DOI:
10.1039/D4RA08493D
(Paper)
RSC Adv., 2025,
15, 2347-2360
Construction of a Bi-doped g-C3N4/Bi2MoO6 ternary nanocomposite for the effective photodegradation of ofloxacin under visible light irradiation†
Received
2nd December 2024
, Accepted 17th January 2025
First published on 24th January 2025
Abstract
Water contamination is a result of the excessive use of antibiotics nowadays. Owing to this environmental toxicity, photocatalytic degradation is the primary approach to non-biological degradation for their removal. In this context, zerovalent Bi-doped g-C3N4/Bi2MoO6 [g-C3N4/Bi@Bi2MoO6] ternary nanocomposite was prepared using the wet impregnation method. Surprisingly, zerovalent Bi is generated simultaneously during the hydrothermal synthesis of Bi2MoO6 without using any additional reducing agent. The performance of the synthesized catalyst for the removal of ofloxacin is measured using visible light radiation. Various techniques like XRD, XPS, DRS, HRTEM, FESEM, etc., were used to characterize the nanocomposites. Additionally, XPS, DRS, and HRTEM confirm the presence of zerovalent Bi. The degradation efficiency was recorded as 82% after 3 h for the optimized catalyst. The control experiments confirm that the superoxide radicals and holes function as reactive entities in the degradation process. HRMS was used to identify the intermediates and various fragments, which support the suggested mechanism. The photocatalyst exhibits outstanding stability and reusability. Due to its stability, easy synthesis, excellent catalytic activity, and reusability, the reported photocatalyst can be considered to be an excellent candidate for photocatalytic pollutant degradation.
1. Introduction
The discharge of effluent containing organic pollutants and toxins into the environment has significant risks for the biosphere and is a serious health threat to humans.1 Among these pollutants are antibiotics, commonly used to treat bacterial infections.2
Among the antibiotics, ofloxacin is a frequently used fluoroquinolone-based drug found in surface water at up to 31.7 μg L−1 concentrations. Even at very low concentrations, ofloxacin can have a detrimental effect on ecological systems by preventing the growth of microorganisms and producing other ecotoxicological effects.3–7 Hence, it is crucial to remove these pollutants from wastewater.8,9 Many methods have been developed so far, including nano-filtration, coagulation, ion exchange, photocatalysis, adsorption, etc. One of the most appealing methods for treating wastewater is photocatalysis as it causes the contaminants to entirely break down rather than merely shifting from one medium to another.10 In recent years, semiconductor catalysed photocatalytic degradation of organic contaminants has grown significantly by consuming solar light energy due to increasing environmental concerns.11–14
Bi2MoO6, an Aurivillius oxide, exhibits interesting physicochemical properties, such as a band gap of nearly 2.7 eV. This unique semiconductor reveals excellent performance under visible light irradiation. Its structure includes [MoO4]−2 alternate sheets with [Bi2O2]+2 layers, distinguishing it from other bismuth-based materials.15 However, because of the low separation efficiency of the photoexcited charge carriers, Bi2MoO6 is insufficiently efficient to meet the requirements of the application.16 This drawback can be avoided by using various approaches like porosity engineering, elemental doping, semiconductor modification by creating heterojunction structures, etc.17 Because of its high stability and low cost, g-C3N4 is a promising material for making semiconductor heterojunctions. Other advantages include strong physicochemical stability, a 2.7 eV band gap, non-toxicity, and a large surface area.10
Furthermore, photocatalytic activity is increased by loading various plasmonic metals due to their localised surface plasmon resonance (LSPR) properties, which help to separate charge carriers and excite electron–hole pairs.18 Bismuth (Bi), a non-noble metal, exhibits a significant LSPR effect along with plasmonic metals (Cu, Ag, Au). One benefit of bismuth over noble metals is its affordable price. This study includes the fabrication of a Bi-doped g-C3N4/Bi2MoO6 ternary composite and examines its photoactivity for the degradation of ofloxacin. To fabricate Bi-doped Bi2MoO6, a hydrothermal method was adopted in which methanol was used as a solvent. Remarkably, Bi(+3) does not need any extra step for the reduction process as methanol itself works as a reducing agent here, reducing Bi(+3) in situ during hydrothermal reaction. The wet impregnation method was used for the successful loading of g-C3N4. The presence of Bi(0) and g-C3N4 can increase the light sensitivity and decrease the charge carriers (e− and h+) recombination. Such characteristics can be beneficial to enhance the photocatalytic activity.
2. Experimental
2.1 Chemicals
Chemical reagents: [Bi(NO3)3·5H2O] 98%, sodium molybdate dihydrate 99% [Na2MoO4·2H2O], urea extra pure 99%, methanol 99.5% extra pure and N-methyl-2-pyrrolidone (NMP) were obtained from Loba Chemie, India. Changshu Hongsheng Fine Chemicals Co. Ltd has provided the absolute 99.9% ethanol. Antibiotic ofloxacin (200 mg) was purchased from Cipla Pharmaceutical, and DI (distilled water) was produced using the Milli-Q Millipore system. The fluorinated tin oxide (FTO) coated glasses were bought from Vritra Technologies (resistance < 10 Ω). Carbon black powder (CB) and polyvinylidene fluoride (PVDF) were purchased from Nanoshell and Sigma Aldrich, respectively.
2.2 Preparation of Bi@Bi2MoO6
Solvothermal method was used to synthesis Bi@Bi2MoO6, 0.25 M of Na2MoO4·2H2O and bismuth nitrate pentahydrate (0.5 M) were mixed in 35 mL of distilled water and 35 mL of methanol respectively and stirred for 2 h. The above mixture was kept in an autoclave of 100 mL at 180 °C for 24 h,19 then centrifuged and dried at 70 °C and will be denoted as BM in this manuscript.
2.3 Synthesis of g-C3N4
To synthesis g-C3N4 sheets using urea as a raw material was prepared by pyrolysis followed by the ultrasonication method. 30 g urea in a crucible was heated at 5 °C min−1 at 500 °C for 2 h then again to 550 °C at 10 °C min−1 for another 2 h. After cooling down naturally, the pale yellow-coloured powder was ground into bulk g-C3N4. The obtained product was dispersed in ethanol for 1 h using ultrasonication to attain g-C3N4 sheets, then the solution was filtered and dried at 50 °C. It will be abbreviated as g-CN in the entire manuscript.20
2.4 Synthesis of g-CN loaded BM nanocomposite
The g-C3N4/Bi@Bi2MoO6 composite was prepared via the wet impregnation method.21 For the preparation, 100 mg of Bi2MoO6 was mixed with the desired quantity of g-C3N4 in 1
:
1 (ethanol and DI water). The solution was kept at stirring for 24 h. Then the product was centrifugated and dehydrated at 50 °C.20 Throughout the manuscript, the 5 and 10 wt% g-C3N4 loaded Bi@Bi2MoO6 composite will be represented as 5GBM and 10GBM respectively.
2.5 Preparation of thin films
The drop-casting method was used to prepare the working electrode for EIS measurement (Nyquist plot). 1 mg PVDF and 50 μL NMP were ground together to form a homogenous paste. Then 1–2 mg carbon black (CB) was added and mixed for an extra 15 min. After that, active material (8 mg) was added to attain a slurry, then it was drop-casted and spread onto the FTO-layered glass and dehydrated for 12 h at 60 °C.
