DOI:
10.1039/D4NR04515G
(Review Article)
Nanoscale, 2025,
17, 1874-1888
Nanomaterial-based synergistic strategies for combating dental caries: progress and perspectives
Received
30th October 2024
, Accepted 6th December 2024
First published on 6th December 2024
Abstract
Dental caries, as the predominant global oral disease, remains a critical public health issue worldwide, particularly in socioeconomically disadvantaged communities. However, common caries prevention approaches (e.g., oral health education, mechanical plaque removal, and delivery of fluoride agents) are still insufficient for optimal caries management, and therefore, alternative regimens that can supplement existing strategies are highly warranted. Nanomaterials exhibit considerable potential in combating cariogenic pathogens and biofilms owing to their promising antimicrobial capacity, improved penetration into biofilms, targeted precision delivery, and versatile physicochemical properties. As unifunctional materials are limited in caries management, this review underscores the latest advancement in multifunctional anti-caries nanomaterials/nanomedicines. It highlights the cutting-edge materials developed or engineered to (i) incorporate diagnostic capabilities to prevent caries at an early stage, thus enhancing treatment efficiency, (ii) integrate mechanical “brushing” with anti-caries approaches to mechanochemically eradicate biofilms, (iii) exert antimicrobial/antibiofilm effects while preserving dental hard tissue. The current work also outlines future directions for optimizing nanosystems in the management of dental caries while emphasizing the need for innovative solutions to improve preventive and therapeutic efficacies.
1 Introduction
Dental caries, the most prevalent oral disease worldwide, continues to be a major public health concern, especially causing severe impacts among the socioeconomically disadvantaged populations with limited oral health knowledge.1,2 Importantly, secondary caries can recur following initial treatment, leading to restorative treatment failure and imposing a significant burden on the dental care workforce.3,4 In general, the pathogenesis of this multifactorial disease can be primarily attributed to three interdependent aspects: (i) loss of symbiosis within the oral microenvironment, (ii) domination of cariogenic pathogens and (iii) formation of pathogenic polymicrobial biofilms, which eventually drive the chronic and progressive destruction of dental hard tissues. Specifically, external cariogenic factors, such as excessive intake of sugars and carbonated drinks, may disrupt the ecological balance, shifting the healthy oral microbiota to a pro-cariogenic state.5 During this transition, the assembly of the matrix and dysbiotic microbiota–matrix interactions aggravate cariogenic plaque accumulation. Notably, excessive fermentable carbohydrates, especially sucrose, are metabolized to generate acid by-products, creating a low pH environment, which further facilitates the colonization and growth of acidogenic and aciduric species (e.g., Streptococcus mutans (S. mutans), Lactobacillus, Actinomyces, and Candida albicans).6–8 Consequently, these species dominate over commensals within the biofilm microbiota and synergistically shape the biofilm community.8,9 Additionally, the biofilm matrix, primarily composed of extracellular polymeric substances (EPSs) in a three-dimensional scaffold, provides a complex biochemical microenvironment that confers penetration barriers and mechanical resistance to support cariogenic microbes in caries pathogenesis.10,11 Simultaneously, acid production on the biofilm-enamel surface causes demineralization and erosion of the enamel or dentine, ultimately resulting in cavitation.12 Therefore, although considerable attention and efforts have been invested in effective management, the advancement of early diagnosis, prevention and treatment is highly warranted with the primary aims targeting cariogenic polymicrobial biofilms, preventing demineralization and/or promoting remineralization of hard dental tissues.
Currently, caries prevention strategies include implementing oral health education programs, applying fluoride treatment, and removing dental plaque mechanically. However, these approaches remain insufficient in managing dental caries. Although fluoride reduces caries through its antimicrobial activity and protects the tooth enamel by enhancing its remineralization and inhibiting its demineralization,13 the emergence of fluoride-resistant strains of bacteria has diminished its caries-preventive effect over time.14 Meanwhile, toothbrushing is the most effective method to remove plaque,15 but it does not prevent microbial recolonization, and the modern diet with refined carbohydrates poses a challenge in controlling the consumption of fermentable sugars. Additionally, the use of composite fillings as the main treatment for caries continues to face limitations from secondary caries.16 Therefore, there is a pressing need to enhance and supplement existing caries control strategies for improved efficacy.
Nanomaterials, either inorganically or organically synthesized, are defined as materials with sizes less than 100 nm,17 which can be categorized based on their dimensions into zero-dimensional, one-dimensional, and two-dimensional forms, represented by nanoparticles (NPs), nanowires/nanorods, and nanofilms, respectively.18 Particularly, many of them exhibit unique properties that are inherently linked to their nanoscale. A key characteristic of nanomaterials is their high surface area-to-volume ratio, which imparts high reactivity and enables them to serve diverse functional purposes. In combating dental caries, nanomaterials exhibit considerable potential in targeting cariogenic pathogens and biofilms, as well as preventing demineralization and facilitating remineralization processes, owing to their antimicrobial properties, improved penetration into biofilms, targeted delivery, and versatile applications.19–22
Recognizing the limitations of materials with single function in caries management, this review focuses on the development of multi-functional anti-caries nanomaterials. The nanotechnology-based platforms conferring anti-caries properties for dental restorative materials have been reviewed elsewhere.23–25 Mainly, we focus on advanced nanomaterials that are designed to (i) incorporate diagnostic capabilities, thereby offering a comprehensive strategy to enhance the caries prevention effectiveness, (ii) combine mechanical “brushing” with anti-caries approaches for synergistic mechanochemical biofilm eradication, (iii) integrate antimicrobial/antibiofilm effects with preservation of dental hard tissue (Scheme 1).
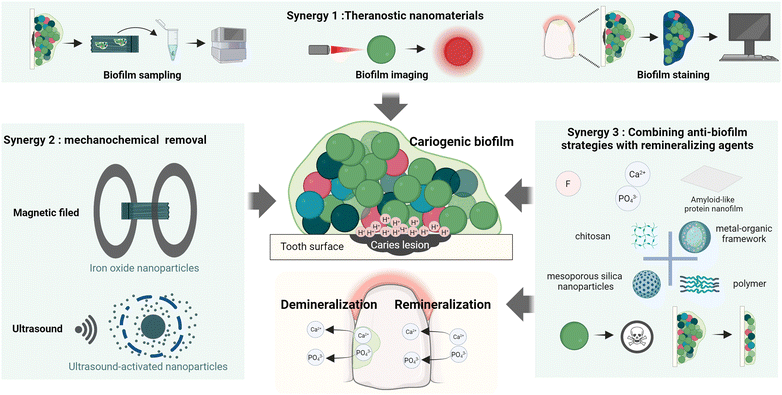 |
| Scheme 1 Illustrative scheme of three nanomaterial-based synergistic strategies utilized as novel caries management approaches for biofilm disruption, mineralization promotion or pathogen detection. | |
2 Theranostic nanomaterials for caries treatment
In addition to targeting cariogenic biofilms and promoting the mineralization process, detecting cariogenic pathogens can facilitate the effective management of dental caries. Specifically, detecting microbes enable early caries prevention, avoiding the progressive and irreversible destruction of dental hard tissues. Taken together, by combining therapeutic and diagnostic approaches (known as theranostics), the efficiency of caries management may be significantly improved through timely intervention to prevent the further progression of decay, as well as establishing personalized treatment/prevention to maximize the therapeutic outcomes. Therefore, the development of innovative methods incorporating these strategies is highly crucial for providing a comprehensive and patient-centered oral healthcare.
Various nanomaterials can conveniently serve as a crucial part of theranostic systems due to their remarkable versatility.26 When combined with materials possessing known antibacterial/anti-biofilm properties, multifunctional nanoplatforms capable of biofilm sampling and imaging/staining can be achieved by utilizing multiple pathways. In addition to utilizing nanomaterials for biofilm removal, innovative approaches with novel designs, such as the above-mentioned nanorobots, have been applied for automated biofilm sampling. Furthermore, advancements in imaging and staining techniques for microbial cells and their biofilm formation have explored the use of photodynamic therapy (PDT)/photothermal therapy (PTT) and nanozymes that generate reactive oxygen species (ROS) for antibacterial and antibiofilm effects. These advancements aid in assessing the microbial conditions, facilitating the identification of caries-infected areas for timely preventive or early treatment interventions.
2.1 Magnetic robot-assisted biofilm sampling
Biofilm/saliva sampling and characterization are essential for understanding the microbial profile and risk/progression of dental caries. Generally, this process requires swabbing biofilms or collecting saliva from the oral cavity, followed by detailed microbial or microbiota analysis, which may enable early prediction, and then intervention of caries. Given that most of the current biofilm sampling approaches require direct manual operation in the oral cavity, they have difficulties or certain chances to cause errors in taking samples from inaccessible areas or non-compliant patients such as children. Hence, the application of nanomaterials may streamline biofilm sampling and retrieval in hard-to-reach areas through straightforward yet reliable and efficient procedures, particularly beneficial for pediatric sampling.
For instance, the magnetic robot surface topography-adaptive robotic superstructures (STARS) could function for biofilm sampling by collecting and retrieving sufficient quantities of biofilm components using its extended bristle structure to mechanically sweep the biofilm with the assistance of a magnetic field generated by its electromagnet core (Fig. 1A). Subsequently, the microorganisms in the sampled biofilm could be analyzed by manifold diagnosis techniques, such as quantitative polymerase chain reaction and quantitative measurement of exoenzyme activity (Fig. 1B).27 Meanwhile, the on-site visualization of biofilms is equally important in clinical examination for theranostic purposes, thereby user-friendly and cutting-edge techniques for biofilm imaging and staining have also been developed and implemented for managing dental caries effectively.
