DOI:
10.1039/D4VA00203B
(Tutorial Review)
Environ. Sci.: Adv., 2025, Advance Article
Environmental restoration of polyaromatic hydrocarbon-contaminated soil through sustainable rhizoremediation: insights into bioeconomy and high-throughput systematic analysis
Received
14th June 2024
, Accepted 14th April 2025
First published on 2nd May 2025
Abstract
Polycyclic aromatic hydrocarbons (PAHs) are ubiquitous hydrophobic environmental contaminants with carcinogenic properties. Due to their persistent nature, they can be present in diverse ecosystems, making their extraction and accurate assessment from contaminated environmental samples vital for quantification before implementing remediation strategies. Thus, this review explores the major sources of PAH pollution and their assessment techniques such as SPME, LPME, HF-LPME, and USAEME, which facilitate faster PAH extraction while minimizing the use of organic solvents. In recent years, there has been growing interest in nature-based, eco-friendly soil remediation approaches as compared to chemical and physical approaches. Rhizoremediation has emerged as a leading bioremediation method due to its effectiveness in field applications. However, understanding the interactions between the plant rhizosphere and its microbiome is essential, especially since current research predominantly focuses on in situ bioremediation and degradation of PAH compounds through plant-microbe partnerships. In natural environments, PAHs are present in intricate mixtures, and microorganisms operate within interconnected communities. Thus, this review explores the detailed mechanisms of plant-microbe interactions and the role of advanced omics approaches, including genomics, proteomics, and metagenomics, in enhancing the efficacy of rhizoremediation. Rhizoremediation not only aids in the removal of contaminants but also promotes biomass production, thereby enhancing soil fertility and productivity, leading to improved agronomic results. This article also reviews the ongoing advancements in PAH remediation techniques, evidenced by increased patent filings and innovative approaches, contributing to substantial growth in global bioeconomy revenue. Nevertheless, the widespread adoption of rhizoremediation faces hurdles related to marketing and commercialization. Furthermore, this review delves into strategies such as rhizosphere engineering and genetic modifications aimed at expediting rhizoremediation processes in PAH polluted soils.
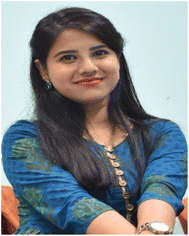 Nandita Das | Dr Nandita Das pursued her PhD in Microbiology at the Department of Microbiology, Assam University, Silchar, India. Her research interests include Environmental Microbiology, Bioremediation, and Environmental and Microbial Genomics. She completed her M.Sc. in Microbiology at Assam University, Assam, in 2016, with first division. She has qualified for several prestigious competitive exams, including the ICAR (Indian Council of Agricultural Research) NET in 2017 and GATE (Graduate Aptitude Test in Engineering) in 2018. She also worked as a Research Fellow on the project “Impact of Rhizosphere-Mediated Bioremediation of Polycyclic Aromatic Hydrocarbons on Different Soil Microbiomes in Contaminated Surface Soil of Oil and Gas Drilling Sites in Assam” (2017–2020). Dr Das has published 11 research papers and book chapters in high-impact international journals. She has also received several awards for oral and poster presentations at international conferences. |
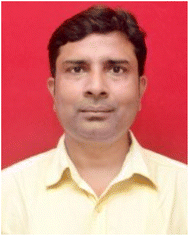 Vijay Kumar | Dr Vijay Kumar is working as an Associate Professor at the Himalayan School of Biosciences, Swami Rama Himalayan University, India. He has over 12 years of teaching and research experience. His research focuses on antibiotics against drug-resistant pathogens, microbial enzyme production, genome analysis, and functional foods. Dr Kumar has made significant contributions to the field, including the discovery of novel actinomycete species and metabolites with antimicrobial properties. His work has been highlighted in leading publications and media. Dr Kumar has published high-impact research papers, supervised several postgraduate and PhD scholars, and secured multiple research grants (2 extramural and 6 intramural). He has received prestigious awards, serves as an editorial board member of reputed journals, and actively contributes to advancing microbiology and food biotechnology through his research and mentorship. |
 Kamlesh Chaure | Dr Kamlesh Choure is serving as a Professor and Head in the Department of Biotechnology, AKS University, Satna (M.P.). He is also acting as the Director, Center for Research Innovation, Incubation and Skill Development (CRIISD), AKS University, Satna (M.P.). He has more than 18 years of teaching and research experience. His area of research includes molecular taxonomy and phylogenetic analysis of bacterial diversity and their application for environmental and agricultural sustainability. He has published several research articles in national and international journals of repute. He is acting as a Principal Investigator of major research projects funded by government funding agencies and also guided doctoral programmes and several postgraduate dissertations. He was awarded the Best Faculty Award 2018 by the MP Private University Regulatory Commission, Govt. of Madhya Pradesh, Bhopal, M.P. (India) on 21 Oct. 2018. He also received the Outstanding Achievement Award of the Society for Bioinformatics and Biological Sciences for the year 2018, in recognition of his contributions to the fields of Biotechnology and Microbiology. In 2020, he was honored with the Biotechnology Excellence Award by the Society of Life Sciences, Satna (M.P.), India, for teaching, learning, research and innovation in the field of biotechnology for rural development. |
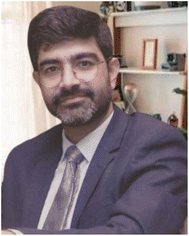 Piyush Pandey | Professor Piyush Pandey, currently affiliated with the Department of Microbiology at Assam University, India, has a distinguished career spanning twenty-four years in academia and research. His research focuses on plant-associated bacteria and their applications in agriculture and environmental sustainability. Prof. Pandey has authored over 160 research and review articles in prestigious journals, along with four books, and has successfully led sixteen research projects. He was awarded the ‘DBT-Centre for Excellence Award’ for his pioneering work on plant-rhizosphere mediated bioremediation of polyaromatic hydrocarbons. Additionally, he has held significant international roles, including as an ‘Overseas Associate’ at Agroscope, Switzerland, SICI-SMP fellow at York University, Canada, and Guest Professor at UGSAS, Japan. Prof. Pandey is a Fellow of the International Society of Environmental Botanists, actively participates in various scientific societies, and serves editorial duties in esteemed journals. His research interests encompass rhizoremediation of environmental pollutants and environmental genomics, and he has supervised more than 20 PhD students. |
Environmental significance
The presence of polycyclic aromatic hydrocarbon (PAH) contamination results in degradation of soil and other ecosystems. PAHs are persistent and ubiquitous in nature and are considered highly carcinogenic, ranking among the most hazardous organic contaminants. Rhizoremediation is a highly effective nature-based technique, using plant-driven remediation mechanisms to restore contaminated air, soil, and water. However, toxic substances in contaminated environments can hinder plant growth and slow remediation, a challenge that can be addressed by introducing an efficient microbial consortium alongside plants. Plant roots provide essential nutrients to microbes in contaminated environments, while microbes, in turn, produce plant growth promoting metabolites and degrade PAHs, preventing their accumulation in plant tissues. This synergistic interaction enhances remediation efficiency.
|
1. Introduction
Polyaromatic hydrocarbons (PAHs) are a class of organic compounds primarily formed as byproducts of the incomplete combustion of fossil fuels such as coal, oil, and natural gas, as well as biomass sources like wood, waste materials, and tobacco.1 Due to their semi-volatile nature, PAHs exist in both gaseous and particulate phases under ambient conditions. They are characterized by their non-polar and lipophilic nature, planar molecular structures, and lack of electrical charge, with many exhibiting no chromatic properties.2 Naphthalene, consisting of two fused aromatic rings, is the simplest PAH. PAHs exhibit a distinctive ability to generate radicals and anions when treated with alkali metals. In environmental contexts, PAHs are typically classified based on the number of fused benzene rings, ranging from two (naphthalene) to seven (coronene) (Karadakov et al., 2023), although PAHs with higher numbers of rings can also be found.3 PAHs are further categorized based on their sources: pyrogenic PAHs result from fossil fuel combustion and are heavily alkylated and oxygenated, leading to the formation of PAH quinones;4 petrogenic PAHs, on the other hand, are associated with crude oil and can enter aquatic environments following oil spills.4
A global study documented a broad spectrum of concentrations for 15 PAH homologues in soils, varying from less than 1 ng g−1 to 7840 ng per g dry weight (dw) of soil.5 Further, in the past decade, PAH concentrations ranging from 10−6 to 10−3 g kg−1 have been observed on almost every continent.6 The highest concentrations were found in Europe, followed by North America, Asia, Oceania, Africa, and South America. Vehicular emissions are recognized as a major anthropogenic source of PAHs in urban environments.7 A study conducted in the United States reported that motor vehicle exhausts contribute approximately 36% of the total annual PAH emissions.8
Elevated levels of PAHs were associated with locations near long-term emission sources and significant atmospheric deposition inputs. Major contributors to PAH emissions include industries such as waste incineration, iron and steel production, coal-tar pitch manufacturing, dye and rubber production, asphalt industries, power generation, and diesel or gasoline-powered machinery.9 Additionally, long-term emissions from exhaust produced by aircraft, ships, trains, and vehicles further contribute to PAH pollution.10 Atmospheric deposition introduces PAH residues into terrestrial and aquatic ecosystems, primarily from fossil fuel combustion and industrial activities.10 A study on the wet deposition of PAHs in Central South China (2014–2017) reported that coal combustion, petroleum sources, and vehicular emissions contributed 58%, 12%, and 30%, respectively.11 Similarly, road vehicle emissions, accounting for 658 metric tons, along with atmospheric deposition, played a significant role in PAH accumulation in Haizhou Bay, China.12 Furthermore, anthropogenic activities emitted nearly 191.5 tonnes of PAH compounds into the atmosphere in Germany.13
Additionally, a positive correlation was observed between microbial population density and PAH concentrations in soil, as well as between soil organic matter (SOM) and black carbon.14 Anthropogenic activities account for approximately 85% of PAH emissions and 99% of related fatalities, with significant disparities in emissions and health effects across different regions.15 Human exposure to PAHs poses substantial health risks, primarily cancer, including skin, lung, bladder, liver, and stomach malignancies, as evidenced by animal studies.16 Additionally, PAH exposure may lead to cardiovascular disease and adversely affect foetal development.17
Biological systems are fundamental to PAH degradation, with plants and microorganisms serving as key contributors due to their diverse metabolic capabilities and pollutant detoxification mechanisms.18 Microorganisms in both terrestrial and aquatic ecosystems exhibit adaptive potential, enabling them to degrade various PAHs over time.19 Rhizoremediation, a bioremediation technique, relies on the mutualistic relationship between plants and microorganisms to sustainably clean environmental pollutants. This process is dependent on plant-microbe interactions with hydrocarbon-degrading capabilities.20 Plant roots offer extensive surface areas for microbial growth and can penetrate soil to depths of 10–15 meters, aiding in contaminant degradation.21,22
In the field of environmental management, bioremediation initially represented only a small segment of the broader market for hazardous waste treatment until the late 1990s. In the United States, the bioremediation industry was valued at approximately $60 million in 1990, which grew to between $175 million and $300 million by 1995.23 Today, bioremediation has significantly expanded, with revenues reaching $46
865.2 million and projected to grow to $333
470.0 million by 2027, according to Emergen Research (2020).24 Bioremediation, along with bioeconomy, provides a common platform for researchers across various disciplines to develop sustainable solutions to environmental challenges.25
This review explores the application of rhizoremediation techniques for the degradation of PAH contaminants and their implications for the global market. It highlights the necessity of developing innovative rhizoremediation strategies to effectively remove PAH pollutants from soil, thereby protecting ecosystems and preserving biodiversity. The review emphasizes the role of plant-microbe interactions in enhancing the remediation of PAH-contaminated soils and discusses advanced molecular approaches aimed at improving rhizoremediation efficiency. Additionally, it provides insights into patent activities within the bioremediation sector, underscoring potential contributions from both governmental agencies and private enterprises in advancing this field.
2. Exposure, toxicity, and assessment of PAH contamination in soil
To date, more than 200 distinct PAHs have been identified in almost every ecosystem.26 Their ubiquity facilitates their adsorption onto suspended particulates in the environment.26 Studies have demonstrated that elevated PAH concentrations in soils and sediments from estuaries, lakes, and marine environments can exert toxic effects on living organisms.27–29 Given their high toxicity, mutagenic potential, and carcinogenic properties, PAHs represent a significant environmental concern. Their toxicity is influenced by factors such as their molecular structure, the biological species exposed, and the specific pathways of exposure.30 Therefore, assessing PAH contamination is crucial for implementing targeted remediation strategies, evaluating toxicity levels, and determining removal efficiency.
2.1 Exposure to PAH compounds
Over 90% of the PAHs present in soils and sediments are predominantly bound to the solid phase, particularly to organic materials. PAHs in soil are retained by soil particles, which reduces their mobility and availability for microbial breakdown.31 PAHs exhibit persistent bioaccumulation and biotransformation characteristics, which contribute to the stability of organic matter components and enhance the resistance of soil-bound PAHs to degradation.32 PAHs present in the air can be deposited into the soil and potentially infiltrate water systems via wet and dry deposition as well as precipitation. Furthermore, PAHs have a high affinity for soil particles and can disperse widely due to their hydrophobic nature.33 They have been detected in remote regions, far from industrial activity, because of their persistence and atmospheric transport. For example, PAHs were found in tropical soils (0.5 to 49 mg per kg dw) and even in Arctic environments, despite the absence of local industrial emissions (186–11,600 mg per kg dw of soil).34 Further, regional economic development, energy production, and population density significantly influence the existence and dispersion of soil PAHs in the environment.35 Implementing a risk assessment strategy identifies potential contaminants and receptors at risk, determining the probability of adverse effects from exposure to specific substances or mixtures in a given area.36 The detected range of 0.6 to 10 mg kg−1 of PAHs in soil signifies varying levels of contamination. PAH-contaminated soils are categorized based on concentration levels as follows: uncontaminated (<0.200 mg kg−1), minimally contaminated (0.200–0.600 mg kg−1), moderately contaminated (0.600–1.000 mg kg−1), and highly contaminated (>1.000 mg kg−1).37 Sites exhibiting PAH concentrations exceeding 10 mg kg−1 are classified as severely contaminated, posing significant risks to agricultural systems by reducing crop productivity, inhibiting seed germination, and decreasing plant longevity.38 Over the past three decades, there has been a substantial escalation in soil PAH concentrations, particularly in industrialized regions worldwide. It is anticipated that these concentrations will continue to rise over the next five years and beyond, primarily due to the ongoing expansion of anthropogenic emissions of PAHs into the environment.39 Soil samples from such industrialised sites exhibit a wide spectrum of PAH contamination levels, with concentrations spanning from 0.001 to 300
000 mg kg−1 of total PAHs.40 In non-industrial regions, PAH contamination is notably concentrated along roadsides, with PAH levels ranging from approximately 0.5 to 49 mg kg−1 of total PAHs.41 Conversely, forested areas typically exhibit lower levels of PAH contamination, with concentrations ranging from approximately 0.2 to 1 mg kg−1 of total PAHs.34 Residential areas tend to exhibit even lower PAH levels, typically falling within the range of approximately 0.1 to 4 mg kg−1 of total PAHs.42
Potential exposure to PAHs may arise via several routes, such as inhalation, ingestion, and direct skin contact. Research conducted on occupational exposure has provided evidence of various adverse health impacts resulting from elevated concentrations of PAHs, such as the onset of carcinogenesis.43 The ingestion of PAHs has the potential to disrupt the normal functioning of cellular membranes and several enzyme systems. The primary focus of PAHs is the potential interaction between the epoxides and dihydrodiols with cellular proteins and DNA, resulting in physiological disturbances, cellular damage, genetic variations, and developmental abnormalities.44 The extended use of industrial effluent combined with the utilization of municipal wastewater for irrigation has resulted in the excessive buildup of PAHs in agricultural soil. Crops cultivated in soil affected by wastewater contamination have the capacity to take up substantial quantities of these pollutants.45 In fact, vegetables in PAH-contaminated soils have been reported to accumulate PAHs, with their concentrations ranging from 508.9 mg kg−1 to 197.3 mg kg−1 in home gardens and 589.9 mg kg−1 to 171.3 mg kg−1 in agricultural fields.46
2.2 Toxicity of PAH compounds
Various PAHs exhibit toxicity, mutagenicity, carcinogenicity, and teratogenicity. Due to their high lipophilicity, PAHs are efficiently absorbed into the gastrointestinal tract of animals and living beings.47 The processing methods, such as smoking, drying, and heating, are the primary cause of contamination by PAHs. The quantity of PAHs in charcoal-grilled meals can reach up to 320 μg kg−1. Seven PAHs have been identified by the Environmental Protection Agency (EPA) as potentially carcinogenic to humans: indeno[1,2,3-cd]pyrene, benzo[b]fluoranthene, benz[a]anthracene, benzo[a]pyrene, benzo[k]fluoranthene, dibenz[ah]anthracene, and chrysene.48 Experiments demonstrated the embryotoxic effects and early pregnancy associated with the several PAHs, including naphthalene, benzo[a]pyrene, and benzo[a]anthracene.49 Benzo[a]pyrene is the first chemical carcinogen to be identified50 and the predominant PAH responsible for inducing carcinogenesis in organisms. The effects of PAHs on aquatic species are determined by their metabolic and photo-oxidation processes, with increased toxicity observed in the presence of UV light. Furthermore, PAHs demonstrate a significant level of acute toxicity towards aquatic organisms and avian lifeforms.51,52
The effects of PAHs on plants include disruption of membrane-related physiological and biochemical processes such as changes in membrane permeability, enzyme malfunction, and interference with photosynthesis.53 PAHs typically distribute into thylakoids, which may affect the chloroplasts and disrupt the electron transport system. The various effects of PAHs on treated plants show that thylakoid membranes accumulate hydrophobic PAHs.54 According to reports, anthracene has been found to have inhibitory effects on the process of carbon fixation, leading to a reduction in net photosynthesis.55 PAHs such as phenanthrene and pyrene, have been observed to have a negative impact on the overall process of net photosynthesis.55 Exposure to phenanthrene and pyrene in higher plants resulted in a reduction in growth, levels of photosynthetic pigments, stomatal conductance, the maximal quantum yield, the effective quantum yield of Photosystem II (PSII), and the photochemical quenching coefficient.56
The presence of PAHs in the top layers of agricultural soils may have an impact on the overall quality of the habitat, resulting in a reduction of its biological characteristics, such as enzyme activity and microbial populations.56 PAHs can easily be absorbed into the soil, causing the aggregation of soil particles and reducing porosity.57 Prolonged exposure to contaminants can have an impact on the geochemical characteristics of soils, including changes in Atterberg limits (a fundamental indicator of the crucial moisture levels in soils with fine particles), permeability, conductivity, and parameters related to strength, consolidation behaviour, compaction properties, infiltration capacity, and shear strength.58 Furthermore, PAH contamination can alter the biological structure of soils, leading to changes in biomass levels and microbial activity. A scientific study reported a significant decline in the relative abundance of microbial phyla, including Alphaproteobacteria, Actinobacteria, Chloroflexi, Crenarchaeota, and Deltaproteobacteria, following exposure to PAH contamination.59
Additionally, the deposition of PAHs onto the soil surface is contingent upon their octanol–water partition coefficient (Kow). A higher Kow value correlates with reduced water solubility of PAH compounds, resulting in an augmented affinity for absorption onto soil particles.60 This partitioning behaviour contributes to the retention and sequestration of PAHs in the soil environment. Soil productivity can decline due to PAH contamination, leading to reduced crop yields, with reports up to a 50% decrease in yields.61 Several consequences of PAH contamination, including biodiversity loss, adverse effects on human health, carcinogenicity, alterations in soil structure and geochemical cycling, increased greenhouse gas emissions contributing to climate change, reduced crop productivity, and disruptions in microbial diversity, are illustrated in Fig. 1.
