DOI:
10.1039/D4PY01076K
(Paper)
Polym. Chem., 2025,
16, 994-1002
Shedding light on surfactant-free emulsion polymerization†
Received
26th September 2024
, Accepted 21st January 2025
First published on 22nd January 2025
Abstract
Herein, we introduce a sustainable method for latex production via surfactant-free emulsion polymerization (SFEP) carrying out a photoinitiated polymerization (photo-SFEP) under both artificial light and sunlight. We discuss the use of sodium phenyl-2,4,6-trimethylbenzoylphosphinate (TPO-Na) as a water-soluble photoinitiator to in situ prepare polymeric nanoparticles under mild conditions, eliminating the need of conventional surfactants. The methodology exploits the rapid photolysis of TPO-Na, which generates anionic radical species that initiate the polymerization of glycidyl methacrylate (GlyMA), selected as a model monomer. Photo-SFEP was optimized to ensure colloidal stability over several months, even under varying environmental ionic strengths. The structural and colloidal properties of the nanoparticles were thoroughly characterized using dynamic light scattering (DLS), transmission electron microscopy (TEM), and zeta potential measurements, confirming the reproducibility and robustness of the latex dispersions. Our methodology shows promise as a scalable, efficient alternative to conventional emulsion polymerization techniques. Additionally, its versatility was affirmed by extending its application to various vinylic monomers, showcasing its broad potential.
1. Introduction
The term ‘latex’ refers to a colloidal dispersion of polymeric nanoparticles in water, stabilized by surfactants.1 Emulsion polymerization, originally developed in the mid-20th century to mimic natural rubber latex,2–4 involves polymerizing hydrophobic monomers in water stabilized by surfactants above their critical micelle concentration. However, the use of surfactants can introduce technical challenges. For instance, in the application of water-based paints, surfactants may migrate to the latex film-air interface after drying, compromising interfacial properties of the material.5,6
Significant efforts have been made over the past decades to prevent surfactant mobility by limiting their dynamic exchange behavior.7 Strategies include covalently linking surfactants to core-forming nanoparticles, and chemically modifying surfactants to introduce reactive groups that act as initiators (inisurf),8–10 monomers (surfmer),11,12 or chain transfer agents (transurf)13,14 in emulsion polymerization. However, issues like unfavourable reactivity ratios of monomers, loss of stability and poor size control of latex persist. A recent alternative involves generating macromolecular surfactants in situ via polymerization-induced self-assembly (PISA).15–20 In emulsion PISA,21 solvophobic monomers are polymerized from reactive solvophilic steric polymers, leading to in situ formation of amphiphilic copolymers that spontaneously self-assemble into monomer-swollen nano-objects. Subsequently, chain polymers continue to develop inside the core of nano-objects.
Another appropriate approach is the complete elimination of surfactants within the process, through surfactant-free emulsion polymerization (SFEP).22–24 In principle, SFEP relies on incorporating charged groups at the polymer chain-ends, providing inter-particle electrostatic repulsion to enhance colloidal latex stability.7 This is achieved in situ by polymerizing hydrophobic monomers in water with a water-soluble radical initiator, forming ionic radical fragments.25–28 In their seminal work, R. H. Ottewill and coll.29 formulated stable polystyrene latex without surfactants, relying on electrostatic repulsion of persulphate fragments produced by the thermal decomposition of potassium persulfate (KPS) initiator. They identified key conditions for successful SFEP: high temperatures to favor rapid radical generation, dilute latex dispersion, and high initiator/monomer ratios. We are considering whether these stringent conditions can be optimized to be more practical, scalable, and applicable for industrial use.
Over the past decades, light has been recognized as a cleaner and environmentally friendly energy source compared to thermal methods in chemistry,30,31 and more specifically in polymer synthesis.32,33 Light can be easily modulated and localized, enabling on-demand initiation and termination of polymerization reactions, resulting in control over polymerization kinetics and molecular weight distribution. In the literature, most studies on radical polymerization focus on thermal initiation,34–38 which involves the homolysis of peroxide or azo compounds that generally require high activation energy at temperatures above 60 °C. In contrast, photolysis of photoinitiators (PIs) is temperature-independent, with low initial activation energy as the energy produced by the excitation of a chromophore exceeds that required for homolysis to generate primary radicals.39,40 The high reactivity of PIs allows for the rapid generation of radicals, which we hypothesize could improve the efficiency of SFEP. Acylphosphine oxide derivatives have attracted significant attention in both academia and industry as PIs due to their strong light absorption and ability to rapidly generate highly reactive radicals.41–47 While most PIs in this important class are organo-soluble, their direct application to SFEP can present challenges related to the heterogeneous reaction medium. This does not necessarily preclude their use, but rather highlights the need to adapt or modify PIs to achieve compatibility with aqueous systems.