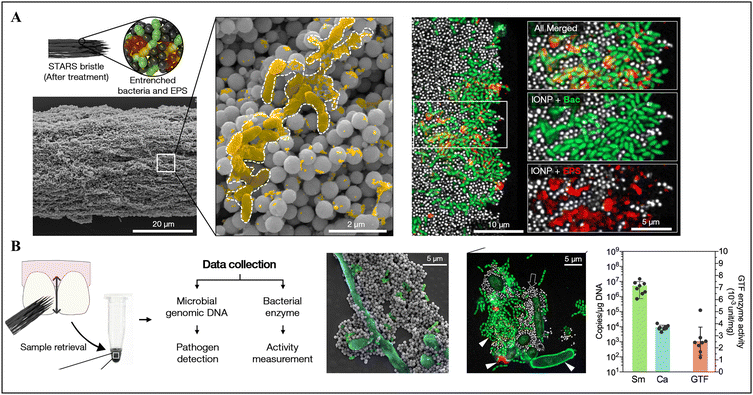 |
| Fig. 1 Magnetic robot-assisted biofilm sampling. (A) Demonstration of STARS bristles and entrenched biofilm components. (B) Illustration of the collection workflow using STARS and further analysis. Reproduced with permission from ref. 27. Copyright 2022, the American Chemical Society. | |
2.2 Nanomaterials with photoacoustic properties or aggregation-induced emission for biofilm imaging
Light-based therapies, such as PDT and PTT, have emerged as non-invasive treatment approaches that rely on producing ROS or heat, instead of antibiotics, to exert antibacterial effects. Recently, they have been synergistically utilized in diagnostics, leveraging the potential photoacoustic (PA) properties or aggregation-induced emission (AIE) for biofilm imaging. Typically, PA imaging combines optical illumination and ultrasound detection via the incorporation of special molecules for non-invasive monitoring. These special molecules absorb energy from a pulsed laser and convert it into heat, causing thermoelastic expansion and generating ultrasound waves, which are later converted into high-resolution images through a transducer system.28 Gold nanocages, as a photosensitizer, have shown strong absorption in the near-infrared (NIR) light region, triggering both PTT and PA imaging.29 Hajfathalian and colleagues reported that gold-in-gold cage photothermal nanoparticles (PTNP) coated with dextran could selectively target S. mutans biofilms (Fig. 2A). Specifically, under NIR irradiation, PTNP exhibited photothermal properties, rapidly killing S. mutans in the biofilms and generating strong contrast PA images for biofilm detection (Fig. 2B).30
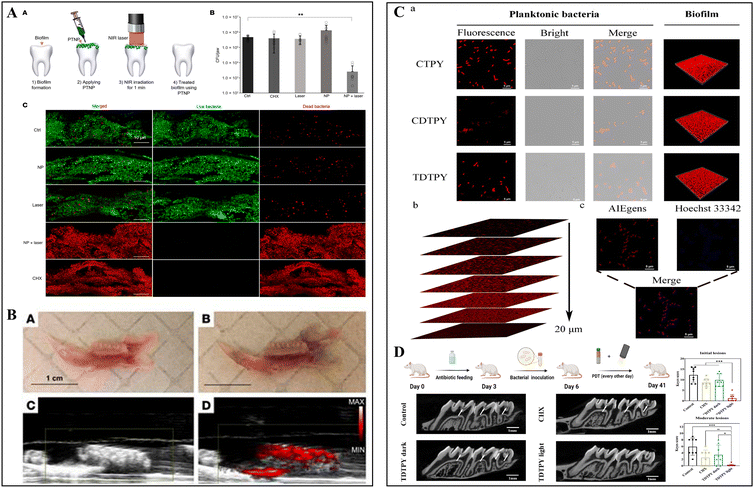 |
| Fig. 2 Biofilm imaging using nanomaterials with photoacoustic properties or aggregation-induced emission. (A) Evaluation of bacterial killing effects of PTNP on S. mutans using PTT. (B) Representative PA images of teeth with stronger signals after PTNP treatment. Reused from ref. 30. (C) Images of planktonic and biofilms of S. mutans stained by synthesized AIEgens, 4-(7-(2-(1-methyl-1l4-pyridin-4-yl)vinyl)-2,3-dihydrothieno[3,4-b][1,4]dioxin-5-yl)-N,N-diphenylaniline (TDTPY). (D) Assessment of anti-caries effects of TDTPY using rodent caries models. Reproduced with permission from ref. 34. Copyright 2024, the American Chemical Society. | |
Moreover, the discovery of AIE31 has overcome the limitations of traditional fluorescence quenching, showing extensive potential in disease diagnosis and treatment.32 Aggregation-induced emission luminogens (AIEgens) can emit strong fluorescence in the aggregated state but not in the dispersed state.31 Previously, photosensitizers with AIE characteristics have been used in antimicrobial PDT to significantly enhance ROS generation.33 Pan's group designed a type of cationic AIEgen to interact with negatively charged bacterial cell surfaces via electrostatic binding to selectively visualize S. mutans, while exerting anti-caries capability mediated by PDT (Fig. 2C and D).34 Cationic pyridinium-substituted photosensitizers with AIE property possessed luminous fluorescence for biofilm visualization and efficient singlet oxygen generation ability for biofilm reduction under light irradiation. Taken together, light-activated biofilm imaging and disruption via PA or AIE effects offer appealing prospects for implementing theranostic strategies for managing caries in a non-invasive and visually intuitive manner.
2.3 Nanozyme-induced colorimetric responses for detecting cariogenic bacteria/biofilms
Biofilm staining can be achieved through colorimetric methods mediated by pathogen interactions or pH changes. Notably, certain nanozymes possess peroxidase/oxidase-like activity, catalyzing hydrogen peroxide (H2O2) to generate ROS for antibacterial/anti-biofilm purposes,35–38 while these ROS can react with specific substrates to produce a discernible colorimetric readout. One typical substrate used in this type of reaction is 3,3′,5,5′-tetramethylbenzidine (TMB), a widely employed chromogenic reagent in immunoassays. Upon exposure to ROS, the amine groups in TMB can be oxidized, shifting its color from colorless to blue.39 Consequentially, the production of the blue-colored product can be quantified by measuring the absorbance at 625 nm. Hence, nanozymes can catalyze antibacterial/antibiofilm treatments and colorimetric staining of biofilms, making them serve as potent theranostic reagents for differentiating and eliminating cariogenic bacterial cells/biofilms.
Recently, the FDA-approved iron oxide nanoparticle formulation FerIONP was shown to function as a nanocatalyst, which preferentially bound to S. mutans via pathogen-specific glucan-binding proteins, catalyzed the in situ generation of ROS for bactericidal effects, and resulted in significant blue staining on tooth surfaces in the presence of S. mutans biofilms when applied with TMB (Fig. 3A).40 As a result, FerIONP shows significant promise in theranostic treatments by producing ROS from H2O2 to selectively eliminate and stain S. mutans biofilms, with minimal impact on other commensal organisms, demonstrating potential clinical utility as a targeted anti-caries nanomedicine. Additionally, high-throughput detection via sensor arrays, assisted by machine learning techniques such as linear discriminant analysis (LDA) and hierarchical clustering analysis (HCA), offer a more advanced approach for overcoming the limitations of the “one-to-one” recognition pattern using nonspecific recognition elements, allowing more comprehensive bacterial discrimination in polymicrobial biofilms. Previously, Zhang and colleagues developed “electronic tongues” inspired by the natural gustatory system (Fig. 3B).41 In this system, programmable DNA-encoded INOPs provided catalysis-dependent ROS production for bacterial killing and notably functioned as sensing elements, in which their peroxidase-like activities were determined by the differential interactions between the particles and different types of bacteria. Followed by TMB oxidation, the corresponding responses from different cariogenic bacteria were analyzed and converted into visualized graphics using LDA and HCA. Similarly, single-atom nanozymes (SANs) exhibited excellent performances in identifying mixed cariogenic bacteria in artificial saliva samples with the assistance of machine learning (Fig. 3C).42 Particularly, different cariogenic bacteria could increase the oxidase-like activity of iron (Fe)–nitrogen (N)–carbon (C) SANs and reduced the activity of Fe–N–C SANs reconstructed with urea to various levels, resulting in varying colorimetric responses correlated as unique "fingerprints". Therefore, a colorimetric sensor array was developed based on the "fingerprint" examination and the results were analyzed and distinguished by machine learning, enabling the cost-effective, precise, and high throughput differentiation and identification of cariogenic bacteria.
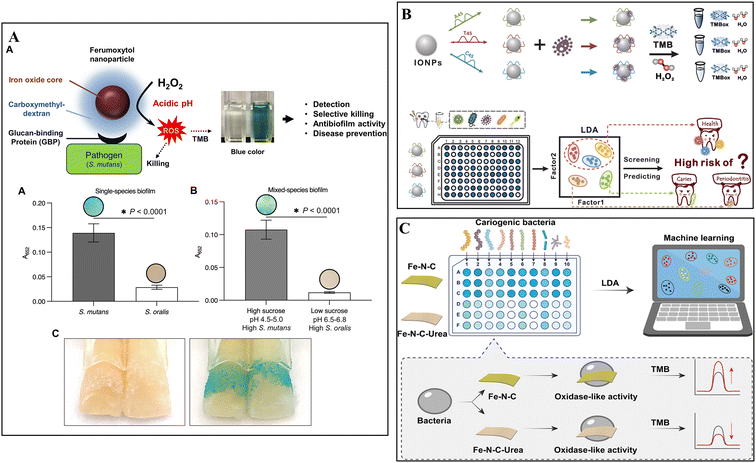 |
| Fig. 3 Biofilm staining using nanozyme-induced colorimetric responses. (A) Illustration of theranostic mechanisms of FerINOPs and results of the higher degree of blue-stained S. mutans biofilms on natural teeth. Reproduced with permission from ref. 40. Copyright 2022, the American Chemical Society. (B) Schematic depiction of precise identification of dental bacteria and prediction of caries risk via TMB oxidation by DNA-coated peroxidase-like nanozyme (INOPs). Reprinted with permission from ref. 41. Copyright 2024, the American Chemical Society. (C) Schematic of machine learning-assisted cariogenic bacterial detection through colorimetric sensor arrays based on different TMB oxidation levels caused by oxidase-like activity of SANs. Reprinted with permission from ref. 42. Copyright 2023, Wiley-VCH. | |
3 Synergistic strategies of nanomaterials for mechanochemical biofilm removal
Mechanical removal of dental plaque by toothbrushing and flossing is the most common and effective strategy in oral health care, owing to its simplicity, accessibility and cost-effectiveness. Following this approach, novel designs have been introduced to utilize specific nanomaterials for mechanochemical biofilm clearance by actively generating mechanical force and integrating antibacterial/antibiofilm agents. Currently, there are two main materials being employed, micro/nano-robots and ultrasonic irradiation-based mechanochemical nanomaterials, demonstrating great potential for biofilm clearance through multiple mechanisms, which makes them ideal candidates for cariogenic biofilm elimination.