 |
| Fig. 1 Adverse effects of PAH contamination encompass biodiversity depletion, detrimental impacts on human health, carcinogenic properties, modifications in the soil structure and geochemical cycles, heightened greenhouse gas emissions exacerbating climate change, diminished agricultural yield, and reduction in microbial diversity. | |
2.3 Assessment of PAH compounds
Two advanced analytical techniques, such as microextraction and miniaturized extraction methods, have been employed for the extraction of PAHs from different samples.62 Microextraction, including solid-phase microextraction (SPME), is a single-step, highly sensitive, and efficient method of sample preparation that eliminates the need for solvents, making it suitable for a diverse array of chemicals across multiple matrices.63 Dispersive solid-phase extraction (d-SPE) is a variant of SPME, where the sorbent is introduced directly into the sample's aqueous solution, resulting in dispersion.64 Ambade et al.65 analysed PAH distribution in surface water and sediments from the Damodar River Basin using GC-FID and GC-MS,66 reporting PAH concentrations between 0.036 mg kg−1 and 582 mg kg−1, with acenaphthylene (ACY) and benzo(a)anthracene (BaA) as the most abundant. Xue et al.66 developed a cost-effective method for PAH quantification in soils using ultrasonic-assisted extraction, solid-phase microextraction, and GC-MS. The 100-μm PDMS fiber exhibited superior performance with high repeatability, low detection limits, and a broad linear range, achieving recoveries above 79.3% in spiked samples and proving effective for field analysis of 16 PAHs. Furthermore, among the various liquid-phase extraction (LPME) methods, such as single-drop microextraction (SDME), hollow fibre liquid-phase microextraction (HF-LPME), and ultrasound-assisted emulsification microextraction (USAEME), dispersive liquid–liquid microextraction (DLLME) is the most widely utilised technique.67 The small volume (5 mm3) within the microsyringe, along with the rapid equilibrium between gaseous analytes and the organic solvent film, SDME enabled the use of high vapor pressure solvents like cyclohexane without significant solvent loss during extraction.68 HF-LPME employs disposable polypropylene porous hollow fibers filled with a minimal amount of extracting solvent, known as the acceptor phase.69 The target analytes are extracted by immersing the fibers into the aqueous sample solution, termed the donor phase. Various DLLME techniques have been developed for PAH extraction.70 Rezaee et al.71 introduced a method using tetrachloroethylene and acetone for PAH quantification in surface water, though its reliance on high-density solvents limits efficiency. Guo et al.72 developed a low-density solvent-based demulsification approach using n-hexane and acetone, eliminating the need for centrifugation and reducing extraction time to 2–3 minutes. Hosseini et al.73 explored air flotation-assisted phase separation with toluene, further simplifying the process. The integration of ultrasound and vortex radiation has also improved mass transfer and extraction efficiency by reducing the diffusion distance and increasing the interfacial area. Fernandez et al.74 developed a laboratory-based valve DLLME technique for PAH extraction, followed by analysis using HPLC-FLD, and the automated system improved efficiency, achieving enhancement factors of 86–95%. Further, fabric phase sorptive extraction (FPSE), magnetic solid-phase extraction (MSPE), flow injection solid-phase extraction (FI-SPE), and in-syringe solid-phase extraction of PAHs are novel miniaturized extraction methods of PAHs from different environmental samples.75 FPSE utilizes a fabric substrate coated with a sol–gel organic–inorganic hybrid sorbent as the extraction medium. The coated FPSE medium is first cleaned with solvents and deionized water and then immersed in the sample solution with magnetic stirring to facilitate analyte adsorption.76 After extraction, the FPSE medium is removed, and the analytes are eluted into a vial with an appropriate solvent for analysis following centrifugation or filtration. A trace-level analysis of specific PAHs in environmental water samples utilising FPSE before their quantification by HPLC-FLD has been documented.77 FPSE-HPLC-FLD has been demonstrated to be direct, effective, rapid, sensitive, environmentally friendly, cost-effective, and dependable for the trace level analysis of significant PAHs.78 Furthermore,79 gas chromatography-mass spectrometry (GC-MS), high-performance liquid chromatography (HPLC), and ultra-high-pressure liquid chromatography (UHPLC) in conjunction with various detection techniques such as diode-array detectors (DAD), tandem mass spectrometry (MS/MS) detectors, flame ionization detectors (FIDs), fluorescence detectors (FDs), and ultraviolet (UV) detectors are the most widely applied PAH detection methods.80 Wu et al.81 proposed the use of flow injection solid-phase extraction (FI-SPE) for the extraction of PAHs from environmental samples, employing novel sorbents. Wu's research utilized a micro-column packed with multi-walled carbon nanotubes (MWCNTs) for PAH extraction, followed by gas chromatography-mass spectrometry (GC-MS) analysis. In contrast, Manousi and Zachariadis82 synthesized a copper(II) isonicotinate coordination polymer as a pre-column sorbent, with subsequent high-performance liquid chromatography-diode array detection (HPLC-DAD) analysis. Both methods exhibited efficient extraction performance. For GC-MS detection, eluates from the FI-SPE process were manually injected, whereas for HPLC-DAD analysis, on-line elution in backflush mode allowed direct transfer of analytes into the chromatographic column, streamlining the analytical workflow. Mirzaee and Sartaj83 demonstrated the effectiveness of magnetic granular activated carbon (MGAC) in removing PAHs from contaminated soil via an optimized soil washing process. XRD analysis confirmed the successful incorporation of Fe3O4 nanoparticles onto MGAC. Moreover, SEM-EDX and fluorescence microscopy used for the determination of PAH removal efficiency of Medicago sativa showed a dissipation rate of 96.2%, followed by Helianthus annuus and Tagetes erecta.84 Ma et al.85 employed Fourier transform infrared (FTIR) spectroscopy, X-ray photoelectron spectroscopy (XPS), and thermogravimetric-mass spectrometry (TG-MS) to investigate the composition and structure of soil aggregates (SAs) of varying particle sizes. The study revealed that partitioning played a dominant role in phenanthrene sorption in SAs larger than 0.002 mm, while adsorption was more pronounced in finer SAs (<0.002 mm), which exhibited the highest sorption coefficient (Kd). Lower aqueous equilibrium concentrations of phenanthrene further enhanced adsorption. Morphological and structural analyses indicated that micropores, soil organic matter (SOM), and minerals contributed to PAH sorption, while TG-MS demonstrated that SOM inhibited PAH release during heating. These findings improve the understanding of PAH-soil interactions and interface modelling.
3. Different remediation strategies used for remediation of PAHs from soil
The remediation of PAHs in soil possesses significant environmental challenges, necessitating the application of physical, chemical, and biological approaches. Among the physical remediation techniques, solvent-based soil washing and membrane filtration technologies, including ultrafiltration, microfiltration, nanofiltration, and reverse osmosis, have demonstrated effectiveness in the extraction of PAHs from contaminated soil matrices.86 Thermal methods such as incineration and in situ thermal desorption (ISTD) are also employed to remediate PAH-contaminated soils.87 Incineration is a highly effective strategy that utilizes temperatures between 900 and 1200 °C to eliminate PAHs. Despite its efficiency, incineration is an energy-intensive and costly remediation technique, largely due to the necessity for stringent control measures to manage off-gas emissions.88 Similarly, in situ thermal desorption (ISTD), which involves heating the soil in place to volatilize and remove PAHs, encounters comparable challenges related to high energy consumption and operational costs.89,90 Physical remediation techniques, such as solvent extraction and electrokinetic remediation, are relatively simple to implement and often utilize non-toxic materials.91 However, their major drawback lies in the necessity for repeated treatments to achieve effective contaminant removal. Additionally, these methods frequently require post-treatment measures to manage gaseous byproducts generated during the remediation process.
Chemical remediation of PAH-contaminated soil primarily relies on oxidation processes. Oxidizing agents such as ozone (O3) and Fenton reagents (Fe2+/H2O2) are widely utilized due to their high efficiency in breaking down PAH compounds.92,93 In situ chemical oxidation (ISCO) represents another chemical remediation approach, particularly effective for both low-molecular-weight (LMW) and high-molecular-weight (HMW) PAHs in agricultural soils. ISCO involves injecting oxidants directly into the soil to break down PAHs. Despite its potential, ISCO can also generate toxic secondary intermediates, posing additional environmental risks.94 Moreover, solvent extraction (SE) and soil washing (SW) are widely employed for the remediation of soils contaminated with HMW PAHs. These methods utilize solvents to dissolve and extract PAHs from the soil matrix, facilitating their removal and subsequent treatment. However, due to the hydrophobic nature of HMW PAHs and their strong binding to the soil matrix, SE/SW is not fully effective in removing all PAHs.95 Furthermore, physical and chemical remediation methods for PAH-contaminated environments often fail to achieve complete removal of pollutants. These approaches may also lead to the formation of secondary intermediates, some of which can exhibit greater environmental toxicity than the original PAH compounds.96 These PAH derivatives include oxygen (O-PAHs), nitrogen (N-PAHs and azarenes AZA), or sulphur (PASHs) inside the aromatic ring. The incorporation of oxygen, nitrogen, or sulphur into the aromatic rings of PAHs increases their toxicity.97 Lundstedt et al.98 illustrated that O-PAHs possess greater mobility compared to their parent PAHs, attributed to their polarity. Knecht et al.99 investigated the toxicity of 38 oxygenated PAHs (O-PAHs) on zebrafish embryos (Danio rerio), revealing that structural variations significantly influence toxicity levels. O-PAHs with adjacent diones on 6-carbon moieties or terminal para-diones on multi-ring structures exhibited varying degrees of toxicity, whereas 5-carbon moieties with adjacent diones were the least toxic. The study further demonstrated that the toxicity of selected O-PAHs was differentially dependent on the aryl hydrocarbon receptor (AHR), emphasizing the role of oxidative stress in their toxicological mechanisms. Additionally, B[a]A or benzanthraquinone in zebrafish resulted in detrimental effects on protein biosynthesis, mitochondrial and cardiac function, and neural and vascular development.100 Further, 3-nitrobenzanthrone (3-NBA), a derivative of benzanthrone, demonstrated superior toxicity relative to 1-nitropyrene (1-NP) or benzo[a]pyrene (B[a]P). This substance also caused an increase of cells in the S-phase, followed by the typical process of apoptotic cell death. The presence of 3-NBA triggers a substantial DNA response via the phosphorylation of the ataxia-telangiectasia mutant, checkpoint kinase (Chk) 2/Chk1, H2AX, and p53.101 Similarly, Wang et al. demonstrated through in vitro and in vivo studies that 1-hydroxypyrene, 1-nitropyrene, and 1-methylpyrene, derivatives of pyrene, exhibit greater toxicity to lung health than the parent compound. Derivatives of benzo[a]anthracene, particularly 7-methylbenz[a]anthracene, exhibited the highest tumorigenic activity among the compounds analysed, leading to the development of subcutaneous sarcomas and multiple tumors in the lungs and liver.102 Additionally, 7-bromomethyl-12-methylbenz[a]anthracene demonstrated comparable tumorigenic effects in the lung and liver. In contrast, 4-chloro-7-bromomethylbenz[a]anthracene exhibited minimal activity, with only a slight increase in liver tumor incidence in male mice.103
Bioremediation is considered a highly effective and environmentally friendly method for addressing PAH contamination in soil. It offers numerous benefits, including minimal energy usage, limited secondary pollution, and cost-effectiveness.104 Bioremediation of PAH-contaminated soil utilizes microorganisms, including bacteria, fungi, and plants, to degrade and transform hydrocarbon compounds.105 In recent years, various bioremediation strategies have demonstrated significant efficacy in the removal of PAHs from polluted environments106–109 (Table 1). Guo et al.129 isolated a bacterial consortium from PAH-contaminated soil capable of utilizing pyrene (PYR) as the sole carbon source, achieving 76% degradation in a liquid medium within 10 days. Xiong et al.130 reported that Mycobacterium gilvum, isolated from PAH-contaminated soil, exhibited high efficiency in PYR removal, degrading 98% within just 5 days. Additionally, Zafra et al.131 constructed a bacterial consortium that effectively degraded phenanthrene (PHE), pyrene (PYR), and benzo[a]pyrene (BaP) in soil, achieving 92% removal of PHE, 64% of PYR, and 65% of BaP within 14 days.
Table 1 Different PAH bioremediation techniques and their applications
SI. no |
Bioremediation techniques |
Application |
References |
1 |
Microbial bioremediation |
Uses microorganisms (bacteria, fungi, archaea, and algae) for organic pollutant remediation |
110 |
2 |
Phytoremediation |
Involves plant-based in situ remediation, including methods like phytoextraction, phytofiltration, phytostabilization, phytovolatilization, phytodegradation, rhizodegradation, and phytodesalination |
111–114 |
3 |
Microbe-assisted phytoremediation |
The utilization of a bioremediation technique involves the establishment of a mutualistic relationship between plants and bacteria, such as rhizobacteria and endophytes. This association is employed to augment the effectiveness of remediation processes in contaminated environments |
115 |
4 |
Electro-bioremediation |
This approach employs a hybrid technology that combines bioremediation with electrokinetic mechanisms for the treatment of environmental pollutants. Electrokinetic phenomena play a crucial role in expediting and directing the transportation of environmental contaminants and microorganisms for the purpose of biological remediation |
116 |
5 |
Electrokinetic-phytoremediation |
It is a hybrid technology that combines phytoremediation with electrokinetic remediation. This method increases the metal mobility in polluted soil to facilitate their plant uptake |
117 and 118 |
6 |
Enzymatic remediation |
Utilizes catabolic enzymes to increase the degradation and detoxification of pollutants |
119 and 120 |
7 |
Microbial fuel cells |
The bio-electrochemical device harnesses the capabilities of aerobic microorganisms to efficiently convert organic substrates found in wastewater and other contaminants into electrical energy |
121 |
8 |
Wetland's construction |
Natural treatment involving wetland vegetation and microbes to improve soil quality |
121 |
9 |
Nano-bioremediation |
Integrated method using nanoparticles alongside bioremediation for sustainable remediation |
122 |
10 |
Natural attenuation |
Enhances indigenous microbiome's degradation capacity by improving soil conditions |
123 and 124 |
11 |
Biostimulation |
This technique involves enhancing the indigenous microbial activity in contaminated soil by introducing nutrients, fertilizers, humic acid, organic wastes, etc. These additions serve to stimulate the growth and activities of microorganisms, thereby promoting the remediation process |
125 |
12 |
Bioaugmentation |
The introduction of a highly efficient microbial consortium into soil contaminated with pollutants aims to enhance the degradation of these contaminants and enhance the catabolic capabilities of the existing native microbiome |
110 |
13 |
Composting |
Cost-effective method of increasing soil organic content and fertility, leading to enhanced degradation |
126 |
14 |
Bioreactor |
A controlled ex situ system is utilized, incorporating the use of surfactants, bioaugmentation, and biostimulation techniques for the purpose of bioremediating PAHs |
127 |
15 |
Vermiremediation |
Interactions between plants and microorganisms are employed for the purpose of PAH removal from fine soil with pore sizes smaller than 0.1 mm. PAHs that have accumulated within small pores exhibit limited accessibility for biodegradation. Through their burrowing, earthworms make soil pores bigger, which makes it easier for microbes and plant roots to get into the soil and break down PAHs |
128 |
During the degradation process, these bacteria utilize PAHs as a carbon source for energy production and growth. Specific bacteria, such as Mycobacterium sp., can oxidatively degrade PAHs via the cytochrome P450 monooxygenase enzyme, producing trans-dihydrodiols.132 The phylum Proteobacteria is often predominant in hydrocarbon-contaminated environments.133 Reports indicate that bacterial communities rapidly adapt to fuel contamination by shifting towards hydrocarbon-degrading species.134–137 Utilizing indigenous microorganisms for ecological restoration is a widely adopted method for in situ bioremediation, where pollutants are converted into non-toxic compounds.138 Conversely, ex situ bioremediation techniques, while effective, can be more costly, potentially less environmentally sustainable, and may increase the risk of secondary contamination compared to in situ methods.139
The primary metabolic pathways for the degradation of aromatic compounds begin with the ortho- and meta-cleavage of catechol molecules. Oxygen is essential for several steps in the aerobic degradation of PAHs by bacteria, including ring hydroxylation, ring cleavage, and the final electron uptake.140,141 Pyrene mineralization can occur at the C-1 and C-2 positions or at the C-4 and C-5 positions of the aromatic ring due to the action of dioxygenase enzymes.142,143 Numerous bacteria, including those from the genera Pseudomonas and Rhodococcus, can oxidize PAHs using dioxygenase enzymes.144,145 Several bacteria have been identified to have a bifunctional enzyme encoded by the paaZ gene. This enzyme features an N-terminal aldehyde dehydrogenase domain and a C-terminal enoyl-CoA hydratase domain.146 The dioxygenase enzyme facilitates the breakdown of catechol through several pathways, ultimately leading to the production of succinyl-CoA, which then enters the TCA cycle.147 Metagenomic function profiling indicates a high abundance of key enzymes involved in the central metabolism of petroleum contaminants, including catechol 1,2-dioxygenase, catechol 2,3-dioxygenase, muconolactone D-isomerase, 3-oxoadipate enol-lactonase, and 4-oxalocrotonate tautomerase.110,148 Various species such as Bacillus subtilis, Rhodococcus erythropolis, Ochrobactrum, Hyphomonas spp., and Actinomycete sp. have been identified for their ability to degrade n-alkanes and aromatic hydrocarbons.111,112 The degradation pathways of PAHs in microbes are illustrated in Fig. 3. These pathways involve enzymatic transformations mediated by dioxygenases, monooxygenases, and oxidoreductases, leading to the breakdown of complex PAH structures into less toxic intermediates and ultimately mineralization into CO2 and H2O.
Despite its advantages, on-site bioremediation is often constrained by several limiting factors, including high contamination levels, low nutrient availability, restricted microbial proliferation due to limited soil space, and competition among microbial populations. In such cases, rhizoremediation emerges as an effective strategy by leveraging plant-microbe interactions. Plants facilitate microbial proliferation by supplying nutrients and root exudates, while microbes enhance plant resilience in contaminated environments by degrading pollutants and improving soil conditions.
4. Rhizoremediation as a method for restoring natural ecosystems
According to the UN Environment Programme (2022), the estimated annual global loss of ecosystem services between 1997 and 2011 ranged from €3.5 to €18.5 trillion.149 The primary focus of ecological restoration research has been on community and ecosystem ecology, with a significant emphasis on plant-based restoration efforts. Restoring degraded ecosystems plays a pivotal role in achieving the Sustainable Development Goals (SDGs),149 particularly those related to climate change mitigation, poverty alleviation, and food security. Furthermore, a substantial £14 billion ($19.2 billion) has been allocated by public and private sectors to advance the SDGs, as reported by the United Nations in 2022.150 Therefore, to tackle such environmental consequences, rhizoremediation is acknowledged as an effective approach for ecosystem restoration, resulting in substantial decreases in PAH concentrations, increased microbial degradation activity, and better soil health metrics in diverse polluted environments.151 The synergistic interactions between microorganisms and plants in contaminant remediation represent a nature-based approach for producing environmentally safe end products. This method not only facilitates effective pollutant degradation but also contributes to the restoration of impaired ecosystems, promoting ecological balance and sustainability.152 Bisht et al.153 demonstrated that Bacillus sp. SBER3 degraded 83.4% of anthracene and 75.1% of naphthalene under laboratory conditions, while anthracene degradation in the rhizosphere of Populus deltoides was 45.6%. Liste154 reported that pyrene degradation reached 74% in vegetated soil, compared to 40% in unplanted soil over an eight-week period. Rostami et al.155 further investigated the role of cysteine in enhancing Festuca resilience to PAH-induced stress, demonstrating that pyrene and phenanthrene (200–400 mg kg−1), in the presence of (100–200 mg kg−1) cysteine, exhibited removal efficiencies of 47.78–93.31% and 55.95–98.16%, respectively.
Rhizoremediation, a specialized form of phytoremediation, leverages the symbiotic relationship between plants and microorganisms to environmentally remediate various waste materials.156 The efficacy of this technique hinges on the synergistic interaction between plants and microorganisms capable of degrading pollutants, notably PAHs.157 The extensive root system of plants offers a substantial surface area, promoting microbial proliferation and enabling the degradation of contaminants even at depths of 10–15 meters below the soil surface.158 Plant growth-promoting rhizobacteria (PGPR) are pivotal in rhizoremediation, as they not only aid in pollutant degradation but also enhance plant growth through organic acid and hormone secretion.159 PGPR strains provide resilience to environmental stresses and offer additional benefits such as metal detoxification, nitrogen fixation, and phosphate solubilization.160 The use of PGPR in rhizoremediation enhances pollutant degradation, highlighting the multiple benefits of this practical, non-destructive, and cost-effective approach to environmental remediation.161 Various PAH-contaminated sources and different plant-based remediation mechanisms, including phytovolatilization, phytoaccumulation, phytoextraction, rhizodegradation, rhizoaccumulation, and rhizofiltration, have been illustrated in Fig. 2. Further, Table 2 shows the comparison of different rhizoremediation methods to decontaminate PAH and petroleum hydrocarbons.
