DOI:
10.1039/D5QO00100E
(Research Article)
Org. Chem. Front., 2025, Advance Article
Recyclable g-C3N4 catalyzed decarboxylative alkenylation of N-aryl glycines with vinyl sulfones under visible-light irradiation†
Received
17th January 2025
, Accepted 11th February 2025
First published on 14th February 2025
Abstract
Allylamines are a versatile class of compounds with significant applications in pharmaceuticals and as building blocks in organic synthesis. Herein we present a straightforward protocol for visible-light driven g-C3N4-catalyzed decarboxylative alkenylation of N-aryl glycines with vinyl sulfones to access allylamines in moderate to excellent yields (up to 91%), and it demonstrated broad substrate compatibility, including primary, secondary, and tertiary N-aryl glycines and diverse vinyl sulfones. Notably, the g-C3N4 catalyst is recyclable up to five times without obvious loss of catalytic performance. Preliminary mechanistic studies indicated that visible light is essential to achieve the desired transformation efficiently.
Introduction
Allylamines are a class of compounds with diverse pharmacological effects, abundant in the pharmaceutical field as antifungals, antihistamines, antidepressants and more (Scheme 1A).1 Over the past few decades, numerous methods for synthesizing allylamine derivatives have been developed.2 However, most of these strategies rely on the use of expensive or toxic transition metal catalysts, high temperatures, and harsh reaction conditions, resulting in limited substrate compatibility. Recently, visible-light promoted photoredox catalysis has been widely used in organic synthesis, leveraging visible light as an energy source to drive chemical reactions in a clean, efficient, and sustainable manner.3 Homogeneous photoredox catalysts, such as transition metal complexes and organic dyes, have been widely utilized in organic synthesis.4 However, resource scarcity, high price and metal contamination in products limited their potential practical application. To address these challenges, the development of practical heterogeneous catalysts has become a promising alternative. g-C3N4, also known as graphitic carbon nitride, is a semiconductor material composed of carbon and nitrogen elements and exhibits excellent photocatalytic and photothermal properties, making it applicable in various fields.5 Moreover, owing to its wide availability and remarkable stability, g-C3N4 has also been employed as a photoredox catalyst in organic synthesis.6
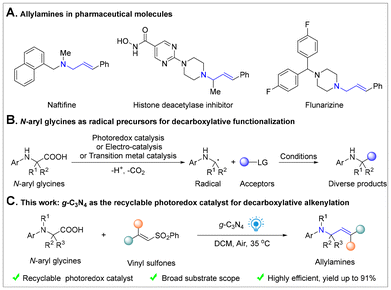 |
| Scheme 1 (A) Allylamines in pharmaceutical molecules. (B) N-Aryl glycines as radical precursors for decarboxylative functionalization. (C) This work: g-C3N4 as the recyclable photoredox catalyst for decarboxylative alkenylation. | |
N-Aryl glycines, a class of natural amino acid derivatives, are widely utilized as pharmaceutical intermediates and a key raw material for generating α-aminoalkyl radicals via decarboxylation, enabling the efficient synthesis of high value-added products (Scheme 1B).7–17 Recently, silver-catalyzed decarboxylation of carboxylic acids has also emerged as a promising strategy for the synthesis of diverse functional molecules.18 Since Rueping et al. pioneered the first visible light-induced g-C3N4-catalyzed decarboxylative alkylation of N-aryl glycines in 2018, the recent advances in this field have opened novel avenues for organic transformations and the synthesis of complex molecules.19,20 Nevertheless, to the best of our knowledge, visible-light-induced g-C3N4-catalyzed decarboxylative alkenylation of N-aryl glycines has not yet been reported. In this study, we have reported g-C3N4 as a recyclable photoredox catalyst which induced the generation of α-aminoalkyl radicals from N-aryl glycines to undergo radical addition with vinyl sulfones for the efficient synthesis of allylamines under visible-light irradiation (Scheme 1C).
