, 62, 1147–1155 Search PubMed.
- N. Babayevska, Ł. Przysiecka, I. Iatsunskyi, G. Nowaczyk, M. Jarek, E. Janiszewska and S. Jurga, Sci. Rep., 2022, 12, 8148 Search PubMed.
- W.-N. Wang, P. Pei, Z.-Y. Chu, B.-J. Chen, H.-S. Qian, Z.-B. Zha, W. Zhou, T. Liu, M. Shao and H. Wang, Chem. Eng. J., 2020, 397, 125488 Search PubMed.
- J. Guo, J. Zhou, Z. Sun, M. Wang, X. Zou, H. Mao and F. Yan, Acta Biomater., 2022, 146, 370–384 Search PubMed.
- R. Sakthivel, S. Kubendhiran and S.-M. Chen, Ultrason. Sonochem., 2019, 54, 68–78 Search PubMed.
- W.-N. Wang, C.-Y. Zhang, M.-F. Zhang, P. Pei, W. Zhou, Z.-B. Zha, M. Shao and H.-S. Qian, Chem. Eng. J., 2020, 381, 122630 Search PubMed.
- S. Puebla, R. D’Agosta, G. Sanchez-Santolino, R. Frisenda, C. Munuera and A. Castellanos-Gomez, npj 2D Mater. Appl., 2021, 5, 37 Search PubMed.
- Q. Gao, Z. Wang, Y. Rao, Y. Zhao, J. Cao, K.-F. Ho, Y. Zhai, M. Xiong, J. Li and Y. Huang, J. Hazard. Mater., 2023, 443, 130275 Search PubMed.
- Y.-Y. Yang, H.-P. Feng, C.-G. Niu, D.-W. Huang, H. Guo, C. Liang, H.-Y. Liu, S. Chen, N. Tang and L. Li, Chem. Eng. J., 2021, 426, 131902 Search PubMed.
- H. Boumeriame, E. S. Da Silva, A. S. Cherevan, T. Chafik, J. L. Faria and D. Eder, J. Energy Chem., 2022, 64, 406–431 Search PubMed.
- T. S. Rad, Z. Ansarian, R. D. C. Soltani, A. Khataee, Y. Orooji and F. Vafaei, J. Hazard. Mater., 2020, 399, 123062 Search PubMed.
- E. Nikoomanzari and A. Fattah-alhosseini, J. Magnesium Alloys, 2024, 12, 2674–2694 Search PubMed.
- D. Zhang, J. Zhou, F. Peng, J. Tan, X. Zhang, S. Qian, Y. Qiao, Y. Zhang and X. Liu, J. Mater. Sci. Technol., 2022, 105, 57–67 Search PubMed.
- Y. Xiong, D. Xu, Y. Feng, G. Zhang, P. Lin and X. Chen, Adv. Mater., 2023, 35, 2206939 Search PubMed.
- W. Lu, T. Gu, X. Jing, Y. Zhu, L. Yu, S. Hou, T. Pang, N. Lu and Z. Zhang, J. Alloys Compd., 2023, 968, 171864 Search PubMed.
- J. Zeng, Z. Li, H. Jiang and X. Wang, Mater. Horiz., 2021, 8, 2964–3008 Search PubMed.
- H. Siddiqui, M. S. Qureshi and F. Z. Haque, Nano-Micro Lett., 2020, 12, 29 Search PubMed.
- J. Liu, B. Yin, S. Li, L. Mi, G. Yin, F. Li, L. Zhong, N. Bai and X. Li, J. Ind. Eng. Chem., 2024, 133, 345–354 Search PubMed.
- F. Chen, Y. Luo, X. Liu, Y. Zheng, Y. Han, D. Yang and S. Wu, Adv. Healthcare Mater., 2022, 11, 2200360 Search PubMed.
- H. Bahramian, A. Fattah-alhosseini, M. Karbasi, E. Nikoomanzari and S. Giannakis, Chem. Eng. J., 2023, 476, 146588 Search PubMed.