 |
| Fig. 2 Illustrations of various PAH-contaminated sources and diverse plant-based remediation mechanisms, including phytovolatilization, phytoaccumulation, phytoextraction, rhizodegradation, rhizoaccumulation, and rhizofiltration. | |
Table 2 Comparison of different rhizoremediation techniques
Remediation type |
Degraded compound |
Microorganism used |
PAH removal efficiency |
Duration (days) |
Advantages |
Limitations |
Field applicability |
References |
Biostimulation and bioaugmentation |
PAHs |
Rhodococcus sp., Achromobacter sp., O. paurometabola, Pantoea sp.,Sejongia sp. Microbacterium sp. |
93% |
56 |
The addition of nutrients to PAH-contaminated soils facilitates an increased rate of biodegradation during the biostimulation process |
Microbial survival and activity depend on environmental factors; may require repeated inoculation |
Applied in crude oil, PAH, and pesticide-contaminated soils |
162–165 |
PAHs |
Rice straw-derived biochar pyrolyzed at 600 °C |
40.00–58.84% |
180 |
PAHs |
— |
73% |
534 |
Alkane PAHs |
Solid inoculants of Bacillus LZ-2 |
21.6% |
30 |
Native microorganisms that are well-suited to the subsurface environment enhanced degradation |
The effectiveness of microbial population density, soil pH, moisture content, temperature, nutrient concentration, soil texture, PAH volatility, chemical structure, concentration, and toxicity are all crucial factors |
Biostimulation aids groundwater, soil, and wastewater remediation and may also facilitate quorum quenching by enhancing signal-degrading bacteria |
Petroleum hydrocarbon |
Bacillus altitudinis strain HRG-1 |
20% |
60 |
PAHs |
Rhodococcus sp. (NH2), Achromobacter sp. (NH13), Oerskovia paurometabola (NH11), Pantoea sp. (NH15), Sejongia sp. (NH20), Microbacterium maritypicum (NH30) and Arthrobacter equi (NH21) |
99% |
30 |
Phytoremediation |
Fluoranthene |
Echinacea purpurea (L.) |
38–60% |
120 |
Effective for organic pollutants like PAHs and hydrocarbons; promotes microbial diversity |
Limited to biodegradable contaminants; slow degradation rates in some cases |
Widely used for petroleum-contaminated soils and pesticide degradation |
166–169 |
Pyrene |
Benzo(a)anthracene |
Chrysene |
Benzo(b)fluoranthene |
Benzo(k)fluoranthene |
Benz(a)pyrene |
Dibenz(a,h)anthracene (PAH) |
Phenanthrene, pyrene |
Wheat |
98% |
90 |
PAH and crude oil |
T. arundinacea |
64% |
90 |
PAHs |
Mimosa, Zinnia, gazania, Cypress vine |
45–49% |
|
Rhizofiltration |
Cd and Pb |
Brassica juncea |
48–59% accumulation |
— |
Efficient for removing heavy metals; applicable for wastewater treatment |
Requires proper plant disposal after pollutant accumulation; limited to waterborne contaminants |
Used in wetlands, constructed treatment systems, and contaminated aquatic environments |
170–172 |
Al, Fe and Mn |
Pistia stratiotes L. |
90% |
15 |
Cd and As |
Cynara cardunculus |
10% |
30 |
Phytovolatilization |
TCE (trichloroethylene) |
Eucalyptus |
0.97 flux (μmol m−2)b |
45 |
Effective for volatile organic pollutants and heavy metals like mercury |
Risk of atmospheric recontamination; limited to specific pollutants |
Used for chlorinated solvents and heavy metal volatilization |
173 and 174 |
TCE (trichloroethylene) |
Poplar |
0.1 (μmol m−2)b |
5 |
Naphthalene |
Poplar |
2.8 (summer) (μmol m−2)b |
— |
Rhizoremediation |
Trichloroethylene (TCE) |
Wheat |
63% |
36 |
Increases biodegradation efficiency; can target specific contaminants |
Microbial survival and activity depend on environmental factors; may require repeated inoculation |
Applied in crude oil, PAH, and pesticide-contaminated soils |
|
PAHs |
Brachiaria serrata and Eleusine coracana |
63–96% |
70 |
175–177 |
PAHs |
Phragmites australis and alfalfa (Medicago sativa) |
68.7–74.5% |
2 years |
Phenanthrene and pyrene |
Wheat |
87–97% and 65–70% |
— |
4.1. Plant-microbe interaction to degrade PAHs in soil
Sayyed et al.161 highlighted the stimulation of microbial activity by root exudates, a phenomenon termed the “rhizospheric effect,” which enhances the degradation of organic compounds by plants. The rhizosphere plays a pivotal role in facilitating crucial processes such as primary and secondary metabolism, as well as the establishment, survival, and ecological interactions with other organisms.178 Plants in contaminated sites stimulate the accumulation of rhizospheric microorganisms near their roots through the release of nutrient-rich root exudates.179 This microbial aggregation supports plant growth and promotes a healthy root system in contaminated environments.180
Hou et al.181 conducted a study where they observed an increase in the biomass of Festuca arundinacea L. and the degradation of PAHs in oil-contaminated soil. This was achieved through bioaugmentation using bacteria that have plant growth-promoting properties and the ability to produce biosurfactants. Moreover, the concentration of bacteria specialized in the degradation of PAHs was observed to be significantly greater in the rhizosphere region of plant species in comparison to soil sites devoid of plant presence.182 Sampaio et al.183 showed that bacterial sp., Bacillus sp. and Pseudomonas aeruginosa effectively colonized the roots ofRhizophora mangle L. In addition to colonization, these bacterial strains played a crucial role in providing protection to the plant, enhancing propagule germination, and achieving the degradation of over 80% of PAHs present in sediment. In a field study conducted on a former coal mine site, researchers evaluated the ability of different legume tree species, including Cassia siamea, Albizia lebbeck, Delonix regia, and Dalbergia sissoo, to lower soil PAH levels. According to the findings, the degradation rates among the tested trees ranged from 51.5% to 81.6%.184 The rhizosphere of Zea mays (maize) and Sorghum sudanense (Sudan grass), cultivated in the presence of benzo[a]pyrene (BaP) and pyrene (PYR), along with Stenotrophomonas sp. MAL1, Arthrobacter sp. MAL3, and Microbacterium sp. MAL2, showed complete PYR degradation and 38.7% BaP degradation over 10 to 14 days, when supplemented with low molecular weight organic acids (LMWOAs).185 Further, Singha et al.186 demonstrated the effectiveness of rhizoremediation in pyrene-contaminated soils through interactions between Oryza sativa (rice) plants and microbial consortia. A bacterial consortium of Klebsiella pneumoniae AWD5 and Pseudomonas aeruginosa PDB1 achieved 60% pyrene degradation. Similarly, Kotoky and Pandey182 investigated the rhizodegradation of benzo[a]pyrene (BaP) using a bacterial consortium (Bacillus subtilis SR1, Serratia marcescens S2I7, andStaphylococcus arlettae S1I1) in the rhizosphere of Melia azedarach. BaP degradation reached 88% in the rhizosphere after 60 days, compared to 68.22% in bulk soil. In a separate study, Bacillus flexus S1I26 and Paenibacillus sp. S1I8 enhanced benzo[a]pyrene (BaP) solubilization by 24.41%, with pot trials, demonstrating even higher rhizosphere degradation efficiencies of 87.42% and 86.08%, respectively.187 Mukhopadhyay and Masto188 evaluated PAH degradation in coal mine sites, where Cassia siamea achieved the highest PAH reduction (81.6%), followed by Albizia lebbeck (55.6%), Delonix regia (51.9%), and Dalbergia sissoo (51.5%). Somtrakoon et al.189 evaluated the impact of plant growth regulators on the phytoremediation potential of sweet grass (Pennisetum purpureum cv. Mahasarakham) in PAH-contaminated soil. The presence of sweet grass led to reductions in acenaphthylene (4.69 ± 0.50%), acenaphthene (10.69 ± 1.47%), and phenanthrene (3.61 ± 0.07%), whereas unplanted soil showed PAH reductions exceeding 30%, in field studies. Zhao et al.190 further investigated the role of an indigenous BaP degrader, Stenotrophomonas BaP-1, in the ryegrass rhizosphere. Following bioaugmentation, the residual BaP mass in ryegrass and bioaugmented microcosms was 2.38 ± 0.10 mg kg−1 and 2.33 ± 0.07 mg kg−1, respectively. Additionally, the degradation rates of Σ15PAHs after 45 days ranged from 32.80% to 74.35% with the application of the consortium to alfalfa plants. Similarly, Gawryluk et al.191 observed an 8% increase in phenanthrene elimination in the rhizosphere after 14 days of ryegrass cultivation. Pyrene degradation in the rhizosphere of Festuca arundinacea (tall fescue) was 8.85–20.7% higher than that in non-plant soils. Dai et al.107 reported a 21.8–28.0% increase in ∑PAH elimination (pyrene, chrysene, benzo(b)fluoranthene, and benzo(k)fluoranthene) in the rhizosphere of ryegrass as compared to unplanted soils.
Root-associated niches play a crucial role in pollutant dissipation, with phenanthrene and pyrene removal decreasing from the rhizoplane to the rhizosphere and near-rhizosphere soil (0–8 mm) after 40–50 days of ryegrass growth.192 Moreover, rhizosphere activity significantly enhances the degradation of freshly spiked PAHs compared to weathered PAHs.193 For instance, a phenanthrene dissipation rate of 86% was observed in the rhizosphere just one week after wheat planting.194 Additionally, the elimination rates of phenanthrene in the rhizosphere of Lolium perenne exceeded those of Elsholtzia splendens under low copper (Cu) treatment. However, Elsholtzia splendens demonstrated higher phenanthrene degradation under elevated Cu treatment.195 Examples of efficient plant-microbe pairs utilized for the degradation of PAH compounds are listed in Table 4.
Plant growth-promoting microorganisms (PGPMOs) have gained recognition for their substantial influence on the rhizosphere environment. They augment the production of growth-promoting hormones, enzymes, siderophores, biosurfactants, and ACC deaminase.196,197 Moreover, there are microorganisms referred to as “superbugs” capable of biodegrading a diverse array of contaminants. Consortia of microorganisms, wherein individual strains fulfil complementary functions, also exhibit advantageous characteristics. The rhizosphere effect, driven by the secretion of PAH compounds, biosurfactants, and organic molecules, positively influences microbial diversity, activity, and the rhizoremediation process.198 Biosurfactants, amphiphilic molecules produced by microorganisms, form micelles in the presence of hydrophobic PAHs, increasing their bioavailability and promoting biodegradation.199 Microbes generate organic acids, which lower soil pH and enhance PAH solubility. Additionally, enzymatic synthesis of degradative agents, like oxidoreductases, significantly boosts the degradation process.200 The application of Verbascum sinuatum L. and a microbial consortium effectively remediated polluted soils, reducing PAHs and 6-ring compounds by up to 68%.201 Mehmannavaz et al.202 found that the introduction of Sinorhizobium meliloti strain A-025, a rhizobacterium that forms a symbiotic relationship with alfalfa and fixes nitrogen, increased the conversion of several polychlorinated biphenyls (PCBs). A wide range of bacterial genera, including Pseudoxanthomonas, Burkholderia, Mycobacterium, Prevotella, Cellulomonas, Actinobacillus, Anaeromyxobacter, Paraburkholderia, Sphingomonas, Novosphingobium, Acetivibrio, Acetobacter, Cycloclasticus, Microbulbifer, Gordonia, and Micrococcus, have been identified as involved in the degradation of PAHs in the rhizosphere.203–206
4.2. Role of plant-root exudates in shaping microbial diversity in PAH contaminated soil
The presence of root exudates plays a crucial role in shaping the composition and population of microorganisms in the rhizosphere, while also playing an essential role in the growth and development of the rhizosphere.207 The chemical composition of root exudates is influenced by the specific type of plant and various environmental factors.208 Root exudates play a crucial role in regulating the soil rhizosphere microbiome, promoting beneficial symbiotic interactions, suppressing the growth of competitive organisms, and enhancing the chemical and physical conditions of the soil.209 Root exudates can be categorised into different groups depending on their chemical composition, such as passive root exudates, root tissue lysates, mucilage chemicals, and secondary metabolites.210
Plants develop various interactions, both beneficial and harmful, through the release of root exudates. These interactions influence relationships among different plants as well as interactions with microorganisms, shaping the rhizosphere environment and affecting processes like nutrient cycling, microbial colonization, and contaminant degradation.211 Soil amended with root exudates containing high concentrations of organic acids has been found to have a reduced capacity for absorbing organic pollutants.182,212 Rajkumari et al.213 found that the application of certain substances, such as organic acids, glucose, and serine, can significantly improve the degradation of PAHs. The presence of glucose in the root exudates triggers the production of dehydrogenase enzyme, which aids in the breakdown of pyrene and promotes the growth of Mycobacterium sp. A study conducted by Jin et al.214 found that Arabidopsis plants with enhanced phenolic exudate secretion experienced significant changes in the microorganisms present in the rhizosphere. The biodegradation of phenanthrene was observed to be most effective within a distance of 3 mm from the roots, with a degradation rate of 86%. However, the degradation rate decreased to 48% at a distance of 3–6 mm and further declined to 36% at a distance of 6–9 mm. There is a positive relationship between the proximity to the roots and the abundance of heterotrophs and PAH-degrading bacteria. In the rhizosphere of perennial ryegrass (Lolium perenne L.) grown in soil contaminated with petroleum hydrocarbons, a majority of the hydrocarbon degraders were found within a 3 mm distance.215 Muungo216 showed that Pseudomonas and Arthrobacter exhibited the highest levels of activity in degrading phenanthrene. Interestingly, this was observed both in the presence and absence of artificial root exudates. The area surrounding the roots of perennial ryegrass, known as the rhizosphere, exhibited the highest levels of microbial activity and contamination.217 The microbial community responsible for phenanthrene degradation shifted when ryegrass exudates were applied. Initially, Pseudoxanthomonas spp. and Microbacterium spp. were identified as the main phenanthrene degraders. However, after the application of ryegrass exudates, the dominant species changed to Arthrobacter spp. and Pseudomonas stutzeri.218 A variety of microbial species have the ability to utilise both root exudates and hydrocarbons as their source of carbon.218 Table 2 shows the different plant-microbe pairs to degrade PAH in soil. Yergeau et al.219 found that key bacterial groups, including Alphaproteobacteria, Betaproteobacteria, Gammaproteobacteria, and Acidobacteria, exhibited increased activity in the willow rhizosphere, while most showed reduced activity in bulk soil. Additionally, fungi such as Basidiomycota, Ascomycota, and Glomeromycota were notably more active in the rhizosphere than in bulk soil. Microbial taxa in the rhizosphere exposed to PAHs became dominant, with Eurotiomycetes increasing from 20.6% to 52.2% and Eurotiales from 20.4% to 51.8%, suggesting their role as primary PAH degraders.107 Rhizosphere stimulation varied significantly depending on soil pollution levels,219 and it enhances PAH-degrading bacterial populations in the rhizosphere compared to bulk soil.219 Bacterial taxa such as Sphingobacteriia and Actinobacteria, along with genera including Pseudomonas, Rhizobium, Sphingomonas, Ilumatobacter, Singulisphaera, and Ensifer meliloti, exhibit increased relative abundances in the rhizosphere of ryegrass and lucerne.220 DNA-SIP studies confirm their role in PAH degradation, as demonstrated by 13C-PAH-labeled components.218,221 Additionally, certain indigenous herb species from coking facility soils promote PAH-degrading bacterial communities like Sphingomonas, Pedomicrobium, and Pseudomonas in the rhizosphere.222 Research indicates that over 90% of phenanthrene and more than 60% of pyrene were eliminated from planted soils after 40 to 80 days of development, surpassing the removal levels in non-planted soils.223 For instance, phenanthrene removal efficiency in the rhizosphere increased by 8% following 14 days of ryegrass cultivation,221 while pyrene removal efficiency in the rhizosphere of tall fescue (Festuca arundinacea) was elevated by 8.85% to 20.7% compared to non-planted soils.223
Huang et al.224 found that different subspecies of Arabidopsis release unique exudates that specifically target the microbiome in the rhizosphere. These exudates play a significant role in altering the microbial community in the root zone. Kimani et al.225 showed that the phenolic substance has the ability to alter the microbial composition, distinguishing it from other compounds like sugar and carbohydrates. In addition to phenolic compounds, the exudates from cucumber roots, specifically p-coumaric acid and vanillic acid, have been found to impact the microbiome in the rhizosphere, leading to alterations and increased abundance.226 Certain compounds found in root exudates have the ability to imitate the signalling molecules known as bacterial quorum-sensing N-acyl homoserine lactones (AHLs).227 These compounds play a role in regulating the interaction between plants and microbes. Additionally, the bacterial functions in the host plant can be regulated by inducing the gene for host infection, promoting the production of biofilms and biosurfactants, enhancing nitrogen fixation, and increasing the production of degrading enzymes.228 Some plant species, like Coronilla varia, Pisum sativum, and Oryza sativa, release compounds similar to AHLs found in certain microbes. These compounds help these plants regulate the population of microbes in the rhizosphere, while also repelling others.229 Enzymes like peroxidase, laccases, and phenol oxidases produced by plants play a crucial role in the oxidation of hydrocarbon products into intermediate derivatives.230 Singha et al.231 showed Pseudomonas fragi DBC and Jatropha curcas interacted for pyrene biodegradation, with yfc upregulation under pyrene stress in the presence of artificial root exudates, enhancing plant growth and stress response in Jatropha roots mediated by P. fragi DBC. The release of flavonoid compounds from plant roots can trigger the co-metabolism of polyaromatic hydrocarbons. This is because flavonoids have a similar structure to aromatic compounds, which enhances the degradation and mineralization process carried out by microorganisms.232 The degradation of non-aromatic plant compounds, specifically linoleic acid-induced pyrene and benzo[a]pyrene, by Gram-positive microorganisms has also been investigated. Various studies have highlighted the enhanced bioavailability of xenobiotic compounds when plant roots secrete low molecular weight organic acids like malic acid, citric acid, succinic acid, tartaric acid, and oxalic acid.233
Despite these beneficial approaches, very few large-scale and long-term studies have been conducted on the rhizoremediation of PAH-contaminated soils. Most existing studies are limited to greenhouse conditions and focus primarily on bioremediation strategies involving microbial populations. Therefore, there is an urgent need to promote awareness and popularize rhizoremediation techniques for global applications. Table 3 provides examples of large-scale field studies on bioremediation, specifically addressing TPH and PAH remediation.
Table 3 Details of field case studies on bioremediation conducted worldwide
SI. no |
Sites |
Type of remediation |
Degradation efficiency |
References |
1 |
University of Calabar (spiked with crude oil) |
Bioremediation |
Bacillus, Pseudomonas, Vibrio, Micrococcus, and Alcaligenes reduced crude oil from 26.7% to 43.3% after 16 days |
234 |
2 |
University of Port Harcourt spiked with crude oil |
Biostimulation and phytoremediation |
Biostimulation of soil with NPK was more effective than phytoremediation using Vigna sp. |
235 |
3 |
Former oil refinery site in Montreal, Canada |
Rhizoremediation |
Willow plantations showed a 60–80% reduction in organic contaminants and heavy metals |
236 |
4 |
Kuwait |
Native microbial species bioremediation |
Over a 12-month period, reduction of TPH up to 82.5% and 90.5% of alkanes |
237 |
5 |
Crude oil polluted farmland in Bodo |
Phytoremediation |
In 90 days, M. alternifolius and F. ferruginea showed 99% TPH removal and 78% PAH removal |
238 |
6 |
Northern France |
Phytoremediation |
Miscanthus × giganteus. 3.19–53.85% removal of PAHs |
239 |
7 |
China |
Phytoremediation |
7 month rhizoremediation using alfalfa and tall fescue. 7.5–17.2% 5(+6)-ring PAH removal by alfalfa and 25.1−30.1% in tall fescue |
240 |
8 |
Shengli Oil Field in Dongying City, China |
Rhizoremediation |
In 150 days, the removal rate of ∑ 8 PAHs was up to 99.40% using Fire Phoenix |
241 |
9 |
India |
Rhizoremediation |
Populus deltoides with Kurthia sp. SBA4, Micrococcus varians SBA8, Deinococcus radiodurans SBA6 and Bacillus circulans SBA12 degraded 43.6% of PAHs in 120 days |
242 |
10 |
Jianghan Oil Field, China |
Bioremediation |
Pseudomonas sp., with rice husk and plowing, showed 95% TPH degradation in 150 days |
243 |
Table 4 Plant-microbe association in rhizoremediation of pollutants
SI. no |
Plant species |
Microbial species |
Type of contaminant |
References |
1 |
Hordeum vulgare |
Burkholderia cepacia |
2,4-Dichlorophenoxyacetic acid |
244 |
2 |
Populus deltoides |
Actinomycete Amycolata sp. CB1190 |
1,4-Dioxane |
245 |
3 |
Populus deltoides |
Sphingomonas yanoikuyae |
Benzo[a]pyrene |
246 |
4 |
Spartina alterniflora |
Gram-negative bacteria and endophytes |
Phenanthrene, pyrene |
247 |
5 |
Glycine max |
Glomus caledonium GM24, Glomus intraradices GG31, Glomus coronatum GU53, Pseudomonas fluorescens PA28, Pseudomonas borealis PA29, Bacillus subtilis BA41 |
Pyrene and others |
248 |
6 |
Cucumis sativus, Daucus carota, Allium cepa, Cucurbita, Petroselinum sativum |
Mixed culture |
Total 16 PAH |
249 |
7 |
Lotus corniculatus L., Oenothera biennis L. |
Rhizobium, Pseudomonas, Stenotrophomonas Rhodococcus |
Hydrocarbon |
250 |
8 |
Trifolium repens, Lolium perenne |
AMF Glomus mosseae |
PAH |
251 |
9 |
Festuca arundinacea, Sorghum x drummondii Lolium perenne, Lolium multiflorum |
Fulvivirga kasyanovii,Massilia niabensis,Novosphingobium indicum |
Phenanthrene, fluoranthene and pyrene |
252 |
10 |
Jatropha curcas |
Pseudomonas aeruginosa PDB1 |
Pyrene |
253 |
11 |
Vallisneria spiralis |
Mixed consortium |
Phenanthrene and pyrene |
254 |
12 |
Melia azedarach |
B. subtilis SR1, B. subtilis S1I26, Paenibacillus sp. S1I8 and S. arlettae S1I1 |
Benzo(a)pyrene (BaP) |
182 |
13 |
Populus deltoides |
Kurthia sp., Micrococcus varians, Deinococcus radiodurans and Bacillus circulans |
Chrysene, benzene, toluene, xylene, anthracene and naphthalene |
255 |
14 |
Vigna unguiculata, Helianthus annus, Austrodanthonia caespitosa, Zea mays, Sorghum sudanense, Vetiveria zizanoides |
Pseudomonas sphingomonas |
Mixed PAH |
256 |
15 |
Italian ryegrass |
Pseudomonas poae, Actinobacter bouvetii, Stenotrophomonas rhizophila, Pseudomonas rhizosphaerae |
Hydrocarbon contamination |
257 |
16 |
Lolium perenne, Medicago sativa |
Mixed culture |
Pyrene |
258 |
17 |
Populus deltoides |
Burkholderia fungorum DBT1 |
Dibenzothiophene, naphthalene, fluorene and phenanthrene |
259 |
18 |
T. patula, M. jalapa |
Mixed culture |
Benzo[a]pyrene |
260 |
19 |
Brassica napus L. |
Rhodoccocus equi, β-proteobacterium, Enterobacter sp., Acinetobacter calcoaceticus, Comamonas sp., Pseudomonas alcaligenes |
Petroleum hydrocarbon |
261 |
20 |
Medicago sativa L. |
Sinorhizobium meliloti strain 1021 |
Dioxin-like PCB |
262 |
The rhizoremediation of PAHs using plants and microbes in the soil is illustrated in Fig. 3. Root exudates, such as carbohydrates, flavonoids, and amino acids, facilitate interactions with rhizospheric microbes, enhancing the microbial degradation of PAH compounds. Additionally, the secretion of various compounds such as indole acetic acid (IAA), siderophores, ACC deaminase, and phytohormones promotes plant growth and provides protection in contaminated environments. Microbial cells contribute to PAH degradation by producing enzymes such as dioxygenases, monooxygenases, and oxidoreductases, which break down complex PAH structures into less toxic intermediates and ultimately lead to their mineralization into CO2 and H2O. Similarly, plants produce enzymes like peroxidases, laccases, and phenol oxidases, which play a crucial role in the oxidation of hydrocarbon compounds into intermediate derivatives, further facilitating PAH degradation and accumulation.