Results and discussion
Initially, we employed phenyl vinyl sulfones (1a) and N-phenyl glycine (2a) as substrates to optimize the reaction conditions (Table 1). When 10 mg g-C3N4 was used as a photoredox catalyst, NaHCO3 (2.0 equiv.) as the base in DCM (2 mL), under 15 W 460 nm LED irradiation in air, the desired alkylation product 3a was detected in a trace amount (entry 1). Then, various bases were screened and it was found that CsF was the most effective, enhancing the yield to 53% (entries 2–8). Further screening of solvents indicated that DCM was the optimal medium (entries 9–15). When the amount of g-C3N4 was adjusted to 15 mg, the efficiency of the reaction was not improved obviously (entry 16). However, 3a was obtained in 60% yield with the use of 2 equivalents of 2a (entry 17). Screening of the reaction concentrations demonstrated that 3 mL of DCM resulted in 72% yield (entries 18–20). Finally, the product 3a was obtained in 85% yield by increasing the reaction temperature to 35 °C (entry 21). Controlled experiments confirmed that visible light, g-C3N4 and base were all essential for achieving the desired transformation (entries 22–24).
Table 1 Optimization of the reaction conditionsa

|
Entry |
2a (equiv.) |
Base |
Solvent (mL) |
T (°C) |
Yieldb (%) |
Reaction conditions: 1a (0.2 mmol, 1.0 equiv.), 2a, g-C3N4 (10 mg), base (2.0 equiv.), solvents, under 15 W 460 nm LED irradiation in air for 12 h. Isolated yield. g-C3N4 (15 mg). In the absence of g-C3N4. In the dark. |
1 |
1.5 |
NaHCO3 |
DCM (2) |
25 |
Trace |
2 |
1.5 |
CH3COOK |
DCM (2) |
25 |
Trace |
3 |
1.5 |
AgF |
DCM (2) |
25 |
0 |
4 |
1.5 |
NH4F |
DCM (2) |
25 |
34 |
5 |
1.5 |
InF3 |
DCM (2) |
25 |
Trace |
6 |
1.5 |
KF |
DCM (2) |
25 |
36 |
7 |
1.5 |
NaF |
DCM (2) |
25 |
Trace |
8 |
1.5 |
CsF |
DCM (2) |
25 |
53 |
9 |
1.5 |
CsF |
DCE (2) |
25 |
41 |
10 |
1.5 |
CsF |
MeOH (2) |
25 |
47 |
11 |
1.5 |
CsF |
Toluene (2) |
25 |
50 |
12 |
1.5 |
CsF |
EA (2) |
25 |
44 |
13 |
1.5 |
CsF |
1,4-Dioxane (2) |
25 |
43 |
14 |
1.5 |
CsF |
MeCN (2) |
25 |
47 |
15 |
1.5 |
CsF |
THF (2) |
25 |
48 |
16c |
1.5 |
CsF |
DCM (2) |
25 |
37 |
17 |
2.0 |
CsF |
DCM (2) |
25 |
60 |
18 |
2.0 |
CsF |
DCM (1) |
25 |
51 |
19 |
2.0 |
CsF |
DCM (3) |
25 |
72 |
20 |
2.0 |
CsF |
DCM (4) |
25 |
57 |
21 |
2.0 |
CsF |
DCM (3) |
35 |
85 |
22d |
2.0 |
CsF |
DCM (3) |
35 |
Trace |
23e |
2.0 |
CsF |
DCM (3) |
35 |
0 |
24 |
2.0 |
— |
DCM (3) |
35 |
28 |
Under the optimal conditions, we next explored the substrate scope of vinyl sulfones as shown in Scheme 2. Generally, aryl vinyl sulfones bearing diverse electron-donating and electron-withdrawing groups, such as –F, –Cl, –Br, –CN, –CF3, –Me, –tBu, –OPh, and –OAc on benzene rings at diverse positions, underwent the reaction smoothly, yielding the corresponding products in 70–86% yields (3b–3n). Multi-substituted aromatic vinyl sulfones were also compatible, delivering the products 3o–3q in moderate to excellent yields. Moreover, naphthyl vinyl sulfone was also tolerated to afford 3r in 78% yield. When 1,1-diphenyl vinyl sulfone was utilized as the substrate, trisubstituted alkene 3s was obtained in 84% yield. Furthermore, ester-substituted vinyl sulfone was also employed to achieve the target product 3u in 34% yield. However, the unexpected dimer product 3v was formed when 4-iodophenyl vinyl sulfone was used as the starting material under standard conditions.