3.1 Magnetic nanoparticle-based multifunctional robots
Robots on the micro- and nanoscale are capable of operating in confined space and delivering drugs on-site, attracting considerable attention for biofilm treatment recently. They can be automatically propelled by the involvement of various sources, either intrinsically, such as catalyst and biological cells, or extrinsically, such as light and magnetic fields.43,44 These sources can work alone or collectively, creating oscillating motions by generating localized forces to mechanically clear out biofilms. Generally, magnetic materials, such as iron oxide nanoparticles (IONPs) and liquid metal droplets,45 actuated by a magnetic field, can generate mechanical forces that facilitate the penetration and disruption of biofilms. This process is further enhanced by the employment of free radicals or active drugs, synergistically demonstrating a remarkable performance in cariogenic biofilm disruption.46–48 Previously, catalytic antimicrobial robots (CARs), based on magnetic IONPs, 1% H2O2 and EPS-degrading enzymes, were first developed by Hwang et al. for biofilm control to combat dental caries. This “kill-degrade-and-remove” robot system with both catalytic and antimicrobial capacities enabled the precise, efficient, and controllable treatment of S. mutans biofilms.49 In particular, INOPs can act as a nanocatalyst with intrinsic peroxidase-like activity, catalyzing H2O2 to generate free radicals.35 EPS-degrading enzymes, mutanase and dextranase can work together to effectively break down the EPS structure,50 facilitating bacterial killing within mature cariogenic biofilms. The magnetic INOPs assembled with biodegraded products, namely biohybrid CARs, could sweep across defined geometrical surfaces under magnetic control to prevent the recolonization of pathogens, thereby completely inhibiting biofilm formation.49
In addition to eradicating biofilms, magnetic robots are also capable of handling the complex topographies of human teeth with irregular surfaces and positions. For example, Oh and co-workers synthesized reconfigurable STARS using a magnetic field-directed assembly of IONPs. Specifically, the dynamic telescoping bristle-like structures of STARS were controlled by a magnetic field coordinated by a programmable microcontroller to achieve multi-axis motion. These structures demonstrated multifunctional potential for eradicating cariogenic biofilms through “toothbrushing-like” and “flossing-like” behaviors and in situ catalysis-mediated antibacterial activity, allowing site-specific diagnosis and microbial sample retrieval (Fig. 4A).27 Taken together, these studies demonstrate that self-supported magnetic robots using INOPs, in combination with enzymes, show great potential by generating mechanochemical effects against cariogenic biofilms.
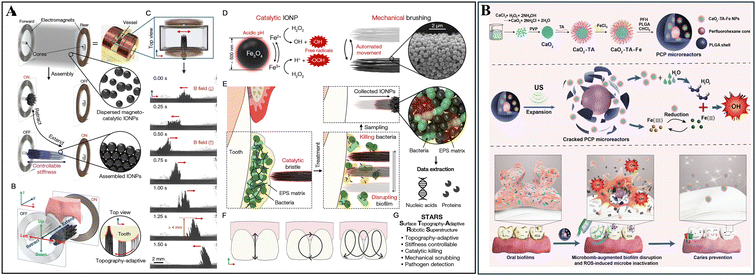 |
| Fig. 4 Magnetic nanoparticle-based multifunctional robots for mechanochemical biofilm removal. (A) Schematic of the development of STARS with topography-adaptive and stiffness-controllable bristles for catalytic killing, mechanical scrubbing and pathogen sampling. Reused with permission from ref. 27. Copyright 2022, the American Chemical Society. (B) Schematic demonstrating the procedures for the synthesis/fabrication of the microreactors, US-induced cascade reactions for augmented ROS generation, and topical application of the microreactors for biofilm disruption with ultrasonic toothbrush irradiation. Reprinted with permission from ref. 58. Copyright 2023, Wiley-VCH GmbH. | |
3.2 Ultrasound-activated nano/micro materials
Sonochemistry, a field that investigates ultrasound (US)-induced physical and chemical effects for multiple applications, has been widely used in biomedicine, food industry, materials science, and environmental remediation.51 The implementation of ultrasound governs the collapse of microbubbles to induce acoustic cavitation in most liquid media, making it a novel strategy for the mechanochemical disruption for biofilms.52,53 Therefore, the incorporation of US irradiation through ultrasonic toothbrushes has become an increasingly attractive approach for the management of dental caries. Recently, an ultrasound-activated phase-shift chemical, perfluorohexane (PFH), has been introduced. As a droplet with a very low boiling point, PFH can easily vaporize upon overheating54 and be converted into nano-emulsions for biofilm eradication.55–57 For instance, Guo and colleagues integrated the ultrasonic effect with ROS to develop a microreactor by utilizing PFH encapsulated with iron-tannin-modified calcium peroxide (CaO2TA-Fe) within poly(lactide-co-glycolide) (PLGA) vesicles (Fig. 4B).58 The rapid vaporization of PFH under the ultrasonic toothbrush induced the immediate rupture of the PLGA shell, leading to direct microbubble-assisted mechanical damage on dental biofilms and triggering an ROS-producing cascade. In turn, this process enabled the self-supply of H2O2 from CaO2, accelerating Fe(III)/Fe(II) conversion in the TA-Fe network and enhancing ROS generation and delivery to the impaired biofilm for effective eradication.58 This strategy provides a promising application for US-activated nanomaterials in the efficient treatment of dental caries. However, further development of more precise and efficient US-activated nanoplatform is still required, and the use of low-intensity, safe frequency ranges of US (such as 1.6 MHz used in US toothbrushes approved by the FDA) should be encouraged.
4 Combined anti-biofilm strategies with molecules regulating the mineralization process
Dental hard tissues, generated from the biomineralization process, possess intricate crystal structures developed through nucleation, growth, and assembly by the selective deposition of mineral ions at specific sites induced and regulated by the organic matrix.59 Predominantly composed of inorganic hydroxyapatite (Ca10(PO4)6(OH)2), these hard tissues also contain small amounts of organic components, primarily proteins. Typically, the demineralization/remineralization process of enamel and dentin has been widely investigated. In the physiological state, demineralization and remineralization are in a delicate balance, which can be disrupted by cariogenic factors, leading to the accumulation of acidic byproducts and decrease in pH value. When the pH falls below 5.5, the dissolution of Ca2+, OH− and PO43− ions from hard tissues can be accelerated, while these ions are unable to deposit into hydroxyapatite for self-repair. A mild imbalance can lead to defects, such as white spots on the enamel and dentin hypersensitivity. Prolonged mineral loss due to continued acid production by cariogenic biofilms can result in the formation of caries cavities. Therefore, an effective clinical caries management strategy involves promoting the remineralization and inhibiting the demineralization of dental hard tissues, while eliminating cariogenic biofilms in a synergistic manner.
Currently, the clinically approved remineralization agents include fluoride, casein phosphopeptide-amorphous calcium phosphate (CPP-ACP), hydroxyapatite (HAP), bioactive glass, and self-assembling peptide P-114.60 Meanwhile, the biomimetic mineralization strategy based on the enamel crystallization process has been extensively investigated, aiming to regenerate the highly ordered HAP crystal microstructure.61 However, the hard tissues generated from these approaches lack the capacity to inhibit cariogenic pathogens and biofilms. Fortunately, certain nanomaterials possess excellent antimicrobial/anti-biofilm abilities due to their inherent physiochemical properties and loading capacity, and their incorporation with agents for biomimetic mineralization can act as an attractive approach in dental caries treatment. Thus, the focus of this section is on synergistic design strategies, such as modifying existing remineralization agents to form novel nanomaterials with dual functions of antimicrobial/anti-biofilm and remineralization or exploring nanoparticles with dual properties.
4.1 Fluoride-loaded nanoparticles with dual functions
For decades, fluoride has been widely used as an effective agent for preventing caries.62 It can react with HAP to form a more acid-resistant fluorapatite, thereby preventing mineral loss caused by acid-induced demineralization. It is important to address appropriate fluoride use and focus on strategies for improving the effectiveness of fluoride therapy and reducing the required amount of fluoride.63 Therefore, extensive studies on nano-based fluoride delivery systems aim to develop more efficient approaches for protecting and repairing dental hard tissues by continuous and controlled release of fluoride agents.
Among the different fluoride-containing nanomaterials, nano-sized calcium fluoride (nano-CaF2) and nano silver fluoride (NSF) have been reported to exhibit multiple functions in managing dental caries from different perspectives. Nano-CaF2 shows higher fluoride deposition compared to NaF, serving as a fluoride reservoir.64 It also demonstrates antibiofilm effects by inhibiting the virulence of S. mutans biofilms without affecting the bacterial viability, making it a potential anti-caries agent.65 Alternatively, silver diamine fluoride has been proven to be effective for caries prevention and treatment;66 however, its drawbacks include tooth discoloration and ulceration, limiting its widespread use. Thus, to address these disadvantages, NSF was synthesized by reducing silver nitrate using sodium borohydride and stabilizing the obtained nanoparticles by chitosan and incorporating NaF.67 Pushpalatha et al. reviewed the anti-caries effects of NSF, highlighting the cumulative benefits of chitosan, silver nanoparticles and sodium fluoride. These benefits include reducing demineralization, accelerating remineralization, interfering with biofilm formation, and inhibiting bacterial growth and metabolism.68 Given that NSF is commonly available in colloidal solutions and varnish forms, further clinical studies with large sample sizes are necessary to investigate and validate the effectiveness of specific concentrations of NSF in combating dental caries through antimicrobial and mineralizing functions based on its economical, safe, non-invasive, and non-discoloring properties.