2.6 Photocatalytic activity evaluation
The photocatalytic activity of the synthesised BM and its composite 5GBM and 10GBM were examined using the hazardous pollutant ofloxacin. In a model experiment, an optimized amount (5 mg) of the as-prepared composite was added to a test tube containing 5 mL of ofloxacin solution at a concentration of 20 ppm. To acquire the adsorption–desorption equilibrium, the mixture was stirred for half an hour in the absence of light. Then, for predefined periods, the reaction mixture was exposed to visible light (Wipro Garnet B22-50 W LED having a wavelength of more than 360 nm and light intensity of 5000 lumens). By centrifugation, the catalyst was extracted, and then the concentration of the pollutant was scrutinized using a UV-Vis spectrophotometer. The percentage degradation efficiency was evaluated using the eqn (1): |
 | (1) |
where C0 is the initial concentration and Ct is the concentration at time “t”.
2.7 Characterization technique
To record the crystallographic properties, X-ray diffraction (XRD) was examined by employing a diffractometer of Xpert pro-Cu-Kα (1.54 Å) at 45 kV having a diffraction angle (2θ, 10°–75°). The structural morphology was studied using high-resolution transmission electron microscopy (HRTEM) (JEOL JEM 2100 plus) and field-emission scanning electron microscopy (FESEM) (500 FEG-SEM, Carl-Zeiss Sigma). The energy dispersive spectroscopy (EDS) was examined using BRUKER. JASCO V-750 spectrophotometer was used to measure the DRS (diffused reflectance spectroscopic) of the synthesized samples. The oxidation state was determined using (XPS, Omicron ESCA, Oxford Instrument Germany). The Microtrac Belsorp Mini-II (Bel, Japan, Inc.) was utilized to govern the surface area. The FTIR Spectra were obtained by IR Tracer-100 (Shimadzu). The fragments were studied by high-resolution mass spectrometry (HRMS) using a Waters mass spectrometer (QTOF) with UPLC (XEVO G2 XS) and Ion source Combined APCI and both positive and negative ESI mode to identify the fragments. EIS tests were conducted in the dark using a Biologic (potentiostat, VSP300 at 10 mV, with a 0.1 Hz to 1 MHz frequency range). The three-electrode system comprised a Pt counter electrode, a BM/10GBM-loaded FTO working electrode, and a standard Ag/AgCl reference electrode in 0.5 M Na2SO4 solution. Chemical oxygen demand was measured using Hach COD digestor DRB200, open reflux method followed by APHA 4200 (21 edition).
3. Results and discussion
3.1 XRD studies
The diffraction studies were recorded to analyse the crystallinity and phase purity of the material (Fig. 1). The pattern of g-CN closely matches with the ICDD no. 87-1526 (Fig. 1a).10 A strong peak at 27.5° corresponds to (002) and another peak at 12.7° (100). The minor peak shift of (002) corresponds to the change in interplanar distance between the graphitic nitride sheets.22 Moreover, two extra peaks between the range 15° and 25° are due to the layered structure of g-CN. The peaks at 10.8°, 23.4°, 28.2°, 32.5°, 36.0°, 39.5°, 47.1°, 55.4°, 56.3°, 58.4° and 68.2° associated with (020), (111), (131), (002), (151), (042), (212), (331), (133), (262) and (004) planes respectively, which justified with the ICDD no. 72-1524 (Fig. 1b) of the orthorhombic crystal phase of Bi2MoO6.19 Additionally, peaks with respect to (101), (110), and (024) planes are observed at 23.4°, 39.5°, and 55.4°, respectively, corresponding to the structure of Bi2MoO6 indicating the presence of Bi metal at Bi2MoO6, confirmed with the ICDD no. 44-1246.16 Moreover, the XRD of synthesized composites 5GBM and 10GBM (Fig. 1c) is almost similar to the bare BM, implying that by introducing g-CN, the structural integrity of the BM is not disrupted. Because of the small amount of loading and lower X-ray scattering coefficient of g-CN than pristine BM, there is no g-CN peaks in the composite.10,21
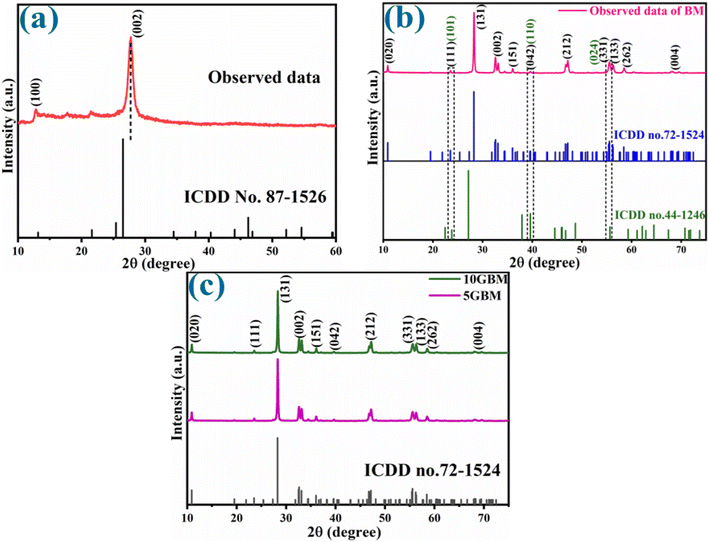 |
| Fig. 1 Diffraction pattern for (a) g-CN (b) pure BM, and (c) as synthesised composites 5GBM and 10GBM. | |
3.2 XPS studies