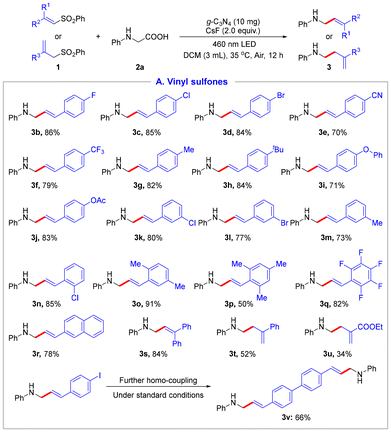 |
| Scheme 2 Substrate scope of vinyl sulfones. Reaction conditions: 1 (0.2 mmol, 1.0 equiv.), 2a (2.0 equiv.), g-C3N4 (10 mg), CsF (2.0 equiv.), DCM (3 mL) at 35 °C under 15 W 460 nm LED irradiation in air for 12 h. | |
Subsequently, a range of diverse N-aryl glycines were explored as shown in Scheme 3. Notably, we varied the steric hindrance or electronic properties of the aryl ring to evaluate the efficiencies, producing the desired products in 21–84% yields (4a–4l). Substituents such as –F (4a), –Cl (4b, 4k), –Br (4c), –I (4d), –CN (4e) and –COMe (4h) at diverse positions of the benzene ring were compatible, allowing the synthesis. N-Aryl glycines with di- or tri-substituents were also accommodated under the optimal conditions to generate 4m–4p in 38–65% yields. In addition, α-methyl or dimethyl substituted N-aryl glycines were effective acceptors, affording the corresponding products in 90% and 47% yields, respectively (4q, 4r). Moreover, N-methyl-N-phenyl glycine was also suitable for the transformation, providing the product 4s in 47% yield. Unfortunately, when N-phenyl-α-phenyl glycine was employed as the substrate, only a trace amount of the product was detected (4t).
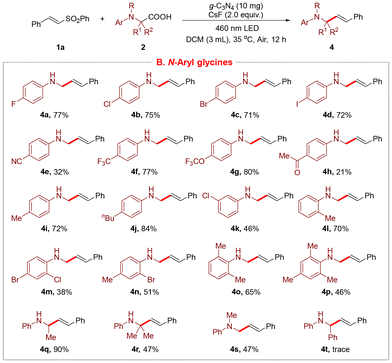 |
| Scheme 3 Substrate scope of N-aryl glycines. Reaction conditions: 1a (0.2 mmol, 1.0 equiv.), 2 (2.0 equiv.), g-C3N4 (10 mg), CsF (2.0 equiv.), DCM (3 mL) at 35 °C under 15 W 460 nm LED irradiation in air for 12 h. | |
To showcase the practical application, we scaled up the model reaction to 5 mmol, and the product 3a was isolated in 61% yield (Scheme 4A). Furthermore, 3a was efficiently transformed into the diene product 5 in 82% yield, underscoring its potential for practical synthetic applications (Scheme 4B). To evaluate the stability and recyclability of g-C3N4, a series of recycling experiments were conducted to assess its performance in multiple reaction cycles. As shown in Scheme 4C, g-C3N4 was successfully recycled and reused in five consecutive reaction cycles without any obvious loss in catalytic performance, highlighting the potential for a sustainable process.