In recent years, researchers have explored various approaches for developing other fluoride-loaded nanoparticles.69–71 One strategy involves loading NaF into diverse nanoplatforms to achieve synergistic functions. For example, NaF was co-loaded with antimicrobial molecules such as tannic, into a pH-responsive spherical polymeric micelles (3-maleimidopropionic acid-poly(ethylene glycol)-block-poly(L-lysine)/phenylboronic acid) with core-shell structures and conjugation of salivary-acquired peptide. This design endowed the polymeric micelles with essential features, such as intelligent release, cariogenic biofilm resistance and adherence of tooth surfaces.72 However, concerns have been raised regarding fluoride-loaded nanoparticles, including issues of insufficient loading and unpredictable release, which may lead to inappropriate and excessive fluoride usage. Thus, to address these concerns, Huang and colleagues found that stannous fluoride (SnF2) could be stabilized by an FDA-approved iron oxide nanoparticle formulation named ferumoxytol (FerIONP). The binding between Sn and carboxylate groups of FerIONP could enhance its catalytic activity, and simultaneously improve the anticaries effects of SnF2. This synergy resulted in the development of the Fer/SnF2 nanocomplex, which, despite containing lower fluoride concentration, demonstrated significant anti-caries effects by controlling biofilm formation through the catalytic activity of Fer and utilizing the novel Fe/Sn/F-rich film formed on the tooth surface to prevent demineralization and promote remineralization.73 Thus, nanomaterials with stable fluoride delivery and antibacterial/anti-biofilm functionality have the potential to provide superior anti-caries effects with low fluoride concentrations, holding promising prospects for effective caries management in the future.
4.2 Nanoparticles for delivering calcium and phosphate
The supplementation of Ca2+ and PO43− on the tooth surface is the basis for promoting remineralization. Owing to the versatile properties of nanoparticles, such as efficient encapsulation of effector molecules and controlled and/or sustainable releasing of payloads, they have been extensively studied and developed to integrate calcium phosphate for remineralization. The typical nanocarriers used in this field include organic nanoparticles, such as chitosan and polymer nanoparticles, as well as inorganic nanoparticles, such as mesoporous silica nanomaterials. Importantly, these nanocarriers, either possessing inherent antimicrobial properties or loaded with additional antimicrobial agents, can demonstrate antimicrobial/anti-biofilm effects and promote remineralization via encapsulated Ca2+ and PO43− ions to treat dental caries in a synergistic manner.
4.2.1 Amorphous calcium phosphate-based nanocomplexes.
Transient amorphous calcium phosphate (ACP) has been identified in newly formed enamel minerals as the precursor of HAP crystals, with its orientation guided by an organic framework during the enamel mineralization process.74,75 Specifically, ACP agents are capable of releasing high levels of Ca2+ and PO43− for the remineralization process, showing potential for preventing and treating enamel demineralization and obstructing dentin tubules.76,77 However, ACP exhibits limited stabilities in preserving its temporary form in aqueous solution, while lacking antimicrobial abilities, significantly reducing their applicability for further use. Meanwhile, the sole ACP application often overlooks the presence of cariogenic biofilms in caries-affected areas, diminishing its remineralization ability and impairing treatment outcomes. Therefore, dual/multi-functional ACP nanocomposites/nanosystems have been mainly developed by (i) stabilizing ACP with molecules possessing antibacterial properties into nanostructures or (ii) loading/incorporating ACP with functional molecules into existing nanomaterials with or without antimicrobial capacity to achieve both remineralization and antimicrobial/biofilm inhibition.
A variety of biomacromolecules and polymers (e.g., amelogenin and its analogs,78,79 casein phosphopeptides,80 polyelectrolyte,81 and amyloid-like protein82) has been developed based on the biomineralization process of dental hard tissues to stabilize ACP and prevent its transformation into the final apatite crystalline mineral through surface adsorption or ion substitution. Notably, several molecules, such as carboxymethyl chitosan (CMC), zwitterionic poly(carboxybetaine acrylamide) (PCBAA) and epigallocatechin gallate (EGCG), are capable of conferring antimicrobial and antibiofilm properties to ACP-based nanocomplexes, while simultaneously stabilizing ACP state. Among them, CMC is a derivative of chitosan with improved solubility by introducing carboxymethyl groups in its structure. Yao et al. demonstrated that CMC-based materials, such as CMC-containing dental adhesive, possessed anti-adhesion properties against S. mutans.83 Meanwhile, the carboxyl groups in the CMC structure could sequester Ca2+ and stabilize ACP by forming nanocomplexes, contributing to the enamel remineralization and dentin tubule occlusion.84–86 Furthermore, the nanocomplexes could inhibit the adhesion of cariogenic pathogen and formation of biofilms on enamel without bactericidal activity, indicating an oral microbiota-friendly approach.87 Likewise, zwitterionic carboxybetaine polymers such as PCBAA offer excellent biocompatibility and antifouling properties, inhibiting bacterial adhesion and biofilm formation of S.mutans.88,89 In particular, their positively charged quaternary ammonium groups can both suppress the growth of the bacteria and adsorb negatively charged ions such as PO43−, while the structural carboxyl groups can bind with Ca2+via electrostatic attraction. Therefore, the PCBAA/ACP nanocomposites with superior stability could effectively prevent S. mutans adhesion, eliminate the bacteria under acidic conditions, and enhance enamel remineralization and dentinal tubule occlusion (Fig. 5A).90 Meanwhile, similar properties can also be observed in the multi-functional natural polyphenolic substance EGCG. Studies have shown that EGCG treatment can reduce planktonic growth and the biofilm virulence of S. mutans, including acidogenesis and EPS production.91–93 Specifically, the phenolic hydroxyl groups of EGCG could interact with Ca2+ and form an EGCG-stabilized ACP nanocomposite, which possessed pH-responsive release capacity, while exhibiting both antibacterial and remineralization effects (Fig. 5B).94 In brief, the direct formation of molecule-ACP nanocomposites offers a concise and bifunctionally effective strategy to combat cariogenic conditions.
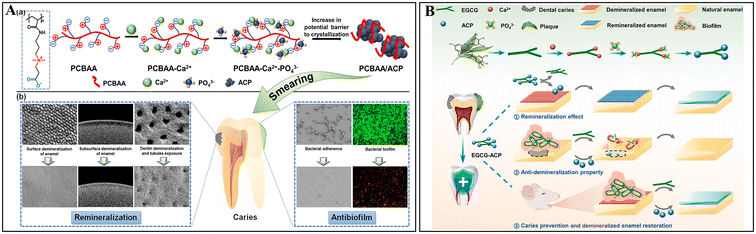 |
| Fig. 5 ACP-based nanocomplexes using antibacterial stabilizers. (A) Graphic abstract showing that PCBAA can interact with Ca2+ and PO43− to stabilize ACP and provide both remineralization and anti-biofilm properties. Reused from ref. 90. Copyright 2022, American Chemical Society. (B) Schematic of EGCG-ACP synthesis through interaction between phenolic hydroxyl groups of EGCG and Ca2+, and anti-caries effects of EGCG-ACP. Reprinted with permission from ref. 94. Copyright 2023, Elsevier. | |
Besides using antimicrobial molecules to directly stabilize ACP and forming nanocomplexes with antimicrobial/antibiofilm properties, ACP-based nanomaterials can also be conveniently incorporated with antimicrobial agents to further enhance their overall effects, offering efficient methods for enamel remineralization and dentin tubule occlusion in the treatment of polymicrobial cariogenic biofilms. Inspired by the nanogel formed via electrostatic interactions between CMC and lysozyme,86 a chimeric lysin ClyR with lytic activity against S. mutans biofilms95 together with ACP was loaded into CMC-formed nanocarriers, fabricating the CMC-ClyR-ACP nanogel. This nanogel reduced the biofilm viability and promoted more efficient remineralization, while the enamel surface hardness could be preserved by synergistic actions of released ACP and ClyR evaluated in a biofilm-based demineralization cycling model.96 In another study by Chen et al., the incorporation of gold nanoparticles (AuNPs) into as-synthesized CMC/ACP nanohybrids conferred dual functions of bactericidal activity under acidic conditions and superior enamel remineralization.97 Particularly, AuNPs with known enhanced antimicrobial and HAP crystal growth regulation characteristics, alongside CMC-ACP could collaboratively augment remineralization effects and showed biofilm destructive activity. Furthermore, ACP-based nanocomplexes combined with antibacterial agents were made for collaboratively managing dentin caries and hypersensitivity. Recently, an innovative work carried out by Yu and co-workers showed that the co-delivery of EGCG and polycation poly(allylamine) hydrochloride (PAH)-stabilized ACP using hollow mesoporous silica (HMS) (E/PA@HMS) could effectively inhibit S. mutans biofilm growth and provide durable dentin tubule sealing.98 This versatile E/PA@HMS nanosystem enabled the successful PAH-directed intrafibrillar mineralization of collagen with ACP,81 while providing an efficient delivery carrier for the storage and release of ACP precursors and EGCC. Other two-dimensional nanosystems have also been explored. For example, dextran and ACP were inserted between the interlayers of vermiculite (VMT) nanosheets to create a hybrid two-dimensional nanocomposite for integrating the respective excellent properties of each component for tackling dental caries, as follows: (i) dextran stabilized ACP in the soluble state and possessed additional ability to target S. mutans; (ii) ACP provided exogenous Ca2+ and PO43−, contributing to mineral deposits and sealing of dentin tubules; and (iii) VMT nanosheets exhibited pH buffering, antibacterial and antibiofilm properties due to their alkalinity and oxidase/peroxidase-like activities to generate ROS.99 Collectively, the utilization of currently available nanomaterials to encapsulate ACP together with supplementary functional agents can achieve dual or even multiple functions to efficiently manage dental caries.
4.2.2 Hydroxyapatite nanoparticles.
HAP, the chemical analogue of the inorganic component of enamel, is a bioactive ceramic material with the formulation of Ca10(PO4)6(OH)2. Research has shown that nano-sized HAP particles have multiple functions in preventing caries. HAP nanoparticles (nHAP) have strong adsorption capabilities on enamel, and as a calcium phosphate reservoir, exhibit significant remineralization effects by releasing calcium and (hydrogen) phosphate ions.100–102 This property has been extensively evaluated in oral care products and dental materials, such as toothpaste,103,104 dental floss105 and resin infiltrant.106
Studies have indicated that the presence of biofilm layers significantly influences the remineralization effects of nHAP.107 However, the investigations into antimicrobial/anti-biofilm capacities suggest that by reducing the agglomeration of nHAP, the disaggregated nHAP (DnHAP) could inhibit bacterial growth and biofilm formation of S. mutans compared to micro-HAP and untreated nHAP.108 Furthermore, DnHAP could decrease biofilm metabolism and lactic acid production, while strongly inhibiting enamel demineralization in the regrown biofilm models.109 Therefore, nHAP shows great potential as a substitute of fluoride agents, while its size and synthesis require precise control for obtaining bifunctional particles.