 |
| Fig. 3 The microbe-associated rhizoremediation of PAHs is a multistep process involving complex plant-microbe interactions. Root exudates secreted by plant roots enhance both plant growth and microbial degradation of contaminants. In the rhizospheric space, plants and microbes engage in chemical signalling and quorum sensing to facilitate cross-talk. In PAH-contaminated soil, microbial populations increase the bioavailability of hydrocarbon contaminants and metabolize them as a carbon source. Additionally, plants can directly absorb and store simpler forms of these contaminants in their biomass, contributing to the overall remediation process. | |
4.3. Genetic attributes for rhizoremediation of PAHs
The rhizosphere enriched both PAH-degrading microorganisms and functional genes associated with PAH degradation.263 The alteration in the chemical properties of PAHs, such as their hydrophobic or hydrophilic character, affects their absorption by microbial communities and plant cells. The use of Trichoderma virens-derived glutathione transferase (GST) enhanced the effectiveness of phytoremediation for recalcitrant PAHs, such as anthracene, by genetically incorporating the gene into Nicotiana tabacum (tobacco) plants.264 Ibáñez et al.265 found that the use of TPX1 (tobacco transgenic hairy roots) in conjunction with arbuscular mycorrhizal fungus (AMF) enhanced the efficiency of phenol phytoremediation as compared to only relying on transgenic hairy root technology. AMF-enhanced transgenic tobacco hairy roots have a notable capacity to withstand elevated concentrations of phenol. This may be attributed to the existence of strong anti-oxidative enzyme systems that protect against oxidative damage caused by phenol. Horizontal gene transfer is a common occurrence in the endophytic niche, where microbial communities adapt to environmental stress.266–268 For instance, the plasmid pTOM-Bu61, which carries genes for enzymes that break down toluene, may spontaneously transfer to many types of plant endophytes. This transfer has played a significant role in facilitating the effective breakdown of toluene in poplar plants. Pseudomonas endophytes carrying plasmids pWWO and pNAH7 exhibited significant levels of horizontal transfer to other endophytes.269 In a separate study, Barac et al.270 documented the process of conjugative transformation of natural endophytes to improve the degradation of toluene. The expression of the bphA gene, which encodes biphenyl dioxygenase in Pseudomonas sp., is apparently induced by the presence of salicylate in root exudates during the degradation of PCBs.271 Enhanced variants of bphA, which demonstrate improved PCB degradation capabilities, were generated from Burkholderia cepacia strain LB400, Comamonas testosteroni B-365, and Rhodococcus globerulus P6 using a family of shuffling technique.272 In the context of hydrocarbon remediation, several genes, including alkB, have been identified as contributing to stress tolerance.272 In Pseudomonas sp., additional genes such as nah, pah, and phn enhanced microbe-assisted phytoremediation.273,274 Nie et al.275 studied the Dietzia genome, focusing on the alkB and CYP153 genes, which encode alkane monooxygenase and P450 alkane hydroxylase potential for PAH phytoremediation. The expression of NahAa, NahAb, NahAc, and NahAd genes in Pseudomonas putida G7 (flavoprotein reductase, ferredoxin, and terminal dioxygenase subunits, respectively) was shown to enhance phenanthrene rhizoremediation in Arabidopsis thaliana and Oryza sativa. Enzymes such as nahAc and C23O (catechol 2,3-dioxygenase) play a vital role in starting the conversion of BaP, as they have evolved over time due to prolonged contact with petroleum.276 Research has demonstrated that certain microorganisms, including Pseudomonas sp., Burkholderia sp., Mycobacterium sp., and Sphingomonas sp., harbour highly conserved catabolic gene clusters (nah, phd, nid, and phn) that encode enzymes responsible for PAH degradation.277 Studies have shown the enrichment of key PAH-degrading genes in the plant rhizosphere, including PAH-RHD (PAH-ring hydroxylating dioxygenase), phtA (phthalate dioxygenase), P34O (protocatechuate 3,4-dioxygenase), and C12O/C23O (catechol dioxygenases).263 These genes were elevated by 6.93–8.33-fold in 13C-DNA metagenomes of the rhizosphere compared to bulk soil.263 The PAH-RHD gene showed a significantly higher abundance in ryegrass rhizospheres exposed to benzo[a]pyrene,190 while tall fescue enhanced PAH-RHDα Gram-negative gene expression.278 Predominant PAH-degrading genes, including those encoding PAH dioxygenase and ring-cleavage dioxygenase, were more abundant in rhizospheres of Betula pendula in PAH-contaminated soil than in bulk soil.190 Additionally, C12O and C23O gene expression in tall fescue rhizospheres increased 1.2–1.9 times relative to bulk soil.273 The relative abundances of PAH-RHDα genes showed a strong correlation with the degradation rates of 13C-phenanthrene, highlighting their role in PAH biodegradation. The current understanding of the genetic basis of PAH degradation in the rhizosphere remains limited, particularly in comparison to non-rhizosphere environments.279 There is a need for further investigation into additional functional genes associated with PAH biodegradation to enhance rhizoremediation efforts. Multi-omics approaches, such as metagenomics, metatranscriptomics, and metabolomics, as explained in the following sections, can provide deeper insights into the functional diversity, metabolic pathways, and regulatory mechanisms involved in microbial PAH degradation. Different peripheral and central metabolic pathways related to PAH metabolism in bacterial systems are illustrated in Fig. 4. Aerobic degradation pathways involve dioxygenase-catalyzed oxidation of aromatic rings, leading to the formation of dihydrodiol intermediates. These intermediates undergo further cleavage via the ortho or meta pathways, generating key intermediates such as protocatechuates and catechols, which are subsequently integrated into the tricarboxylic acid (TCA) cycle for complete mineralization.
 |
| Fig. 4 Illustration of an overview of the peripheral and central metabolic pathways, including the genes responsible for the enzymes involved in PAH metabolism.178,179 | |
5. Microbial diversity and functional analysis of PAH contaminated soil
A significant and persistent challenge in the study of the microbial role in PAH rhizoremediation lies in the restricted knowledge to discern the majority of microbial taxa within the community.280 To address this limitation, emerging methodologies and advanced omics technologies have been developed to explore the physiological, metabolic, and structural aspects of the microbiome linked to the degradation of pollutants.
5.1. Metagenomics
The study of metagenomes in the rhizosphere offers valuable insights into the organization and content of the microbiome, particularly focusing on bacterial 16SrDNA.281 Advancements in next-generation sequencing and bioinformatics methodologies have enabled scientists to develop techniques and pipelines that enhance understanding of metagenomes.282 Metagenomics enables the comprehensive understanding of microbial diversity and their ecological roles within a specific habitat.283 The phylum Proteobacteria continues to be prominently featured in such habitats, highlighting its significant role in the natural attenuation of PAH-contaminated soils.284 In the context of soil samples collected from crude oil wells, the predominant microbial diversity was characterized by the presence of various taxonomic groups, including members of the Acetobacteraceae, Hyphomicrobiaceae, Rhodobacteraceae, and Sphingomonadaceae families.285 In contaminated soil, Alphaproteobacteria, Betaproteobacteria, and Gammaproteobacteria exhibited a robust association with other biological processes.286 A greater prevalence of Rhodocyclaceae and Polaromonas in agricultural soils polluted with PAHs was positively correlated with the degradation of LMW PAHs.287–289
In a recent study, Wang et al.290 observed a higher abundance of Alphaproteobacteria and Actinobacteria in contaminated soil. Similarly, the metagenomics analysis of a soil sample amended with anthracene has indicated that the relative abundance of Gammaproteobacteria was greater than that of Alphaproteobacteria.291 Further, the prevalence of Actinobacteria was significantly higher in soil that has been spiked with anthracene, while Acidobacteria was predominantly found in soil that has not been spiked.292 Redfern et al.,293 in metagenomic studies, showed that the Geobacter species was a predominant biostimulation candidate in PAH-contaminated soil due to its significant prevalence and high abundance of degradative genes. Further, Mycobacterium and Sphingomonas were found to interact with different PAHs and have notable abundance in contaminated soil, making them suitable candidates for bioaugmentation studies.294 Metagenomic analyses revealed the presence of key metabolic intermediates such as phthalate, protocatechuate, naphthalene, and salicylate in the rhizosphere, reconstructing PAH degradation pathways based on functional gene annotations.190
5.2. Genomics
Genomic methodologies that include the comprehensive analysis of an organism's whole genome or partial draft sequencing provide distinct benefits for investigations related to a group of genes participating in the complete breakdown of hydrocarbon compounds. These high-throughput molecular techniques play a crucial role in the identification of microbial traits and operons that exhibit collective activity involving numerous genes.295 Additionally, these techniques aid in the identification of genes with similar functions, which may be linked to various additional genes in different microbes. The whole genome of the newly identified sp. of Stenotrophomonas had a total of 145 genes that were associated with the breakdown of PAHs such as anthraquinone, biphenyl, naphthalene, phenanthrene, and phenanthridine.296
The whole genome sequencing of Sphingobium yanoikuyae B1 revealed the presence of around 5140 putative open reading frames, composed of 35 dioxygenase genes including catechol 1, 2-dioxygenase, biphenyl 2,3-dioxygenase, and biphenyl-2, 3-diol 1, 2-dioxygenase.297 Similarly, whole genome sequencing of the Bacillus marisflavi Bac 144 strain revealed the existence of genes associated with hydrocarbon degradation along with significant plant growth-promoting (PGP) attributes. Several species, including Gordonia bronchialis, Gordonia sputi, Williamsia muralis, and Corynebacterium efficiens, were detected to express the catechol 1,2-dioxygenase gene under different PAH stress conditions demonstrating its role in degradation pathways.298–300 The genomic analysis of Bacillus subtilis SR1 reveals 12 genes involved in the metabolic processes of aromatic compounds, including peripheral pathways like biphenyl 2,3-dioxygenase, gentisate 1,2-dioxygenase, fumarylacetoacetase, and catechol 2,3-dioxygenase, which catalyze the degradation of hydrocarbons.301 Pseudomonas aeruginosa DN1 was found to be proficient in breaking down HMW PAHs and crude oil from soil samples contaminated with petroleum at Changqing Oilfield. The strain's genome contains numerous genes and gene clusters that contribute to the degradation of aromatic compounds, including catA (catechol 1,2-dioxygenase), pcaG, which encodes the beta subunit of protocatechuate 3,4-dioxygenase, hmgA (homogentisate 1,2-dioxygenase), dad (2,4′-dihydroxyacetophenone dioxygenase), benzoate/toluate 1,2-dioxygenase and gentisate 1,2-dioxygenase. Several genes, including nah, phn, and nid, which encode enzymes such as naphthalene dioxygenase, salicylate hydroxylase, and phenanthrene dioxygenase, have been identified as being responsible for PAH degradation.302 Ivanova et al.303 documented that Paraburkholderia aromaticivorans strain BN5 possesses a total of 29 monooxygenase and 54 dioxygenase genes associated with the biodegradation of various hydrocarbons.
5.3. Metatranscriptomics
Metatranscriptomic analysis is employed to investigate the mRNA expression patterns of genes within a specific microorganism or a community of microorganisms present in an ecosystem.304 Mukhtar et al.305 studied the metatranscriptome of functional gene expression in the Willow plant soil microbiome grown in contaminated and uncontaminated soil. The contaminated rhizosphere showed increased expression of genes related to competitive traits like antibiotic resistance and biofilm formation due to selective pressure from pollutants and the rhizosphere environment.306 Additionally, soils contaminated with pollutants had higher expression levels of genes associated with PHC degradation. A study conducted by Peng et al.307 employed a transcriptomics-based approach to elucidate the specific microbial species involved in the degradation of PHCs within the context of willow-microbe systems. The study observed an increased expression of four key genes associated with PHC degradation. This enhanced gene expression was notably detected within several bacterial orders, including Actinomycetales, Rhodospirillales, Burkholderiales, Alteromonadales, Solirubrobacterales, Caulobacterales, and Rhizobiales. In a study, de Menezes et al.308 investigated the effects of phenanthrene on the soil microbial community. It was demonstrated that the addition of phenanthrene resulted in a significant increase in the abundance of transcripts related to dioxygenase, stress response, and detoxification. The relative quantities of heavy metal P-type adenosine triphosphatases (ATPases) and thioredoxin proteins in microorganisms, specifically in relation to their response to PAH stress, have also been identified.
5.4. Metaproteomics
The field of proteomics, or metaproteomics analysis, involves the comprehensive examination of the whole protein composition within a certain ecological environment.309 Metaproteomics can also be used for ongoing monitoring of the soil microbial community as remediation efforts progress, providing feedback on the effectiveness of bioremediation strategies. Guazzaroni et al.310 used shotgun metagenomics and metaproteomics to study microbial diversity in soil contaminated with PAHs in northern Spain. Their primary objective was to gain insights into how biostimulation influenced the microbial community after exposure to naphthalene. The researchers successfully reconstructed the metabolic pathway responsible for the degradation of naphthalene, focusing on the gentisate pathway, activated by specific bacterial groups within the soil's complex microbial communities. Bastida et al.311 investigated the compost-assisted bioremediation process in semiarid soil contaminated with petroleum. Surprisingly, they found that only 0.55% of the proteins identified in the compost-treated soils were associated with biodegradation, despite the successful removal of 88% of alkanes and PAHs within 50 days of compost treatment. The primary influencers in the compost-assisted bioremediation process were the Sphingomonadales. These microorganisms exhibited a higher abundance of catabolic enzymes, including dioxygenases and cis-dihydrodiol dehydrogenases. Furthermore, in the presence of benzoate, p-hydroxybenzoate, and vanillin, Pseudomonas putida KT2440 induced around 80 unique proteins, including various dioxygenases, hydrolases, and thiolases.312 Through proteomic methods, researchers found that nidA is closely linked to the metabolism of pyrene, while nidA3 is associated with fluoranthene. This suggests that the bacterium employs different initial RHO enzymes in response to HMW-PAHs when serving as a carbon source.313
Rabus314 reported metaproteomics analysis and found Burkholderiales as the active community member responsible for the degradation of PAHs in the presence of dioxygenase enzymes within this microbial group. Further, the work by Guazzaroni et al.310 unveiled the presence of the naphthalene degradation pathway within certain bacterial species inhabiting complex microbial communities. A metaproteomic approach identified 847 proteins from microorganisms involved in naphthalene and fluorene degradation. About 70% of these proteins came from taxonomic groups like Burkholderiales, Actinomycetales, and Rhizobiales.315
5.5. Metabolomics
A metabolomic platform has the potential to be used for the purpose of quantitatively and extensively investigating the metabolic reactions of living organisms in response to external influences.316 The application of metabolomic technology has the potential to enhance the detection of biological reactions resulting from soil changes, thereby elucidating distinct phenotypic variations such as alterations in the composition and quantity of soil metabolites.317 Bao et al.318 carried out an extensive investigation to study the microbial community of petroleum-contaminated soil. The results of their research revealed a remarkable level of diversity within the microbial population, as well as the presence of numerous metabolites. Experimental investigations have shown a substantial increase in the expression of enzymes during the breakdown of various external aromatic compounds. Similarly, Li et al.319 investigated the profound impact of crude oil pollution on the composition of soil microorganisms and their metabolites. The levels of metabolites derived from PAH degradation pathways included 9-fluorenone and gentisic acid. Wang et al.320 determined the metabolites producing during phenanthrene degradation using Rhodococcus qingshengii strain FF. The primary metabolite identified was pyrogallol, and notably, 59% of the metabolites were oxygen-containing PAHs with a single benzene ring.
PAHs reduce hydroxypyruvate levels and alter amino acid metabolism, thereby influencing gluconeogenesis. Furthermore, phenanthrene exposure in wheat root cells leads to decreased cellular pyruvate levels and downregulation of key metabolic enzymes such as glyceraldehyde-3-phosphate dehydrogenase (involved in NADH production) and adenosine kinase (related to ATP generation), which significantly impact the dynamics of the TCA cycle.321 The existence of PAHs triggers the activation of metabolic pathways related to galactose, sucrose, inositol galactoside, and melibiose in plants. This activation leads to elevated levels of D-mannose, D-galactose, raffinose, galactinol, melibiose, sucrose, and D-glucose metabolites in plant tissues. The findings indicate a suppression of energy-producing processes, specifically the synthesis of ATP and NADPH, coupled with an induction of fermentative metabolism within plant cells in the presence of different kinds of PAHs.321 Fig. 5 illustrates various omics approaches, including metagenomics, genomics, metatranscriptomics, metabolomics, and metaproteomics, for the identification of PAH-degrading genes, metabolic pathways, enzyme expression, and associated microbial abundance in soil.
 |
| Fig. 5 Various omics approaches employed to analyze contaminated soil samples from PAH-contaminated environments. | |
6. Factors affecting the rhizoremediation process
Rhizoremediation, being a mutually beneficial biological process, is influenced by a multitude of factors, where the interplay between plants and microorganisms is pivotal for effective degradation.6 Numerous abiotic and biotic factors affect the biodegradation of polyaromatic hydrocarbons in contaminated sites.322 These include soil properties, pollutant concentrations, pH, soil composition, organic matter content, temperature, nutrient availability, soil moisture, oxygen levels, contaminant solubility, microbial community diversity, metabolic capabilities, substrate specificity, carbon sources, and biofilm/biosurfactant production. Also, the intensity of the plant-microbe interactions has a significant impact on different stages of rhizoremediation.323 Furthermore, the rhizoremediation process is influenced by several interconnecting factors. These factors include the physical and chemical complexity of PAH compounds, the history of pollution, the composition, porosity, and permeability of the soil, the density of the soil, the distribution of contaminants, the metabolic functioning of microbes, and the diversity of the microbial population involved in PAH mineralization.324
Contamination by PAHs modifies the organic matter and composition of soil, specifically influencing parameters such as carbon-to-nitrogen ratio, carbon-to-phosphorus ratio, salinity, pH, and electrical conductivity.325 Maintaining a neutral soil pH is crucial for effective PAH biodegradation. Wu et al.326 observed that in soils contaminated with PAHs (0.18–20.68 mg kg−1), the pH varied widely (4.26–8.43), significantly influencing bacterial diversity. Moreover, the benzo[a]pyrene degradation rate was noted to be pH-dependent, with the highest efficiency observed at pH 8.0 and the lowest at pH 5.0. Pawar reported that 50% of PAHs degraded within three days at pH 7.5, while the same degradation took 21 days at pH 5.0–6.5.327 Vipotnik et al.328 observed that approximately 85–90% of fluorene, pyrene, and benzo[a]pyrene degraded more efficiently in soil at pH 5, while chrysene exhibited greater degradability at pH 7 through fungal remediation processes. Qi et al.329 also demonstrated that PAHs significantly increased soil organic carbon while reducing total phosphorus levels. The abundance of Nitrospinota, Dadabacteria, Planctomycetota, and Acidobacteria showed a positive correlation with soil PAH and total phosphorus levels but a negative correlation with total salt and organic carbon content.
Soil contamination with PAHs typically involves a complex mixture of hydrocarbon molecules. The presence of multiple pollutants in varying combinations increases toxicity compared to individual contaminants. PAH concentrations in contaminated soils are often accompanied by co-contaminants such as heavy metals (Cu, Ni, V, Zn, Pb, and Cr) and elevated salt levels. Co-contamination of PAHs and heavy metals can have both synergistic and antagonistic effects on pollutant removal in the rhizosphere. For instance, Cd-PAH co-contamination enhanced Cd removal in the rhizosphere of Fire Phoenix.107 Similarly, low concentrations of pyrene-Cu/Cd co-contamination promoted metal removal and pyrene degradation in maize.330 However, high Cu levels (1000–1500 mg kg−1) reduced PAH removal efficiency in maize, Elsholtzia splendens, and Lolium perenne.330 This inhibition may result from heavy metal-induced suppression of root growth and exudation, altering enzyme activity and microbial communities in the rhizosphere.330 Su et al.194 reported that microbial diversity in the rhizosphere was higher than in the endosphere under phenanthrene stress. Additionally, organic pollutants like phenanthrene had a substantial effect on microbial communities in the endosphere and rhizosphere compared to bulk soil.330
Studies have elucidated the mechanisms driving differences in the microbial community structure and activity among plant species and genotypes, which can be attributed to variations in the root structure and plant-specific exudates.331 Under pollution stress, root exudation patterns differ across plant species in terms of composition and concentration,332 leading to changes in microbial biomass, activity, and structural composition.331 The release of root exudates by plants contributes to the availability of carbon and nitrogen sources, which in turn creates a conducive environment for microorganisms to degrade organic contaminants. Moreover, certain plants, such as maize, can synthesize secondary metabolites like benzoxazinoids, which influence the selective depletion and enrichment of microbial species in the rhizosphere.333 Such plant-specific microbial recruitment can lead to cultivar-specific rhizosphere microbiota. However, the primary factors shaping rhizosphere microbial diversity remain inconsistent across studies. While Robertson et al.334 identified plant species and soil factors as the main determinants of microbial community structure, Pritchina et al.335 suggested that PAH pollution levels had a more pronounced impact than plant species. Therefore, further research is needed to determine the dominant factors controlling rhizosphere microbiome composition under organic pollution, which could aid in optimizing plant-microbe interactions for improved bioremediation efficiency. Additionally, in soils co-contaminated with Cu and phenanthrene, the rhizosphere microbiome of Elsholtzia splendens and Lolium perenne exhibited substantial shifts in the enrichment and depletion of microbial phyla such as Actinobacteria and Bacteroidetes.336 These findings highlight the complexity of plant-microbe interactions in contaminated environments and underscore the need for targeted microbiome engineering to enhance rhizoremediation.