The chemical composition of the 10GBM composite has been determined using XPS analysis as shown in Fig. 2. The 4f signals of Bi in Bi2MoO6 (Fig. 2b) at 158.5 eV (Bi 4f7/2) and 163.8 eV (Bi 4f5/2) is attributed to trivalent bismuth [Bi(III)]. Additionally, two other peaks at 156.4 eV and 161.7 eV are referred to as zerovalent Bi which validates that methanol reduces Bi(+3) to zerovalent Bi.10 Mo(+5) 3d (Fig. 2c) deconvolutes into two peaks, at 231.7 eV and 234.9 eV relative to Mo 3d5/2 and Mo 3d3/2, respectively.23,24 Typically for O 1s (Fig. 2d), lattice oxygen in the crystal structure is assigned to 529.7 eV and 530.3 eV attributed to oxygen vacancies.25 The XPS spectra of C1s showed four peaks that corresponded to binding energies of 283.9 eV, 286.1 eV, 287.6 eV, and 292.9 eV (Fig. 2e) due to extraneous carbon in the surface, N–C
N bonds, sp2 hybridised carbon of (N)2–C
N heterocyclic ring and C–NH2 respectively.10,26,27 The signals observed at 397.8 eV and 398.7 eV attributed to sp2 nitrogen of triazine rings corresponding to C–N
C and bridged nitrogen atom N–(C)3 respectively (Fig. 2f).28
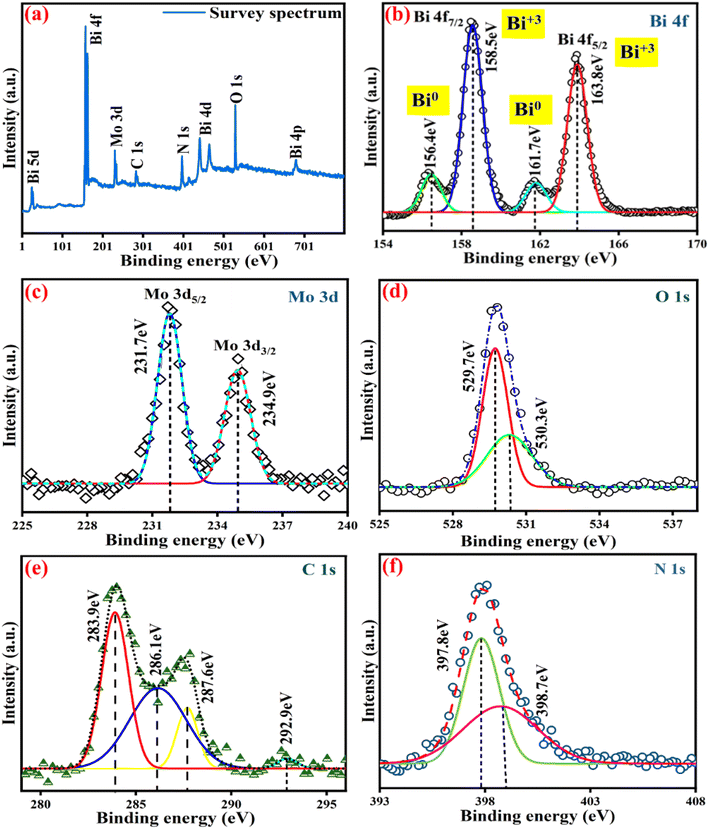 |
| Fig. 2 XPS graphs representing (a) survey diagram (b) Bi [4f] (c) Mo [3d] (d) O [1s] (e) C [1s] and (f) N [1s] of the 10GBM nanocomposite. | |
3.3 Optical studies
Optical studies were examined to determine the bandgap of BM and the synthesised nanocomposites. The Bi2MoO6 and g-CN have an absorption edge value (Fig. 3a) of 490 nm and 470 nm respectively.29,30 A broad absorption band at 500–800 nm in the UV-Vis DRS spectra of BM is referred to as the LSPR effect shown by Bi(0).31 Tauc plot (Fig. 3b and c) indicates that the band gap of BM is 2.55 eV, significantly less than the reported value.19 This is probably because plasmonic Bi is present in the Bi2MoO6 lattice. As g-CN loading increases, the composites' bandgap reduces to 2.40 eV from 2.55 eV, revealing improved absorption of visible light. In contrast, the band gap values at the absorption edge remain practically similar for BM, 5GBM, and 10GBM as shown in Fig. 3c. Such observation is quite similar to the result reported previously12 and reveals that the loading of g-CN over BM enhances the light sensitivity of the prepared composites.
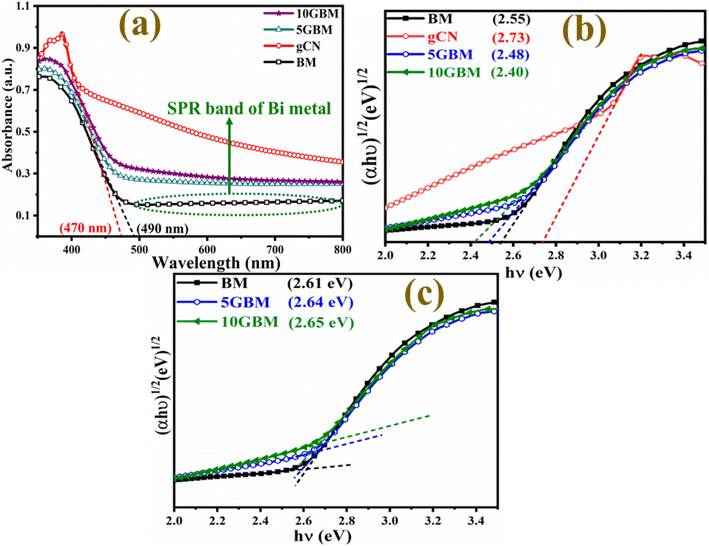 |
| Fig. 3 (a) DRS of BM, g-CN, and all synthesised nanocomposites (b) band gap values and (c) band gap values at absorption edge using Tauc plot. | |
The PL (photoluminescence) experiments' results are indeterminate because the bare BM showed non-fluorescent behaviour.
3.4 FTIR analysis
The synthesised materials were further verified by FTIR analysis. All the nanocomposites 5GBM and 10GBM show peaks of both the bare BM and g-CN (Fig. 4). Several peaks in the region 1100–1700 cm−1 are attributed to the C–N heterocycle. The broad band between 3000 and 3400 cm−1 is due to the N–H and O–H groups of g-CN. At 810 cm−1, the out-of-plane bending mode of the heptazine unit was detected.
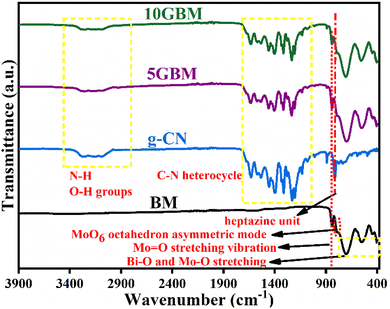 |
| Fig. 4 The FTIR spectra of pristine BM, g-CN and all composites. | |
The bands between 400 and 900 cm−1 are because of the stretching mode of Bi–O, Mo–O, and the bridging mode of Mo–O–Mo. At 790 cm−1, MoO6 octahedron asymmetric mode was observed.32
3.5 EIS analysis
The EIS analysis was carried out to understand the transfer efficiency of photogenerated charge carriers.33 The experiment was conducted in the dark for g-CN as well as bare BM and 10GBM composite (Fig. 5). The g-CN has an extremely small charge transfer resistance due to its high conductivity. As a result, the charge transfer resistance34 of 10GBM composites becomes smaller compared to bare BM as the composite possesses smaller radii in the Nyquist plot compared to its pristine analog, as represented in Fig. 5(b).35 This indicates that incorporating g-CN helps the electron migration from BM to the g-CN surface. Hence, the composites can serve as more proficient photocatalysts than pristine BM.
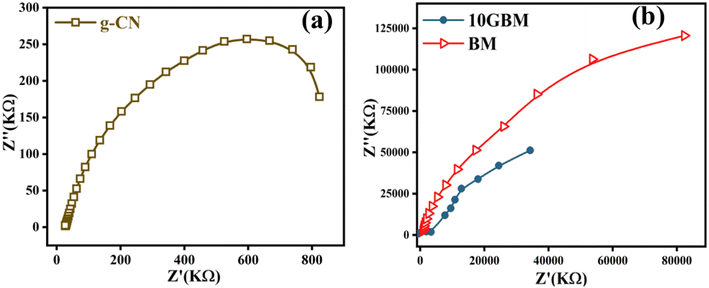 |
| Fig. 5 Nyquist plots of (a) g-CN and (b) bare BM and 10GBM composite. | |
3.6 Morphology
The morphology of bare BM and its 10GBM ternary composite were studied using FESEM shown in Fig. 6. Remarkably, the pristine Bi2MoO6 depicts an irregular crystals-like structure (Fig. 6a and b).36 The presence of both the CN layers and Bi2MoO6 irregular crystals is shown in Fig. 6c and d confirming the development of 10GBM nanocomposite. The elemental mapping (EDS) of the bare BM and nanocomposite 10GBM exhibits every component (Mo, O, Bi, C, and N) elements were uniformly dispersed across the entire surface (Fig. S2 and S3†).