In addition, nHAP has been co-delivered or conjugated with diverse molecules into multifunctional nanosystems for more effectively combating dental caries through synergistic anti-bacterial and remineralization effects. For example, nHAP and EGCG were co-loaded into mesoporous silica nanoparticles to create dentin bio-barriers, demonstrating good permeability and occlusion into dentin tubules, as well as inhibiting S. mutans biofilms by released EGCG.110 Moreover, photosensitizers have been incorporated into nanocomposites for antibacterial PDT with the addition of nHAP for remineralization. For instance, a nanosystem consisting of quaternary chitosan (QCS)-coated nHAP loaded with chlorin e6 (Ce6), denoted as Ce6@QCS/nHAP, was developed to eradicate S. mutans biofilms and protect enamel from demineralization through QCS-guided biofilm penetration and interaction with S. mutans, Ce6-mediated photodynamic killing, and nHAP-induced demineralization inhibition.111 Likewise, titanium dioxide (TiO2), activated by a dental curing lamp (385–515 nm) as a photosensitizer, together with nHAP, showed anti-bacterial activity upon exposure to a light-emitting diode and remineralization capacity by increasing the Ca/P ratio of the demineralized enamel surface.112 Accordingly, nHAP functions as a biocompatible reservoir of calcium and phosphate ions and represents a promising anti-caries agent for clinical applications.
4.2.3 Other nanosystems loading calcium phosphate (CaP).
Calcium and phosphate ions can be loaded by some organic nanomaterials possessing antibacterial/antibiofilm capabilities, which can be converted to other forms, and eventually released for mineralization. For example, Yuan and colleagues synthesized antibacterial PNPDC/PGA nanogels by physically cross-linking p(N-isopropylacrylamide-co-2-methacryloyloxyethyl-trimethyl ammonium chloride) (PNPDC) copolymers with γ-polyglutamic acid (PGA), followed by the addition of calcium and phosphate ions and subsequent mineralization into low-crystallinity HAP. The resultant CaP-loaded PNPDC/PGA nanogels served as a multifunctional biomimetic system for dentin remineralization.113 Moreover, Li et al. developed a CaP coating on the surface of Mg-MOF@PDA particles by chelation of polydopamine (PDA), where a pH-responsive metal–organic framework (MOF) synthesized from magnesium (Mg) and gallic acid (GA) was coated with PDA. The antibacterial compound, GA, together with calcium and phosphate ions, was released from MOF in response to the acidic conditions induced by cariogenic biofilms. Furthermore, the photothermal sterilization activated by near infrared light could eradicate biofilms, while simultaneously facilitating the accumulation of Mg2+, Ca2+ and PO43− to promote self-repairing of demineralized enamel.114 Therefore, CaP can be co-loaded into multifunctional nanosystems to provide ionic feedstock for biomimetic mineralization processes.
4.3 Novel protein nanofilm coating formed through amyloid-like aggregation
Recently, nanofilms formed through amyloid-like aggregation of protein have gained significant attention in the field of dentistry due to their ability to promote biomineralization and offer antifouling/antibacterial functions. Initially, Yang and colleagues discovered that lysozyme can undergo a phase transition and amyloid-like aggregation, transitioning from the soluble state to protein thin films (PTFs) through breaking the disulfide bonds in the presence of a disulfide reducing agent, tris(2-carboxyethyl) phosphine (TCEP).115 Surprisingly, the lysozyme-based PTFs could transform into a nanofilm-coating via simple one-step aqueous assembly, adhering stably to various surfaces, while exhibiting multifunctionality.116 The phase-transition lysozyme (PTL) nanofilms, enriched with arginine and tryptophan residues, demonstrated enhanced broad-spectrum antimicrobial efficacy.117 Additionally, due to the presence of abundant carboxyl and hydroxyl groups of PTL nanofilms for Ca2+ binding, they could effectively promote the biomimetic mineralization of HAP crystals.118
Remarkably, through the conjugation of the antifouling agent polyethylene glycol (PEG) with lysozyme, the as-obtained lyso-PEG oligomers could efficiently bind to HAP, forming nanofilms that rapidly coat the deep dentin tubular wall. These unique properties enabled the nanofilms to prevent S. mutans adherence, and significantly enhanced the occlusion of dentinal tubules, offering a potential treatment for dentin hypersensitivity (Fig. 6A).119 In addition, lyso-PEG could penetrate pits and fissures in teeth and promote enamel epitaxial growth with mechanical stability similar to natural enamel. The remineralized enamel-like layers exhibited bactericidal properties, which may be attributed to the antibiofilm capacity of PEG, the positive charge enrichment of the lysozyme nanofilms, and the disruption of EPS by the formed nanostructures.120 Moreover, Fang and colleagues developed lysozyme nanofilms on tooth enamel, incorporating the antimicrobial peptide polyphemusin I (PI), which enhanced the bactericidal capacity, inhibited biofilm formation of S. mutans, prevented demineralization and promoted remineralization (Fig. 6B).121 Collectively, the lysozyme nanofilm coating formed through amyloid-like aggregation exhibited superior adhesive and remineralization capabilities for both enamel and dentin, offering immobilization sites for anchoring antibacterial agents to achieve synergistic functions.
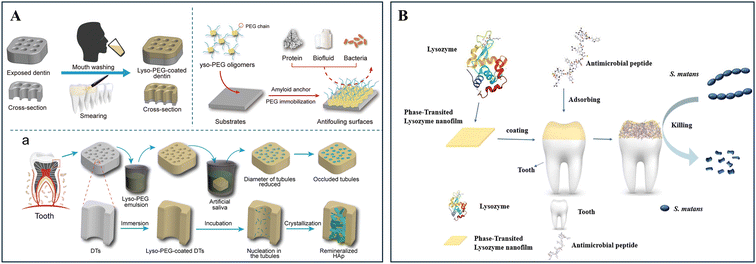 |
| Fig. 6 Bifunctional lysozyme-based nanofilm coating formed through amyloid-like aggregation. (A) Schematic showing that lyso-PEG oligomers rapidly coated the deep dentin tubular wall to form nanofilms, demonstrating antifouling surfaces to prevent S. mutans adherence and significantly enhancing the occlusion of dentinal tubules after nucleation and crystallization. Reproduced from ref. 119. Copyright 2019, Wiley-VCH Verlag GmbH & Co. KGaA, Weinheim. (B) Graphic abstract demonstrating that phase-transition lysozyme nanofilms, absorbing an antimicrobial peptide, coated on the enamel to kill S. mutans for effective caries prevention. Reprinted with permission from ref. 121. Copyright 2022, Elsevier. | |
Besides lysozyme-based nanofilms, other proteins can also form phase-transition nanofilms through amyloid-like aggregation. For instance, the intramolecular disulfide bonds of bovine serum albumin (BSA) could be rapidly reduced by TCEP, resulting in the formation of a robust antifouling proteinaceous nanofilm coating through amyloid-like aggregation.122 When BSA nanofilms were loaded with the cationic antibacterial agent octenidine (OCT), a phase-transited BSA-OCT (PTB-OCT) coating was formed, which efficiently covered teeth, resin composite and sealant surfaces through simple smearing. The PTB-OCT coating offered dual benefits, including the remineralization of demineralized enamel and dentin, while providing antibiofilm properties to prevent primary and secondary caries.123 Likewise, a lactoferrin-derived amyloid (PTLF) nanofilm was developed as a bifunctional coating inspired by the saliva-acquired membrane (SAP), but unlike SAP, the PTLF nanofilm coating could inhibit the adhesion of cariogenic bacteria, such as S. mutans and Lactobacillus acidophilus, while concurrently promoting in situ dentin remineralization.124 In summary, the utilization of nanofilm coatings formed through amyloid-like protein aggregation provides a promising research avenue for the development of novel anti-caries materials. These coatings possess exceptional features, including simple and efficient synthesis, robust stability, strong adhesion to dental hard tissues and restorative materials, flexible structural modification, and the ability to promote mineralization, as well as offer antimicrobial capabilities.
5 Conclusions and perspectives
Currently, the focus of dental caries management is transitioning from reactive to proactive prevention. The precision dentistry emphasizes the unique risk factors brought by the patient, thereby advocating tailor-made preventive and treatment plans, which enable early detection and intervention, targeted therapies, and minimal interventions for the efficient and non-invasive treatment of dental caries. Meanwhile, the development of innovative nanomedicines to combat dental caries in multiple aspects aligns with the advanced concepts raised in caries treatment. Herein, we summarized the noteworthy strategies of developing nanomaterials to prevent and treat dental caries from different levels, such as simultaneously detecting and disrupting cariogenic pathogens within biofilms through nanomedicines with diagnostic capabilities, removing biofilms through a mechanochemical mechanism using magnetic nanorobots or ultrasound-responsive materials, and eradicating bacteria/biofilms, while promoting remineralization using multifunctional nanomaterials.
Moreover, together with the enriched understanding in this field, the importance of healthy oral microbiome has been emphasized in maintaining oral health and preventing caries. Therefore, ecological preventive approaches aiming for long-term caries control have been proposed. Suppressing/neutralizing the virulence factors of cariogenic pathogens/biofilms, while preserving microbe viability or specifically targeting the cariogenic pathogens without interfering with oral microbiota are representative and promising approaches of this topic. Hence, we propose several aspects for the future development and optimization of novel nanosystems/nanomedicines in dental caries treatments, as follows:
(1) Improving biofilm removal efficiency using chemotherapeutic agent-free approaches such as mechanically disrupting biofilm structures using nanoparticles with specific surface properties or shapes, or dismantling biofilms from the tooth surface by nano-based "bulldozers" driven by remote force, such as magnetic field or ultrasound.
(2) Enabling early diagnosis and prevention to achieve the cost-effective management of dental caries by (i) constructing multifunctional theranostic nanomedicines for detecting early lesions and releasing therapeutic agents to promote remineralization or (ii) developing nanomedicines with pH-responsive properties to release therapeutic payloads such as acid neutralizer and remineralization agents in response to the changes of pH.
(3) Designing nanosystems with anti-virulence effects to combat dental caries, while preserving the oral microbiota via (i) targeted delivery of effector molecules to suppress virulence factors or constrain the growth of cariogenic pathogens and (ii) controlled release system to deliver anti-virulence agents in a sustained mode.