Organic pollution exerts significant toxicity on microbial populations, leading to reduced microbial biomass and diversity in the rhizosphere.337 Phenanthrene exhibits greater toxicity to microorganisms than pyrene, due to its higher bioavailability.338 As PAH concentrations increase, microbial biomass declines markedly in both rhizospheres.338 Gram-positive bacteria demonstrate heightened susceptibility to PAHs compared to Gram-negative bacteria. Bacteria exhibit greater sensitivity to organic pollutants such as lindane, PCBs, and co-contaminants like cadmium and DDT compared to fungal communities.337,339
Further, Eriksson et al.340 investigated PAH biodegradation under low temperatures and anaerobic conditions, demonstrating that aerobic conditions at 20 °C facilitated the removal of 52% to 88% of PAHs over 90 days, whereas the highest PAH degradation at 7 °C reached 53%. Similarly, Amponsah et al.341 found that increasing soil temperatures from 10 °C to 20 °C and subsequently to 25 °C reduced pyrene, fluorene, chrysene, and anthracene concentrations at three Canadian well sites. These findings align with previous studies,342–344 which reported enhanced PAH degradation with increasing soil moisture. Similar studies have highlighted the critical role of soil moisture in organic pollutant degradation by regulating microbial oxygen availability, with optimal moisture levels varying by climate and soil type, ranging from 30–90%345 to 12–32%.346
Moreover, electrical conductivity quantifies the soil's capacity to conduct electricity, directly correlating with the quantity of dissolved salts and nutrients. Elevated electrical conductivity may signify heightened salinity, potentially detrimental to plant development and microbial activity. Maintaining suitable electrical conductivity in the soil is essential for facilitating effective rhizoremediation processes. Cation exchange capacity refers to the soil's capability to retain and exchange positively charged ions (cations) such as potassium, calcium, and magnesium. A high cation exchange capacity signifies that the soil has an enhanced ability to store and provide necessary nutrients to plants and microbes. This directly impacts the efficacy of rhizoremediation by guaranteeing that plants and microorganisms get sufficient nutrients to sustain their development and metabolic functions.347
7. Methods for improving the effectiveness of rhizoremediation
To enhance the effectiveness of rhizoremediation in addressing PAH-contaminated soil, a combination of carefully chosen strategies can be employed to optimize plant-microbe interactions and the degradation processes within the rhizosphere. These strategies include plant selection, bioaugmentation, nutrient management, and several others, with a focus on long-term sustainable solutions.348
7.1. Microbiome engineering
The process of microbiome engineering involves the in situ application of a “bacterial consortium” as an artificial community. This community is used to manipulate and control the existing microbial community in order to achieve remediation objectives.349 The primary objective of bioaugmentation is to enhance the removal of contaminants by leveraging the metabolic activities of these externally introduced microorganisms.350–352 Derz et al.353 demonstrated the effectiveness of bioaugmentation by introducing a mixed bacterial culture into soil contaminated with PAHs, specifically pyrene and benzo[a]pyrene. They observed a pyrene mineralization rate of approximately 36% after 150 days and a benzo[a]pyrene removal rate of 5% after 70 days. The application of bioaugmentation in landfarming led to an 86% reduction in total petroleum hydrocarbons within a 90-day timeframe. The phytochelating activity of Mesorhizobium haukuii was enhanced by introducing a chelating gene (phytochelatin synthase; PCSAt) from A. thaliana.354 The enhancement of siderophore production in polluted soil can be achieved through the regulation of the transcriptional unit pvdS regulator on the pvdD and pvdA genes of Pseudomonas fluorescens.355 The evaluation of bioaugmentation involving the introduction of efficient hydrocarbon-degrading Pseudomonas bacteria into the rhizosphere of teak (T. grandis), gmelina (G. arborea), neem (Azadirachta indica), and champak (Michelia champaca) plants has been conducted. The objective of this evaluation is to enhance the biodegradation of crude oil.356 From a practical standpoint, employing a microbial consortium instead of a pure culture for bioremediation offers distinct advantages, primarily due to its capacity to deliver the necessary metabolic diversity and resilience essential for field applications.357 However, the effectiveness of this procedure significantly relies on the chosen microbial consortia's adaptability to the specific site conditions and their capability to outcompete the indigenous microorganisms.358 The bacterial culture is introduced into the polluted area using methods like spraying, injection, or soil or water blending, depending on the specific environment. After the bacteria become established, they initiate the process of metabolizing the PAH compounds found in the polluted surroundings.359 This process at play is referred to as mineralization, resulting in the complete removal of PAH contaminants.360 Throughout the course of the bioaugmentation procedure, continuous monitoring of the site is conducted to assess the progress in PAH degradation and the efficacy of the introduced bacterial strains.361 Further, Lolium perenne and Medicago sativa can improve pyrene degradation in soil up to 46% when biocompost is applied.362
7.2. Soil engineering
Biostimulation is a procedure used to increase the growth of indigenous organisms in habitats polluted by contaminants. This is achieved by providing nutrients that promote co-metabolism.363 Biostimulation is the introduction of organic nutrients into the polluted area to enhance the development and functioning of native microorganisms.364 As the microbial population experiences growth, it initiates the synthesis of various enzymes, such as dioxygenases and ring-hydroxylating enzymes. The enzymes are essential in the process of breaking down PAHs, converting them into compounds that are simpler and pose less risk.365 The PAHs undergo subsequent enzymatic reactions, ultimately resulting in their complete conversion into CO2, H2O, and biomass. This process ensures the complete removal of PAHs from the environment.366,367 The utilisation of diverse materials, including crop residues, sugarcane bagasse pith, sewage sludge compost, vermicompost, food waste compost, corn stalks, corn fermentation byproduct, peat and sawdust,368 wastewater sludge, ground rice hulls, and dried blood,369 has been observed to lead to a noticeable increase in PAH degradation. When introduced into a PAH-polluted environment, the addition of 5% manure resulted in an increase in available phosphorus, potassium, and hydrolysable nitrogen. This, in turn, supported the growth of bacteria capable of degrading PAHs. The utilisation of PGPR and biochar in the process of bioremediation for soil contaminated with hydrocarbons has gained significant importance in recent years.370 Furthermore, a thorough examination was conducted to analyse the impacts of nutrients (NPK), aeration, and the bio-induction of native soil microorganisms, as well as the stimulation of external microbial communities. This investigation revealed that these factors have positive effects on the remediation of oil-contaminated soil.371
7.3. Phyto-engineering
The initial step in the phyto-engineering approach for crude oil degradation is the selection of plants that possess high contaminant degrading efficiency. The presence of a plant species with a significant amount of aboveground biomass is crucial in the phytoextraction process.372 The successful implementation of rhizoremediation requires the inclusion of a plant species that has an extensive root system or significant belowground biomass. However, the presence of contaminants frequently hinders the growth of plants in polluted regions.373 The incorporation of ACC deaminase genes into genetically modified plants has been demonstrated to successfully decrease ethylene levels, leading to a stronger and more extensive root system.374 The process of plant breeding and genetic modification can lead to several beneficial outcomes, including enhanced nutrient intake, increased production of root exudates, improved survival rates, and more efficient mineralization of pollutants.375 The researchers Uchida et al.376 conducted genetic engineering on Arabidopsis plants to introduce a root-specific laccase (LAC1) obtained from cotton plants.377 The researchers conducted an observation and found that the modified plants demonstrated enhanced tolerance to phenolic compounds and 2, 4, 6-trichlorophenol when these substances were secreted into the rhizosphere. Moreover, the successful enhancement of the degradation process of polychlorinated biphenyls (PCBs) was observed through the insertion of the bphC gene derived from Pandoraea pnomenusa B-356 into tobacco plants.277 In addition, Uchida et al.376 reported that the introduction of estradiol dioxygenase genes responsible for aromatic cleavage (DBfB) into Arabidopsis plants resulted in an increased degradation rate of 2,3-dihydroxybiphenyl (2,3-DHB).
8. The global bioremediation markets
According to Biospace Reports (2022), the bioremediation market had a value of USD 105.68 billion in 2019 and is expected to see a compound annual growth rate (CAGR) of 15.5%. It is forecast to reach USD 334.70 billion by 2027.378 The environmental remediation market in the United States is projected to experience significant growth over the forecast period. It is anticipated to increase from USD 19.96 billion in 2021 to USD 22.86 billion in 2022 and further reach USD 37.26 billion by 2027, reflecting a CAGR of 10.96%. In recent years, there has been a significant increase in global awareness and acknowledgment of natural-based bioremediation methods. The biotreatment industry in the United States is currently composed of around 130 businesses, which can be categorised into three primary sectors: product vendors, transdisciplinary environmental services, and bioremediation services.379 Due to its cost-effectiveness and accessibility, widespread promotion of rhizoremediation, along with bioaugmentation and biostimulation, is recommended.380
In 2018, the in situ bioaugmentation segment held the largest market share at 23.9%, followed by the biostimulation segment at 16.91%. Soil remediation accounted for the highest revenue, with a market share of 46.64%, driven by the increasing prevalence of soil pollutants. In terms of application, the industrial sector led the market with a 27.09% share, followed by agriculture and aquaculture at 21.18%.381 In 2022, the phytoremediation segment accounted for the largest portion of global revenue, exceeding 32.02%. This growth in the industrial segment is attributed to the significant number of contaminants and pollution originating from this sector (Global Bioremediation Market, 2020).382
The phytoremediation market was valued at USD 1.07 billion in 2019. The fungal bioremediation method, which is employed for the purpose of eliminating radioactive contaminants, is currently experiencing a CAGR of around 15.2%. The market is projected to be dominated by the soil-based bioremediation category, with a CAGR of 15.8% from 2019 onwards.383 However, in situ bioremediation is anticipated to generate the most revenue in the coming years due to its cost-effectiveness and minimal risk of cross-contamination. In situ bioremediation methods include bio-slurping, bio-vending, and rhizoremediation.384 The biostimulation sector generated revenues of $12,094 million in 2021 and is projected to grow at an annual rate of 7.1%, reaching $24,500.2 million by 2030 (Share and Trends Report, 2030).385
The North American region has recently dominated the market for bioremediation, accounting for a market share of approximately 41.8%. This can be attributed to the presence of numerous major industrial firms in the region. Asia-Pacific is projected to experience the highest CAGR of 16.5% during the forecast period (Biospace Reports, 2022).386 The expansion of the bioremediation market in these areas is mainly propelled by the rising environmental concerns and regulatory objectives for environmental protection established by diverse government sectors (Environmental Remediation Market, 2022).387
The Netherlands has achieved successful remediation of over 6000 sites since 1982, establishing itself as the European country with the most significant advancements in this field (Europe Sustainable Development Report, 2021).388 Companies like Gist-Brocades are marketing improved anaerobic wastewater clean-up techniques. The Dutch government endorses the utilisation of compact fermenters for the conversion of agricultural waste into commercially viable fertilisers, with a specific focus on providing support to underdeveloped nations. Additionally, they are actively engaged in conducting research on soil bioremediation.389 The Ministry of Construction in Japan launched a project in the 1980s with a budget of five billion yen. The project aimed to develop and implement biotechnological methods for treating wastewater (Sanitation and Sustainable Development in Japan, 2016).390 The Swedish National Environment Protection Board has recently contracted a biotreatment company from the UK to carry out in situ bioremediation of soil contaminated with creosote. The bioremediation process will involve the use of Pseudomonas bacteria. The total value of the contract is estimated at US $1.6 million.390 The progress in bioremediation underscores its critical role in addressing environmental challenges across various sectors, including industry, agriculture, and remediation, highlighting its potential for continuous innovation and global application.
9. The global landscape of patents related to bioremediation
Patents play a crucial role in measuring economic development as they enable the efficient exchange and spread of technology between different countries.391 The United States is the leading country in terms of bioremediation technology patents, accounting for 61.85% of the total. China follows with 79% and Japan with 67%.392 The contributions of South Korea and India are approximately 4.51% and 2.93%, respectively.393 Bioremediation research is less prevalent in other nations such as Australia, Belgium, France, Spain, Canada, Great Britain, and Russia. The distribution of patent applications among different regions is as follows: out of a total of 443 applications, North American countries accounted for 67%, Asian countries accounted for 23%, and European countries accounted for 10%. The data suggest that Asian nations, specifically Japan and Korea, are making significant investments in research and development (R&D) to improve their technological capabilities.394
Developed countries possess robust research infrastructures to address oil contamination issues due to their financial resources, availability of trained scientific personnel, and stable economies (National Innovation Systems, OECD).395 PAH contaminants are prevalent in developing and economically disadvantaged countries as well. The capacity of biological systems to break down aromatic hydrocarbons and the utilisation of bioremediation techniques have been well-documented since the early 1970s.396 The earliest patent applications, dating back to 1971, discuss the use of emulsifiers or fertilizers to promote oil-degrading bacteria. The Exxon Valdez oil spill in 1989 marked a significant increase in hydrocarbon degradation patent applications in the early 1990s.397 Initially, chemical and physical methods were employed to mitigate the damage, but bioremediation soon emerged as a viable cleanup technique.398
An analysis was conducted on the global database maintained by the European Patent Office to examine the growth of bioremediation technologies for water, soil, and sludge. The results indicate a steady increase in these technologies, with water accounting for 53% of the patents, soil accounting for 36%, and sludge accounting for 11%.399 In India, government agencies are less involved in cutting-edge bioremediation R&D compared to private entities. Organizations such as the Council of Scientific and Industrial Research (CSIR), the Indian Council of Agricultural Research (IARI), Bharat Petroleum Corporation Ltd, Indian Oil Corporation Ltd, and M/S Avestha Gengraine Technologies Pvt. Ltd have secured numerous patents, yet private entities have obtained more patents than government institutions.400 Fig. 6 highlights the leading continents in the bioremediation market based on market revenue and patent filings. Continents with a significant bioremediation market, along with market size and CAGR values, are presented in Table 5, which also lists several key bioremediation companies involved in the sector.386
 |
| Fig. 6 A map illustrating countries with major bioremediation markets and the percentage of patents filed from different geographic regions. Transparent bubbles represent continents with significant patent activity, while coloured dots within the bubbles indicate countries with high bioremediation market revenue. The size of each dot corresponds to the market share percentage of the respective country. | |
Table 5 Overview of the bioremediation market across different continents, including market size, CAGR, and key companies actively involved in bioremediation386
SI no. |
Continents |
CAGR |
Market size |
Key companies |
1 |
North America |
8.7% |
US$ 5040.96 million |
• Altogen Labs |
• InSitu Remediation Services Ltd |
• Probiosphere |
• Xylem |
• REGENESIS |
2 |
Europe |
7.70% |
US$ 3487.61 million |
• Altogen Labs |
• InSitu Remediation Services Ltd |
• Probiosphere |
• Xylem |
• REGENESIS |
3 |
Asia-Pacific |
8.43% |
US$ 2249.68 million |
• Aquatech International Carus Group Inc |
• Drylet Inc |
• InSitu Remediation Services Ltd |
• Remediation Solutions |
• RT Environmental Services Inc. |
• Soilutions Ltd, Verde Environmental Group |
• Xylem Inc. |
4 |
Latin America |
8.26% |
US$ 3898.20 million |
• Aquatech International Carus Group Inc. |
• Drylet Inc. |
• InSitu Remediation Services Ltd |
• Remediation Solutions |
• RT Environmental Services Inc. |
• Soilutions Ltd |
• Verde Environmental Group |
• Xylem Inc. |
5 |
Middle East & Africa (MEA) |
6.4% |
US$ 695.57 million |
• Aquatech International LLC |
• InSitu Remediation Services Limited |
• Ivey International Inc. |
• Xylem Inc. |
10. Limitations and challenges
Rhizoremediation, as a plant-microbe-driven strategy for PAH degradation, offers a promising approach for restoring contaminated soils. However, its long-term sustainability is influenced by several critical factors. Some limitations of rhizoremediation include the need for a large field area for in situ remediation, the age of the plant being used, hindered plant and microbial growth due to severe pollution, dependence on environmental factors, uncertainty regarding the disposal of plant parts containing PAHs, unknown by-products of biodegradation, and the possibility of PAHs entering the food chain. The use of seasonal plants in rhizoremediation may be limited in certain situations.401–403 To facilitate efficient rhizoremediation, it is crucial to monitor and regulate soil pH, electrical conductivity, and cation exchange capacity. The soil pH affects the activity of microorganisms and influences the solubility and transportation of contaminants in the soil. A further crucial factor for rhizoremediation is the possibility of unforeseen ecological repercussions. The introduction of non-indigenous plant species or microbes might provide unforeseen consequences for the surrounding environment. Hyperaccumulator plants, capable of absorbing and withstanding elevated concentrations of certain pollutants, are exemplary candidates for bioremediation. Moreover, the efficacy of bioremediation may be enhanced by using plants with broad root systems that foster interactions between plant roots and the rhizosphere microbial population, enhancing biotransformation activities.404 Prioritizing climate-adaptive plant-microbe systems is crucial for sustainable remediation. Plant health and growth metrics act as indirect markers of rhizoremediation effectiveness. Monitoring root exudation patterns, stress responses, and biomass buildup is essential for evaluating plant-microbe interactions and their influence on PAH breakdown.
The effectiveness of rhizoremediation is contingent upon the persistence of PAH-degrading microbial populations within the rhizosphere. Soil that is contaminated can offer the combined advantages of increased fertility and bioremediation through microbial processes, leading to soil that is both healthy and productive.405 Microorganisms in the soil play a crucial role in increasing the availability of nutrients and aiding in the production of regulators that promote plant growth. Concurrently, they participate in numerous transformations of organic substances within the soil and play a role in the degradation of xenobiotics. Another function involves participating in the processes of adsorption and desorption of various substances, as well as the detoxification of both organic and inorganic contaminants.405 Specific strains exhibit the ability to break down particular pollutants, notably those that include functional genes like PAH-RHDα and dioxygenase genes, which are essential for maintaining degradation efficiency.405 Conventional bioremediation techniques encompass continuous biostimulation, which involves the supplementation of nutrients to promote microbial activity, and bioaugmentation, which entails the introduction of external microflora, aimed at achieving long-term sustainability.406 For instance, the inoculation of plant growth-promoting rhizobacteria in soil cultivated with tall fescue and rice plants augmented the extraction of total petroleum hydrocarbons (particularly the C21–C34 fraction) and phthalate esters during phytoremediation.407 However, more advanced strategies are required to optimize rhizoremediation efficiency for diverse organic contaminants.
The particular genes associated with the biodegradation of various organic contaminants and their degradation pathways within the rhizosphere are not yet fully understood. Furthermore, numerous recent investigations concerning rhizosphere microorganisms were predominantly conducted in controlled greenhouse environments utilising artificially contaminated soil, which markedly differs from natural field conditions. Li et al.407 explored the impact of ryegrass root exudates on phenanthrene biodegradation using both 12C- and 13C-labeled phenanthrene. Understanding microbial community shifts within the rhizosphere, including the role of pollutant-degrading microbes and their functional genes, is essential for optimizing in situ remediation strategies.
To ensure the long-term effectiveness of rhizoremediation, continuous monitoring of PAH concentrations and microbial activity is crucial. Advances in nanocomposite technologies have enhanced PAH analysis across various matrices. Pre-treatment, extraction, and clean-up techniques, primarily using gas chromatography-mass spectrometry (GC-MS) or high-performance liquid chromatography-ultraviolet (HPLC-UV), enable precise detection. GC-MS and GC-MS/MS offer high sensitivity and selectivity, with GCxGC-FID achieving recovery rates of 95–120% for 24 PAHs in soil. Eventually, to quantify the PAHs adsorbed, techniques such as Raman spectroscopy, Fourier-transform infrared spectroscopy (FTIR), and scanning electron microscopy (SEM) play a crucial role in the initial identification of PAHs.408 Ma et al.409 developed a sensor integrating pre-concentration and in situ electrochemical analysis using electropolymerized poly(3-methylthiophene) (P3MT) for 1-hydroxypyrene detection, a key PAH exposure marker.
Despite these advancements, large-scale PAH degradation in field studies remains underexplored. Post-rhizoremediation monitoring is essential to evaluate residual PAH levels and ensure successful long-term remediation outcomes. Following rhizoremediation, soil rehabilitation occurs alongside microbial community shifts that enhance nutrient cycling, predominantly involving native microbes. Incorporating amendments such as biochar, compost, or surfactants can further stimulate microbial activity and improve PAH bioavailability, thereby increasing remediation efficiency. Integrating these strategies can optimize rhizoremediation as a sustainable, long-term solution for PAH-contaminated soils.
In addition, several significant factors are anticipated to restrict the revenue growth of the global bioremediation market. These include the high cost of excavation equipment and the slow adoption of environmental protection regulations. The costs related to the use of heavy machinery in treatment operations, such as bulldozers, loading trucks, and excavators, are considerably higher. Environmental protection regulations and policies pertaining to environmental flow are being implemented globally; however, several interconnected challenges are evident. Expanding bioscience research, whether in the academic sector or the commercial sphere, depends on having advanced laboratory infrastructure and cutting-edge technology. Modern research facilities must be established, upgraded, and expanded. Strong business relationships must be forged.410 Collaboration initiatives with other countries' premier reference centres must be encouraged.411 The exploration of better alternatives to the use of technology and natural resources and to organise economic growth without endangering the ecosystem's sustainability must be implemented in the future.412 To achieve the bioeconomy and sustainability goals, land decontamination should be viewed as critical. The remedial technology of bioremediation seems to be sustainable.413 While lacking statutory authority, sustainability criteria are nonetheless infrequently used to evaluate remedial technology.414
11. Conclusion and future prospects
The pollution of PAHs is a long-lasting global issue. Nevertheless, higher levels of production are also associated with a greater number of instances of contamination in various ecosystems. The soil is a primary ecosystem that is consistently contaminated by numerous PAH molecules as a result of human interference. Inadequate management practices and accidents result in the pollution of PAHs, leading to the depletion of soil fertility and negative effects on plants and indigenous soil bacteria. Consequently, there exists a significant disparity in the commercialization of rhizoremediation for the purpose of treating soil contaminated with PAHs. This method has the potential to offer a sustainable solution for restoring ecosystems. Rhizoremediation not only removes contaminants from damaged soil, but also improves soil fertility by restoring its natural structure. The bioremediation technique often involves the use of microorganisms to clean up contaminated places or to enhance the activity of naturally occurring microbes in polluted areas. Therefore, by employing suitable combinations of plants and microbes in polluted areas, this issue could be effectively resolved. The use of modern sequencing techniques to manipulate the rhizosphere also improves our understanding of the intricate plant–microbe relationship. Nevertheless, it is imperative for both the public and private sectors involved in bioremediation to prioritise the commercialization of rhizoremediation techniques and the global dissemination of knowledge. Additionally, the process must be improved to achieve both environmental and commercial advantages. Effective strategic planning and the formulation of enduring policies on rhizoremediation will support nations in attaining their objectives for a sustainable future.
The process of rhizoremediation is regarded as an efficient approach; however, a significant research gap remains that must be addressed for future advancements. As highlighted in previous sections, large-scale field studies on rhizoremediation are still very limited. There is a need to raise awareness for the beneficial attributes of rhizoremediation and effectively commercialize such plant-microbe pairs for field application. Rhizoremediation not only degrades contaminants but also enhances soil health and microbial communities, making it a promising strategy for restoring farmlands and agricultural sites. Conducting more data-driven studies will provide a comprehensive analysis and reinforce confidence in the efficacy of rhizoremediation. Additionally, process optimization is crucial, as rhizoremediation depends on several biotic and abiotic factors. The selection of efficient plant-microbe pairs and the assessment of proper remediation strategies are essential for ensuring successful implementation. Furthermore, the effectiveness and duration of the rhizoremediation process must be considered for long-term field applications. Research is also needed to optimize the benefit-to-cost ratio, particularly regarding the cost of implementing rhizoremediation relative to the biomass generated. The processing of rhizoremediated biomass requires additional precautions and refinement. However, there is significant potential to utilize this biomass for other applications like biochar production, contributing to the circular economy, as reviewed in previous studies.21 To overcome current limitations in evaluating nature-based remediation systems, it is essential to refine life cycle assessment (LCA) and cost-benefit analysis (CBA) approaches.415 Addressing these challenges will facilitate the broader adoption of rhizoremediation as a sustainable and cost-effective strategy for environmental restoration.
Data availability
No data were used for the research described in the article.
Author contributions
Writing – original draft: ND; conceptualization: PP; data curation: ND; formal analysis: ND, PP; funding acquisition: ND, PP; investigation: ND, VK, KC; validation: PP; visualization: PP; writing – review & editing: PP.
Conflicts of interest
There are no conflicts to declare.
Acknowledgements
The authors gratefully acknowledge the financial support received from the Department of Biotechnology, Ministry of Science and Technology, Government of India.
References
- H. I. Abdel-Shafy and M. S. Mansour, A review on polycyclic aromatic hydrocarbons: source, environmental impact, effect on human health and remediation, Egyptian journal of petroleum, 2016, 25(1), 107–123 CrossRef.
- L. M. Palade, M. Negoită, A. C. Adascălului and A. L. Mihai, Polycyclic aromatic hydrocarbon occurrence and formation in processed meat, edible oils, and cereal-derived products: A review, Appl. Sci., 2023, 13(13), 7877 CrossRef CAS.