- G. Guan, L. Zhang, J. Zhu, H. Wu, W. Li and Q. Sun, J. Hazard. Mater., 2021, 402, 123542 Search PubMed.
- A. George, D. M. A. Raj, A. D. Raj, A. A. Irudayaraj, J. Arumugam, M. Senthil kumar, H. J. Prabu, S. J. Sundaram, N. A. Al-Dhabi, M. V. Arasu, M. Maaza and K. Kaviyarasu, Surf. Interfaces, 2020, 21, 100761 Search PubMed.
- Z. Zou, J. Sun, Q. Li, Y. Pu, J. Liu, R. Sun, L. Wang and T. Jiang, Colloids Surf., B, 2020, 189, 110875 Search PubMed.
- X. Xue, H. Zhang, H. Liu, S. Wang, J. Li, Q. Zhou, X. Chen, X. Ren, Y. Jing, Y. Deng, Z. Geng, X. Wang and J. Su, Adv. Funct. Mater., 2022, 32, 2202470 Search PubMed.
- X. L. Hu, L. Chu, X. Dong, G. R. Chen, T. Tang, D. Chen, X. P. He and H. Tian, Adv. Funct. Mater., 2019, 29, 1806986 Search PubMed.
- Z. H. Jabbar and B. H. Graimed, J. Water Process Eng., 2022, 47, 102671 Search PubMed.
- D. Shiny, D. Usha, P. M. Anjana, S. Smonia Joe Princy, M. H. Almutairi, B. O. Almutairi, M. R. Bindhu and T. M. Aminabhavi, Chem. Eng. J., 2024, 498, 155720 Search PubMed.
- R. Zhang, R. Chilivery, D. Yao, W. Chen, F. Lu, W. Gao, Y. Fang, Z. Zhong and Y. Song, Appl. Surf. Sci., 2022, 574, 151683 Search PubMed.
- S. Somekawa, H. Watanabe, Y. Ono, Y. Oaki and H. Imai, Mater. Lett., 2021, 304, 130609 Search PubMed.
- B. Sun, F. Wu, Q. Zhang, X. Chu, Z. Wang, X. Huang, J. Li, C. Yao, N. Zhou and J. Shen, J. Colloid Interface Sci., 2021, 584, 505–519 Search PubMed.
- C. Jing, Y. Zhang, J. Zheng, S. Ge, J. Lin, D. Pan, N. Naik and Z. Guo, Particuology, 2022, 69, 111–122 Search PubMed.
- W. Wang, J. Fang and H. Chen, J. Alloys Compd., 2020, 819, 153064 Search PubMed.
- M. Kazemi, A. Akbari, Z. Sabouri, S. Soleimanpour, H. Zarrinfar, M. Khatami and M. Darroudi, Bioprocess Biosyst. Eng., 2021, 44, 1215–1225 Search PubMed.
- Y. Liu, Y. Tian, Q. Han, J. Yin, J. Zhang, Y. Yu, W. Yang and Y. Deng, Chem. Eng. J., 2021, 410, 128209 Search PubMed.
- X. Zhang, G. Zhang, M. Chai, X. Yao, W. Chen and P. K. Chu, Bioact. Mater., 2021, 6, 12–25 Search PubMed.
- M. Yang, S. Qiu, E. Coy, S. Li, K. Załęski, Y. Zhang, H. Pan and G. Wang, Adv. Mater., 2022, 34, 2106314 Search PubMed.
- F. Li, L. Cheng, J. Fan and Q. Xiang, J. Chem. Mater. A, 2021, 9, 23765–23782 Search PubMed.
- J. Ma, T. J. Miao and J. Tang, Chem. Soc. Rev., 2022, 51, 5777–5794 Search PubMed.
- Y. Ma, D. Xu, W. Chen, Y. Tang, X. Wang, L. Li and J. Wang, Appl. Surf. Sci., 2022, 572, 151432 Search PubMed.
- M. Wang, L. Nian, Y. Cheng, B. Yuan, S. Cheng and C. Cao, Chem. Eng. J., 2021, 426, 130832 Search PubMed.