Taken together, the use of nanomedicines for treating dental caries offers numerous advantages, and ongoing research and development in this field accelerate the emergence of more effective and personalized approaches for caries management. By harnessing the unique characteristics of nanoparticles, the development of nanomedicines capable of treating dental caries at different levels can benefit dentistry to improve the treatment efficiency and patient outcomes in the management of dental caries. Further studies on the safety, efficacy, and long-term effects of nanomedicines are essential for their subsequent clinical translation and broad utilization in dental practice.
Abbreviations
EPS | Extracellular polymeric substances |
NPs | Nanoparticles |
IONPs | Iron oxide nanoparticles |
CARs | Catalytic antimicrobial robots |
STARS | Surface topography-adaptive robotic superstructures |
US | Ultrasound |
PFH | Perfluorohexane |
ROS | Reactive oxygen species |
CaO2TA-Fe | Iron-tannin modified calcium peroxide |
PLGA | Poly(lactide-co-glycolide) |
CPP-ACP | Casein phosphopeptide-amorphous calcium phosphate |
nano-CaF2 | Nano-sized calcium fluoride |
NSF | Nano silver fluoride |
FerIONP | Ferumoxytol |
CaP | Calcium phosphate |
ACP | Amorphous calcium phosphate |
PNPDC |
p(N-Isopropylacrylamide-co-2-methacryloyloxyethyl-trimethyl ammonium chloride) |
PGA | Polyglutamic acid |
MOF | Metal–organic frameworks |
HAP | Hydroxyapatite |
CMC | Carboxymethyl chitosan |
PCBAA | Poly(carboxybetaine acrylamide) |
EGCG | Epigallocatechin gallate |
AuNPs | Gold nanoparticles |
PAH | Poly(allylamine) hydrochloride |
HMS | Hollow mesoporous silica |
E/PA@HMS | EGCG and polycation poly(allylamine) hydrochloride (PAH)-stabilized ACP using hollow mesoporous silica (HMS) |
VMT | Vermiculite |
nHAP | Hydroxyapatite nanoparticles |
DnHAP | Disaggregated nHAP |
QCS | Quaternary chitosan |
PDT | Photodynamic therapy |
Ce6 | Chlorin e6 |
Ce6@QCS/nHAP | Quaternary chitosan (QCS)-coated nHAP loaded with chlorin e6 |
PTFs | Protein thin films |
TCEP | Tris(2-carboxyethyl) phosphine |
PTL | Phase-transition lysozyme |
PEG | Polyethylene glycol |
PI | Polyphemusin I |
BSA | Bovine serum albumin |
OCT | Octenidine |
SAP | Saliva-acquired membrane |
PTT | Photothermal therapy |
PA | Photoacoustic |
AIE | Aggregation-induced emission |
NIR | Near-infrared |
PTNP | Photothermal nanoparticles |
AIEgens | Aggregation-induced emission luminogens |
TDTPY | 4-(7-(2-(1-Methyl-1l4-pyridin-4-yl)vinyl)-2,3-dihydrothieno[3,4-b][1,4]dioxin-5-yl)-N,N-diphenylaniline |
TMB | 3,3′,5,5′-Tetramethylbenzidine |
LDA | Linear discriminant analysis |
HCA | Hierarchical clustering analysis |
SANs | Single-atom nanozymes |
Data availability
Data sharing is not applicable to this article as no datasets were generated or analysed during the current study.
Conflicts of interest
There are no conflicts to declare.
Acknowledgements
This work was supported by the Health and Medical Research Fund (09203776) and Hong Kong Baptist University (RMGS-2022-13-07). The schematic figure and table of content graphical abstract were created in BioRender. Science Suite Inc. dba BioRender (“BioRender”) has granted Xuan Li permission to use the Graphics in accordance with BioRender's Terms of Service and Academic License Terms.
References
- H. Benzian, R. Watt, Y. Makino, N. Stauf and B. Varenne, Lancet, 2022, 400, 1909–1910 CrossRef PubMed.
- P. Y. F. Wen, M. X. Chen, Y. J. Zhong, Q. Q. Dong and H. M. Wong, J. Dent. Res., 2022, 101, 392–399 CrossRef CAS PubMed.
- I. A. Mjör, J. E. Moorhead and J. E. Dahl, Int. Dent. J., 2000, 50, 361–366 CrossRef PubMed.
- I. Nedeljkovic, J. De Munck, A. Vanloy, D. Declerck, P. Lambrechts, M. Peumans, W. Teughels, B. Van Meerbeek and K. L. Van Landuyt, Clin. Oral Investig., 2020, 24, 683–691 CrossRef PubMed.
- B. T. Rosier, M. De Jager, E. Zaura and B. P. Krom, Front. Cell. Infect. Microbiol., 2014, 4, 92 Search PubMed.
- N. Takahashi and B. Nyvad, J. Dent. Res., 2011, 90, 294–303 CrossRef CAS PubMed.
- Y. Zhu, Y. Wang, S. Zhang, J. Li, X. Li, Y. Ying, J. Yuan, K. Chen, S. Deng and Q. Wang, Front. Microbiol., 2023, 14, 1162380 CrossRef PubMed.
- G. Spatafora, Y. Li, X. He, A. Cowan and A. C. R. Tanner, Microorganisms, 2024, 12, 121 CrossRef CAS PubMed.
- W. H. Bowen, R. A. Burne, H. Wu and H. Koo, Trends Microbiol., 2018, 26, 229–242 CrossRef CAS PubMed.
- H. Koo, M. L. Falsetta and M. I. Klein, J. Dent. Res., 2013, 92, 1065–1073 CrossRef CAS PubMed.
- L. Karygianni, Z. Ren, H. Koo and T. Thurnheer, Trends Microbiol., 2020, 28, 668–681 CrossRef CAS PubMed.
- F. García-Godoy and M. J. Hicks, J. Am. Dent. Assoc., 2008, 139, 25S–34S CrossRef PubMed.
-
M. A. R. Buzalaf, J. P. Pessan, H. M. Honório and J. M. ten Cate, in Monographs in Oral Science, ed. M. A. R. Buzalaf and S. Karger AG, Switzerland, 2011, 22, 97–114 Search PubMed.
- Y. Liao, B. W. Brandt, J. Li, W. Crielaard, C. Van Loveren and D. M. Deng, J. Oral Microbiol., 2017, 9, 1344509 CrossRef PubMed.
- P. Axelsson, B. Nyström and J. Lindhe, J. Clin. Periodontol., 2004, 31, 749–757 CrossRef CAS PubMed.
- D. Eltahlah, C. D. Lynch, B. L. Chadwick, I. R. Blum and N. H. F. Wilson, J. Dent., 2018, 72, 1–7 CrossRef PubMed.
- B. Mekuye and B. Abera, Nano Sel., 2023, 4, 486–501 CrossRef CAS.
-
G. Cao, Nanostructures & Nanomaterials: Synthesis, Properties & Applications, Imperial College Press, London, 2004 Search PubMed.
- A. Besinis, T. De Peralta, C. J. Tredwin and R. D. Handy, ACS Nano, 2015, 9, 2255–2289 CrossRef CAS PubMed.
- C. Hu, L. L. Wang, Y. Q. Lin, H. M. Liang, S. Y. Zhou, F. Zheng, X. L. Feng, Y. Y. Rui and L. Q. Shao, Adv. Healthcare Mater., 2019, 8, 1901301 CrossRef CAS PubMed.
- K. D. Jandt and D. C. Watts, Dent. Mater., 2020, 36, 1365–1378 CrossRef CAS PubMed.
- T. Zhu, Z. Huang, X. Shu, C. Zhang, Z. Dong and Q. Peng, Colloids Surf., B, 2022, 218, 112761 CrossRef CAS PubMed.
- L. Cheng, K. Zhang, M. D. Weir, M. A. S. Melo, X. Zhou and H. H. Xu, Nanomedicine, 2015, 10, 627–641 CrossRef CAS PubMed.
- A. A. Balhaddad, A. A. Kansara, D. Hidan, M. D. Weir, H. H. K. Xu and M. A. S. Melo, Bioact. Mater., 2019, 4, 43–55 Search PubMed.
- A. Clarin, D. Ho, J. Soong, C. Looi, D. S. Ipe and S. K. Tadakamadla, Materials, 2021, 14, 1688 CrossRef CAS PubMed.
- R. Huang, Q. Hu, C.-N. Ko, F. K. Tang, S. Xuan, H. M. Wong, L. Jin, X. Li and K. C.-F. Leung, Mater. Chem. Front., 2024, 8, 9–40 RSC.
- M. J. Oh, A. Babeer, Y. Liu, Z. Ren, J. Wu, D. A. Issadore, K. J. Stebe, D. Lee, E. Steager and H. Koo, ACS Nano, 2022, 16, 11998–12012 CrossRef CAS PubMed.
- A. B. E. Attia, G. Balasundaram, M. Moothanchery, U. S. Dinish, R. Bi, V. Ntziachristos and M. Olivo, Photoacoustics, 2019, 16, 100144 CrossRef PubMed.
- X. Xu, Y. Chong, X. Liu, H. Fu, C. Yu, J. Huang and Z. Zhang, Acta Biomater., 2019, 84, 328–338 CrossRef CAS PubMed.
- M. Hajfathalian, C. R. de Vries, J. C. Hsu, A. Amirshaghaghi, Y. C. Dong, Z. Ren, Y. Liu, Y. Huang, Y. Li, S. A. B. Knight, P. Jonnalagadda, A. Zlitni, E. A. Grice, P. L. Bollyky, H. Koo and D. P. Cormode, J. Clin. Invest., 2023, 133, e168485 CrossRef CAS PubMed.
- J. Luo, Z. Xie, J. W. Y. Lam, L. Cheng, H. Chen, C. Qiu, H. S. Kwok, X. Zhan, Y. Liu, D. Zhu and B. Z. Tang, Chem. Commun., 2001, 18, 1740–1741 RSC.
- Y. Liao, Z. Peng, X. Liu, Y. Hu and J. Zhang, Interdiscip. Med., 2023, 1, e20230024 CrossRef.