- P. B. Karadakov and M. A. Al-Yassiri, Excited-state aromaticity reversals in naphthalene and anthracene, J. Phys. Chem. A, 2023, 127(14), 3148–3162 CrossRef CAS PubMed.
- J. E. Balmer, H. Hung, Y. Yu, R. J. Letcher and D. C. Muir, Sources and environmental fate of pyrogenic polycyclic aromatic hydrocarbons (PAHs) in the Arctic, Emerging Contam., 2019, 5, 128–142 CrossRef.
- K. Nam, W. Rodriguez and J. J. Kukor, Enhanced degradation of polycyclic aromatic hydrocarbons by biodegradation combined with a modified Fenton reaction, Chemosphere, 2001, 45(1), 11–20 CrossRef CAS PubMed.
- L. P. Singha and P. Pandey, Rhizosphere assisted bioengineering approaches for the mitigation of petroleum hydrocarbons contamination in soil, Crit. Rev. Biotechnol., 2021, 41(5), 749–766 CrossRef CAS PubMed.
- M. C. Lim, G. A. Ayoko, L. Morawska, Z. D. Ristovski and E. R. Jayaratne, The effects of fuel characteristics and engine operating conditions on the elemental composition of emissions from heavy duty diesel buses, Fuel, 2007, 86(12–13), 1831–1839 CrossRef CAS.
- F. Alkurdi, F. Karabet and M. Dimashki, Characterization, concentrations and emission rates of polycyclic aromatic hydrocarbons in the exhaust emissions from in-service vehicles in Damascus, Atmos. Res., 2013, 120, 68–77 CrossRef.
- M. A. Mallah, L. Changxing, M. A. Mallah, S. Noreen, Y. Liu, M. Saeed, H. Xi, B. Ahmed, F. Feng, A. A. Mirjat and W. Wang, Polycyclic aromatic hydrocarbon and its effects on human health: An overeview, Chemosphere, 2022, 296, 133948 CrossRef CAS PubMed.
- J. J. Nam, A. J. Sweetman and K. C. Jones, Polynuclear aromatic hydrocarbons (PAHs) in global background soils, J. Environ. Monit., 2009, 11(1), 45–48 RSC.
- Y. Li and X. Duan, Wet deposition of polycyclic aromatic hydrocarbons in a remote area of Central South China from 2014 to 2017, Atmos. Sci. Lett., 2024, 25(3), e1201 CrossRef.
- S. Zhang, Q. Li, Y. Zou, B. Liu, J. Yang, H. Zheng and G. Liu, Using isotopic lead and strontium in sediments to trace natural and anthropogenic sources in the Bohai Sea, Sci. Rep., 2024, 14(1), 1–8 CrossRef PubMed.
- R. Li, P. Hua, J. Cai, X. Wang, Y. Zhu, Z. Huang, P. Li, Z. Wang, Y. Bai, B. X. Hu and J. Zhang, A sixteen-year reduction in the concentrations of aquatic PAHs corresponding to source shifts in the Elbe River, Germany, J. Clean. Prod., 2019, 223, 631–640 CrossRef CAS.
- A. Ukalska-Jaruga, B. Smreczak and A. Klimkowicz-Pawlas, Soil organic matter composition as a factor affecting the accumulation of polycyclic aromatic hydrocarbons, J. Soils Sediments, 2019, 19, 1890–1900 CrossRef CAS.
- A. Ramesh, A. E. Archibong, D. B. Hood, Z. Guo and B. G. Loganathan, Global environmental distribution and human health effects of polycyclic aromatic hydrocarbons, Global Contam. Trends Persistent Org. Chem., 2011, 63, 97–126 Search PubMed.
- R. Li, J. Zhang and P. Krebs, Global trade drives transboundary transfer of the health impacts of polycyclic aromatic hydrocarbon emissions, Commun. Earth Environ., 2022, 3(1), 170 CrossRef PubMed.
- C. E. Boström, P. Gerde, A. Hanberg, B. Jernström, C. Johansson, T. Kyrklund, A. Rannug, M. Törnqvist, K. Victorin and R. Westerholm, Cancer risk assessment, indicators, and guidelines for polycyclic aromatic hydrocarbons in the ambient air, Environ. Health Perspect., 2002, 110(suppl 3), 451–488 CrossRef PubMed.
- A. K. Haritash and C. P. Kaushik, Biodegradation aspects of polycyclic aromatic hydrocarbons (PAHs): a review, J. Hazard Mater., 2009, 169(1–3), 1–5 CrossRef CAS PubMed.
- A. Das, S. Das, N. Das, P. Pandey, B. Ingti, V. Panchenko, V. Bolshev, A. Kovalev and P. Pandey, Advancements and innovations in harnessing microbial processes for enhanced biogas production from waste materials, Agriculture, 2023, 13(9), 1689 CrossRef CAS.
- R. Miglani, N. Parveen, A. Kumar, M. A. Ansari, S. Khanna, G. Rawat, A. K. Panda, S. S. Bisht, J. Upadhyay and M. N. Ansari, Degradation of xenobiotic pollutants: an environmentally sustainable approach, Metabolites, 2022, 12(9), 818 CrossRef CAS PubMed.
- N. Das, D. K. Maheshwari and P. Pandey, Energy crop-based rhizoremediation and lignocellulosic biomass production as sustainable bioeconomy-driven solution for biofuel generation and waste mitigation, Prog. Energy Combust. Sci., 2024, 103, 101161 CrossRef.
- S. Eskandary, A. Tahmourespour, M. Hoodaji and A. Abdollahi, The synergistic use of plant and isolated bacteria to clean up polycyclic aromatic hydrocarbons from contaminated soil, J. Environ. Health Sci. Eng., 2017, 15, 1–8 CrossRef PubMed.
- I. M. Gillespie and J. C. Philp, Bioremediation, an environmental remediation technology for the bioeconomy, Trends Biotechnol., 2013, 31(6), 329–332 CrossRef CAS PubMed.
- https://www.emergenresearch.com/.
- Bioremediation and Bioeconomy: A Circular Economy Approach, ed. M. N. Prasad, Elsevier, 2023 Search PubMed.
- A. Ball and A. Truskewycz, Polyaromatic hydrocarbon exposure: an ecological impact ambiguity, Environ. Sci. Pollut. Res., 2013, 20, 4311–4326 CrossRef CAS PubMed.
- I. Tolosa, S. de Mora, M. R. Sheikholeslami, J. P. Villeneuve, J. Bartocci and C. Cattini, Aliphatic and aromatic hydrocarbons in coastal Caspian Sea sediments, Mar. Pollut. Bull., 2004, 48(1–2), 44–60 CrossRef CAS PubMed.
- M. Brazkova and A. Krastanov, Polycyclic aromatic hydrocarbons: Sources, effects and biodegradation, Hayxyb Tpylode Ha Pyceycrbz Eybdepcbnen, 2013, 10, 2, pp. 52–56 Search PubMed.
- O. S. Olatunji, O. S. Fatoki, B. J. Ximba and B. O. Opeolu, Polycyclic Aromatic Hydrocarbons (PAHs) in Edible Oil: Temperature Effect on Recovery from Base Hydrolysis Product and Health Risk Factor, Food Public Health, 2014, 4, 23–30 Search PubMed.
- N. Das, B. Bhuyan and P. Pandey, Correlation of soil microbiome with crude oil contamination drives detection of hydrocarbon degrading genes which are independent to quantity and type of contaminants, Environ. Res., 2022, 215, 114185 CrossRef CAS PubMed.
- T. Kariyawasam, G. S. Doran, J. A. Howitt and P. D. Prenzler, Polycyclic aromatic hydrocarbon contamination in soils and sediments: Sustainable approaches for extraction and remediation, Chemosphere, 2022, 291, 132981 CrossRef CAS PubMed.
- H. I. Eldos, N. Zouari, S. Saeed and M. A. Al-Ghouti, Recent advances in the treatment of PAHs in the environment: Application of nanomaterial-based technologies, Arab. J. Chem., 2022, 15(7), 103918 CrossRef CAS.
- A. B. Patel, S. Shaikh, K. R. Jain, C. Desai and D. Madamwar, Polycyclic aromatic hydrocarbons: sources, toxicity, and remediation approaches, Front. Microbiol., 2020, 11, 562813 CrossRef PubMed.
- K. Srogi, Monitoring of environmental exposure to polycyclic aromatic hydrocarbons: a review, Environ. Chem. Lett., 2007, 5, 169–195 CrossRef CAS PubMed.
- L. Mohammadi, A. Rahdar, E. Bazrafshan, H. Dahmardeh, M. A. Susan and G. Z. Kyzas, Petroleum hydrocarbon removal from wastewaters: a review, Processes, 2020, 8(4), 447 CrossRef CAS.
- G. Yerulker, P. Patel, A. Chafale, V. Rathod, S. Das, P. Pandey, N. A. Khan, A. Devi, N. S. Munshi, R. Dhodapkar and A. Kapley, Comparative assessment of soil microbial community in crude oil contaminated sites, Environ. Pollut., 2023, 328, 121578 CrossRef CAS PubMed.
- N. Ailijiang, N. Zhong, X. Zhou, A. Mamat, J. Chang, S. Cao, Z. Hua and N. Li, Levels, sources, and risk assessment of PAHs residues in soil and plants in urban parks of Northwest China, Sci. Rep., 2022, 12(1), 21448 CrossRef CAS PubMed.
- G. S. Siemering and R. Thiboldeaux, Background concentration, risk assessment and regulatory threshold development: Polycyclic aromatic hydrocarbons (PAH) in Milwaukee, Wisconsin surface soils, Environ. Pollut., 2021, 268, 115772 CrossRef CAS PubMed.
- P. Pandey, A. Kapley and S. K. Brar, Biodegradation of high molecular weight polyaromatic hydrocarbons in different environments, Front. Microbiol., 2021, 12, 704897 CrossRef PubMed.
- K. E. Ugwu and P. O. Ukoha, Polycyclic aromatic hydrocarbons (PAHs) in surface sediments near a mining site in Okobo-Enjema, Nigeria: concentrations, source apportionment and risk assessment, Environ. Geochem. Health, 2018, 40, 359–373 CrossRef CAS PubMed.
- B. A. Bandowe, N. Shukurov, S. Leimer, M. Kersten, Y. Steinberger and W. Wilcke, Polycyclic aromatic hydrocarbons (PAHs) in soils of an industrial area in semi-arid Uzbekistan: spatial distribution, relationship with trace metals and risk assessment, Environ. Geochem. Health, 2021, 43(11), 4847–4861 CrossRef CAS PubMed.
- M. N. Tanić, D. Dinić, B. Kartalović, Ž. Mihaljev, S. Stupar, M. Ćujić and A. Onjia, Occurrence, source apportionment, and health risk assessment of polycyclic aromatic hydrocarbons in soil of urban parks in a mid-sized city, Water, Air, Soil Pollut., 2023, 234(7), 484 CrossRef.
- H. Choi and C. S. Kim, Polycyclic aromatic hydrocarbons from fine particulate matter induce oxidative stress and the inflammatory response in human vocal fold fibroblast cells, Oxid. Med. Cell. Longev., 2021, 2021(1), 5530390 CrossRef PubMed.
- P. J. Tsai, H. Y. Shieh, W. J. Lee and S. O. Lai, Health-risk assessment for workers exposed to polycyclic aromatic hydrocarbons (PAHs) in a carbon black manufacturing industry, Sci. Total Environ., 2001, 278(1–3), 137–150 CrossRef CAS PubMed.
- T. Rengarajan, P. Rajendran, N. Nandakumar, B. Lokeshkumar, P. Rajendran and I. Nishigaki, Exposure to polycyclic aromatic hydrocarbons with special focus on cancer, Asian Pac. J. Trop. Biomed., 2015, 5(3), 182–189 CrossRef CAS.
- A. Dovlatabadi, E. H. Estiri, M. L. Najafi, A. Ghorbani, H. Rezaei, M. Behmanesh, E. Momeni, A. Gholizadeh, A. Cristaldi, G. Mancini and A. Alahabadi, Bioaccumulation and health risk assessment of exposure to potentially toxic elements by consuming agricultural products irrigated with wastewater effluents, Environ. Res., 2022, 205, 112479 CrossRef CAS PubMed.
- Y. Chen, F. Zhang, J. Zhang, M. Zhou, F. Li and X. Liu, Accumulation characteristics and potential risk of PAHs in vegetable system grow in home garden under straw burning condition in Jilin, Northeast China, Ecotoxicol. Environ. Saf., 2018, 162, 647–654 CrossRef CAS PubMed.
- Jr F. Barbosa, B. A. Rocha, M. C. Souza, M. Z. Bocato, L. F. Azevedo, J. A. Adeyemi, A. Santana and A. D. Campiglia, Polycyclic aromatic hydrocarbons (PAHs): updated aspects of their determination, kinetics in the human body, and toxicity, J. Toxicol. Environ. Health, Part B, 2023, 26(1), 28–65 Search PubMed.
- A. T. Lawal, Polycyclic aromatic hydrocarbons. A review, Cogent Environ. Sci., 2017, 3(1), 1339841 CrossRef.
- Q. Shi, R. W. Godschalk and F. J. Van Schooten, Inflammation and the chemical carcinogen benzo [a] pyrene: Partners in crime, Mutat. Res., Rev. Mutat. Res., 2017, 774, 12–24 CrossRef CAS PubMed.
- Z. Zelinkova and T. Wenzl, The occurrence of 16 EPA PAHs in food–a review, Polycyclic Aromat. Compd., 2015, 35(2–4), 248–284 CrossRef CAS PubMed.
- M. Honda and N. Suzuki, Toxicities of polycyclic aromatic hydrocarbons for aquatic animals, Int. J. Environ. Res. Public Health, 2020, 17(4), 1363 CrossRef CAS PubMed.
- L. Molina and A. Segura, Biochemical and metabolic plant responses toward polycyclic aromatic hydrocarbons and heavy metals present in atmospheric pollution, Plants, 2021, 10(11), 2305 CrossRef CAS PubMed.
- A. Jajoo, N. R. Mekala, R. S. Tomar, M. Grieco, M. Tikkanen and E. M. Aro, Inhibitory effects of polycyclic aromatic hydrocarbons (PAHs) on photosynthetic performance are not related to their aromaticity, J. Photochem. Photobiol., B, 2014, 137, 151–155 CrossRef CAS PubMed.
- R. Singh-Tomar and A. Jajoo, Alteration in PS II heterogeneity under the influence of polycyclic aromatic hydrocarbon (fluoranthene) in wheat leaves (Triticum aestivum), Plant Sci., 2013, 209, 58–63 CrossRef CAS PubMed.
- S. Kottuparambil and J. Park, Anthracene phytotoxicity in the freshwater flagellate alga Euglena agilis Carter, Sci. Rep., 2019, 9(1), 15323 CrossRef PubMed.
- B. Maliszewska-Kordybach and B. Smreczak, Habitat function of agricultural soils as affected by heavy metals and polycyclic aromatic hydrocarbons contamination, Environ. Int., 2003, 28(8), 719–728 CrossRef CAS PubMed.
- J. Mo, J. Feng, W. He, Y. Liu, N. Cao, Y. Tang and S. Gu, Effects of polycyclic aromatic hydrocarbons fluoranthene on the soil aggregate stability and the possible underlying mechanism, Environ. Sci. Pollut. Res., 2023, 30(4), 10245–10255 CrossRef CAS PubMed.
- G. Ren, W. Ren, Y. Teng and Z. Li, Evident bacterial community changes but only slight degradation when polluted with pyrene in a red soil, Front. Microbiol., 2015, 6, 22 Search PubMed.
- M. Z. Hoque, A. Alqahtani, S. Sankaran, D. Anand, M. M. Musa, A. Nzila, G. Guerriero, K. S. Siddiqui and I. Ahmad, Enhanced biodegradation of phenanthrene and anthracene using a microalgal-bacterial consortium, Front. Microbiol., 2023, 14, 1227210 CrossRef PubMed.
- M. Rahman, S. Ghosh and P. C. Sarma, Disappearance time of a few polycyclic aromatic hydrocarbons in soil, Asian J. Water Environ. Pollut., 2021, 18(1), 67–75 CrossRef.
- Q. Zhang, P. Liu, S. Li, X. Zhang and M. Chen, Progress in the analytical research methods of polycyclic aromatic hydrocarbons (PAHs), J. Liq. Chromatogr. Relat. Technol., 2020, 43(13–14), 425–444 CrossRef CAS.
- N. Reyes-Garces, E. Gionfriddo, G. A. Gómez-Ríos, M. N. Alam, E. Boyacı, B. Bojko, V. Singh, J. Grandy and J. Pawliszyn, Advances in solid phase microextraction and perspective on future directions, Anal. Chem., 2017, 90(1), 302–360 CrossRef PubMed.
- I. Sowa, M. Wójciak-Kosior, M. Strzemski, J. Sawicki, M. Staniak, S. Dresler, W. Szwerc, J. Mołdoch and M. Latalski, Silica modified with polyaniline as a potential sorbent for matrix solid phase dispersion (MSPD) and dispersive solid phase extraction (d-SPE) of plant samples, Materials, 2018, 11(4), 467 CrossRef PubMed.
- B. Ambade, S. S. Sethi, S. Kurwadkar, A. Kumar and T. K. Sankar, Toxicity and health risk assessment of polycyclic aromatic hydrocarbons in surface water, sediments and groundwater vulnerability in Damodar River Basin, Groundw. Sustain. Dev., 2021, 13, 100553 CrossRef.
- Z. Xue, X. Zheng, W. Yu, A. Li, S. Li, Y. Wang and X. Kou, Research progress in detection technology of polycyclic aromatic hydrocarbons, J. Electrochem. Soc., 2021, 168(5), 057528 CrossRef CAS.
- Y. Yan, X. Chen, S. Hu and X. Bai, Applications of liquid-phase microextraction techniques in natural product analysis: A review, J. Chromatogr. A, 2014, 1368, 1–7 CrossRef CAS PubMed.
- J. M. Kokosa, Recent trends in using single-drop microextraction and related techniques in green analytical methods, Trac. Trends Anal. Chem., 2015, 71, 194–204 CrossRef CAS.
- M. Ghambarian, Y. Yamini and A. Esrafili, Developments in hollow fiber based liquid-phase microextraction: principles and applications, Microchim. Acta, 2012, 177, 271–294 CrossRef CAS.
- P. Avino, I. Notardonato, L. Perugini and M. V. Russo, New protocol based on high-volume sampling followed by DLLME-GC-IT/MS for determining PAHs at ultra-trace levels in surface water samples, Microchem. J., 2017, 133, 251–257 CrossRef CAS.
- M. Rezaee, Y. Yamini and M. Faraji, Evolution of dispersive liquid–liquid microextraction method, J. Chromatogr. A, 2010, 1217(16), 2342–2357 CrossRef CAS PubMed.
- L. Guo, S. Tan, X. Li and H. K. Lee, Fast automated dual-syringe based dispersive liquid–liquid microextraction coupled with gas chromatography–mass spectrometry for the determination of polycyclic aromatic hydrocarbons in environmental water samples, J. Chromatogr. A, 2016, 1438, 1–9 CrossRef CAS PubMed.
- M. Haji Hosseini, P. Asaadi, M. Rezaee, M. R. Rezaei, M. R. Pourjavid, M. Arabieh and A. A. Abhari, Homogeneous liquid–liquid microextraction via flotation assistance (HLLME-FA) method for the pretreatment of organochlorine pesticides in aqueous samples and determination by GC–MS, Chromatographia, 2013, 76, 1779–1784 CrossRef CAS.
- N. M Fernández, A. M Coto-García, R. Gonzalo-Lumbreras, J. Sanz-Landaluze, C. Perez-Conde and C. Cámara, Sample Treatment in Organic Compound Determination: A Green Chemistry Perspective, Curr. Green Chem., 2016, 3(2), 133–144 CrossRef.
- E. Zilfidou, A. Kabir, K. G. Furton and V. Samanidou, Fabric phase sorptive extraction: Current state of the art and future perspectives, Separations, 2018, 5(3), 40 CrossRef CAS.
- V. Kazantzi and A. Anthemidis, Fabric sol–gel phase sorptive extraction technique: a review, Separations, 2017, 4(2), 20 CrossRef.
- C. M. Almeida, Overview of sample preparation and chromatographic methods to analysis pharmaceutical active compounds in waters matrices, Separations, 2021, 8(2), 16 CrossRef CAS.
- S. S. Saini, A. Kabir, A. L. Rao, A. K. Malik and K. G. Furton, A novel protocol to monitor trace levels of selected polycyclic aromatic hydrocarbons in environmental water using fabric phase sorptive extraction followed by high performance liquid chromatography-fluorescence detection, Separations, 2017, 4(2), 22 CrossRef.
- Z. Szczepaniak, J. Czarny, J. Staninska-Pięta, P. Lisiecki, A. Zgoła-Grześkowiak, P. Cyplik, Ł. Chrzanowski, Ł. Wolko, R. Marecik, W. Juzwa and K. Glazar, Influence of soil contamination with PAH on microbial community dynamics and expression level of genes responsible for biodegradation of PAH and production of rhamnolipids, Environ. Sci. Pollut. Res., 2016, 23, 23043–23056 CrossRef CAS PubMed.
- L. Duan, R. Naidu, P. Thavamani, J. Meaklim and M. Megharaj, Managing long-term polycyclic aromatic hydrocarbon contaminated soils: a risk-based approach, Environ. Sci. Pollut. Res., 2015, 22, 8927–8941 CrossRef CAS PubMed.
- H. Wu, B. Sun and J. Li, Polycyclic aromatic hydrocarbons in sediments/soils of the rapidly urbanized lower reaches of the River Chaohu, China, Int. J. Environ. Res. Public Health, 2019, 16(13), 2302 CrossRef CAS PubMed.
- N. Manousi and G. A. Zachariadis, Recent advances in the extraction of polycyclic aromatic hydrocarbons from environmental samples, Molecules, 2020, 25(9), 2182 CrossRef CAS PubMed.
- E. Mirzaee and M. Sartaj, Remediation of PAH-contaminated soil using a combined process of soil washing and adsorption by nano iron oxide/granular activated carbon composite, Environ. Nanotechnol. Monit. Manag., 2023, 20, 100800 CAS.
- Z. I. Takai, M. K. Mustafa, S. Asman and K. A. Sekak, Preparation and characterization of magnetite (Fe3O4) nanoparticles by sol-gel method, Int. J. Nanoelectron. Mater., 2019, 12(1), 37–46 Search PubMed.