- J. Li, P. Jiménez-Calvo, E. Paineau and M. N. Ghazzal, Catalysts, 2020, 10, 89 Search PubMed.
- D. Salazar-Marín, G. Oza, J. A. D. Real, A. Cervantes-Uribe, H. Pérez-Vidal, M. K. Kesarla, J. G. T. Torres and S. Godavarthi, Appl. Surf. Sci. Adv., 2024, 19, 100536 Search PubMed.
- H. Derikvandi and A. Nezamzadeh-Ejhieh, J. Colloid Interface Sci., 2017, 490, 314–327 Search PubMed.
- Q. Xu, L. Zhang, B. Cheng, J. Fan and J. Yu, Chem, 2020, 6, 1543–1559 Search PubMed.
- A. Meng, B. Zhu, Y. Zhong, S. Zhou, P. Han and Y. Su, Energy Rev., 2023, 2, 100052 Search PubMed.
- Z. Yang, C. Chen, B. Li, Y. Zheng, X. Liu, J. Shen, Y. Zhang and S. Wu, Chem. Eng. J., 2023, 451, 139127 Search PubMed.
- H. Li, J. Zhong, H. Zhu, Y. Yang, M. Ding, L. Luo, Y. Huo and H. Li, ACS Appl. Bio Mater., 2019, 2, 4892–4903 Search PubMed.
- M. Faraji, N. Mohaghegh and A. Abedini, J. Photochem. Photobiol., B, 2018, 178, 124–132 Search PubMed.
- Y. Lan, Z. Li, D. Li, W. Xie, G. Yan and S. Guo, Chem. Eng. J., 2020, 392, 123686 Search PubMed.
- F. Guo, W. Shi, M. Li, Y. Shi and H. Wen, Sep. Purif. Technol., 2019, 210, 608–615 Search PubMed.
- X. Zhao, S. Wang, D. Shan, Y. Xu, L. Wu, H. Guo, G. Che, C. Liu and J. Du, Chem. Eng. J., 2024, 495, 153675 Search PubMed.
- W. Jiang, H. Zhu, J. Yang, B. Q. L. Low, W.-Y. Wu, M. Chen, J. Ma, R. Long, J. Low, H. Zhu, J. Z. X. Heng, K. Y. Tang, C. H. T. Chai, M. Lin, Q. Zhu, Y.-W. Zhang, D. Chi, Z. Li, X. J. Loh, Y. Xiong and E. Ye, Adv. Sci., 2023, 10, 2303448 Search PubMed.
- E. Erusappan, S. Thiripuranthagan, R. Radhakrishnan, M. Durai, S. Kumaravel, T. Vembuli and N. J. Kaleekkal, J. Environ. Chem. Eng., 2021, 9, 105776 Search PubMed.
- S. Patnaik, D. P. Sahoo and K. Parida, Carbon, 2021, 172, 682–711 Search PubMed.
- X. Lu, F. Liu, Y. Dang, M. Li, M. Ruan, M. Wu, C. Zhu, T. Mani, S. L. Suib and P.-X. Gao, Appl. Surf. Sci., 2022, 591, 153116 Search PubMed.
- M. S. Rajan, A. John, M. Yoon and J. Thomas, Environ. Sci. Pollut. Res., 2023, 30, 60638–60653 Search PubMed.
- S. Kohtani, M. Mori, E. Yoshioka and H. Miyabe, Catalysts, 2015, 5, 1417–1424 Search PubMed.
- Z. Nie, X. Ke, D. Li, Y. Zhao, L. Zhu, R. Qiao and X. L. Zhang, J. Phys. Chem. C, 2019, 123, 22959–22970 Search PubMed.
- H. Yang, Y. Yang, L. Jiang, Y. Wang, Y. Chen, J. He, W. Wang and J. Wang, Nano Select, 2022, 3, 140–146 Search PubMed.
- L. Wei, X. Li and S. Feng, Int. J. Electrochem. Sci., 2021, 16, 151032 Search PubMed.