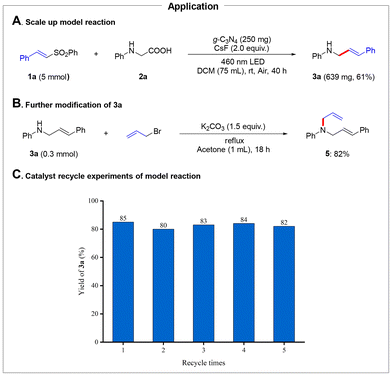 |
| Scheme 4 (A) Scale up model reaction. (B) Further modification of 3a. (C) Catalyst recycle experiments of the model reaction. | |
Next, we performed radical trapping and light on/off experiments to elucidate the possible mechanism. As shown in Scheme 5, the addition of a radical scavenger, such as 2,6-di-tert-butyl-4-methylphenol (BHT), to the standard reaction conditions completely inhibited the formation of product 3a. Moreover, the aminomethyl-BHT adduct was detected by high-resolution mass spectrometry, indicating the probable involvement of a radical species (Scheme 5A). In addition, the results of light on/off experiments suggested that a radical chain pathway was excluded, and product formation was sustained under light irradiation but completely ceased in the absence of light (Scheme 5B).
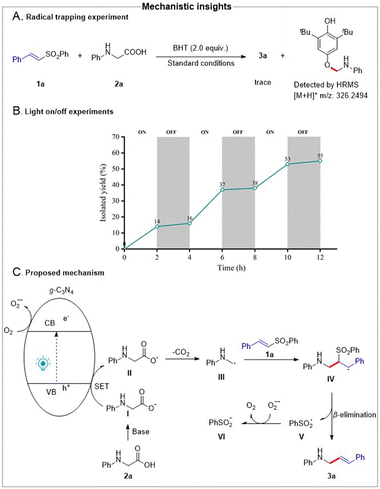 |
| Scheme 5 (A) Radical trapping experiment. (B) Light on/off experiments. (C) Proposed mechanism. | |
Based on the experimental results and precedent literature,18–21 the proposed reaction mechanism is illustrated in Scheme 5C. Initially, under visible-light irradiation, g-C3N4 absorbed photons to generate holes in the valence band (VB) and electrons in the conduction band (CB). Then, oxygen in air was reduced by electrons in the CB to produce the superoxide radical anion. Simultaneously, the deprotonation of 2a proceeded in the presence of base to generate carboxylate anion I, which was oxidized by holes in the VB to form carboxyl radical II. Following the release of CO2 from II, α-aminoalkyl radical III was generated, which underwent radical addition with 1a to form the intermediate IV. The intermediate IV then underwent β-elimination, leading to the desired alkenylation product 3a and phenylsulfone radical V. Finally, the phenylsulfone radical was reduced to the corresponding phenylsulfone anion VI by the superoxide radical anion.
Conclusions
In summary, we have developed an efficient protocol for the decarboxylative alkenylation of N-aryl glycines with vinyl sulfones using recyclable g-C3N4 as a heterogeneous photoredox catalyst. This strategy accommodated a wide variety of N-aryl glycines, demonstrating excellent functional group tolerance and facilitating the rapid synthesis of allylamines. Additionally, this method was scalable to the gram-scale and also provided direct access to valuable diene compounds through straightforward product derivatization, underscoring its practicality and potential application in organic synthesis.
Data availability
The data supporting this article have been included as part of the ESI.†
Conflicts of interest
The authors declare no conflict of interest.
Acknowledgements
We gratefully acknowledge the grants from the National Natural Science Foundation of China (22401081), the Natural Science Foundation of Henan Province (242300420546, 242300421349) and the Start-up Grant of Henan University of Technology (2023BS007, 2024PYJH064). We gratefully acknowledge the financial support from the Distinguished University Professor grant (Nanyang Technological University) and the Agency for Science, Technology, and Research (A*STAR) under its MTC Individual Research Grant (M21K2c0114) and RIE2025 MTC Programmatic Fund (M22K9b0049) for T.-P. L.