The primary objective of the present study was to enhance the efficiency of SFEP strategy by utilizing light instead of heat for the radical initiation process. After evaluating various PIs, sodium phenyl-2,4,6-trimethylbenzoylphosphinate (TPO-Na, Fig. 1) emerged as the most suitable candidate, owing to its water solubility, rapid photolysis, and ability to generate ionic radical fragments. TPO-Na has been previously investigated as a PI to drive PISA in aqueous dispersions.48–50 However, to the best of our knowledge, its potential application in SFEP has not yet been thoroughly explored or reported in the literature. Glycidyl methacrylate (GlyMA) was chosen as a model monomer to optimize the experimental conditions (Fig. 1). Our study demonstrates that TPO-Na can rapidly form and stabilize latex dispersions in a simple reaction medium, achieving colloidal stable latex up to 20% w/w solids content by using very low TPO-Na/monomer molar ratios (R), even in the presence of salts. In this study, the latex formulations are denoted as AS-RGlyMaSC, where ‘AS’ indicates the activation source (T for thermal polymerization at 60 °C, P for photopolymerization at 405 nm, and SL for sunlight), ‘R’ refers to the TPO-Na/GlyMA molar ratio expressed in ‰, and ‘SC’ represents the solids content expressed in w/w%.
 |
| Fig. 1 (a) Synthesis pathway and (b) schematic illustration for the preparation of PGlyMA latex via photo-SFEP of GlyMA in the presence of TPO-Na photoinitiator. | |
2. Materials and methods
2.1. Chemicals and reagents
Glycidyl methacrylate (GlyMA, Merck, 97%), vinyl acetate (VAc, Merck, ≥99%), styrene (S, Merck, 99%), methyl methacrylate (MMA, Merck, 99%), n-butyl acrylate (BA, Merck, ≥99%), ethyl (2,4,6-trimethylbenzoyl) phenylphosphinate (TPO-L, Fluorochem), sodium bromide (NaBr, Merck, ≥99.99%), sodium chloride (NaCl, VWR Chemicals), N,N-dimethylformamide (DMF, Merck, ≥99.8%) and 2-butanone (Acros organics, 99%), potassium persulfate (KPS, Merck, ≥99%), Alcian blue (Merck) were used as received.
2.2. Synthesis of water-soluble photoinitiator TPO-Na
TPO-Na was prepared via chemical modification of TPO-L (Scheme S1†) as previously reported in the literature.51 In a 100 mL round-bottom flask, TPO-L (5 g, 15.8 mmol) and NaBr (1.75 g, 17 mmol) were added under nitrogen to 2-butanone (50 mL) and the reaction mixture was stirred overnight at 50 °C in the dark. The white precipitate formed was recovered by filtration, washed three times with 2-butanone, dried overnight under reduced pressure at 50 °C, and then analyzed by 1H NMR (Fig. S1†).
2.3. Photoinitiated surfactant-free emulsion polymerization (photo-SFEP) of GlyMA at room temperature
The experimental conditions used for all formulations are summarized in Table S1.† All experiments were conducted at ambient temperature (RT), which varied between 20 and 25 °C, as photopolymerization is not significantly influenced by such temperature deviations. In a typical experiment targeting a solids content (SC%) of 10% w/w and R = (nTPO–Na/nGlyMA) × 1000 = 1‰: into 20 mL dried glass vial, 700 μL of GlyMA (0.7 g, 4.9 mmol) were dispersed in 6.1 mL of distilled water (pH ∼ 6.5). 20 μL of DMF were added as an internal standard for 1H NMR analysis. A stirrer magnetic bar was added, and the glass vial was sealed with a septum prior to deoxygenation by nitrogen bubbling for 10 min. 159 μL (1.6 mg, 4.9 μmol) of a separately degassed stock solution of TPO-Na in water (10 mg mL−1) were transferred to the reaction medium under nitrogen. Subsequently, the heterogeneous mixture was irradiated at 405 nm (30 mW) using a light source (Opto-Spectrum Generator L12194-00-39-070) or sunlight, as shown in Fig. S2† and Fig. 6b respectively. After less than 30 min of irradiation, the crude white latex was analyzed by 1H NMR to evaluate the monomer conversion (Fig. S3†).
2.4. Photo-SFEP of GlyMA at 60 °C
Experiments were performed according to the experimental protocol described above (section 2.3) but at 60 °C instead of RT.
2.5. Surfactant-free emulsion polymerization of GlyMA at 60 °C
Experiments were performed according to the experimental protocol described above (section 2.3) in dark at 60 °C and in the presence of KPS instead of TPO-Na.
2.6. Photo-SFEP of GlyMA at large scale
In a 1.5 L homemade glass photoreactor, 188 mL of GlyMA (202 g, 1.4 mol) were dispersed in 805 mL of distilled water, and 2 mL of DMF were added as an internal standard for NMR analysis. Once the reactor was sealed, the mixture was stirred using a propeller agitator (500 rpm, BIOLOCK SCIENTIFIC), and deoxygenation was performed by nitrogen bubbling for 30 minutes. Then, 4.6 mL (0.4 g, 1.4 mmol) of a degassed stock solution of TPO-Na in water (100 mg mL−1) were transferred into the reaction medium under nitrogen. Subsequently, the heterogeneous mixture was irradiated. After 1 hour of irradiation, the crude white latex obtained was analysed by 1H NMR to assess the monomer conversion.
2.7. Characterization methods
2.7.1.
1H NMR spectroscopy.
1H NMR analysis was conducted on a Bruker Avance 300 MHz NMR spectrometer in DMSO-d6.
2.7.2. Size exclusion chromatography.
Prior to SEC analysis, the samples underwent pre-treatment to neutralize the surface charges of the nanoparticles. This pre-treatment involved five cycles where 200 μL of the particle dispersion was added to 800 μL of distilled water and 20 μL of 1 M HCl. After each addition, the mixture was centrifuged for 1 minute, and the excess liquid was carefully removed. The resulting precipitate was lyophilized and dissolved in THF at a concentration of 10 mg mL−1. The molecular weights of the nanoparticles were determined using a SEC system operating in THF at a flow rate of 1 mL min−1 and a temperature of 25 °C. The system was equipped with a multi-angle laser light scattering (MALLS) detector (miniDawn Wyatt), a differential refractometer detector (RID-10A, Shimadzu), an HPLC pump (LC-20AD, Shimadzu), a degasser (DGU-20A3R, Shimadzu), and three PLgel columns with pore sizes of 100
000 Å, 1000 Å, and 100 Å.