- Y. Zhang, P. Huang, D. Wang, J. Chen, W. Liu, P. Hu, M. Huang, X. Chen and Z. Chen, Nanoscale, 2018, 10, 15485–15495 Search PubMed.
- G. Zhang, Z. Wu, Y. Yang, J. Shi, J. Lv, Y. Fang, Z. Shen, Z. Lv, P. Li, X. Yao, W. Chen, X. Wei, P. K. Chu and X. Zhang, Chem. Eng. J., 2022, 428, 131155 Search PubMed.
- K. Su, L. Tan, X. Liu, Z. Cui, Y. Zheng, B. Li, Y. Han, Z. Li, S. Zhu, Y. Liang, X. Feng, X. Wang and S. Wu, ACS Nano, 2020, 14, 2077–2089 Search PubMed.
- B. E. Nagay, C. Dini, J. M. Cordeiro, A. P. Ricomini-Filho, E. D. De Avila, E. C. Rangel, N. C. Da Cruz and V. A. Barao, ACS Appl. Mater. Interfaces, 2019, 11, 18186–18202 Search PubMed.
- C. Shuai, X. Yuan, Y. Shuai, G. Qian, J. Yao, W. Xu, S. Peng and W. Yang, Mater. Today Nano, 2022, 18, 100210 Search PubMed.
- L. Hong, X. Liu, L. Tan, Z. Cui, X. Yang, Y. Liang, Z. Li, S. Zhu, Y. Zheng and K. W. K. Yeung, Adv. Healthcare Mater., 2019, 8, 1900835 Search PubMed.
- Z. Wu, Q. Tian, J. Wang, Y. Feng, L. Li, C. Xu, J. Lv and Z. Lv, Colloids Surf., B, 2022, 211, 112296 Search PubMed.
- V. Saxena and L. M. Pandey, J. Trace Elem. Med. Biol., 2020, 57, 126416 Search PubMed.
- X. Feng, J. Lei, L. Ma, Q. Ouyang, Y. Zeng, H. Liang, C. Lei, G. Li, L. Tan, X. Liu and C. Yang, Small, 2022, 18, 2105775 Search PubMed.
- Z. Li, X. Wu, W. Gu, P. Zhou, H. Chen, W. Wang, Z. Cai, S. Cao, K. Guo, X. Zheng and F. Gao, Chem. Eng. J., 2022, 446, 136904 Search PubMed.
- L. Jin, Y. Zheng, X. Liu, Y. Zhang, Z. Li, Y. Liang, S. Zhu, H. Jiang, Z. Cui and S. Wu, Small, 2022, 18, 2204028 Search PubMed.
- T. Ding, F. Liu, H. Xin, Y. Chen, L. Kong, J. Han, D. Ma, Y. Han and L. Zhang, Nano Today, 2024, 54, 102069 Search PubMed.
- J. Ye, B. Li, M. Li, Y. Zheng, S. Wu and Y. Han, Acta Biomater., 2020, 107, 313–324 Search PubMed.
- Y. Xu, Y. Luo, Z. Weng, H. Xu, W. Zhang, Q. Li, H. Liu, L. Liu, Y. Wang, X. Liu, L. Liao and X. Wang, ACS Nano, 2023, 17, 18732–18746 Search PubMed.
- T. He, H. Chen, P. Liu, H. Shi, X. Xu, C. Feng, Y. Wang, X. Li, N. Lei, Y. Xiao, X. Zhu, J. Xu and X. Zhang, J. Mater. Sci. Technol., 2023, 163, 168–181 Search PubMed.
- Z. Wen, X. Shi, X. Li, W. Liu, Y. Liu, R. Zhang, Y. Yu and J. Su, ACS Appl. Mater. Interfaces, 2023, 15, 15235–15249 Search PubMed.
- X. Han, G. Zhang, M. Chai and X. Zhang, Biomed. Mater., 2021, 16, 025018 Search PubMed.
- M.-Z. Chai, M.-W. An, X.-Y. Zhang and P.-K. Chu, Rare Met., 2022, 41, 540–545 Search PubMed.