References
-
(a) A. Stuetz and G. Petranyi, Synthesis and antifungal activity of (E)-N-(6,6-dimethyl-2-hepten-4-ynyl)-N-methyl-1-naphthalenemethanamine (SF 86–327) and related allylamine derivatives with enhanced oral activity, J. Med. Chem., 1984, 27, 1539 CrossRef CAS PubMed;
(b) L. Mohammadkhani and M. M. Heravi, Applications of Transition-Metal-Catalyzed Asymmetric Allylic Substitution in Total Synthesis of Natural Products: An Update, Chem. Rec., 2021, 21, 29 CrossRef CAS PubMed;
(c) R. Hairani, R. Mongkol and W. Chavasiri, Allyl and prenyl ethers of mansonone G, new potential semisynthetic antibacterial agents, Bioorg. Med. Chem. Lett., 2016, 26, 5300 CrossRef CAS PubMed.
-
(a) M. Johannsen and K. A. Jørgensen, Allylic Amination, Chem. Rev., 1998, 98, 1689 CrossRef CAS PubMed;
(b) P. Evans, R. Grange and E. Clizbe, Recent Developments in Asymmetric Allylic Amination Reactions, Synthesis, 2016, 48, 2911 CrossRef;
(c) R. A. Fernandes, P. Kattanguru, S. P. Gholap and D. A. Chaudhari, Recent advances in the Overman rearrangement: synthesis of natural products and valuable compounds, Org. Biomol. Chem., 2017, 15, 2672 RSC.
-
(a) T. P. Yoon, M. A. Ischay and J. Du, Visible light photocatalysis as a greener approach to photochemical synthesis, Nat. Chem., 2010, 2, 527 CrossRef CAS PubMed;
(b) J. M. R. Narayanam and C. R. J. Stephenson, Visible light photoredox catalysis: applications in organic synthesis, Chem. Soc. Rev., 2011, 40, 102 Search PubMed;
(c) C. K. Prier, D. A. Rankic and D. W. C. MacMillan, Visible Light Photoredox Catalysis with Transition Metal Complexes: Applications in Organic Synthesis, Chem. Rev., 2013, 113, 5322 CrossRef CAS PubMed.
-
(a) N. A. Romero and D. A. Nicewicz, Organic Photoredox Catalysis, Chem. Rev., 2016, 116, 10075 CrossRef CAS PubMed;
(b) T. Y. Shang, L. H. Lu, Z. Cao, Y. Liu, W. M. He and B. Yu, Recent advances of 1,2,3,5-tetrakis(carbazol-9-yl)-4,6-dicyanobenzene (4CzIN) in photocatalytic transformations, Chem. Commun., 2019, 55, 5408 Search PubMed;
(c) W.-M. Cheng and R. Shang, Transition Metal-Catalyzed Organic Reactions under Visible Light: Recent Developments and Future Perspectives, ACS Catal., 2020, 10, 9170 CrossRef CAS.
-
(a) S. C. Yan, Z. S. Li and Z. G. Zou, Photodegradation of Rhodamine B and Methyl Orange over Boron-Doped g-C3N4 under Visible Light Irradiation, Langmuir, 2010, 26, 3894 Search PubMed;
(b) M. Ismael and Y. Wu, A mini-review on the synthesis and structural modification of g-C3N4-based materials, and their applications in solar energy conversion and environmental remediation, Sustainable Energy Fuels, 2019, 3, 2907 Search PubMed;
(c) N. Kumar, M. Kumari, M. Ismael, M. Tahir, R. K. Sharma, K. Kumari, J. R. Koduru and P. Singh, Graphitic carbon nitride (g-C3N4)–assisted materials for the detection and remediation of hazardous gases and VOCs, Environ. Res., 2023, 231, 116149 CrossRef CAS PubMed.