2.7.3. Dynamic light scattering.
The hydrodynamic diameter (Dh) and size distribution (PDI) of nano-objects were determined at 20 °C using an ALV/CGS-3 compact goniometer system equipped with an ALV7004 multiple tau digital correlator and a vertically polarized He–Ne laser of 22 mW output power operating at wavelength λ = 632.8 nm. The autocorrelation functions were analyzed in terms of relaxation time (τ) distribution according to the REPES routine.52 Measurements were done at different angles θ, which varied from 20 to 150°, corresponding to scattering wave vectors q ranging from q = 4.6 × 10−3 to 2.55 × 10−2 nm−1. Dh was estimated using the Stokes–Einstein relation (1), where D0 is the diffusion coefficient, kB is the Boltzmann constant, T is the experimental temperature (293 K), and ηs is the viscosity of water. |  | (1) |
2.7.4. Transmission electron microscopy.
Prior to sample deposition, the carbon-coated grids were treated with Alcian blue, which was used both for negative staining and to facilitate adhesion of the negatively charged nanoparticles. Specifically, the grids were first exposed to a drop of Alcian blue solution for 1 minute, followed by gentle washing with a drop of distilled water. Ten microliters of the nano-object suspension were then placed on the treated grids and left for 2 minutes to allow proper adsorption. Excess liquid was carefully blotted. The grids were subsequently visualized at 5.5 kV using a LVEM5 Benchtop Electron Microscope (Delong Instruments). The TEM diameter (DTEM) corresponds to the average of at least 50 nanoparticle diameters using ImageJ software.
2.7.5. Zeta potential.
The zeta potential (ζ) values were determined at 20 °C using a Zetasizer nano series of Malvern instruments. Each value corresponds to the average of 3 runs of the analyzer.
3. Results and discussion
3.1. TPO-Na: synthesis and photophysical properties
TPO-Na is easily synthesized in a single step51 (Scheme S1†) from the commercially available Ethyl (2,4,6-trimethylbenzoyl) phenylphosphinate (TPO-L).53–55 Fig. S1† shows the 1H NMR spectra for both TPO-L and TPO-Na, confirming the successful synthesis of TPO-Na. The disappearance of the methyl and methylene protons (f and g) in the spectrum of TPO-Na, which are previously present in the spectrum of TPO-L, indicates the quantitative synthesis of TPO-Na. On the other hand, the modification of TPO-L to TPO-Na induces a noticeable change in the UV absorption spectrum, particularly in the region between 320–370 nm. DMSO was used as a common solvent for both TPO-Na and TPO-L, allowing for a more direct comparison of their absorption spectra in a non-aqueous medium. As shown in Fig. S4,† TPO-Na in water exhibits a slight blue shift in comparison to TPO-Na and TPO-L in DMSO. Beyond the region around 370 nm, no significant changes are observed between the spectra.
3.2. Photo-SFEP versus thermal-SFEP
To compare the performance of photoinitiation versus thermal-initiation, we reproduced the requested experiment conditions suggested by R. H. Ottewill and coll.,29 using GlyMA as model monomer and KPS as thermal initiator. All experiments were conducted using distilled water (pH ∼ 6.5) to prevent the hydrolysis of the epoxy group of GlyMA moities.56 Their experiment, carried out at 60 °C in a dilute medium (SC = 2.2% w/w) with a high initiator-to-monomer ratio (R = 20‰), required over 2 hours to achieve high monomer conversion. Spherical nanoparticles with a diameter estimated by TEM (DTEM) of 118 nm were produced (Fig. 2a). Additional TEM images are provided in the ESI (Fig. S5†). However, dynamic light scattering (DLS) indicated much larger hydrodynamic diameters (Dh = 977 nm), suggesting significant particle aggregation. This apparent size disparity between Dh and DTEM can be attributed to the aggregation behaviour in the dispersion. Such aggregation leads to an increased apparent size in DLS measurements, which record the motion of particle clusters rather than individual nanoparticles. Precipitation occurring after a few hours further supports the occurrence of aggregation of the particles (Fig. 2b).
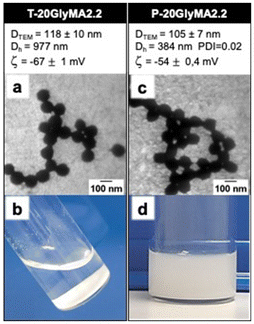 |
| Fig. 2 Surfactant-free latex prepared via SFEP of GlyMA at 60 °C (T-20GlyMA2.2) and under 405 nm irradiation at RT (P-20GlyMA2.2) using R = 20‰ and SC = 2.2% w/w in water. (a and c) TEM images of latex immediately after synthesis. (b and d) Photographs of latex dispersions after three months in dark at RT. | |
Photoinitiated SFEP (photo-SFEP) using TPO-Na under 405 nm irradiation at room temperature (RT) was performed to evaluate the impact of photoinitiation on nanoparticle stability. The use of 405 nm visible light reduces light scattering compared to shorter UV wavelengths, enabling effective penetration in the aqueous reaction medium. Additionally, the high solubility of TPO-Na in the continuous phase ensures uniform initiation. Complete monomer conversion occurred in less than 30 minutes when TPO-Na was used instead of KPS. In comparison with previous experiment, Fig. 2c shows the formation of nanoparticles with smaller hydrodynamic and TEM diameters. Negative zeta potentials were observed in both cases. Note that TEM images revealed nanoparticles appearing connected, likely due to water pulling them together through capillary action during TEM-grid drying. High-resolution TEM confirms this behaviour (Fig. S6†). Importantly, the nanoparticle dispersion remained stable for at least three months (Fig. 2d), indicating that TPO-Na is an effective photoinitiator for surfactant-free latex formulations. A similar synthesis using photo-SFEP conducted at 60 °C resulted in the production of stable latex nanoparticles of similar diameters to those obtained at RT (Fig. S7a†), indicating that temperature does not significantly affect the final product.