- H. Shi, X. Pan, Y. Wang, H. Wang, W. Liu, L. Wang and Z. Chen, ACS Appl. Mater. Interfaces, 2022, 14, 17055–17064 CrossRef CAS PubMed.
- T. Pan, Y. Li, F.-S. Liu, H. Lin and Y. Zhou, ACS Appl. Mater. Interfaces, 2024, 16, 30833–30846 CrossRef CAS PubMed.
- L. Gao, Y. Liu, D. Kim, Y. Li, G. Hwang, P. C. Naha, D. P. Cormode and H. Koo, Biomaterials, 2016, 101, 272–284 CrossRef CAS PubMed.
- Y. Liu, P. C. Naha, G. Hwang, D. Kim, Y. Huang, A. Simon-Soro, H.-I. Jung, Z. Ren, Y. Li, S. Gubara, F. Alawi, D. Zero, A. T. Hara, D. P. Cormode and H. Koo, Nat. Commun., 2018, 9, 2920 CrossRef PubMed.
- P. C. Naha, Y. Liu, G. Hwang, Y. Huang, S. Gubara, V. Jonnakuti, A. Simon-Soro, D. Kim, L. Gao, H. Koo and D. P. Cormode, ACS Nano, 2019, 13, 4960–4971 CrossRef CAS PubMed.
- Y. Huang, Y. Liu, S. Shah, D. Kim, A. Simon-Soro, T. Ito, M. Hajfathalian, Y. Li, J. C. Hsu, L. M. Nieves, F. Alawi, P. C. Naha, D. P. Cormode and H. Koo, Biomaterials, 2021, 268, 120581 CrossRef CAS PubMed.
- X. Zhang, Q. Yang, Y. Lang, X. Jiang and P. Wu, Anal. Chem., 2020, 92, 12400–12406 CrossRef CAS PubMed.
- Y. Liu, Y. Huang, D. Kim, Z. Ren, M. J. Oh, D. P. Cormode, A. T. Hara, D. T. Zero and H. Koo, Nano Lett., 2021, 21, 9442–9449 CrossRef CAS PubMed.
- L. Zhang, Z. Qi, Y. Yang, N. Lu and Z. Tang, ACS Appl. Mater. Interfaces, 2024, 16, 11228–11238 CrossRef CAS PubMed.
- Y. Zhang, M. A. Khan, Z. Yu, W. Yang, H. Zhao, D. Ye, X. Chen and J. Zhang, Small, 2024, 2403878 CrossRef CAS PubMed.
- C. C. Mayorga-Martinez, L. Zhang and M. Pumera, Chem. Soc. Rev., 2024, 53, 2284–2299 RSC.
- H. H. Tran, A. Watkins, M. J. Oh, A. Babeer, T. P. Schaer, E. Steager and H. Koo, Trends Biotechnol., 2024, 42, 479–495 CrossRef CAS PubMed.
- M. Sun, K. F. Chan, Z. Zhang, L. Wang, Q. Wang, S. Yang, S. M. Chan, P. W. Y. Chiu, J. J. Y. Sung and L. Zhang, Adv. Mater., 2022, 34, 2201888 CrossRef CAS PubMed.
- Y. Dong, L. Wang, K. Yuan, F. Ji, J. Gao, Z. Zhang, X. Du, Y. Tian, Q. Wang and L. Zhang, ACS Nano, 2021, 15, 5056–5067 CrossRef CAS PubMed.
- C. C. Mayorga-Martinez, J. Zelenka, K. Klima, P. Mayorga-Burrezo, L. Hoang, T. Ruml and M. Pumera, ACS Nano, 2022, 16, 8694–8703 CrossRef CAS PubMed.
- C. C. Mayorga-Martinez, J. Zelenka, K. Klima, M. Kubanova, T. Ruml and M. Pumera, Adv. Mater., 2023, 35, 2300191 CrossRef CAS PubMed.
- G. Hwang, A. J. Paula, E. E. Hunter, Y. Liu, A. Babeer, B. Karabucak, K. Stebe, V. Kumar, E. Steager and H. Koo, Sci. Rob., 2019, 4, eaaw2388 CrossRef PubMed.
- Z. Ren, D. Kim, A. J. Paula, G. Hwang, Y. Liu, J. Li, H. Daniell and H. Koo, J. Dent. Res., 2019, 98, 322–330 CrossRef CAS PubMed.
- C. Siebenmorgen, A. Poortinga and P. van Rijn, Ultrason. Sonochem., 2023, 100, 106630 CrossRef CAS PubMed.
- G. LuTheryn, C. Hind, C. Campbell, A. Crowther, Q. Wu, S. B. Keller, P. Glynne-Jones, J. M. Sutton, J. S. Webb, M. Gray, S. A. Wilks, E. Stride and D. Carugo, Front. Cell. Infect. Microbiol., 2022, 12, 956808 CrossRef CAS PubMed.
- W. Xiu, L. Ren, H. Xiao, Y. Zhang, D. Wang, K. Yang, S. Wang, L. Yuwen, X. Li, H. Dong, Q. Li, Y. Mou, Y. Zhang, Z. Yin, B. Liang, Y. Gao and L. Wang, Sci. Adv., 2023, 9, eade5446 CrossRef CAS PubMed.
- R. Ramesh, C. Thimonier, S. Desgranges, V. Faugeras, F. Coulouvrat, J. Laurent, G. Marrelec, C. Contino-Pépin, W. Urbach, C. Tribet and N. Taulier, Langmuir, 2023, 39, 15716–15729 CrossRef CAS PubMed.
- L. Xin, C. Zhang, J. Chen, Y. Jiang, Y. Liu, P. Jin, X. Wang, G. Wang and P. Huang, ACS Appl. Mater. Interfaces, 2022, 14, 47420–47431 CrossRef CAS PubMed.
- R. Yang, H. Zhang, Y. Chen, L. Zhang, J. Chu, K. Sun, C. Yuan and K. Tao, ACS Appl. Mater. Interfaces, 2024, 16, 21582–21594 CrossRef CAS PubMed.
- R. Yang, H. Zhang, Z. Marfavi, Q. Lv, Y. Han, K. Sun, C. Yuan and K. Tao, ACS Appl. Mater. Interfaces, 2024, 16, 3126–3138 CrossRef CAS PubMed.
- J. Guo, M. Liu, W. Lei, Y. Xu, K. Li, J. Yu, Y. Sun, C. Huang and X. Zhang, Adv. Funct. Mater., 2023, 33, 2213729 CrossRef CAS.
- J. Moradian-Oldak and A. George, J. Dent. Res., 2021, 100, 1020–1029 CrossRef CAS PubMed.
- N. Philip, Caries Res., 2018, 53, 284–295 CrossRef PubMed.
- Y. Y. Zhang, Q. L. Li and H. M. Wong, Crystals, 2021, 11, 1385 CrossRef CAS.
- H. P. Whelton, A. J. Spencer, L. G. Do and A. J. Rugg-Gunn, J. Dent. Res., 2019, 98, 837–846 CrossRef CAS PubMed.
- C. M. Carey, J. Evid. Based Dent. Pract., 2014, 14(Suppl), 95 CrossRef PubMed.
- L. Sun and L. C. Chow, Dent. Mater., 2008, 24, 111–116 CrossRef CAS PubMed.
- S. Kulshrestha, S. Khan, S. Hasan, M. E. Khan, L. Misba and A. U. Khan, Appl. Microbiol. Biotechnol., 2016, 100, 1901–1914 CrossRef CAS PubMed.
- A. Rosenblatt, T. C. M. Stamford and R. Niederman, J. Dent. Res., 2009, 88, 116–125 CrossRef CAS PubMed.
- A. G. R. Targino, M. A. P. Flores, V. E. dos Santos Junior, F. de Godoy Bené Bezerra, H. de Luna Freire, A. Galembeck and A. Rosenblatt, J. Mater. Sci. Mater. Med., 2014, 25, 2041–2047 CrossRef CAS PubMed.
- C. Pushpalatha, K. V. Bharkhavy, A. Shakir, D. Augustine, S. V. Sowmya, H. A. Bahammam, S. A. Bahammam, N. H. Mohammad Albar, B. Zidane and S. Patil, Front. Bioeng. Biotechnol., 2022, 10, 931327 CrossRef CAS PubMed.
- S. Nguyen, C. Escudero, N. Sediqi, G. Smistad and M. Hiorth, Eur. J. Pharm. Sci., 2017, 104, 326–334 CrossRef CAS PubMed.
- M. Iafisco, L. Degli Esposti, G. B. Ramírez-Rodríguez, F. Carella, J. Gómez-Morales, A. C. Ionescu, E. Brambilla, A. Tampieri and J. M. Delgado-López, Sci. Rep., 2018, 8, 17016 CrossRef PubMed.
- H. Samarehfekri, M. Ranjbar, A. Pardakhty and A. Amanatfard, J. Cluster Sci., 2020, 31, 453–461 CrossRef CAS.
- Y. Xu, Y. You, L. Yi, X. Wu, Y. Zhao, J. Yu, H. Liu, Y. Shen, J. Guo and C. Huang, Bioact. Mater., 2022, 20, 418–433 Search PubMed.
- Y. Huang, Y. Liu, N. K. Pandey, S. Shah, A. Simon-Soro, J. C. Hsu, Z. Ren, Z. Xiang, D. Kim, T. Ito, M. J. Oh, C. Buckley, F. Alawi, Y. Li, P. J. M. Smeets, S. Boyer, X. Zhao, D. Joester, D. T. Zero, D. P. Cormode and H. Koo, Nat. Commun., 2023, 14, 6087 CrossRef CAS PubMed.
- E. Beniash, R. A. Metzler, R. S. K. Lam and P. U. P. A. Gilbert, J. Struct. Biol., 2009, 166, 133–143 CrossRef CAS PubMed.
- S. Habelitz and Y. Bai, J. Dent. Res., 2021, 100, 1434–1443 CrossRef CAS PubMed.
- J. Li, X. Xie, Y. Wang, W. Yin, J. S. Antoun, M. Farella and L. Mei, J. Dent., 2014, 42, 769–777 CrossRef CAS PubMed.
- C. Berg, E. Unosson, H. Engqvist and W. Xia, ACS Biomater. Sci. Eng., 2020, 6, 3599–3607 CrossRef CAS PubMed.