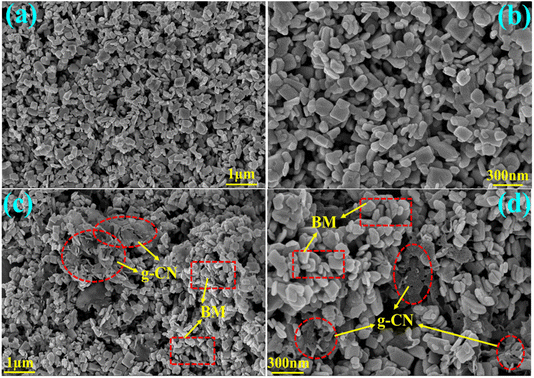 |
| Fig. 6 FESEM images of pristine BM (a and b) and 10GBM nanocomposite (c and d). | |
HRTEM analysis (Fig. 7) was conducted to further examine morphology and crystallinity. The heterostructure consists of g-CN, a sheet-like structure of g-CN, and irregular, disorganized particles of Bi2MoO6 (Fig. 7a).37 In Fig. 7b, the loading of g-CN over Bi2MoO6 is confirmed. The lighter sheet-like structure corresponds to g-CN while the darker square shape is of BM. Moreover, spherical Bi(0) particle deposition on the BMO surface was also detected in Fig. 7c and d. Additionally, the fringes of BMO and Bi-metal (Fig. 7e and f) were also represented which are well matched with the (151) and (101) planes, respectively. The selected area diffraction pattern in Fig. 7g exhibits the spots that are respective to (002), (131) and (202), (024) planes of BMO and Bi-metal, respectively. The observations validate the crystallinity and formation of 10GBM ternary composite.
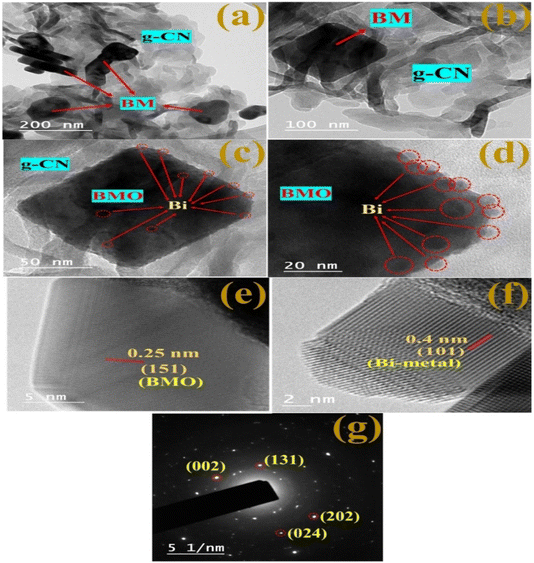 |
| Fig. 7 (a and b) HRTEM micrograph of g-CN and BM, (c and d) showing Bi-metal over BMO and (e and f) lattice fringes (g) selected area diffraction pattern of the synthesised 10GBM ternary nanocomposite. | |
The hydrodynamic particle size of the synthesised catalyst is measured using the DLS technique. The particle size of bare BM and g-CN was observed to be 1099 and 771 nm respectively. As the loading of g-CN increases, the size decreases to 411 nm and 338 nm for 5GBM and 10GBM respectively (Fig. S4†) which is due to more hydrophobicity around the catalyst which reduces the hydrodynamic particle size.
3.7 N2 sorption analysis
BET sorption isotherms in Fig. 8 were determined for pristine BM and the 10GBM heterostructure to explain the difference in surface properties after g-CN loading. The prepared samples exhibit an H3 hysteresis loop and type-III sorption isotherm.38 This indicates the weak adsorbate–adsorbent interaction and implies the occurrence of small pores. Among the two, the 10GBM composite possesses almost double the surface area compared to bare BM due to the incorporation of g-CN which augments the total active sites and resultantly increases the surface area. The total pore volume of bare BM and 10GBM is 1.67 × 10−2 cm3 g−1 and 3.44 × 10−2 cm3 g−1 whereas the pore diameter is 10.07 nm and 10GBM is 11.33 nm, respectively. Such observation suggests that the 10GBM heterostructure is also having higher porosity compared to BM. As a result, 10GBM can act as a superior photocatalyst.
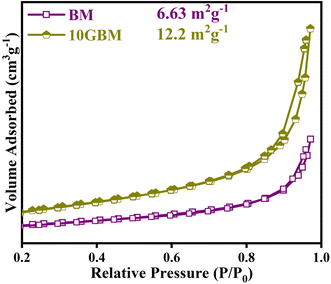 |
| Fig. 8 BET analysis of pristine BM and 10GBM ternary composite. | |
3.8 Photocatalytic activity
The photocatalytic performance of bare BM, g-C3N4, and synthesised composites (5GBM and 10GBM) were examined using visible light (Fig. 9 and S1†). Before irradiating the visible light, the reaction mixture was vigorously stirred in the dark for half an hour to attain the adsorption–desorption equilibrium. The bare BM and g-CN exhibit lower photocatalytic degradation activity in Fig. 9a and b while in the case of ternary nanocomposite (5GBM and 10GBM), upon loading of g-CN on BM photocatalytic activity gradually increases as ∼72(3)% and ∼82(3)% respectively after 3 h (Fig. 9a, b and d). The photodegradation process followed the pseudo-first-order kinetics as depicted in the following eqn (2) (ref. 21) |
 | (2) |
where C0 = initial concentration, C = concentration at the time ‘t’ and ‘k’ = rate constant for pseudo-first-order kinetics.
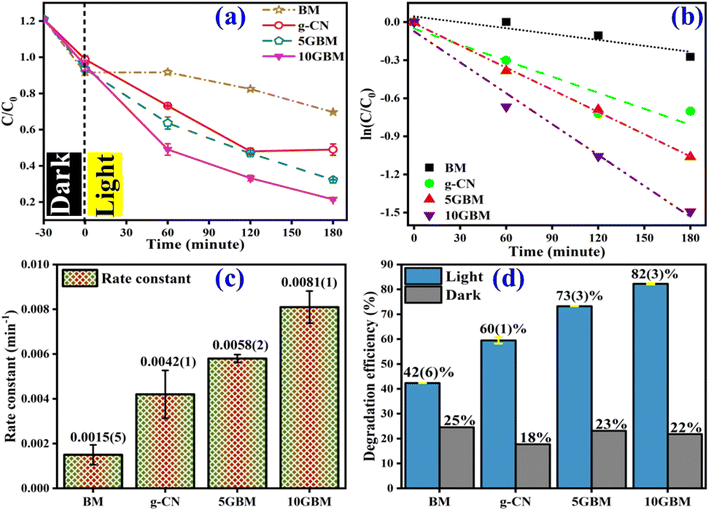 |
| Fig. 9 Photocatalytic deterioration of ofloxacin with time ‘t’ (a), displaying pattern with ln C/C0 at different time period of pristine BM, g-CN and hybrid composites 5GBM and 10GBM (b), rate constant values (c) and bar plot of degradation efficiency in a dark and light environment of all the samples (d). | |
Substantially, a reaction rate enhances on loading of g-CN and the hybrid nanocomposite 10GBM has the maximum rate constant value 0.0081(1) min−1 (Fig. 9c).