To further investigate the origin of this stability, a similar synthesis was performed using TPO-L, the oil-soluble precursor of TPO-Na. In this case, only precipitation was observed (Fig. S8†), demonstrating that the ionic charges introduced by TPO-Na play a critical role in stabilizing the latex dispersions through electrostatic repulsion.
3.3. Effect of TPO-Na/GlyMA molar ratio
We addressed the challenge of minimizing the amount of TPO-Na photoinitiator employed during SFEP while ensuring the stability and desired properties of the resulting latex particles. Consequently, the TPO-Na/GlyMA molar ratio (R) was progressively reduced to 0.08‰. All experiments were performed at SC of 10% w/w. Furthermore, analysis of the produced polymeric chains by SEC revealed bimodal and broad chromatograms, with dispersities exceeding 1.5 (Fig. S9 and S10†). This behaviour is expected due to the conventional radical polymerization mechanism employed in this work, where chain length control is inherently absent. It is important to note that controlling the polymer chains was not an objective of this study.
Colloidal stability was tracked using DLS, TEM and zeta potential (ζ) for three months (Fig. 3). Additional TEM images are provided in the ESI (Fig. S11–S16†). Immediately after formulation, varying the ratio TPO-Na/GlyMA from 2.5‰ to 0.5‰ did not significantly affect the polymerization rate (complete conversion after less than 1 h of irradiation), nor did influence the physical characteristics of latex, as Dh, DTEM and ζ remain low and relatively constants (Fig. 3). All R ratios in this range lead to suspension with great stability for at least three months. Further reducing the R ratio below the threshold value of R = 0.5‰ resulted in a gradual increase in Dh, leading to a rapid precipitation at R = 0.08‰. This highlights the critical role of maintaining a sufficient PI concentration to ensure effective polymerization and prevent particle aggregation. Our results demonstrate that at SC = 10%, the concentration of TPO-Na in photo-SFEP for ensuring stable latex dispersions could be reduced to 0.5‰. Below this ratio, there is insufficient PIs to generate enough anionic radicals for initiating the polymerization and subsequently stabilizing the dispersion by electrostatic repulsions. Note that latex synthesis via SFEP conducted at 60 °C in the presence of low KPS/GlyMA molar ratio (R = 1‰) resulted in a direct precipitation (Fig. S7b†), confirming the limitation of persulphate in latex stabilization at low R.
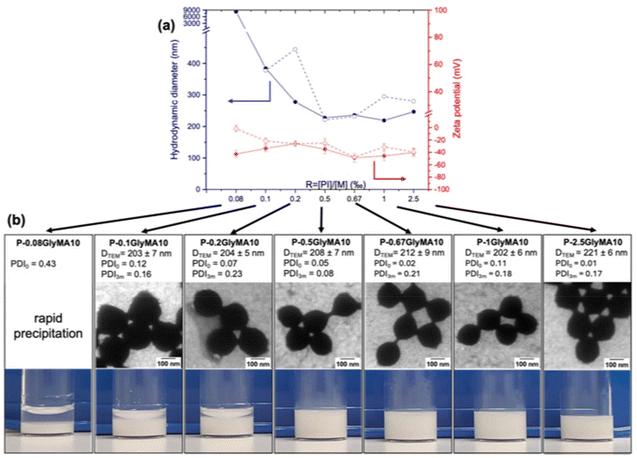 |
| Fig. 3 Surfactant-free latex prepared via photo-SFEP of GlyMA under 405 nm irradiation at SC = 10% w/w in water, at RT and using different TPO-Na/GlyMA molar ratios. (a) Hydrodynamic diameters (circle) and zeta potentials (diamond) of nanoparticles immediately after formulation (open symbols) and after three months (solid symbols). (b) TEM images of nanoparticles immediately after formulation, and photographs of latex dispersions after three months. DTEM values represent the average diameter of at least 50 nanoparticles, measured using ImageJ software. PDI values were obtained from DLS measurements at a 90° angle, recorded immediately after formulation (PDI0) and after 3 months (PDI3m). | |
3.4. Effect of the solids content
Solids content (SC) is a critical parameter to consider when developing any novel latex formulation methodology. To evaluate the ability of photo-SFEP to produce more concentrated dispersion, latex was prepared with SC values ranging from 2.2% w/w to 50% w/w, while maintaining a constant TPO-Na/GlyMA ratio of 1‰. Experiments conducted at SC equal to 30%, 40%, and 50% w/w produced unstable latex dispersions (Fig. S17†). In contrast, latex synthesized at SC below 20% w/w (Fig. 4) displayed similarly low zeta potentials, with a dependency of nanoparticle size on SC. Specifically, increasing SC led to a corresponding increase in both Dh and DTEM of the nanoparticles. Additional TEM images are provided in the ESI (Fig. S18 and S19†). After three months, the zeta potentials remained relatively stable, indicating excellent colloidal stability, as also observed in the latex dispersion images.