- R. Lv, Y.-Q. Liang, Z.-Y. Li, S.-L. Zhu, Z.-D. Cui and S.-L. Wu, Rare Met., 2022, 41, 639–649 Search PubMed.
- Y.-L. Li, J. He, H.-X. Ye, C.-C. Zhao, W.-W. Zhu, X. Lu and F.-Z. Ren, Rare Met., 2022, 41, 546–558 Search PubMed.
- B. Li, H. Zhu, Y. Lv, C. Wang, S. Wu, S. Zhu, Y. Zheng, H. Jiang, Y. Zhang, Z. Li, Z. Cui and X. Liu, Small, 2023, 19, 2303484 Search PubMed.
- L. Jin, S. Wu, C. Mao, C. Wang, S. Zhu, Y. Zheng, Y. Zhang, Z. Li, Z. Cui, H. Jiang and X. Liu, Bioact. Mater., 2024, 31, 284–297 Search PubMed.
- L. Jin, Y. Zheng, X. Liu, Y. Zhang, Z. Li, Y. Liang, S. Zhu, H. Jiang, Z. Cui and S. Wu, Small, 2022, 18, 2204028 Search PubMed.
- F. Qi, W. Liu, Z. Li, S. Peng, Y. Chen, W. Tan and C. Shuai, ACS Appl. Polym. Mater., 2024, 6, 6581–6593 Search PubMed.
- W. Yang, C. Zhou, C. He, Y. Yang, W. Aiyiti, L. Xu and C. Shuai, J. Colloid Interface Sci., 2025, 678, 260–271 Search PubMed.
- X. Yao, D. Li, C. Gao, Y. Deng, J. Zhang and C. Shuai, Adv. Power. Mater., 2024, 3, 100216 Search PubMed.
- X. Tian, D. Li, Q. Lian, L. Wang, Z. Lu, K. Huang, F. Wang, Q. Liang, H. Zhang, Z. Meng, J. He, C. Sun, T. Liu, C. Huo, L. Wu and B. Lu, Addit Manuf. Front., 2024, 3, 200140 Search PubMed.
- D. Lin, C. Zhang, X. Chen, N. Wang and L. Yang, Addit. Manuf. Front., 2024, 3, 200123 Search PubMed.
- C. Ma, Q. Zeng, L. Yu, S. Yu, J. Song, Y. Ma and X. Dong, Addit. Manuf. Front., 2023, 2, 100097 Search PubMed.
- A. Chen, J. Su, Y. Li, H. Zhang, Y. Shi, C. Yan and J. Lu, Int. J. Extreme. Manuf., 2023, 5, 032007 Search PubMed.
- J. Feng, J. Fu, X. Yao and Y. He, Int. J. Extreme. Manuf., 2022, 4, 022001 Search PubMed.
- Z. Meng, X. Mu, J. He, J. Zhang, R. Ling and D. Li, Int. J. Extreme. Manuf., 2023, 5, 025001 Search PubMed.
- F. Qi, W. Liu, Y. Chen, F. Ding, W. Li, S. Peng, P. He and C. Shuai, J. Colloid Interface Sci., 2025, 682, 210–221 Search PubMed.
- X. Wu, J. Liu, Y. Yang, J. Bai, C. Shuai, J. Buhagiar and X. Ning, Int. J. Extreme. Manuf., 2025, 7, 022007 Search PubMed.
- P. Feng, R. He, F. Yang, Y. Lin, L. Fan, H. Pan and C. Shuai, Sustainable Mater. Technol., 2025, 43, e01205 Search PubMed.
- X. Gao, W. Deng, J. Tan, X. Shuai, J. Zan, T. Ye, K. Luo, F. Qi, Y. Wei and C. Shuai, Mater. Today Chem., 2025, 43, 102455 Search PubMed.
- P. Feng, L. Liu, F. Yang, R. Min, P. Wu and C. Shuai, Biofabrication, 2025, 17, 012005 Search PubMed.