To achieve the optimized condition, the effect of reaction time, catalyst amount, and different wt% loading of g-CN was scrutinized (Fig. 10). It was observed in Fig. 10a that on increasing reaction time, the degradation efficiency enhances considerably till 3 h, but after that, no such improvement in the efficiency was observed. It has also been noted (Fig. 10b) that when the catalyst amount increases from 2.5 mg to 5 mg, the degradation efficiency significantly enhances from 47% to 82% due to the greater counts of active sites but with a further increase in the amount, the degradation efficiency remains practically same. Further, evaluated the different wt% loading of g-CN over BM, and it was found that the maximum degradation efficiency was achieved through 10GBM (Fig. 10c), increasing the loading of g-CN above the optimal condition would result in no substantial improvement.
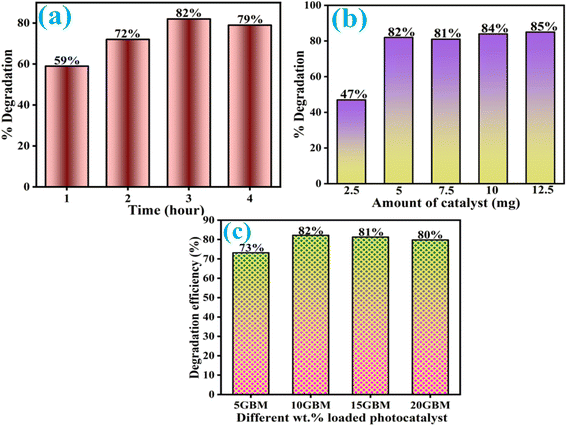 |
| Fig. 10 Optimization of reaction time (a), catalyst dosage (b) and loading of g-CN over BM (c) towards the degradation of ofloxacin. | |
The degree of mineralization was also evaluated along with the degradation studies. Therefore, COD measurements were carried out to study the demineralization of the organic matter39 using the 10GBM composite. The demineralisation efficiency (DE) was evaluated from the following eqn (3)
|
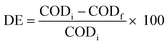 | (3) |
The COD of the reaction mixture was decreased from 164 mg L
−1 to 35.4 mg L
−1, which is attributed to ∼78.4% demineralization efficiency, which is very close to degradation efficiency, suggesting almost complete degradation of a pollutant without the presence of any residual intermediate.
3.9 Mechanistic study
3.9.1 Proposed mechanism. A heterojunction structure of type II (Fig. 11)21,40,41 was constructed on the formation of electron–hole pairs produced by excitation via a band gap to study the proposed reaction mechanism. The following equation calculates the band energy values of BM and g-CN to validate the mechanism42
EVB = energy of the valence band, ECB = energy of the conduction band, X= electronegativity of free electron, Ee = energy of free electron, Eg = band gap energy.
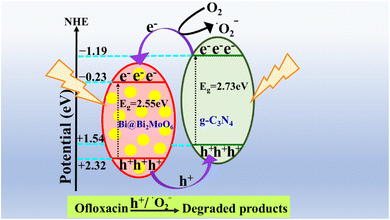 |
| Fig. 11 Representation of the proposed mechanism of type-II heterojunction structure formed by 10GBM photocatalyst. | |
The values of CB and VB of BM and g-CN are −0.23 eV and +2.32 eV (ref. 43) and −1.19 eV and +1.54 eV (ref. 44) respectively. Now, in the 10GBM, the primary reaction occurs in the presence of light irradiation when it falls on the semiconductor. Then the charge carriers (e− and h+) will generate, and electrons (e−) will excite to the CB from the VB and concurrently leave behind the holes (h+) in the VB. Therefore, for the photocatalytic reaction to occur lower redox potential is required so according to the respective (type-II) mechanism, the electrons that are excited to the conduction band of g-CN migrating to the conduction of BM and holes that correspond to the valence band of BM will transfer to the valence band of g-CN, which reduces the recombination of the photogenerated charge carriers (electron–hole pair). Hydroxyl radical production is unfavourable here as the reduction potential is lower than the −OH/˙OH redox couple.10
3.9.2 Scavenger studies. Several control studies were investigated to verify the role of reactive entities (Fig. 12). It was observed that upon the addition of NH4SCN, the degradation efficiency decreased from 82% to 69% and by that of EDTA, it was reduced to 2%, as they function like a hole scavenger so accordingly suppress the oxidation of the pollutant (ofloxacin) with the hole. Additionally, the degradation efficiency was reduced to 28% after Ar gas purging resulting in a lowering of the ˙O2− production by eliminating O2 from a reaction solution. All the conclusions support that the holes (h+) and superoxide radicals (˙O2−) act as reactive species in the degradation mechanism.45,46 However, the degradation efficiency does not alter significantly with the presence of isopropyl alcohol (iPA) which shows that hydroxyl radicals (˙OH) are not participating in the degradation process.
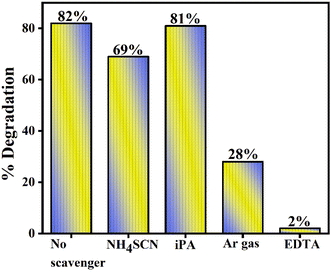 |
| Fig. 12 Effect of Ar purging and NH4SCN, EDTA, and iPA addition on the photocatalytic activity of the 10GBM catalyst. | |
3.9.3 HRMS studies. HRMS analyses were studied to obtain a detailed understanding of the reaction mechanism in Scheme 1 and Fig. S5.† The peak at m/z 362 is the corresponding peak of the protonated form of ofloxacin. Furthermore, the signals at m/z 363 (I1), 158 (I2), 142 (I3), 125 (I4) and 123 (I5) indicate the intermediates formed during the degradation process (marked as I, stands for Identified fragments) and m/z 379 (U1), 365 (U2), 364 (U3) are the Unidentified fragments. It is worth noticeable that with increasing reaction time, the pollutant is degraded a little more into smaller products after half time as showed by the Identified fragments.
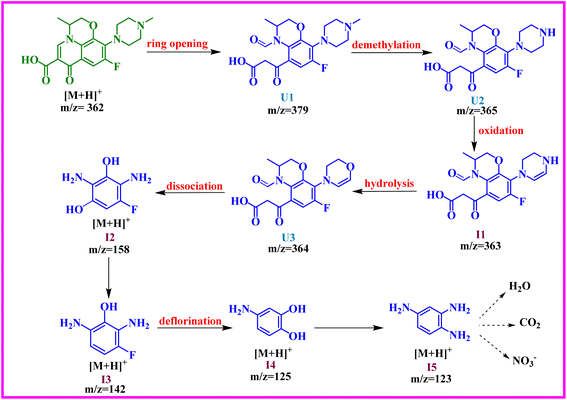 |
| Scheme 1 A plausible mechanism for the degradation of ofloxacin pollutant by 10GBM photocatalyst. | |
3.9.4 Recyclability and stability. For the application of the photocatalysts, their recyclability and stability are essential. The catalyst was extracted from the reaction mixture once it was completed and used in a successive reaction. The degradation efficiency using 10GBM photocatalyst has been found to decrease by just 10% following the fourth cycle when compared to the first (Fig. 13), hence revealing that the composite has great reusability.
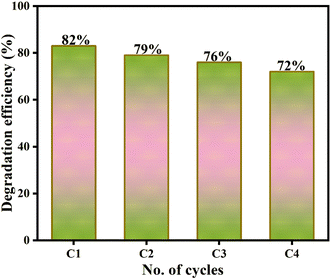 |
| Fig. 13 Reusability using 10GBM composite up to four consecutive cycles of ofloxacin degradation. | |
The apparent reduction in the photocatalytic activity can be due to the catalyst loss during washing and separation.