 |
| Fig. 4 Surfactant-free latex prepared via photo-SFEP of GlyMA under 405 nm irradiation using R = 1‰ at solids content of (a) 2.2% w/w, (b) 10% w/w and (c) 20% w/w. | |
3.5. Colloidal stability in ionic environment
One of the well-known challenges of SFEP is the destabilization of latex formulations in the presence of electrolytes.29 In this context, our study seeks to assess the colloidal stability of latex produced via photo-SFEP, formulated with different ratios of TPO-Na and subsequently exposed to saline environments. Samples, initially prepared at a SC of 10% w/w, were diluted to 0.1% w/w using aqueous brine solutions with NaCl concentrations ranging from 10−4 mol L−1 to 1 mol L−1. The colloidal stability of these nanoparticles was systematically monitored at two distinct time intervals: 2 hours and 3 months post-preparation. As shown in Fig. 5, all formulations exhibit consistent Dh up to a [NaCl] = 0.01 mol L−1. However, the zeta potential values demonstrate a progressive increase with the salt concentration, indicating the progressive screening of the surface nanoparticles charge. Above a threshold [NaCl] of 0.01 mol L−1, the formulations show marked signs of instability, highlighted by the increase of particles diameter and zeta potentials, especially when high TPO-Na/GlyMA molar ratio (R = 2.5‰) was used. This trend points to the aggregation and subsequent precipitation after three months as evidenced in the Fig. S20.† These findings illustrate that latex synthesized via photo-SFEP using TPO-Na exhibit good stability up to a brine concentration of 0.01 mol L−1 (Fig. S21†), which approximates the average ionic strength encountered in tap water. Similar observations were made during photo-SFEP in the presence of salts, where increasing the ionic strength above 0.01 g L−1 resulted in destabilization and particle aggregation (Fig. S22†).
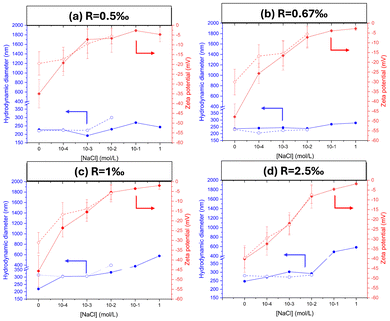 |
| Fig. 5 Colloidal stability against salts of surfactant-free latex prepared via photo-SFEP of GlyMA under 405 nm irradiation in water at SC = 10% and differents R (a) 0.5‰, (b) 0.67‰, (c) 1‰ and (d) 2.5‰. Hydrodynamic diameter (circle) and zeta potential (diamond) of nanoparticles after 2 h (open symbols) and three months (solid symbols) of preparation. | |
3.6. Photo-SFEP sustainability
In line with the goal of developing more sustainable approaches to SFEP, we firstly investigated the lyophilization of latex and its subsequent re-dispersion in water. Lyophilization offers significant sustainability benefits enabling easier transportation, longer shelf-life, and reduced need for refrigeration or bulky liquid storage. Latex produced via photo-SFEP can be successfully lyophilized and re-dispersed with minimal impact on its colloidal stability and particle size distribution. Specifically, zeta potential of nanoparticles remained consistent after re-dispersion, highlighting the resilience of the colloidal system. Fig. 6a shows latex prepared at TPO-Na/GlyMA ratio of 0.67‰, then lyophilized and re-dispersed. TEM images further confirm that the morphological integrity of the latex particles is preserved after the drying and rehydration process. Similar results were observed for other ratios (Fig. S23–S26†). However, it is noted that the Dh increases from 231 nm to 376 nm upon redispersion, suggesting some degree of particle aggregation. This is attributed to the drying process, where particle interactions in the absence of stabilizers can lead to partial aggregation. Despite this, the system retains colloidal stability, demonstrating a relatively high degree of redispersibility.
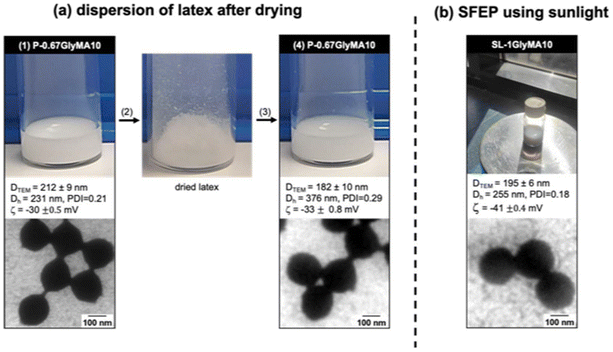 |
| Fig. 6 Sustainability of photo-SFEP process. (a) (1) Surfactant-free latex prepared via photo-SFEP of GlyMA under 405 nm irradiation using R = 0.67‰ at SC = 10 w/w. (2) The nanoparticles were lyophilized and subsequently (3) re-dispersed in water targeting initial SC = 10 w/w. (b) Surfactant-free latex prepared via photo-SFEP of GlyMA under sunlight using R = 1‰ at SC = 10 w/w in water. | |
Furthermore, we explored the potential of using sunlight as a direct energy source to carry out the photoinitiated polymerization, eliminating the need of artificial light source, and further enhancing the eco-friendliness of the process. We compared latex synthesized under sunlight (SL-1GlyMA10, Fig. 6b) with those prepared using artificial 405 nm irradiation (P-1GlyMA10, Fig. 4b). Both methods yielded stable dispersions after less than one hour of irradiation with similar diameters and zeta potentials, demonstrating that sunlight is an effective and sustainable alternative for driving photo-SFEP. Additional TEM images are available in the ESI (Fig. S27†).