- I. Ullah, P. Ou, L. Xie, Q. Liao, F. Zhao, A. Gao, X. Ren, Y. Li, G. Wang, Z. Wu, P. K. Chu, H. Wang and L. Tong, Acta Biomater., 2024, 175, 382–394 Search PubMed.
- Y. Si, H. Liu, H. Yu, X. Jiang and D. Sun, Surf. Coat. Technol., 2022, 431, 128008 Search PubMed.
- M. Wang, J. Yao, S. Shen, C. Heng, Y. Zhang, T. Yang and X. Zheng, Nano Res., 2023, 16, 757–770 Search PubMed.
- H.-T. Lu, G.-Y. Huang, W.-J. Chang, T.-W. Lu, T.-W. Huang, M.-H. Ho and F.-L. Mi, Carbohydr. Polym., 2022, 281, 119035 Search PubMed.
- W. Chen, D. Gu, J. Yang, Q. Yang, J. Chen and X. Shen, Int. J. Extreme. Manuf., 2022, 4, 045002 Search PubMed.
- W. Ren, J. Xu, Z. Lian, X. Sun, Z. Xu and H. Yu, Int. J. Extreme. Manuf., 2022, 4, 015101 Search PubMed.
- C. Shao, H. Li, Y. Zhu, P. Li, H. Yu, Z. Zhang, H. Gleiter, A. McDonald and J. Hogan, Int. J. Extreme. Manuf., 2023, 5, 015102 Search PubMed.
- S. Sui, Y. Chew, F. Weng, C. Tan, Z. Du and G. Bi, Int. J. Extreme. Manuf., 2022, 4, 035102 Search PubMed.
- Q. Sun, Z. Xue, Y. Chen, R. Xia, J. Wang, S. Xu, J. Zhang and Y. Yue, Int. J. Extreme. Manuf., 2022, 4, 015001 Search PubMed.
- C. Wei, H. Gu, Y. Gu, L. Liu, Y. Huang, D. Cheng, Z. Li and L. Li, Int. J. Extreme. Manuf., 2022, 4, 025002 Search PubMed.
- W. Zhang, W. Hou, L. Deike and C. Arnold, Int. J. Extreme. Manuf., 2022, 4, 015201 Search PubMed.
- H. N. Pantaroto, A. P. Ricomini-Filho, M. M. Bertolini, J. H. D. da Silva, N. F. A. Neto, C. Sukotjo, E. C. Rangel and V. A. Barão, Dent. Mater., 2018, 34, e182–e195 Search PubMed.
- Y. Ma, Y.-Z. Guo, Y. Liu, Y.-R. Wang, J.-C. Yang, X.-Z. Kong, H.-L. Jia, R.-Z. Li, Q.-Z. Han, C.-D. Zheng, X.-J. Hu and B. Liu, Front. Mater., 2022, 9, 883027 Search PubMed.
- B. Chen, W. Wang, M. Hu, Y. Liang, N. Wang, C. Li and Y. Li, ACS Nano, 2024, 18, 24968–24983 Search PubMed.
- T. R. Kyriakides, A. Raj, T. H. Tseng, H. Xiao, R. Nguyen, F. S. Mohammed, S. Halder, M. Xu, M. J. Wu, S. Bao and W. C. Sheu, Biomed. Mater., 2021, 16, 042005 Search PubMed.
- U. Theuretzbacher, K. Outterson, A. Engel and A. Karlén, Nat. Rev. Microbiol., 2020, 18, 275–285 Search PubMed.
- L. Mei, S. Zhu, Y. Liu, W. Yin, Z. Gu and Y. Zhao, Chem. Eng. J., 2021, 418, 129431 Search PubMed.
- F. J. Álvarez-Martínez, E. Barrajón-Catalán, M. Herranz-López and V. Micol, Phytomedicine, 2021, 90, 153626 Search PubMed.
Footnote |
† These authors contributed equally to this work. |
|
This journal is © The Royal Society of Chemistry 2025 |
Click here to see how this site uses Cookies. View our privacy policy here.