Moreover, the stability of the composite in Fig. 14 was evaluated using FESEM, FTIR, and XRD after and before the photocatalytic reaction. Notably, before-after spectra of FTIR and XRD are nearly identical (Fig. 14). Additionally, the morphology of the catalyst before and after the reaction remains similar as depicted by FESEM analysis. These outcomes confirm the excellent stability of the 10GBM composite.
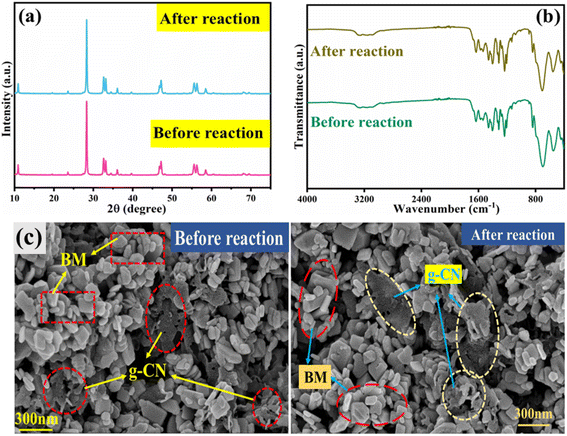 |
| Fig. 14 Before and after reaction XRD and FTIR respectively (a and b) and FESEM (c) of 10GBM photocatalyst. | |
4. Comparison of degradation efficiency
Notably, the 10GBM hybrid catalyst displays either superior or similar photocatalytic performance in the degradation of ofloxacin compared to many reported catalysts as shown in Table 1.
Table 1 Comparative study of degradation efficiency using different catalysts for ofloxacin
S. no. |
Catalyst |
Degradation efficiency |
Light source |
References |
1. |
AgBr/WO3 |
82.5% |
Solar |
47 |
2. |
BiOCl/TiO2 |
70% |
Solar |
48 |
3. |
ZnO/CdS |
73% |
Visible |
49 |
4. |
CdS |
79% |
Visible |
50 |
5. |
CdS/TiO2 |
86% |
Visible |
51 |
6. |
Bi-doped g-C3N4/Bi2MoO6 |
82% |
Visible |
This work |
5. Conclusion
To conclude, this report validates the fabrication of ternary Bi(0)-doped g-C3N4/Bi2MoO6 nanocomposite and evaluates their photodegradation process using the pharmaceutical drug ofloxacin as a pollutant. Substantially, no additional reducing agent is needed to facilitate the formation of photocatalyst as the methanol which serves as a solvent here also plays the role of reducing agent and reduces Bi(III) to zerovalent Bi during the synthesis of Bi2MoO6. Bi(0) is dispersed throughout the Bi2MoO6 structure because of an in situ hydrothermal reaction. The g-C3N4 loading simplifies the process of electron transfer in type-II heterojunction structure. In contrast, the metallic Bi increases the visible light response through the LSPR effect, resulting in better photocatalytic activity in degrading ofloxacin under visible light. The reactive species are holes and superoxide radicals as demonstrated by the controlled experiments. The formation of various intermediates was examined by HRMS, which further provided more clarification to the reaction mechanism. Moreover, the recyclability studies confirmed the catalyst has outstanding stability and reusability. As a result, the current work may pave the way for developing plasmonic element-doped materials intended to remove harmful pollutants from wastewater.
Data availability
The supporting data have been uploaded as part of the ESI.†
Author contributions
Priti Rohilla: writing – original draft, visualization, validation, methodology, investigation, formal analysis, data curation, conceptualization. Bonamali Pal: visualization, supervision, resources, project administration, writing – review & editing, funding acquisition. Raj Kumar Das: writing – review & editing, visualization, supervision, project administration, resources, formal analysis, funding acquisition.
Conflicts of interest
The authors declare that they have no known competing financial interests or personal relationships that could have appeared to influence the work reported in this paper.
Acknowledgements
The authors are thankful to SPMS, TIET for their support with XRD and FESEM analysis, and we are grateful to DST-FIST (Grant number: SR/FST/CS-II/2018/69) for providing the HRMS facility at TIET-Patiala and SPRINT Testing Solution in Mumbai for HRTEM, and XPS analysis. The authors are thankful to Dr O. P. Pandey and Mr Abhishek Chandel for the electrochemical measurements, and Dr Soumen Basu and Mr Suvankar Sau for the BET analysis. Moreover, the authors express gratitude to the TIET-VT Centre of Excellence for Emerging Materials (CEEMS) for FTIR measurements. The authors express gratitude to Mr Samarjit Mandal for providing the ICDD data. The authors also acknowledge Mr Bimalpreet Singh and Dr Amit Dhir for the COD measurements.
References
- S. H. Ammar, F. D. Ali, H. J. Hadi and Z. H. Jabbar, Visible-light-induced photodegradation of ofloxacin antibiotic by facile assembled BiFeO3/Bi-modified g-C3N4 heterojunctions, Mater. Sci. Semicond. Process., 2023, 171, 108026 CrossRef.
- S. Adhikari and D. H. Kim, Synthesis of Bi2S3/Bi2WO6 hierarchical microstructures for enhanced visible light driven photocatalytic degradation and photoelectrochemical sensing of ofloxacin, Chem. Eng. J., 2018, 354, 692–705 CrossRef CAS.
- T. Chankhanittha and S. Nanan, Visible-light-driven photocatalytic degradation of ofloxacin (OFL) antibiotic and Rhodamine B (RhB) dye by solvothermally grown ZnO/Bi2MoO6 heterojunction, J. Colloid Interface Sci., 2021, 582, 412–427 CrossRef CAS PubMed.
- P. Chen, L. Blaney, G. Cagnetta, J. Huang, B. Wang, Y. Wang, S. Deng and G. Yu, Degradation of Ofloxacin by Perylene Diimide Supramolecular Nanofiber Sunlight-Driven Photocatalysis, Environ. Sci. Technol., 2019, 53, 1564–1575 CrossRef CAS PubMed.
- Q. Shang, X. Liu, M. Zhang, P. Zhang, Y. Ling, G. Cui, W. Liu, X. Shi, J. Yue and B. Tang, Photocatalytic degradation of ofloxacin antibiotic wastewater using TS-1/C3N4 composite photocatalyst: Reaction performance optimisation and estimation of wastewater component synergistic effect by artificial neural network and genetic algorithm, Chem. Eng. J., 2022, 443, 136354 CrossRef CAS.
- Q. Su, J. Li, H. Yuan, B. Wang, Y. Wang, Y. Li and Y. Xing, Visible-light-driven photocatalytic degradation of ofloxacin by g-C3N4/NH2-MIL-88B(Fe) heterostructure: Mechanisms, DFT calculation, degradation pathway and toxicity evolution, Chem. Eng. J., 2022, 427, 131594 CrossRef CAS.
- W. Chen, Z. Xing, N. Zhang, T. Cheng, B. Ren, X. Liu, Z. Wang, Z. Li and W. Zhou, Hierarchical Bi2Fe4O9/BiOI S-scheme heterojunctions with exceptional hydraulic shear induced photo-piezoelectric catalytic activity, npj Clean Water, 2024, 7, 86 CrossRef CAS.