- F. Ma, Y. Wang, Z. Fu, Y. Tang, J. Dai, C. Li and W. Dong, Thermal ageing mechanism of a natural rock-modified asphalt binder using Fourier Transform Infrared Spectroscopy analysis, Constr. Build. Mater., 2022, 335, 127494 CrossRef CAS.
- M. Kumar, N. S. Bolan, S. A. Hoang, A. D. Sawarkar, T. Jasemizad, B. Gao, S. Keerthanan, L. P. Padhye, L. Singh, S. Kumar and M. Vithanage, Remediation of soils and sediments polluted with polycyclic aromatic hydrocarbons: to immobilize, mobilize, or degrade?, J. Hazard. Mater., 2021, 420, 126534 CrossRef CAS PubMed.
- N. Manousi and G. A. Zachariadis, Recent advances in the extraction of polycyclic aromatic hydrocarbons from environmental samples, Molecules, 2020, 25(9), 2182 CrossRef CAS PubMed.
- M. Cortazar, L. Santamaria, G. Lopez, J. D. Alvarez, L. Zhang, R. Wang, X. Bi and M. Olazar, A comprehensive review of primary strategies for tar removal in biomass gasification, Energy Convers. Manag., 2023, 276, 116496 CrossRef CAS.
- Z. Chen, Z. Chen, Y. Li, R. Zhang, Y. Liu, A. Hui, W. Cao, J. Liu, H. Bai and J. Song, A review on remediation of chlorinated organic contaminants in soils by thermal desorption, J. Ind. Eng. Chem., 2024, 133, 112–121 CrossRef CAS.
- J. Płotka-Wasylka, N. Jatkowska, M. Paszkiewicz, M. Caban, M. Y. Fares, A. Dogan, S. Garrigues, N. Manousi, N. Kalogiouri, P. M. Nowak and V. F. Samanidou, Miniaturized solid phase extraction techniques for different kind of pollutants analysis: state of the art and future perspectives–PART 1, Trac. Trends Anal. Chem., 2023, 162, 117034 CrossRef.
- A. A. Akinpelu, M. E. Ali, M. R. Johan, R. Saidur, M. A. Qurban and T. A. Saleh, Polycyclic aromatic hydrocarbons extraction and removal from wastewater by carbon nanotubes: A review of the current technologies, challenges and prospects, Process Saf. Environ. Prot., 2019, 122, 68–82 CrossRef CAS.
- M. S. Santana, L. Sandrini-Neto, F. F. Neto, C. A. Ribeiro, M. Di Domenico and M. M. Prodocimo, Biomarker responses in fish exposed to polycyclic aromatic hydrocarbons (PAHs): systematic review and meta-analysis, Energy Convers. Manag., 2018, 242, 449–461 CAS.
- K. Nam, W. Rodriguez and J. J. Kukor, Enhanced degradation of polycyclic aromatic hydrocarbons by biodegradation combined with a modified Fenton reaction, Chemosphere, 2001, 45(1), 11–20 CrossRef CAS PubMed.
- J. Lemaire, V. Mora, P. Faure, K. Hanna, M. Buès and M. O. Simonnot, Chemical oxidation efficiency for aged, PAH-contaminated sites: An investigation of limiting factors, J. Environ. Chem. Eng., 2019, 7(3), 103061 CrossRef CAS.
- L. Nemček and I. Hagarová, The recent strategies employed in chemical analysis of contaminated waters, sediments and soils as a part of the remediation process: Extraction, Environ. Pollut Remed., 2021, 131–173 Search PubMed.
- S. Manzetti, Polycyclic aromatic hydrocarbons in the environment: environmental fate and transformation, Polycyclic Aromat. Compd., 2013, 33(4), 311–330 CrossRef CAS.
- A. Krzyszczak and B. Czech, Occurrence and toxicity of polycyclic aromatic hydrocarbons derivatives in environmental matrices, Sci. Total Environ., 2021, 788, 147738 CrossRef CAS PubMed.
- S. Lundstedt, B. A. Bandowe, W. Wilcke, E. Boll, J. H. Christensen, J. Vila, M. Grifoll, P. Faure, C. Biache, C. Lorgeoux and M. Larsson, First intercomparison study on the analysis of oxygenated polycyclic aromatic hydrocarbons (oxy-PAHs) and nitrogen heterocyclic polycyclic aromatic compounds (N-PACs) in contaminated soil, Trac. Trends Anal. Chem., 2014, 57, 83–92 CrossRef CAS.
- A. L. Knecht, B. C. Goodale, L. Truong, M. T. Simonich, A. J. Swanson, M. M. Matzke, K. A. Anderson, K. M. Waters and R. L. Tanguay, Comparative developmental toxicity of environmentally relevant oxygenated PAHs, Toxicol. Appl. Pharmacol., 2013, 271(2), 266–275 CrossRef CAS PubMed.
- M. R. Elie, J. Choi, Y. M. Nkrumah-Elie, G. D. Gonnerman, J. F. Stevens and R. L. Tanguay, Metabolomic analysis to define and compare the effects of PAHs and oxygenated PAHs in developing zebrafish, Environ. Res., 2015, 140, 502–510 CrossRef CAS PubMed.
- E. Øya, J. Øvrevik, V. M. Arlt, E. Nagy, D. H. Phillips and J. A. Holme, DNA damage and DNA damage response in human bronchial epithelial BEAS-2B cells following exposure to 2-nitrobenzanthrone and 3-nitrobenzanthrone: role in apoptosis, Mutagenesis, 2011, 26(6), 697–708 CrossRef PubMed.
- M. Wang, N. Luo, Y. Gao, G. Li and T. An, Pyrene and its derivatives increase lung adverse effects by activating aryl hydrocarbon receptor transcription, Sci. Total Environ., 2024, 916, 170030 CrossRef CAS PubMed.
- A. Dipple and T. A. Slade, Structure and activity in chemical carcinogenesis: Reactivity and carcinogenicity of 7-bromomethylbenz [a] anthracene and 7-bromomethyl-12-methylbenz [a] anthracene, Eur. J. Cancer, 1970, 6(5), 417–423 CrossRef CAS PubMed.
- D. Ghosal, S. Ghosh, T. K. Dutta and Y. Ahn, Current state of knowledge in microbial degradation of polycyclic aromatic hydrocarbons (PAHs): a review, Front. Microbiol., 2016, 7, 1369 Search PubMed.
- T. Sayara and A. Sánchez, Bioremediation of PAH-contaminated soils: process enhancement through composting/compost, Appl. Sci., 2020, 10(11), 3684 CrossRef CAS.
- M. Megharaj and R. Naidu, Soil and brownfield bioremediation, Microb. Biotechnol., 2017, 10(5), 1244–1249 CrossRef CAS PubMed.
- C. Dai, Y. Han, Y. Duan, X. Lai, R. Fu, S. Liu, K. H. Leong, Y. Tu and L. Zhou, Review on the contamination and remediation of polycyclic aromatic hydrocarbons (PAHs) in coastal soil and sediments, Environ. Res., 2022, 205, 112423 CrossRef CAS PubMed.
- J. K. Bwapwa, Factors affecting the bioremediation of industrial and domestic wastewaters, in Microbial Biodegradation and Bioremediation, Elsevier, 2022, pp. 461–472 Search PubMed.
- S. Bala, D. Garg, B. V. Thirumalesh, M. Sharma, K. Sridhar, B. S. Inbaraj and M. Tripathi, Recent strategies for bioremediation of emerging pollutants: a review for a green and sustainable environment, Toxics, 2022, 10(8), 484 CrossRef CAS PubMed.
- L. P. Singha and P. Pandey, Rhizosphere assisted bioengineering approaches for the mitigation of petroleum hydrocarbons contamination in soil, Crit. Rev. Biotechnol., 2021, 41(5), 749–766 CrossRef CAS PubMed.
- A. Das, N. Das, J. Rajkumari, P. Pandey and P. Pandey, Exploring the bioremediation potential of Bacillus spp. for sustainable mitigation of hydrocarbon contaminants, Environ. Sustain., 2024, 7(2), 135–156 CrossRef CAS.
- S. Das and H. R. Dash, Microbial bioremediation: A potential tool for restoration of contaminated areas, in Microbial Biodegradation and Bioremediation, Elsevier, 2014, pp. 1–21 Search PubMed.
- A. Yan, Y. Wang, S. N. Tan, M. L. Mohd Yusof, S. Ghosh and Z. Chen, Phytoremediation: a promising approach for revegetation of heavy metal-polluted land, Front. Plant Sci., 2020, 11, 359 CrossRef PubMed.
- J. Bramley-Alves, J. Wasley, C. K. King, S. Powell and S. A. Robinson, Phytoremediation of hydrocarbon contaminants in subantarctic soils: an effective management option, J. Environ. Manag., 2014, 142, 60–69 CrossRef CAS PubMed.
- D. Singh, S. K. Singh, V. K. Singh, H. Verma, M. Mishra, K. Rashmi and A. Kumar, Plant Growth-promoting Bacteria: Application in Bioremediation of Salinity and Heavy Metal–Contaminated Soils, in Microbe Mediated Remediation of Environmental Contaminants, ed. A., Kumar, V. K., Singh, P., Singh, V. K., Mishra, Woodhead Publishing, Sawston, UK, 2021, pp. 73–78 Search PubMed.
- S. Annamalai and M. Sundaram, Electro-bioremediation: an advanced remediation technology for the treatment and management of contaminated soil. Bioremediation of Industrial Waste for Environmental Safety: Volume II: Biological Agents and Methods for Industrial, Waste Manage., 2020, 183–214 Search PubMed.
- Z. Li and X. Li, Bibliometric analysis and systematic review on the electrokinetic remediation of contaminated soil and sediment, Environ. Geochem. Health, 2025, 47(1), 1–23 CrossRef PubMed.
- J. Dionísio, N. Couto, P. Guedes, C. Gonçalves and A. B. Ribeiro, Electrokinetic-Phytoremediation of Mixed Contaminants in Soil, Electrokinetic Remediation for Environmental Security and Sustainability, 2021, pp. 271–286 Search PubMed.
- K. Lakhan and N. Bharadvaja, Enzymatic bioremediation: a smart tool to fight environmental pollutants, in Smart Bioremediation Technologies ed. P. Bhatt, Academic Press, 2019, pp. 99–118, DOI:10.1016/B978-0-12-818307-6.00006-8.
- M. A. Ramadan, M. H. Abd-Alla and U. M. Abdul-Raouf, Bioelectricity generation from agro-industrial waste water using dual-chambered microbial fuel cell, Int. J. Sci. Res. Eng. Trends., 2020, 6(1), 103–109 Search PubMed.
- P. Srivastava, R. Abbassi, V. Garaniya, T. Lewis and A. K. Yadav, Performance of pilot-scale horizontal subsurface flow constructed wetland coupled with a microbial fuel cell for treating wastewater, J. Water Proc. Eng., 2020, 33, 100994 CrossRef.
- A. P. Ingle, A. B. Seabra, N. Duran and M. Rai, Nanoremediation: a new and emerging technology for the removal of toxic contaminant from environment, in Microbial Biodegradation and Bioremediation, Elsevier, 2014, pp. 233–250 Search PubMed.
- C. B. Chikere, C. C. Azubuike and E. M. Fubara, Shift in microbial group during remediation by enhanced natural attenuation (RENA) of a crude oil-impacted soil: a case study of Ikarama Community, Bayelsa, Nigeria, 3 Biotech, 2017, 7(2), 152 CrossRef PubMed.
- A. B. Patel, S. Singh, A. Patel, K. Jain, S. Amin and D. Madamwar, Synergistic biodegradation of phenanthrene and fluoranthene by mixed bacterial cultures, Bioresour. Technol., 2019, 284, 115–120 CrossRef CAS PubMed.
- Y. Chen, Y. Liu, Y. Li, Y. Wu, Y. Chen, G. Zeng, J. Zhang and H. Li, Influence of biochar on heavy metals and microbial community during composting of river sediment with agricultural wastes, Bioresour. Technol., 2017, 243, 347–355 CrossRef CAS PubMed.
- B. Antizar-Ladislao, J. Lopez-Real and A. Beck, Bioremediation of polycyclic aromatic hydrocarbon (PAH)-contaminated waste using composting approaches, Crit. Rev. Environ. Sci. Technol., 2004, 34(3), 249–289 CrossRef CAS.
- S. V. Mohan, M. Ramakrishna, S. Shailaja and P. N. Sarma, Influence of soil–water ratio on the performance of slurry phase bioreactor treating herbicide contaminated soil, Bioresour. Technol., 2007, 98(13), 2584–2589 CrossRef PubMed.
- S. Kuppusamy, P. Thavamani, K. Venkateswarlu, Y. B. Lee, R. Naidu and M. Megharaj, Remediation approaches for polycyclic aromatic hydrocarbons (PAHs) contaminated soils: Technological constraints, emerging trends and future directions, Chemosphere, 2017, 168, 944–968 CrossRef CAS PubMed.
- G. Guo, F. Tian, K. Ding, L. Wang, T. Liu and F. Yang, Effect of a bacterial consortium on the degradation of polycyclic aromatic hydrocarbons and bacterial community composition in Chinese soils, Int. Biodeterior. Biodegrad., 2017, 123, 56–62 CrossRef CAS.
- B. Xiong, Y. Zhang, Y. Hou, H. P. Arp, B. J. Reid and C. Cai, Enhanced biodegradation of PAHs in historically contaminated soil by M. gilvum inoculated biochar, Chemosphere, 2017, 182, 316–324 CrossRef CAS PubMed.
- G. Zafra, Á. E. Absalón, M. Á. Anducho-Reyes, F. J. Fernandez and D. V. Cortés-Espinosa, Construction of PAH-degrading mixed microbial consortia by induced selection in soil, Chemosphere, 2017, 172, 120–126 CrossRef CAS PubMed.
- N. Das, A. Das, S. Das, V. Bhatawadekar, P. Pandey, K. Choure, S. Damare and P. Pandey, Petroleum hydrocarbon catabolic pathways as targets for metabolic engineering strategies for enhanced bioremediation of crude-oil-contaminated environments, Fermentation, 2023, 9(2), 196 CrossRef CAS.
- P. A. Mendy and M. E. Entonu, Bioremediation of heavy metal ions from contaminated soil and water by microbes: A review, Int. Biodeterior. Biodegrad., 2021, 3(2), 1–8 CAS.
- A. Roy, P. Sar, J. Sarkar, A. Dutta, P. Sarkar, A. Gupta, B. Mohapatra, S. Pal and S. K. Kazy, Petroleum hydrocarbon rich oil refinery sludge of North-East India harbours anaerobic, fermentative, sulfate-reducing, syntrophic and methanogenic microbial populations, BMC Microbiol., 2018, 18, 1–22 CrossRef PubMed.
- M. Mahjoubi, A. Jaouani, A. Guesmi, S. B. Amor, A. Jouini, H. Cherif, A. Najjari, A. Boudabous, N. Koubaa and A. Cherif, Hydrocarbonoclastic bacteria isolated from petroleum contaminated sites in Tunisia: isolation, identification and characterization of the biotechnological potential, New Biotechnol., 2013, 30(6), 723–733 CrossRef CAS PubMed.
- C. P. Okafor, N. L. Udemang, C. B. Chikere, O. Akaranta and K. Ntushelo, Indigenous microbial strains as bioresource for remediation of chronically polluted Niger Delta soils, Sci. Afr., 2021, 11, e00682 CAS.
- O. N. Ruiz, L. M. Brown, O. Radwan, L. L. Bowen, T. S. Gunasekera, S. S. Mueller, Z. J. West and R. C. Striebich, Metagenomic characterization reveals complex association of soil hydrocarbon-degrading bacteria, Int. Biodeterior. Biodegrad., 2021, 157, 105161 CrossRef CAS.
- S. Fuentes, B. Barra, J. G. Caporaso and M. Seeger, From rare to dominant: a fine-tuned soil bacterial bloom during petroleum hydrocarbon bioremediation, Appl. Environ. Microbiol., 2016, 82(3), 888–896 CrossRef CAS PubMed.
- I. Sharma, Bioremediation Techniques for Polluted Environment: Concept, Advantages, Limitations, and Prospects, Trace Metals in the Environment - New Approaches and Recent Advances, IntechOpen, 2021, DOI:10.5772/intechopen.90453.
- D. Kour, T. Kaur, R. Devi, A. Yadav, M. Singh, D. Joshi, J. Singh, D. C. Suyal, A. Kumar, V. D. Rajput and A. N. Yadav, Beneficial microbiomes for bioremediation of diverse contaminated environments for environmental sustainability: present status and future challenges, Environ. Sci. Pollut. Res., 2021, 28, 24917–24939 CrossRef CAS PubMed.
- S. Srivastava, M. Kumar, Biodegradation of polycyclic aromatic hydrocarbons (PAHs): a sustainable approach, Sustainable Green Technologies for Environmental Management, 2019, pp. 111–139 Search PubMed.
- M. A. Baboshin and L. A. Golovleva, Aerobic bacterial degradation of polycyclic aromatic hydrocarbons (PAHs) and its kinetic aspects, Microbiology, 2012, 81, 639–650 CAS.
- R. U. Meckenstock, M. Safinowski and C. Griebler, Anaerobic degradation of polycyclic aromatic hydrocarbons, FEMS Microbiol. Ecol., 2004, 49(1), 27–36 CrossRef CAS PubMed.
- A. Imam, S. K. Suman, P. K. Kanaujia and A. Ray, Biological machinery for polycyclic aromatic hydrocarbons degradation: A review, Bioresour. Technol., 2022, 343, 126121 CrossRef CAS PubMed.
- E. Pandolfo, A. Barra Caracciolo and L. Rolando, Recent advances in bacterial degradation of hydrocarbons, Water, 2023, 15(2), 375 CrossRef CAS.
- E. Barnsley, Naphthalene metabolism by pseudomonads: The oxidation of 1, 2-dihydroxynaphthalene to 2-hydroxychromene-2-carboxylic acid and the formation of 2′-hydroxybenzalpyruvate, Biochem. Biophys. Res. Commun., 1976, 72(3), 1116–1121 CrossRef CAS PubMed.
- D. Kregiel, Succinate Dehydrogenase of Saccharomyces cerevisiae – The Unique Enzyme of TCA Cycle – Current Knowledge and New Perspectives, Dehydrogenases, InTech, 2012, DOI:10.5772/48413.
- A. A. Aghapour, G. Moussavi and K. Yaghmaeian, Biological degradation of catechol in wastewater using the sequencing continuous-inflow reactor (SCR), J. Environ. Health Sci. Eng., 2013, 11, 1 CrossRef PubMed.
- I. Susilowati, M. Sholeh, N. R. Yunus and D. A. Ainaya, The Role of the United Nations Environment Program (UNEP) In Overcoming Deforestation In Central Kalimantan 2017-2020, in IOP Conference Series: Earth and Environmental Science, IOP Publishing, 2024, vol. 1323, 1, p. 012017 Search PubMed.
- C. Hernandez, F. S. Lopez and F. A. Pacheco, The sustainable development goals index: An analysis (2000-2022), Transdiscipl. J. Eng. Sci., 2022, 13, 111–128 Search PubMed.
- N. K. Arora, T. Fatima, I. Mishra, M. Verma, J. Mishra and V. Mishra, Environmental sustainability: challenges and viable solutions, Environ. Sustain., 2018, 1, 309–340 CrossRef.
- V. Pande, S. C. Pandey, D. Sati, V. Pande and M. Samant, Bioremediation: an emerging effective approach towards environment restoration, Environ. Sustain., 2020, 3, 91–103 CrossRef CAS.
- S. Bisht, P. Pandey, B. Bhargava, S. Sharma, V. Kumar and K. D. Sharma, Bioremediation of polyaromatic hydrocarbons (PAHs) using rhizosphere technology, Braz. J. Microbiol., 2015, 46, 7–21 CrossRef CAS PubMed.
- H. H. Liste and M. Alexander, Plant-promoted pyrene degradation in soil, Chemosphere, 2000, 40(1), 7–10 CrossRef CAS PubMed.
- R. Rostami, A. Zarei, B. Saranjam, H. R. Ghaffari, S. Hazrati, Y. Poureshg and M. Fazlzadeh, Exposure and risk assessment of PAHs in indoor air of waterpipe cafés in Ardebil, Iran, Build. Environ., 2019, 155, 47–57 CrossRef.
- R. Zainab, M. Hasnain, F. Ali, D. A. Dias, A. El-Keblawy and Z. Abideen, Exploring the bioremediation capability of petroleum-contaminated soils for enhanced environmental sustainability and minimization of ecotoxicological concerns, Environ. Sci. Pollut. Res., 2023, 30(48), 104933–104957 CrossRef CAS PubMed.
- Q. Saeed, W. Xiukang, F. U. Haider, J. Kučerik, M. Z. Mumtaz, J. Holatko, M. Naseem, A. Kintl, M. Ejaz, M. Naveed and M. Brtnicky, Rhizosphere bacteria in plant growth promotion, biocontrol, and bioremediation of contaminated sites: a comprehensive review of effects and mechanisms, Int. J. Mol. Sci., 2021, 22(19), 10529 CrossRef CAS PubMed.
- B. Bhuyan and P. Pandey, Remediation of petroleum hydrocarbon contaminated soil using hydrocarbonoclastic rhizobacteria, applied through Azadirachta indica rhizosphere, Int. J. Phytoremediation, 2022, 24(13), 1444–1454 CrossRef CAS PubMed.
- M. Shah and S. Ahmed, Bioremediation potential of rhizosphere microbes—current perspectives, Rhizobiome, 2023, 81–94 Search PubMed.
- S. Zhang, P. B. Gedalanga and S. Mahendra, Advances in bioremediation of 1, 4-dioxane-contaminated waters, J. Environ. Manag., 2017, 204, 765–774 CrossRef CAS PubMed.
- R. Z. Sayyed, S. Seifi, P. R. Patel, S. S. Shaikh, H. P. Jadhav and H. E. Enshasy, Siderophore production in groundnut rhizosphere isolate, Achromobacter sp. RZS2 influenced by physicochemical factors and metal ions, Environ. Sustain., 2019, 2(2), 117–124 CrossRef CAS.
- S. M. Dehnavi and G. Ebrahimipour, Comparative remediation rate of biostimulation, bioaugmentation, and phytoremediation in hydrocarbon contaminants, Int. J. Environ. Sci. Technol., 2022, 19(11), 11561–11586 CrossRef CAS.