3.7. Photo-SFEP at large scale
To demonstrate the scalability of our photo-SFEP process, we successfully increased the reaction volume from 7 mL to 1000 mL, while maintaining both nanoparticle size and colloidal stability. Fig. 7a shows the setup for the scaled-up reaction, carried out under 405 nm irradiation, yielding a stable 1-liter latex dispersion (Fig. 7b).
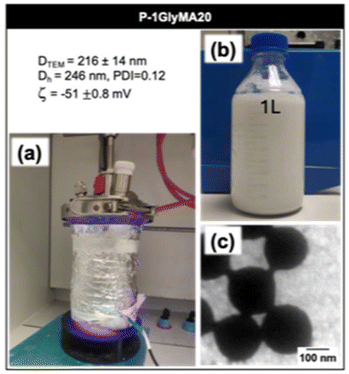 |
| Fig. 7 Scale-up of surfactant-free latex synthesis via photo-SFEP of GlyMA under 405 nm irradiation. (a) Reaction setup for the scale-up to 1 liter, using R = 1‰ and SC = 20% w/w. (b) The resulting stable latex dispersion produced in a 1 L batch. (c) TEM image of nanoparticles in the latex. | |
The hydrodynamic diameter, polydispersity index, and zeta potential of the nanoparticles were measured after synthesis, with values of Dh = 246 nm, PDI = 0.12, and ζ = −51 ± 0.8 mV. These results are comparable to those obtained at smaller volumes (Fig. 4b), demonstrating that the scale-up of the process did not compromise the quality of the latex. TEM analysis (Fig. 7c and Fig. S28†) confirmed that the nanoparticles maintained their spherical morphology, with an average diameter of 216 ± 14 nm. Although some optimization is required, particularly regarding stirring rates to ensure uniform mixing in larger volumes, this experiment serves as a proof of concept for the scalability of the photo-SFEP process. It demonstrates the robustness of the method, allowing for larger volume production while preserving the desired particle properties and colloidal stability.
3.8. Photo-SFEP versatility
The versatility of the photo-SFEP process was assessed by exploring different hydrophobic monomer families, including vinyl acetate (VAc), methyl methacrylate (MMA), styrene (S), and n-butyl acrylate (BA). Photo-SFEP was conducted in distilled water with a R ratio of 1‰ and a SC of 10%. Full monomer conversion was obtained after less than one hour of irradiation at 405 nm, demonstrating the robustness of this approach across a wide range of monomers.
On one hand, as shown in Fig. 8, photo-SFEP of Vac, MMA and S resulted in the formation of spherical nanoparticles, with suspensions remaining stable for several months. While DLS measurements confirmed consistent size distributions for most of the monomers, TEM images revealed some deviation in particle size for S, where a broader particle distribution was observed (Fig. S29 and S31†). This indicates slight irregularities in particle size control for styrene, despite overall successful polymerization. Interestingly, compared to GlyMA, which produced larger nanoparticles (DTEM = 202 nm, and Dh = 219 nm), Vac, MMA and S monomers yielded smaller nanoparticles. However, we do not yet have a rational explanation for the reduced particle sizes observed with these monomers. Further investigation will be required to fully understand the underlying mechanisms driving this size reduction.
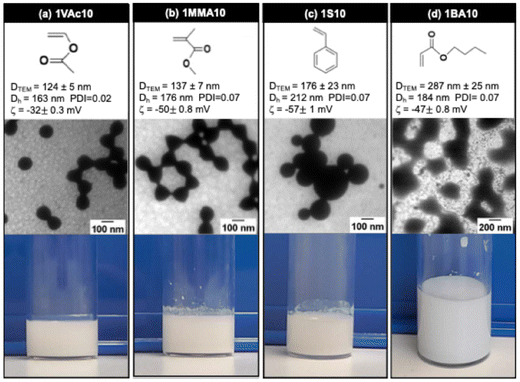 |
| Fig. 8 TEM images and photographs of surfactant-free latex dispersions prepared via photo-SFEP under 405 nm irradiation at R = 1‰ and SC = 10% w/w of various monomers: (a) vinyl acetate (VAc), (b) methyl methacrylate (MMA), (c) and styrene (S), and (d) n-butyl acrylate (BA). | |
On the other hand, experiments conducted with BA successfully produced nanoparticle dispersions, as confirmed by DLS measurements. However, obtaining clear TEM images proved challenging due to the low glass transition temperature (Tg) of poly(n-butyl acrylate), around −45 °C,57 which caused deformation of the nanoparticles and hindered their visualization under electron microscopy at RT. Despite this limitation, the DLS data confirm successful polymerization and stable nanoparticle formation.
4. Conclusions
The present paper offers fresh insights into surfactant-free latex synthesis by utilizing light-driven surfactant-free emulsion polymerization, leading to an enhanced quality of the final latex. Using TPO-Na as a photoinitiator allowed us to conduct the process efficiently at relatively high solids content and with a minimal environmental impact. The study highlights the critical role of photoinitiator concentration and solids content in optimizing the stability and properties of the latex. Remarkably, we were able to use sunlight as energy source, which underscores the sustainability of our process. Additionally, the successful scale-up to 1 L demonstrated the robustness of this approach, showing that it remains effective even in larger volumes. We also demonstrated that the latex could be dried and successfully re-dispersed, potentially reducing transportation and handling costs significantly.