- Q. Ruan, Y. Zhang, X. Yang, S. Nutt and J. Moradian-Oldak, Acta Biomater., 2013, 9, 7289–7297 CrossRef CAS PubMed.
- J. Wang, Z. Liu, B. Ren, Q. Wang, J. Wu, N. Yang, X. Sui, L. Li, M. Li, X. Zhang, X. Li and B. Wang, J. Mater. Sci. Mater. Med., 2021, 32, 115 CrossRef CAS PubMed.
- K. J. Cross, N. L. Huq, J. E. Palamara, J. W. Perich and E. C. Reynolds, J. Biol. Chem., 2005, 280, 15362–15369 CrossRef CAS PubMed.
- L. Niu, S. E. Jee, K. Jiao, L. Tonggu, M. Li, L. Wang, Y. Yang, J. Bian, L. Breschi, S. S. Jang, J. Chen, D. H. Pashley and F. R. Tay, Nat. Mater., 2017, 16, 370–378 CrossRef CAS PubMed.
- D. Wang, J. Deng, X. Deng, C. Fang, X. Zhang and P. Yang, Adv. Mater., 2020, 32, 2002080 CrossRef CAS PubMed.
- S. Yao, S. Chen, R. Wang, K. Zhang, X. Lin and S. Mai, Int. J. Adhes. Adhes., 2022, 119, 103269 CrossRef CAS.
- Z. Chen, S. Cao, H. Wang, Y. Li, A. Kishen, X. Deng, X. Yang, Y. Wang, C. Cong, H. Wang and X. Zhang, PLoS One, 2015, 10, e0116553 CrossRef PubMed.
- Z. Xiao, K. Que, H. Wang, R. An, Z. Chen, Z. Qiu, M. Lin, J. Song, J. Yang, D. Lu, M. Shen, B. Guan, Y. Wang, X. Deng, X. Yang, Q. Cai, J. Deng, L. Ma, X. Zhang and X. Zhang, Dent. Mater., 2017, 33, 1217–1228 CrossRef CAS PubMed.
- J. Song, H. Wang, Y. Yang, Z. Xiao, H. Lin, L. Jin, Y. Xue, M. Lin, F. Chen, M. Zhu, Y. Zhao, Z. Qiu, Y. Li and X. Zhang, J. Mater. Sci. Mater. Med., 2018, 29, 84 CrossRef PubMed.
- J. He, Y. Bao, J. Li, Z. Qiu, Y. Liu and X. Zhang, J. Dent., 2019, 80, 15–22 CrossRef CAS PubMed.
- M. Lee, H. Kim, J. Seo, M. Kang, S. Kang, J. Jang, Y. Lee and J.-H. Seo, Appl. Surf. Sci., 2018, 427, 517–524 CrossRef CAS.
- D. Kim, M.-J. Lee, J.-Y. Kim, D. Lee, J.-S. Kwon and S.-H. Choi, Sci. Rep., 2019, 9, 19550 CrossRef CAS PubMed.
- J. He, J. Yang, M. Li, Y. Li, Y. Pang, J. Deng, X. Zhang and W. Liu, ACS Nano, 2022, 16, 3119–3134 CrossRef CAS PubMed.
- M. Schneider-Rayman, D. Steinberg, R. V. Sionov, M. Friedman and M. Shalish, BMC Oral Health, 2021, 21, 447 CrossRef CAS PubMed.
- S. Han, Y. Abiko, J. Washio, Y. Luo, L. Zhang and N. Takahashi, Caries Res., 2021, 55, 205–214 CrossRef CAS PubMed.
- M. G. B. Aragão, X. He, C. P. Aires and S. A. M. Corona, Arch. Oral Biol., 2024, 164, 105990 CrossRef PubMed.
- D. Dai, J. Wang, H. Xie and C. Zhang, Mater. Today Bio, 2023, 21, 100715 CrossRef CAS PubMed.
- H. Yang, Y. Bi, X. Shang, M. Wang, S. B. Linden, Y. Li, Y. Li, D. C. Nelson and H. Wei, Antimicrob. Agents Chemother., 2016, 60, 7436–7443 CrossRef CAS PubMed.
- Y. Zhu, J. Yan, B. M. Mujtaba, Y. Li, H. Wei and S. Huang, Eur. J. Oral Sci., 2021, 129, e12784 CrossRef CAS PubMed.
- X. Chen, H. Liu, Q. Zhang, X. Chen, L. Wang, Y. Yu and Y. Hao, Front. Bioeng. Biotechnol., 2024, 12, 1421887 CrossRef PubMed.
- J. Yu, H. Bian, Y. Zhao, J. Guo, C. Yao, H. Liu, Y. Shen, H. Yang and C. Huang, Bioact. Mater., 2023, 23, 394–408 CAS.
- Y. Xu, J. Mou and J. Dai, J. Nanobiotechnol., 2024, 22, 490 CrossRef CAS PubMed.
- H. Chen, B. H. Clarkson, K. Sun and J. F. Mansfield, J. Colloid Interface Sci., 2005, 288, 97–103 CrossRef CAS PubMed.
- L. Li, H. Pan, J. Tao, X. Xu, C. Mao, X. Gu and R. Tang, J. Mater. Chem., 2008, 18, 4079–4084 RSC.
- F. Cieplik, C. M. Rupp, S. Hirsch, D. Muehler, J. Enax, F. Meyer, K. A. Hiller and W. Buchalla, BMC Oral Health, 2020, 20, 85 CrossRef CAS PubMed.
- P. Tschoppe, D. L. Zandim, P. Martus and A. M. Kielbassa, J. Dent., 2011, 39, 430–437 CrossRef CAS PubMed.
- A. C. Ionescu, G. Cazzaniga, M. Ottobelli, F. Garcia-Godoy and E. Brambilla, J. Funct. Biomater., 2020, 11, 36 CrossRef CAS PubMed.
- N. Babayevska, M. Woźniak-Budych, J. Litowczenko, B. Peplińska, M. Jarek, P. Florczak, G. Bartkowiak, B. Czarnecka and S. Jurga, Mater. Sci. Eng., C, 2021, 124, 112062 CrossRef CAS PubMed.
- D. M. Andrade Neto, E. V. Carvalho, E. A. Rodrigues, V. P. Feitosa, S. Sauro, G. Mele, L. Carbone, S. E. Mazzetto, L. K. Rodrigues and P. B. A. Fechine, Dent. Mater., 2016, 32, 784–793 CrossRef CAS PubMed.
- M. Zhang, L. B. He, R. A. M. Exterkate, L. Cheng, J. Y. Li, J. M. ten Cate, W. Crielaard and D. M. Deng, J. Dent. Res., 2015, 94, 602–607 CrossRef CAS PubMed.
- W. Luo, Y. Huang, X. Zhou, Q. Han, X. Peng, B. Ren, J. Li, M. Li and L. Cheng, Dent. Mater., 2020, 36, e207–e216 CrossRef CAS PubMed.
- Y. Huang, Q. Han, X. Peng, B. Ren, J. Li, X. Zhou, M. Li and L. Cheng, J. Dent. Res., 2023, 102, 777–784 CrossRef CAS PubMed.
- J. Yu, L. Yi, R. Guo, J. Guo, H. Yang and C. Huang, Int. J. Nanomed., 2021, 16, 3041–3057 CrossRef PubMed.
- W. Guo, Y. Li, S. Wang, Y. Wang, C. Li, Y. Jin, Y. Li, X. Chen and W. Miao, Colloids Surf., B, 2023, 225, 113242 CrossRef CAS PubMed.
- R. Wang, C. Jia, N. Zheng, S. Liu, Z. Qi, R. Wang, L. Zhang, Y. Niu and S. Pan, Photodiagn. Photodyn. Ther., 2023, 42, 103141 CrossRef CAS PubMed.
- R. Yuan, Y. Zhang, L. Liao, Y. Ge, W. Li and Q. Zhi, Int. J. Nanomed., 2023, 18, 4933–4947 CrossRef CAS PubMed.
- Q. Li, J. Liu, H. Liu, Y. Sun, Y. Xu, K. Wang, W. Huang, L. Liao and X. Wang, Bioact. Mater., 2023, 29, 72–84 CAS.
- P. Yang, Macromol. Biosci., 2012, 12, 1053–1059 CrossRef CAS PubMed.
- D. Wang, Y. Ha, J. Gu, Q. Li, L. Zhang and P. Yang, Adv. Mater., 2016, 28, 7414–7423 CrossRef CAS PubMed.
- J. Gu, Y. Su, P. Liu, P. Li and P. Yang, ACS Appl. Mater. Interfaces, 2017, 9, 198–210 CrossRef CAS PubMed.
- Y. Ha, J. Yang, F. Tao, Q. Wu, Y. Song, H. Wang, X. Zhang and P. Yang, Adv. Funct. Mater., 2018, 28, 1704476 CrossRef.
- C. Li, D. Lu, J. Deng, X. Zhang and P. Yang, Adv. Mater., 2019, 31, 1903973 CrossRef CAS PubMed.
- X. Yang, J. Guo, B. Hu, Z. Li, M. Wu, H. Guo, X. Huang, X. Liu, X. Guo, P. Liu, Y. Chen, S. Li, Y. Gu, H. Wu, K. Xuan and P. Yang, Adv. Healthcare Mater., 2022, 11, 2200872 CrossRef CAS PubMed.
- Z. Fang, Y. Zhang, C. Y. Cao, Q. L. Li and H. M. Wong, Mater. Des., 2022, 220, 110891 CrossRef CAS.
- X. Hu, J. Tian, C. Li, H. Su, R. Qin, Y. Wang, X. Cao and P. Yang, Adv. Mater., 2020, 32, 2000128 CrossRef CAS PubMed.
- D. Lu, F. Li, C. Zhao, Y. Ye, X. Zhang, P. Yang and X. Zhang, J. Dent. Res., 2023, 102, 1315–1325 CrossRef CAS PubMed.
- B. Sun, J. Sun, K. Zhang, Y. Pang, C. Zhi, F. Li, Y. Ye, J. Wang, Y. Liu, J. Deng, P. Yang and X. Zhang, Acta Biomater., 2024, 188, 393–405 CrossRef CAS PubMed.
|
This journal is © The Royal Society of Chemistry 2025 |
Click here to see how this site uses Cookies. View our privacy policy here.