-
(a) H. Ji, F. Chang, X. Hu, W. Qin and J. Shen, Photocatalytic degradation of 2,4,6-trichlorophenol over g-C3N4 under visible light irradiation, Chem. Eng. J., 2013, 218, 183 Search PubMed;
(b) F. Su, S. C. Mathew, L. Möhlmann, M. Antonietti, X. Wang and S. Blechert, Aerobic Oxidative Coupling of Amines by Carbon Nitride Photocatalysis with Visible Light, Angew. Chem., Int. Ed., 2011, 50, 657 CrossRef CAS PubMed;
(c) J. Guo, Y. Wang, Y. Li, K. Lu, S. Liu, W. Wang and Y. Zhang, Graphitic Carbon Nitride Polymer as a Recyclable Photoredox Catalyst for Decarboxylative Alkynylation of Carboxylic Acids, Adv. Synth. Catal., 2020, 362, 3898 CrossRef CAS;
(d) J. Wang, B. Ni, T. Niu and F. Ji, C3N4-Photocatalyzed aerobic oxidative cleavage of C
C bonds in alkynes with diazonium salts leading to two different aldehydes or esters in one pot, Catal. Sci. Technol., 2020, 10, 8458 RSC;
(e) J.-Q. Di, M. Zhang, Y.-X. Chen, J.-X. Wang, S.-S. Geng, J.-Q. Tang and Z.-H. Zhang, Copper anchored on phosphorus g-C3N4 as a highly efficient photocatalyst for the synthesis of N-arylpyridin-2-amines, Green Chem., 2021, 23, 1041 RSC;
(f) B. Ni, B. Zhang, J. Han, B. Peng, Y. Shan and T. Niu, Heterogeneous Carbon Nitrides Photocatalysis Multicomponent Hydrosulfonylation of Alkynes To Access β-Keto Sulfones with the Insertion of Sulfur Dioxide in Aerobic Aqueous Medium, Org. Lett., 2020, 22, 670 CrossRef CAS PubMed. - L. Chen, C. S. Chao, Y. Pan, S. Dong, Y. C. Teo, J. Wang and C.-H. Tan, Amphiphilic methyleneamino synthon through organic dye catalyzed-decarboxylative aminoalkylation, Org. Biomol. Chem., 2013, 11, 5922 RSC.
-
(a) T. Shao, Y. Yin, R. Lee, X. Zhao, G. Chai and Z. Jiang, Sequential Photoredox Catalysis for Cascade Aerobic Decarboxylative Povarov and Oxidative Dehydrogenation Reactions of N-Aryl α-Amino Acids, Adv. Synth. Catal., 2018, 360, 1754 Search PubMed;
(b) Y. Liu, X. Liu, J. Li, X. Zhao, B. Qiao and Z. Jiang, Catalytic enantioselective radical coupling of activated ketones with N-aryl glycines, Chem. Sci., 2018, 9, 8094 Search PubMed;
(c) G. Zeng, Y. Li, B. Qiao, X. Zhao and Z. Jiang, Photoredox asymmetric catalytic enantioconvergent substitution of 3-chlorooxindoles, Chem. Commun., 2019, 55, 11362 RSC;
(d) X. Liu, Y. Yin and Z. Jiang, Photoredox-catalysed formal [3 + 2] cycloaddition of N-aryl α-amino acids with isoquinoline N-oxides, Chem. Commun., 2019, 55, 11527 RSC;
(e) H. Yang, G. Wei and Z. Jiang, Access to Isoxazolidines through Visible-Light-Induced Difunctionalization of Alkenes, ACS Catal., 2019, 9, 9599 Search PubMed;
(f) W. Hu, Q. Zhan, H. Zhou, S. Cao and Z. Jiang, Radical-based functionalization-oriented construction: rapid assembly of azaarene-substituted highly functionalized pyrroles, Chem. Sci., 2021, 12, 6543 Search PubMed;
(g) X. Bai, J. Yao, W. Li, X. Zhao, Y. Yin, S. Yu and Z. Jiang, Enantioselective Hydroaminoalkylation of Azaaryl Ketones through Asymmetric Photoredox Catalysis, Org. Lett., 2024, 26, 5037 CrossRef CAS PubMed.