Further work is currently in progress to investigate the kinetics of photo-SFEP, with the goal of gaining a deeper understanding of the reaction mechanism underlying this methodology. Additionally, we have demonstrated the high efficiency of TPO-Na, even at very low concentrations, in the formulation of nanoparticles. This opens further avenues of research to investigate its influence on nanoparticle morphology when used as a photoinitiator instead of TPO-L58,59 in PISA for instance. This approach not only adheres to sustainable practices but also advances the field of polymer chemistry, offering promising implications for industrial production.
Author contributions
Erika Paola Fonseca PARRA performed the experiments and wrote the first draft of the manuscript. Jean-Luc SIX supervised the work and revised the manuscript. Khalid FERJI conceptualized and designed the project, supervised the work, and wrote the final manuscript.
Data availability
The data supporting the findings of this study are fully included in the ESI† provided with this article. This includes all datasets, detailed methodologies, and analysis scripts necessary to reproduce the results.
Conflicts of interest
There are no conflicts to declare.
Acknowledgements
The present work has benefited from the platform APPEL of LCPM for NMR and DLS analyses. The authors acknowledge Ana Andrea Arteni from I2BC for high TEM resolution.
References
- H. Kawaguchi, Prog. Polym. Sci., 2000, 25, 1171–1210 CrossRef CAS.
- W. V. Smith and R. H. Ewart, J. Chem. Phys., 1948, 16, 592–599 CrossRef CAS.
- G. S. Whitby and M. Katz, Ind. Eng. Chem., 1933, 25, 1338–1348 CrossRef.
- W. D. Harkins, J. Am. Chem. Soc., 1947, 69, 1428–1444 CrossRef CAS PubMed.
- A. Guyot, Adv. Colloid Interface Sci., 2004, 108–109, 3–22 CrossRef CAS PubMed.
- P. A. Lovell and F. J. Schork, Biomacromolecules, 2020, 21, 4396–4441 CrossRef CAS PubMed.
- C. Pichot, B. Charleux, M.-T. Charreyre and J. Revilla, Macromol. Symp., 1994, 88, 71–87 CrossRef CAS.
- S. S. Ivanchev, V. N. Pavljuchenko and N. A. Byrdina, J. Polym. Sci., Part A: Polym. Chem., 1987, 25, 47–62 CrossRef CAS.
- J. M. H. Kusters, D. H. Napper, R. G. Gilbert and A. L. German, Macromolecules, 1992, 25, 7043–7050 CrossRef CAS.
- M. Wu, L. M. F. Ramirez, A. R. Lozano, D. Quemener, J. Babin, A. Durand, E. Marie, J. L. Six and C. Nouvel, Carbohydr. Polym., 2015, 130, 141–148 CrossRef CAS PubMed.
- A. Guyot and K. Tauer, Polym. Synth., 2005, 43–65 Search PubMed.
- H. A. S. Schoonbrood and J. M. Asua, Macromolecules, 1997, 30, 6034–6041 CrossRef CAS.
- T. S. Wilkinson, A. Boonstra, A. Montoya-Goñi, S. van Es, M. J. Monteiro and A. L. German, J. Colloid Interface Sci., 2001, 237, 21–27 CrossRef CAS PubMed.
- L. M. Forero Ramirez, J. Babin, A. Boudier, C. Gaucher, M. Schmutz, M. Er-Rafik, A. Durand, J.-L. Six and C. Nouvel, Carbohydr. Polym., 2019, 224, 115153 CrossRef CAS PubMed.
- N. J. W. Penfold, J. Yeow, C. Boyer and S. P. Armes, ACS Macro Lett., 2019, 8, 1029–1054 CrossRef CAS PubMed.
- D. Ikkene, J.-L. Six and K. Ferji, Eur. Polym. J., 2023, 188, 111848 CrossRef CAS.
- C. Liu, C.-Y. Hong and C.-Y. Pan, Polym. Chem., 2020, 11, 3673–3689 RSC.
- F. D'Agosto, J. Rieger and M. Lansalot, Angew. Chem., Int. Ed., 2020, 59, 8368–8392 CrossRef PubMed.
- J. L. Six and K. Ferji, Polym. Chem., 2019, 10, 45–53 RSC.
- J. Yeow and C. Boyer, Adv. Sci., 2017, 4, 1700137 CrossRef PubMed.
- B. Charleux, G. Delaittre, J. Rieger and F. D'Agosto, Macromolecules, 2012, 45, 6753–6765 CrossRef CAS.
- P. J. Feeney, D. H. Napper and R. G. Gilbert, Macromolecules, 1987, 20, 2922–2930 CrossRef CAS.
- S. T. Camli, F. Buyukserin, O. Balci and G. G. Budak, J. Colloid Interface Sci., 2010, 344, 528–532 CrossRef CAS PubMed.
- Q. Cao, T. Heil, B. Kumru, M. Antonietti and B. Schmidt, Polym. Chem., 2019, 10, 5315–5323 RSC.
- F. A. Bovey and I. M. Kolthoff, J. Polym. Sci., 1950, 5, 487–504 CrossRef CAS.
- J. W. Goodwin, J. Hearn, C. C. Ho and R. H. Ottewill, Br. Polym. J., 1973, 5, 347–362 CrossRef CAS.
- A. Dunn and L. H. Chong, Br. Polym. J., 1970, 2, 49–59 CrossRef CAS.
- F. Stoffelbach, L. Tibiletti, J. Rieger and B. Charleux, Macromolecules, 2008, 41, 7850–7856 CrossRef CAS.
- J. W. Goodwin, J. Hearn, C. C. Ho and R. H. Ottewill, Colloid Polym. Sci., 1974, 252, 464–471 CrossRef CAS.