- H. Li, W. Zhang and Y. Liu, HZSM-5 zeolite supported boron-doped TiO2 for photocatalytic degradation of ofloxacin, J. Mater. Res. Technol., 2020, 9, 2557–2567 CrossRef CAS.
- K. Rabé, L. Liu, N. A. Nahyoon, Y. Zhang, A. M. Idris, J. Sun and L. Yuan, Fabrication of high efficiency visible light Z-scheme heterostructure photocatalyst g-C3N4/Fe0(1%)/TiO2 and degradation of rhodamine B and antibiotics, J. Taiwan Inst. Chem. Eng., 2019, 96, 463–472 CrossRef.
- P. Rohilla, B. Pal and R. K. Das, Bi-doped g-C3N4/Bi2WO6 ternary composites for superior photocatalytic degradation of reactive orange 16 under visible light irradiation, J. Ind. Eng. Chem., 2025, 141, 456–467 CrossRef CAS.
- J. Wang, L. Tang, G. Zeng, Y. Liu, Y. Zhou, Y. Deng, J. Wang and B. Peng, Plasmonic Bi metal deposition and g-C3N4 coating on Bi2WO6 Microspheres for Efficient visible light Photocatalysis, ACS Sustainable Chem. Eng., 2017, 5, 1062–1072 CrossRef CAS.
- H. Huang, J. Zhao, H. Guo, B. Weng, H. Zhang, R. A. Saha, M. Zhang, F. Lai, Y. Zhou, R. Z. Juan, P. C. Chen, S. Wang, J. A. Steele, F. Zhong, T. Liu, J. Hofkens, Y. M. Zheng, J. Long and M. B. J. Roeffaers, Noble-Metal-Free High-Entropy Alloy Nanoparticles for Efficient Solar-Driven Photocatalytic CO2 Reduction, Adv. Mater., 2024, 36, 2313209 CrossRef CAS PubMed.
- W. Cheng, L. Wang, H. Lao, Y. Wei, J. Xu and B. Weng, Unraveling the Plasmonic Effect of Au in Promoting Photocatalytic H2 Generation and Organic Synthesis, ACS Sustainable Chem. Eng., 2024, 12, 17026–17034 CrossRef CAS.
- H. Zhang, Y. Gao, S. Meng, Z. Wang, P. Wang, Z. Wang, C. Qiu, S. Chen, B. Weng and Y. M. Zheng, Metal Sulfide S-Scheme Homojunction for Photocatalytic Selective Phenylcarbinol Oxidation, Adv. Sci., 2024, 11, 2400099 CrossRef CAS PubMed.
- Q. Liang, M. Zhang, C. Yao, C. Liu, S. Xu and Z. Li, High performance visible-light driven photocatalysts of Bi2MoO6-g-C3N4 with controllable solvothermal fabrication, J. Photochem. Photobiol., A, 2017, 332, 357–363 CrossRef CAS.
- Z. Zhao, W. Zhang, Y. Sun, J. Yu, Y. Zhang, H. Wang, F. Dong and Z. Wu, Bi Cocatalyst/Bi2MoO6 Microspheres Nanohybrid with SPR-Promoted Visible-Light Photocatalysis, J. Phys. Chem. C, 2016, 120, 11889–11898 CrossRef CAS.
- W. Guo, K. Fan, J. Zhang and C. Xu, 2D/2D Z-scheme Bi2WO6/Porous-g-C3N4 with synergy of adsorption and visible-light-driven photodegradation, Appl. Surf. Sci., 2018, 447, 125–134 CrossRef CAS.
- Y. Huang, S. Kang, Y. Yang, H. Qin, Z. Ni, S. Yang and X. Li, Facile synthesis of Bi/Bi2WO6 nanocomposite with enhanced photocatalytic activity under visible light, Appl. Catal., B, 2016, 196, 89–99 CrossRef CAS.
- B. J. Rani, R. Yuvakkumar, G. Ravi, M. Pannipara, A. G. Al-Sehemi and D. Velauthapillai, Bi2MoO6 hierarchical microflowers for electrochemical oxygen evolution reaction, Int. J. Hydrogen Energy, 2021, 46, 18719–18728 CrossRef CAS.
- V. D. Dang, J. Adorna, T. Annadurai, T. A. N. Bui, H. L. Tran, L. Y. Lin and R. A. Doong, Indirect Z-scheme nitrogen-doped carbon dot decorated Bi2MoO6/g-C3N4 photocatalyst for enhanced visible-light-driven degradation of ciprofloxacin, Chem. Eng. J., 2021, 422, 130103 CrossRef CAS.
- P. Rohilla, B. Pal and R. K. Das, Improved photocatalytic degradation of rhodamine B by g-C3N4 loaded BiVO4 nanocomposites, Heliyon, 2023, 9, e21900 CrossRef CAS PubMed.
- D. Y. Liu, J. H. Dong, F. M. Liu, X. F. Gao, Y. Yu, S. B. Zhang, L. M. Dong and Y. K. Guo, Synthesis and photocatalytic performance of g-C3N4 composites, J. Ovonic Res., 2019, 15, 239–246 CAS.
- L. Wang, Z. Liu, D. Wang, S. Ni, D. Han, W. Wang and L. Niu, Tailoring hetero structured Bi2MoO6/Bi2S3 nanobelts for highly selective photoelectrochemical analysis of gallic acid at drug level, Biosens. Bioelectron., 2017, 94, 107–114 CrossRef CAS PubMed.
- F. Xu, J. Wang, N. Zhang, H. Liang and H. Sun, Simultaneously generating Bi quantum dot and oxygen vacancy on Bi2MoO6 nanosheets for boosting photocatalytic selective alcohol oxidation, Appl. Surf. Sci., 2022, 575, 151738 CrossRef CAS.
- J. Bai, X. Li, Z. Hao and L. Liu, Enhancement of 3D Bi2MoO6 mesoporous spheres photocatalytic performance by vacancy engineering, J. Colloid Interface Sci., 2020, 560, 510–518 CrossRef CAS PubMed.
- D. S. Vavilapalli, R. G. Peri, R. K. Sharma, U. K. Goutam, B. Muthuraaman, M. S. Ramachandra Rao and S. Singh, g-C3N4/Ca2Fe2O5 heterostructures for enhanced photocatalytic degradation of organic effluents under sunlight, Sci. Rep., 2021, 11, 19639 CrossRef CAS PubMed.
- Z. Mao, J. Chen, Y. Yang, L. Bie, B. D. Fahlman and D. Wang, Modification of surface properties and enhancement of photocatalytic performance for g-C3N4 via plasma treatment, Carbon, 2017, 123, 651–659 CrossRef CAS.
- Y. Wang, S. Zhao, Y. Zhang, J. Fang, Y. Zhou, S. Yuan, C. Zhang and W. Chen, One-pot synthesis of K-doped g-C3N4 nanosheets with enhanced photocatalytic hydrogen production under visible-light irradiation, Appl. Surf. Sci., 2018, 440, 258–265 CrossRef CAS.
- M. Zhu, S. Kim, L. Mao, M. Fujitsuka, J. Zhang, X. Wang and T. Majima, Metal-Free Photocatalyst for H2 Evolution in Visible to Near-Infrared Region: Black Phosphorus/Graphitic Carbon Nitride, J. Am. Chem. Soc., 2017, 139, 13234–13242 CrossRef CAS PubMed.