-
(a) T. Shi, K. Sun, X.-L. Chen, Z.-X. Zhang, X.-Q. Huang, Y.-Y. Peng, L.-B. Qu and B. Yu, Recyclable Perovskite as Heterogeneous Photocatalyst for Aminomethylation of Imidazo-Fused Heterocycles, Adv. Synth. Catal., 2020, 362, 2143 CrossRef;
(b) Y.-F. Si, K. Sun, X.-L. Chen, X.-Y. Fu, Y. Liu, F.-L. Zeng, T. Shi, L.-B. Qu and B. Yu, Arylaminomethyl Radical-Initiated Cascade Annulation Reaction of Quinoxalin-2(1H)-ones Catalyzed by Recyclable Photocatalyst Perovskite, Org. Lett., 2020, 22, 6960 CrossRef CAS PubMed.
- Y. Chen, X. Ye, F. He and X. Yang, Asymmetric synthesis of oxazolines bearing α-stereocenters through radical addition–enantioselective protonation enabled by cooperative catalysis, Org. Chem. Front., 2021, 8, 5804 RSC.
-
(a) W.-T. Ouyang, H.-T. Ji, J. Jiang, C. Wu, J.-C. Hou, M.-H. Zhou, Y.-H. Lu, L.-J. Ou and W.-M. He, Ferrocene/air double-mediated FeTiO3-photocatalyzed semi-heterogeneous annulation of quinoxalin-2(1H)-ones in EtOH/H2O, Chem. Commun., 2023, 59, 14029 RSC;
(b) Y.-H. Lu, S.-Y. Mu, H.-X. Li, J. Jiang, C. Wu, M.-H. Zhou, W.-T. Ouyang and W.-M. He, EtOH-catalyzed electrosynthesis of imidazolidine-fused
sulfamidates from N-sulfonyl ketimines, N-arylglycines and formaldehyde, Green Chem., 2023, 25, 5539 RSC;
(c) Y.-H. Lu, Z.-T. Zhang, H.-Y. Wu, M.-H. Zhou, H.-Y. Song, H.-T. Ji, J. Jiang, J.-Y. Chen and W.-M. He, TBAI/H2O-cooperative electrocatalytic decarboxylation coupling-annulation of quinoxalin-2(1H)-ones with N-arylglycines, Chin. Chem. Lett., 2023, 34, 108036 CrossRef CAS.
- H.-H. Li, J.-Q. Li, X. Zheng and P.-Q. Huang, Photoredox-Catalyzed Decarboxylative Cross-Coupling of α-Amino Acids with Nitrones, Org. Lett., 2021, 23, 876 CrossRef CAS PubMed.
- S. Pan, M. Jiang, J. Hu, R. Xu, X. Zeng and G. Zhong, Synthesis of 1,2-amino alcohols by decarboxylative coupling of amino acid derived α-amino radicals to carbonyl compounds via visible-light photocatalyst in water, Green Chem., 2020, 22, 336 RSC.
- W. Zeng, W. Li, H. Chen and L. Zhou, Relay Photocatalytic Reaction of N-Aryl Amino Acids and 2-Bromo-3,3,3-trifluoropropene: Synthesis of 4-(Difluoromethylidene)-tetrahydroquinolines, Org. Lett., 2022, 24, 3265 CrossRef CAS PubMed.
- L. Dai, Q. Zhu, J. Zeng, Y. Liu, G. Zhong, X. Han and X. Zeng, Asymmetric synthesis of chiral imidazolidines by merging copper and visible light-induced photoredox catalysis, Org. Chem. Front., 2022, 9, 2994 RSC.
- J.-F. Cui, W.-Q. Zhong and J.-M. Huang, Annulation Reaction of Quinoxalin-2(1H)-ones Initiated by Electrochemical Decarboxylation of N-Arylglycines, J. Org. Chem., 2023, 88, 1147 CrossRef CAS PubMed.
- K.-H. Lv, L. Chen, K.-H. Zhao, J.-M. Yang and S.-J. Yan, Cu-Catalyzed Decarboxylative Annulation of N-Phenylglycines with Maleimides: Synthesis of 1H-Pyrrolo[3,4-c]quinoline-1,3(2H)-diones, J. Org. Chem., 2023, 88, 2358 CrossRef CAS PubMed.