- C. K. Prier, D. A. Rankic and D. W. C. MacMillan, Chem. Rev., 2013, 113, 5322–5363 CrossRef CAS PubMed.
- D. Staveness, I. Bosque and C. R. J. Stephenson, Acc. Chem. Res., 2016, 49, 2295–2306 CrossRef CAS PubMed.
- S. C. Ligon, B. Husar, H. Wutzel, R. Holman and R. Liska, Chem. Rev., 2014, 114, 557–589 CrossRef CAS PubMed.
- M. Chen, M. J. Zhong and J. A. Johnson, Chem. Rev., 2016, 116, 10167–10211 CrossRef CAS PubMed.
- K. Matyjaszewski and J. H. Xia, Chem. Rev., 2001, 101, 2921–2990 CrossRef CAS PubMed.
- J. Chiefari, Y. K. Chong, F. Ercole, J. Krstina, J. Jeffery, T. P. T. Le, R. T. A. Mayadunne, G. F. Meijs, C. L. Moad, G. Moad, E. Rizzardo and S. H. Thang, Macromolecules, 1998, 31, 5559–5562 CrossRef CAS.
- C. J. Hawker, A. W. Bosman and E. Harth, Chem. Rev., 2001, 101, 3661–3688 CrossRef CAS PubMed.
- M. Kamigaito, T. Ando and M. Sawamoto, Chem. Rev., 2001, 101, 3689–3745 CrossRef CAS PubMed.
- M. Husseman, E. E. Malmström, M. McNamara, M. Mate, D. Mecerreyes, D. G. Benoit, J. L. Hedrick, P. Mansky, E. Huang, T. P. Russell and C. J. Hawker, Macromolecules, 1999, 32, 1424–1431 CrossRef CAS.
- F. Jasinski, P. B. Zetterlund, A. M. Braun and A. Chemtob, Prog. Polym. Sci., 2018, 84, 47–88 CrossRef CAS.
- P. Xiao, J. Zhang, F. Dumur, M. A. Tehfe, F. Morlet-Savary, B. Graff, D. Gigmes, J. P. Fouassier and J. Lalevee, Prog. Polym. Sci., 2015, 41, 32–66 CrossRef CAS.
-
J.-P. Fouassier and J. Lalevée, in Photoinitiators, ed. Wiley, 2021, pp. 35–53 Search PubMed.
- J.-P. Fouassier, D. Burr and F. Wieder, J. Polym. Sci., Part A: Polym. Chem., 1991, 29, 1319–1327 CrossRef CAS.
- Y. Shi, G. Liu, H. Gao, L. Lu and Y. Cai, Macromolecules, 2009, 42, 3917–3926 CrossRef CAS.
- G. W. Sluggett, P. F. McGarry, I. V. Koptyug and N. J. Turro, J. Am. Chem. Soc., 1996, 118, 7367–7372 CrossRef CAS.
- C. Decker, Prog. Polym. Sci., 1996, 21, 593–650 CrossRef CAS.
- M. Jacobi, Polym. Paint Color J., 1985, 175, 636 CAS.
- G. W. Sluggett, C. Turro, M. W. George, I. V. Koptyug and N. J. Turro, J. Am. Chem. Soc., 1995, 117, 5148–5153 CrossRef CAS.
- J. Tan, H. Sun, M. Yu, B. S. Sumerlin and L. Zhang, ACS Macro Lett., 2015, 4, 1249–1253 CrossRef CAS PubMed.
- Y. Jiang, N. Xu, J. Han, Q. Yu, L. Guo, P. Gao, X. Lu and Y. Cai, Polym. Chem., 2015, 6, 4955–4965 RSC.
- J. Tan, D. Liu, Y. Bai, C. Huang, X. Li, J. He, Q. Xu, X. Zhang and L. Zhang, Polym. Chem., 2017, 8, 1315–1327 RSC.
- T. Majima, W. Schnabel and W. Weber, Makromol. Chem., 1991, 192, 2307–2315 CrossRef CAS.
- J. Jakes, Collect. Czech. Chem. Commun., 1995, 60, 1781–1797 CrossRef CAS.
- K. Ferji, P. Venturini, F. Cleymand, C. Chassenieux and J.-L. Six, Polym. Chem., 2018, 9, 2868–2872 RSC.
- P. Lertturongchai, M. I. A. Ibrahim, A. Durand, P. Sunintaboon and K. Ferji, Macromol. Rapid Commun., 2020, 41, 2000058 CrossRef CAS PubMed.
- D. Ikkene, A. A. Arteni, C. Boulogne, J.-L. Six and K. Ferji, Macromolecules, 2022, 55, 4268–4275 CrossRef CAS.
- L. P. D. Ratcliffe, A. J. Ryan and S. P. Armes, Macromolecules, 2013, 46, 769–777 CrossRef CAS.
- A. I. Buzin, M. Pyda, P. Costanzo, K. Matyjaszewski and B. Wunderlich, Polymer, 2002, 43, 5563–5569 CrossRef CAS.
- D. Ikkene, A. A. Arteni, M. Ouldali, G. Francius, A. Brûlet, J.-L. Six and K. Ferji, Biomacromolecules, 2021, 22, 3128–3137 CrossRef CAS PubMed.
- D. Ikkene, A. A. Arteni, M. Ouldali, J.-L. Six and K. Ferji, Polym. Chem., 2020, 11, 4729–4740 RSC.
|
This journal is © The Royal Society of Chemistry 2025 |
Click here to see how this site uses Cookies. View our privacy policy here.