- N. Li, L. Zhu, W. De Zhang, Y. X. Yu, W. H. Zhang and M. F. Hou, Modification of TiO2 nanorods by Bi2MoO6 nanoparticles for high performance visible-light photocatalysis, J. Alloys Compd., 2011, 509, 9770–9775 CrossRef CAS.
- M. Sierra, E. Borges, P. Esparza, J. Méndez-Ramos, J. Martín-Gil and P. Martín-Ramos, Photocatalytic activities of coke carbon/g-C3N4 and Bi metal/Bi mixed oxides/g-C3N4 nanohybrids for the degradation of pollutants in wastewater, Sci. Technol. Adv. Mater., 2016, 17, 659–668 CrossRef CAS PubMed.
- E. Vesali-Kermani, A. Habibi-Yangjeh, H. Diarmand-Khalilabad and S. Ghosh, Nitrogen photo fixation ability of g-C3N4 nanosheets/Bi2MoO6 heterojunction photocatalyst under visible-light illumination, J. Colloid Interface Sci., 2020, 563, 81–91 CrossRef CAS PubMed.
- W. Xue, D. Huang, J. Li, G. Zeng, R. Deng, Y. Yang, S. Chen, Z. Li, X. Gong and B. Li, Assembly of AgI nanoparticles and ultrathin g-C3N4 nanosheets codecorated Bi2WO6 direct dual Z-scheme photocatalyst: An efficient, sustainable and heterogeneous catalyst with enhanced photocatalytic performance, Chem. Eng. J., 2019, 373, 1144–1157 CrossRef CAS.
- T. Wang, Y. Bai, W. Si, W. Mao, Y. Gao and S. Liu, Heterogeneous photo-Fenton system of novel ternary Bi2WO6/BiFeO3/g-C3N4 heterojunctions for highly efficient degrading persistent organic pollutants in wastewater, J. Photochem. Photobiol., A, 2021, 404, 112856 CrossRef CAS.
- Y. Su, G. Tan, T. Liu, L. Lv, Y. Wang, X. Zhang, Z. Yue, H. Ren and A. Xia, Photocatalytic properties of Bi2WO6/BiPO4 Z-scheme photocatalysts induced by double internal electric fields, Appl. Surf. Sci., 2018, 457, 104–114 CrossRef CAS.
- D. Chen, Q. Hao, Z. Wang, H. Ding and Y. Zhu, Influence of phase structure and morphology on the photocatalytic activity of bismuth molybdates, CrystEngComm, 2016, 18, 1976–1986 RSC.
- G. Yang, Y. A. Zhu, Y. Liang, J. Yang, K. Wang, Z. Zeng, R. Xu and X. Xie, Crystal defect-mediated {0 1 0} facets of Bi2MoO6 nanosheets for removal of TC: Enhanced mechanism and degradation pathway, Appl. Surf. Sci., 2021, 539, 148038 CrossRef CAS.
- A. Kundu, S. Sharma and S. Basu, Modulated BiOCl nanoplates with porous g-C3N4 nanosheets for photocatalytic degradation of color/colorless pollutants in natural sunlight, J. Phys. Chem. Solids, 2021, 154, 110064 CrossRef CAS.
- S. Singla, P. Devi and S. Basu, Revolutionizing the Role of Solar Light Responsive BiVO4/BiOBr Heterojunction Photocatalyst for the Photocatalytic Deterioration of Tetracycline and Photo electro catalytic Water Splitting, Materials, 2023, 16, 5661 CrossRef CAS PubMed.
- C. Murugan, M. Karnan, M. Sathish and A. Pandikumar, Construction of heterostructure based on hierarchical Bi2MoO6 and g-C3N4 with ease for impressive performance in photoelectrocatalytic water splitting and supercapacitor, Catal. Sci. Technol., 2020, 10, 2427–2442 RSC.
- K. Wu, X. Qiu, Y. Luo and C. Zhang, Oxygen Vacancy Mediated-Bismuth Molybdate/Graphitic Carbon Nitride Type II Heterojunction Chemiresistor for Efficient NH3 Detection at Room Temperature, ACS Sens., 2024, 9, 6698–6708 CrossRef CAS PubMed.
- N. Tian, H. Huang, Y. He, Y. Guo, T. Zhang and Y. Zhang, Mediator-free direct Z-scheme photocatalytic system: BiVO4/g-C3N4 organic–inorganic hybrid photocatalyst with highly efficient visible-light-induced photocatalytic activity, Dalton Trans., 2015, 44, 4297–4307 RSC.
- T. Han, Y. Chen and H. Shi, Construction of a Bi2MoO6/CoOx/Au system with a dual-channel charge transfer path for enhanced tetracycline degradation, Catal. Sci. Technol., 2022, 12, 5565–5574 RSC.
- W. Hu, J. Yu, X. Jiang, X. Liu, R. Jin, Y. Lu, L. Zhao, Y. Wu and Y. He, Enhanced photocatalytic activity of g-C3N4 via modification of NiMoO4 nanorods, Colloids Surf., A, 2017, 514, 98–106 CrossRef CAS.
- B. R. Shah and U. D. Patel, Mechanistic aspects of photocatalytic degradation of lindane by TiO2 in the presence of oxalic acid and EDTA as hole-scavengers, J. Environ. Chem. Eng., 2021, 9, 105458 CrossRef CAS.
- M. Chahkandi and M. Zargazi, New water based EPD thin BiVO4 film: Effective photocatalytic degradation of Amoxicillin antibiotic, J. Hazard. Mater., 2020, 389, 121850 CrossRef CAS PubMed.
- J. Piriyanon, P. Takhai, S. Patta, T. Chankhanittha, T. Senasu, S. Nijpanich, S. Juabrum, N. Chanlek and S. Nanan, Performance of sunlight responsive WO3/AgBr heterojunction photocatalyst toward degradation of Rhodamine B dye and ofloxacin antibiotic, Opt. Mater., 2021, 121, 111573 CrossRef CAS.
- Q. Wang, P. Li, Z. Zhang, C. Jiang, K. Zuojiao, J. Liu and Y. Wang, Kinetics and mechanism insights into the photodegradation of tetracycline hydrochloride and ofloxacin mixed antibiotics with the flower-like BiOCl/TiO2 heterojunction, J. Photochem. Photobiol., A, 2019, 378, 114–124 CrossRef CAS.
- T. Senasu, T. Chankhanittha, K. Hemavibool and S. Nanan, Visible-light-responsive photocatalyst based on ZnO/CdS nanocomposite for photodegradation of reactive red azo dye and ofloxacin antibiotic, Mater. Sci. Semicond. Process., 2021, 123, 105558 CrossRef CAS.
- M. Kaur, S. K. Mehta and S. K. Kansal, Visible light driven photocatalytic degradation of ofloxacin and malachite green dye using cadmium sulphide nanoparticles, J. Environ. Chem. Eng., 2018, 6, 3631–3639 CrossRef CAS.
- A. Kaur, A. Umar, W. A. Anderson and S. K. Kansal, Facile synthesis of CdS/TiO2 nanocomposite and their catalytic activity for ofloxacin degradation under visible illumination, J. Photochem. Photobiol., A, 2018, 360, 34–43 CrossRef CAS.
|
This journal is © The Royal Society of Chemistry 2025 |
Click here to see how this site uses Cookies. View our privacy policy here.