- Seminal studies on silver-catalyzed decarboxylation.
(a) Z. Wang, L. Zhu, F. Yin, Z. Su, Z. Li and C. Li, Silver-Catalyzed Decarboxylative Chlorination of Aliphatic Carboxylic Acids, J. Am. Chem. Soc., 2012, 134, 4258 CrossRef CAS PubMed;
(b) F. Yin, Z. Wang, Z. Li and C. Li, Silver-Catalyzed Decarboxylative Fluorination of Aliphatic Carboxylic Acids in Aqueous Solution, J. Am. Chem. Soc., 2012, 134, 10401 CrossRef CAS PubMed;
(c) X. Liu, Z. Wang, X. Cheng and C. Li, Silver-Catalyzed Decarboxylative Alkynylation of Aliphatic Carboxylic Acids in Aqueous Solution, J. Am. Chem. Soc., 2012, 134, 14330 CrossRef CAS PubMed;
(d) C. Liu, X. Wang, Z. Li, L. Cui and C. Li, Silver-Catalyzed Decarboxylative Radical Azidation of Aliphatic Carboxylic Acids in Aqueous Solution, J. Am. Chem. Soc., 2015, 137, 9820 CrossRef CAS PubMed;
(e) L. Cui, H. Chen, C. Liu and C. Li, Silver-Catalyzed Decarboxylative Allylation of Aliphatic Carboxylic Acids in Aqueous Solution, Org. Lett., 2016, 18, 2188 CrossRef CAS PubMed;
(f) Y. Dong, Z. Wang and C. Li, Controlled radical fluorination of poly(meth)acrylic acids in aqueous solution, Nat. Commun., 2017, 8, 277 CrossRef PubMed;
(g) X. Tan, Z. Liu, H. Shen, P. Zhang, Z. Zhang and C. Li, Silver-Catalyzed Decarboxylative Trifluoromethylation of Aliphatic Carboxylic Acids, J. Am. Chem. Soc., 2017, 139, 12430 CrossRef CAS PubMed.
- Y. Cai, Y. Tang, L. Fan, Q. Lefebvre, H. Hou and M. Rueping, Heterogeneous Visible-Light Photoredox Catalysis with Graphitic Carbon Nitride for α-Aminoalkyl Radical Additions, Allylations, and Heteroarylations, ACS Catal., 2018, 8, 9471 CrossRef CAS.
-
(a) Y.-F. Si, X.-L. Chen, X.-Y. Fu, K. Sun, Xi. Song, L.-B. Qu and B. Yu, Divergent g-C3N4-catalyzed Reactions of Quinoxalin-2(1H)-ones with N-Aryl Glycines under Visible Light: Solvent-Controlled Hydroaminomethylation and
Annulation, ACS Sustainable Chem. Eng., 2020, 8, 10740 CAS;
(b) A. Shi, K. Sun, Y. Wu, P. Xiang, I. B. Krylov, A. O. Terent'ev, X. Chen and B. Yu, Oxygen-doped carbon nitride for enhanced photocatalytic activity in visible-light-induced decarboxylative annulation reactions, J. Catal., 2022, 415, 28 CrossRef CAS;
(c) T. Shi, Y.-T. Liu, S.-S. Wang, Q.-Y. Lv and B. Yu, Recyclable Carbon Nitride Nanosheet-Photocatalyzed Aminomethylation of Imidazo[1,2-a]pyridines in Green Solvent, Chin. J. Chem., 2022, 40, 97 CrossRef CAS.
- J. Tian, L. Zhao, C. Yang, C. Yang, L. Guo and W. Xia, Four-Component Synthesis of Spiro-Imidazolidines Enabled by Carbon Nitride Photocatalysis, ACS Catal., 2023, 13, 866 CrossRef CAS.
|
This journal is © the Partner Organisations 2025 |
Click here to see how this site uses Cookies. View our privacy policy here.