- Y. Huang, H. Pan, Q. Wang, Y. Ge, W. Liu and P. Christie, Enrichment of the soil microbial community in the bioremediation of a petroleum-contaminated soil amended with rice straw or sawdust, Chemosphere, 2019, 224, 265–271 CrossRef CAS PubMed.
- H. Li, Y. Li, M. Bao and S. Li, Solid inoculants as a practice for bioaugmentation to enhance bioremediation of hydrocarbon contaminated areas, Chemosphere, 2021, 263, 128175 CrossRef CAS PubMed.
- N. Haleyur, E. Shahsavari, S. S. Jain, E. Koshlaf, V. B. Ravindran, P. D. Morrison, A. M. Osborn and A. S. Ball, Influence of bioaugmentation and biostimulation on PAH degradation in aged contaminated soils: response and dynamics of the bacterial community, J. Environ. Manag., 2019, 238, 49–58 CrossRef CAS PubMed.
- R. Liu, L. Zhao, C. Jin, N. Xiao, R. N. Jadeja and T. Sun, Enzyme responses to phytoremediation of PAH-contaminated soil using Echinacea purpurea (L.), Water, Air, Soil Pollut., 2014, 225, 1 Search PubMed.
- R. Liu, L. Zhao, C. Jin, N. Xiao, R. N. Jadeja and T. Sun, Enzyme responses to phytoremediation of PAH-contaminated soil using Echinacea purpurea (L.), Water, Air, Soil Pollut., 2014, 225, 1 Search PubMed.
- B. Cai, J. Ma, G. Yan, X. Dai, M. Li and S. Guo, Comparison of phytoremediation, bioaugmentation and natural attenuation for remediating saline soil contaminated by heavy crude oil, Biochem. Eng. J., 2016, 112, 170–177 CrossRef CAS.
- H. Ikeura, Y. Kawasaki, E. Kaimi, J. Nishiwaki, K. Noborio and M. Tamaki, Screening of plants for phytoremediation of oil-contaminated soil, Int. J. Phytoremediation, 2016, 18(5), 460–466 CrossRef CAS PubMed.
- P. Rani, P. K. Rose, M. K. Kidwai and S. Meenakshi, Brassica Juncea L.: a potential crop for phytoremediation of various heavy metals in Heavy Metal Toxicity: Environmental Concerns, Remediation and Opportunities, Springer Nature Singapore, Singapore, 2023, pp. 285–311 Search PubMed.
- T. M. Galal, E. M. Eid, M. A. Dakhil and L. M. Hassan, Bioaccumulation and rhizofiltration potential of Pistia stratiotes L. for mitigating water pollution in the Egyptian wetlands, Int. J. Phytoremediation, 2018, 20(5), 440–447 CrossRef CAS PubMed.
- A. Cristaldi, G. O. Conti, E. H. Jho, P. Zuccarello, A. Grasso, C. Copat and M. Ferrante, Phytoremediation of Contaminated Soils by Heavy Metals and PAHs. A Brief Review, Environmental Technology & Innovation., 2017, vol. 8, pp. 309–326 Search PubMed.
- W. Doucette, H. Klein, J. Chard, R. Dupont, W. Plaehn and B. Bugbee, Volatilization of trichloroethylene from trees and soil: Measurement and scaling approaches, Environ. Sci. Technol., 2013, 47(11), 5813–5820 CrossRef CAS PubMed.
- L. C. Marr, E. C. Booth, R. G. Andersen, M. A. Widdowson and J. T. Novak, Direct volatilization of naphthalene to the atmosphere at a phytoremediation site, Environ. Sci. Technol., 2006, 40(17), 5560–5566 CrossRef CAS PubMed.
- D. C. Yee, J. A. Maynard and T. K. Wood, Rhizoremediation of trichloroethylene by a recombinant, root-colonizing Pseudomonas fluorescens strain expressing toluene ortho-monooxygenase constitutively, Appl. Environ. Microbiol., 1998, 64(1), 112–118 CrossRef CAS PubMed.
- M. P. Maila, P. Randima and T. E. Cloete, Multispecies and monoculture rhizoremediation of polycyclic aromatic hydrocarbons (PAHs) from the soil, Int. J. Phytoremediation, 2005, 7(2), 87–98 CrossRef CAS PubMed.
- A. Y. Muratova, O. V. Turkovskaya, T. Hübner and P. Kuschk, Studies of the efficacy of alfalfa and reed in the phytoremediation of hydrocarbon-polluted soil, Appl. Biochem. Microbiol., 2003, 39, 599–605 CrossRef CAS.
- K. Velmourougane, G. Saxena, R. Prasanna, Plant-microbe interactions in the rhizosphere: mechanisms and their ecological benefits, Plant-Microbe Interactions in Agro-Ecological Perspectives, Microbial Interactions and Agro-Ecological Impacts, vol. 2, 2017, pp. 193–219 Search PubMed.
- P. Agarwal, B. S. Giri and R. Rani, Unravelling the role of rhizospheric plant-microbe synergy in phytoremediation: a genomic perspective, Curr. Genomics, 2020, 21(5), 334–342 CrossRef CAS PubMed.
- J. Bramley-Alves, J. Wasley, C. K. King, S. Powell and S. A. Robinson, Phytoremediation of hydrocarbon contaminants in
subantarctic soils: an effective management option, J. Environ. Manag., 2014, 142, 60–69 CrossRef CAS PubMed.
- J. Hou, W. Liu, B. Wang, Q. Wang, Y. Luo and A. E. Franks, PGPR enhanced phytoremediation of petroleum contaminated soil and rhizosphere microbial community response, Chemosphere, 2015, 138, 592–598 CrossRef CAS PubMed.
- R. Kotoky and P. Pandey, Difference in the rhizosphere microbiome of Melia azedarach during removal of benzo (a) pyrene from cadmium co-contaminated soil, Chemosphere, 2020, 258, 127175 CrossRef CAS PubMed.
- C. J. Sampaio, J. R. de Souza, A. O. Damião, T. C. Bahiense and M. R. Roque, Biodegradation of polycyclic aromatic hydrocarbons (PAHs) in a diesel oil-contaminated mangrove by plant growth-promoting rhizobacteria, 3 Biotech, 2019, 9, 1 CrossRef PubMed.
- S. Mukhopadhyay, J. George and R. E. Masto, Changes in polycyclic aromatic hydrocarbons (PAHs) and soil biological parameters in a revegetated coal mine spoil, Land Degrad. Dev., 2017, 28(3), 1047–1055 CrossRef.
- A. K. Sivaram, P. Logeshwaran, R. Lockington, R. Naidu and M. Megharaj, Low molecular weight organic acids enhance the high molecular weight polycyclic aromatic hydrocarbons degradation by bacteria, Chemosphere, 2019, 222, 132–140 CrossRef CAS PubMed.
- L. P. Singha, N. Sinha and P. Pandey, Rhizoremediation prospects of polyaromatic hydrocarbon degrading rhizobacteria, that facilitate glutathione and glutathione-S-transferase mediated stress response, and enhance growth of rice plants in pyrene contaminated soil, Ecotoxicol. Environ. Saf., 2018, 164, 579–588 CrossRef CAS PubMed.
- R. Kotoky, S. Nath, D. Kumar Maheshwari and P. Pandey, Cadmium resistant plant growth promoting rhizobacteria Serratia marcescens S2I7 associated with the growth promotion of rice plant, Environ. Sustain., 2019, 2, 135–144 CrossRef CAS.
- S. Mukhopadhyay and R. E. Masto, Effect of fly ash on carbon mineralization of biochar and organic manures added to mine spoil, SN Appl. Sci., 2019, 1(9), 1119 CrossRef CAS.
- K. Somtrakoon, C. Thala, C. Thinnok, C. Thumjan and W. Chouychai, Using plant growth regulators to stimulate growth of napier grass under atrazine contamination, Soil Sediment Contam., 2024, 33(8), 1235–1251 CrossRef.
- X. Zhao, X. Cheng, X. Cai, S. Wang, J. Li, Y. Dai, L. Jiang, C. Luo and G. Zhang, SIP-metagenomics reveals key drivers of rhizospheric Benzo [a] pyrene bioremediation via bioaugmentation with indigenous soil microbes, Environ. Pollut., 2024, 360, 124620 CrossRef CAS PubMed.
- A. Gawryluk, A. Stępniowska and H. Lipińska, Effect of soil contamination with polycyclic aromatic hydrocarbons from drilling waste on germination and growth of lawn grasses, Ecotoxicol. Environ. Saf., 2022, 236, 113492 CrossRef CAS PubMed.
- J. H. Gao, Y. X. Mu, Y. L. Zhang, Z. S. Ge, W. Yan and H. M. Shao, Effects of exogenous nitric oxide on the growth and physiological characteristics of perennial ryegrass seedlings under osmotic stress, Acta Agrestia Sin., 2011, 19(4), 625 CAS.
- X. Ma, X. Li, J. Liu, Y. Cheng, J. Zou, F. Zhai, Z. Sun and L. Han, Soil microbial community succession and interactions during combined plant/white-rot fungus remediation of polycyclic aromatic hydrocarbons, Sci. Total Environ., 2021, 752, 142224 CrossRef CAS PubMed.
- A. Su, Y. Xu, M. Xu, S. Ding, M. Li and Y. Zhang, Resilience of the wheat root-associated microbiome to the disturbance of phenanthrene, Sci. Total Environ., 2022, 838, 156487 CrossRef CAS PubMed.
- Z. Huang, L. Jiang, W. Lu, C. Luo and M. Song, Elsholtzia splendens promotes phenanthrene and polychlorinated biphenyl degradation under Cu stress through enrichment of microbial degraders, J. Hazard. Mater., 2022, 438, 129492 CrossRef CAS PubMed.
- M. Bouri, S. Mehnaz and F. Şahin, Extreme environments as potential sources for PGPR, in Secondary Metabolites and Volatiles of PGPR in Plant-Growth Promotion, Springer International Publishing, Cham, 2022, pp. 249–276 Search PubMed.
- G. Malik, S. Hooda, S. Majeed and V. C. Pandey, Understanding assisted phytoremediation: potential tools to enhance plant performance, in Assisted Phytoremediation, V. Pandey, Elsevier, 2022, pp. 1–24, ISBN: 9780128228937, DOI:10.1016/B978-0-12-822893-7.00015-X.
- P. J. Yesankar, M. Pal, A. Patil and A. Qureshi, Microbial exopolymeric substances and biosurfactants as ‘bioavailability enhancers’ for polycyclic aromatic hydrocarbons biodegradation, Int. J. Environ. Sci. Technol., 2023, 20(5), 5823–5844 CrossRef CAS.
- S. S. Mohapatra, A. Ray, S. Panda, S. Satapathy and N. Moharana, Arbuscular Mycorrhizal Fungi and Their Association for Bioremediation in Rice Cultivation, in Arbuscular Mycorrhizal Fungi, CRC Press, 2023, pp. 123–129 Search PubMed.
- D. Zuzolo, R. Sciarrillo, A. Postiglione and C. Guarino, The remediation potential for PAHs of Verbascum sinuatum L. combined with an enhanced rhizosphere landscape: A full-scale mesocosm experiment, Biotechnol. Rep., 2021, 31, e00657 CrossRef CAS PubMed.
- S. Rostami, A. Azhdarpoor, M. Rostami, F. Mohammadi, M. Jaskulak, M. Dehghani, M. R. Samaei and M. A. Baghapour, Improvement of the Rhizoremediation efficiency of PAHs contaminated soil under cysteine treatment along with modeling, Environ. Nanotechnol. Monit. Manag., 2021, 16, 100519 CAS.
- R. Mehmannavaz, S. O. Prasher and D. Ahmad, Rhizospheric effects of alfalfa on biotransformation of polychlorinated biphenyls in a contaminated soil augmented with Sinorhizobium meliloti, Process Biochem., 2002, 37(9), 955–963 CrossRef CAS.
- Y. Teng, X. Wang, L. Li, Z. Li and Y. Luo, Rhizobia and their bio-partners as novel drivers for functional remediation in contaminated soils, Front. Plant Sci., 2015, 6, 32 Search PubMed.
- A. K. Sivaram, S. R. Subashchandrabose, P. Logeshwaran, R. Lockington, R. Naidu and M. Megharaj, Rhizodegradation of PAHs differentially altered by C3 and C4 plants, Sci. Rep., 2020, 10(1), 16109 CrossRef CAS PubMed.
- G. Adam and H. Duncan, Development of a sensitive and rapid method for the measurement of total microbial activity using fluorescein diacetate (FDA) in a range of soils, Soil Biol. Biochem., 2001, 33(7–8), 943–951 CrossRef CAS.
- M. O. Eze, G. C. Hose, S. C. George and R. Daniel, Diversity and metagenome analysis of a hydrocarbon-degrading bacterial consortium from asphalt lakes located in Wietze, Germany, AMB Express, 2021, 11(1), 89 CrossRef CAS PubMed.
- A. Iannucci, L. Canfora, F. Nigro, P. De Vita and R. Beleggia, Relationships between root morphology, root exudate compounds and rhizosphere microbial community in durum wheat, Appl. Soil Ecol., 2021, 158, 103781 CrossRef.
- A. Canarini, C. Kaiser, A. Merchant, A. Richter and W. Wanek, Root exudation of primary metabolites: mechanisms and their roles in plant responses to environmental stimuli, Front. Plant Sci., 2019, 10, 157 CrossRef PubMed.
- R. Mendes, P. Garbeva and J. M. Raaijmakers, The rhizosphere microbiome: significance of plant beneficial, plant pathogenic, and human pathogenic microorganisms, FEMS Microbiol. Rev., 2013, 37(5), 634–663 CrossRef CAS PubMed.
- B. Bhuyan, R. Kotoky, D. K. Maheshwari and P. Pandey, Rhizoremediation of Cd-contaminated soil using Zea mays Sturt, with heavy metal resistant rhizobacteria that alleviate Cd-induced stress in plant, Environ. Sustain., 2022, 5(3), 375–387 CrossRef CAS.
- R. Kotoky, N. Ogawa and P. Pandey, The structure-function relationship of bacterial transcriptional regulators as a target for enhanced biodegradation of aromatic hydrocarbons, Microbiol. Res., 2022, 262, 127087 CrossRef CAS PubMed.
- S. Hayat, A. Faraz and M. Faizan, Root exudates: composition and impact on plant–microbe interaction, Biofilms in Plant and Soil Health, 2017, 18, pp. 179–193 Search PubMed.
- J. Rajkumari, Y. Choudhury, K. Bhattacharjee and P. Pandey, Rhizodegradation of pyrene by a non-pathogenic Klebsiella pneumoniae isolate applied
with Tagetes erecta l. and changes in the rhizobacterial community, Front. Microbiol., 2021, 12, 593023 CrossRef PubMed.
- J. Jin, M. Wang, W. Lu, L. Zhang, Q. Jiang, Y. Jin, K. Lu, S. Sun, Q. Cao, Y. Wang and M. Xiao, Effect of plants and their root exudate on bacterial activities during rhizobacterium–plant remediation of phenol from water, Environ. Int., 2019, 127, 114–124 CrossRef CAS PubMed.
- I. Mishra and N. K. Arora, Rhizoremediation: a sustainable approach to improve the quality and productivity of polluted soils, in Phyto and Rhizo Remediation, Springer, Singapore, 2019, pp. 33–66 Search PubMed.
- L. Muungo, Root exudation the ecological driver of hydrocarbon rhizoremediation, PsyArXiv, 2020, DOI:10.31234/osf.io/yujae.
- B. Bhuyan, S. Debnath and P. Pandey, The rhizosphere microbiome and its role in plant growth in stressed conditions, Rhizosphere Microbes: Soil and Plant Functions, 2020, pp. 503–529 Search PubMed.
- J. Li, C. Luo, D. Zhang, X. Cai, L. Jiang, X. Zhao and G. Zhang, Diversity of the active phenanthrene degraders in PAH-polluted soil is shaped by ryegrass rhizosphere and root exudates, Soil Biol. Biochem., 2019, 128, 100–110 CrossRef CAS.
- E. Yergeau, C. Michel, J. Tremblay, A. Niemi, T. L. King, J. Wyglinski, K. Lee and C. W. Greer, Metagenomic survey of the taxonomic and functional microbial communities of seawater and sea ice from the Canadian Arctic, Sci. Rep., 2017, 7(1), 42242 CrossRef CAS PubMed.
- A. Muratova, E. Dubrovskaya, S. Golubev, V. Grinev, M. Chernyshova and O. Turkovskaya, The coupling of the plant and microbial catabolisms of phenanthrene in the rhizosphere of Medicago sativa, J. Plant Physiol., 2015, 188, 1–8 CrossRef CAS PubMed.
- L. Jiang, C. Luo, D. Zhang, M. Song, W. Mei, Y. Sun and G. Zhang, Shifts in a phenanthrene-degrading microbial community are driven by carbohydrate metabolism selection in a ryegrass rhizosphere, Environ. Sci. Technol., 2020, 55(2), 962–973 CrossRef PubMed.
- L. Song, X. Niu, Y. Tian and Y. Xiao, Assessment of PAH degradation potential of native species from a coking plant through identifying of the beneficial bacterial community within the rhizosphere soil, Chemosphere, 2021, 264, 128513 CrossRef CAS PubMed.
- S. A. Cheema, M. I. Khan, X. Tang, C. Zhang, C. Shen, Z. Malik, S. Ali, J. Yang, K. Shen, X. Chen and Y. Chen, Enhancement of phenanthrene and pyrene degradation in rhizosphere of tall fescue (Festuca arundinacea), J. Hazard. Mater., 2009, 166(2–3), 1226–1231 CrossRef CAS PubMed.
- X. F. Huang, J. M. Chaparro, K. F. Reardon, R. Zhang, Q. Shen and J. M. Vivanco, Rhizosphere interactions: root exudates, microbes, and microbial communities, Botany, 2014, 92(4), 267–275 CrossRef.
- V. N. Kimani, L. Chen, Y. Liu, W. Raza, N. Zhang, L. K. Mungai, Q. Shen and R. Zhang, Characterization of extracellular polymeric substances of Bacillus amyloliquefaciens SQR9 induced by root exudates of cucumber, J. Basic Microbiol., 2016, 56(11), 1183–1193 CrossRef CAS PubMed.
- X. Zhou and F. Wu, Vanillic acid changed cucumber (Cucumis sativus L.) seedling rhizosphere total bacterial, Pseudomonas and Bacillus spp. communities, Sci. Rep., 2018, 8(1), 4929 CrossRef PubMed.
- R. Ortiz-Castro and J. López-Bucio, Phytostimulation and root architectural responses to quorum-sensing signals and related molecules from rhizobacteria, Plant Sci., 2019, 284, 135–142 CrossRef CAS PubMed.
- S. Das, N. Das, K. Choure and P. Pandey, Biodegradation of asphaltene by lipopeptide-biosurfactant producing hydrocarbonoclastic, crude oil degrading Bacillus spp, Bioresour. Technol., 2023, 382, 129198 CrossRef CAS PubMed.
- C. H. Kong, Z. Li, F. L. Li, X. X. Xia and P. Wang, Chemically mediated plant–plant interactions: Allelopathy and allelobiosis, Plants, 2024, 13(5), 626 CrossRef CAS PubMed.
- M. Sadowsky and P. Graham, Root and stem nodule bacteria of legumes, The Prokaryotes, 2006, 2, pp. 818–841 Search PubMed.
- L. P. Singha, K. M. Singha and P. Pandey, Functionally coherent transcriptional responses of Jatropha curcas and Pseudomonas fragi for rhizosphere mediated degradation of pyrene, Sci. Rep., 2024, 14(1), 1014 CrossRef CAS PubMed.
- R. Devi, T. Kaur, D. Kour, K. L. Rana, A. Yadav and A. N. Yadav, Beneficial fungal communities from different habitats and their roles in plant growth promotion and soil health, Microb. Biosyst., 2020, 5(1), 21–47 CrossRef.
- T. Guha, P. Verma and R. Kundu, Role of Chloride and Organic Acid Anions in Environmental Stress Tolerance, in Biology and Biotechnology of Environmental Stress Tolerance in Plants, Apple Academic Press, 2023, pp. 415–472 Search PubMed.
- U. J. Ijah and S. P. Antai, Removal of Nigerian light crude oil in soil over a 12-month period, Int. Biodeterior. Biodegrad., 2003, 51(2), 93–99 CrossRef CAS.
- F. B. Tanee and P. D. Kinako, Comparative studies of biostimulation and phytoremediation in the mitigation of crude oil toxicity in tropical soil, J. Appl. Sci. Environ. Manage., 2008, 12(2), 143–147 Search PubMed.
- W. Guidi, H. Kadri and M. Labrecque, Establishment techniques to using willow for phytoremediation on a former oil refinery in southern Quebec: achievements and constraints, Chem. Ecol., 2012, 28(1), 49–64 CrossRef.
- M. T. Balba, R. Al-Daher, N. Al-Awadhi, H. Chino and H. Tsuji, Bioremediation of oil-contaminated desert soil: the Kuwaiti experience, Environ. Int., 1998, 24(1–2), 163–173 CrossRef CAS.
- C. C. Chukwuma, J. C. Ikewuchi and M. O. Monanu, Removal of hydrocarbons from crude oil contaminated agricultural soil by phytoremediation using Mariscus alternifolius and Fimbristylis ferruginea, Eur. J. Biol. Chem. Res., 2019, 9(1), 34–44 CAS.
- D. Techer, C. Martinez-Chois, P. Laval-Gilly, S. Henry, A. Bennasroune, M. D’innocenzo and J. Falla, Assessment of Miscanthus× giganteus for rhizoremediation of long term PAH contaminated soils, Appl. Soil Ecol., 2012, 62, 42–49 CrossRef.
- M. Sun, D. Fu, Y. Teng, Y. Shen, Y. Luo, Z. Li and P. Christie, In situ phytoremediation of PAH-contaminated soil by intercropping alfalfa (Medicago sativa L.) with tall fescue (Festuca arundinacea Schreb.) and associated soil microbial activity, J. Soils Sediments, 2011, 11, 980–989 CrossRef CAS.
- W. A. Yongshi, H. A. Xuefeng and H. U. Yang, Orderly distribution and differential enrichment of hydrocarbon in oil-rich sags: A case study of Dongying Sag, Jiyang Depression, Bohai Bay Basin, East China, Petrol. Explor. Dev., 2018, 45(5), 840–850 CrossRef.
- S. Bisht, P. Pandey, G. Kaur, H. Aggarwal, A. Sood, S. Sharma, V. Kumar and N. S. Bisht, Utilization of endophytic strain Bacillus sp. SBER3 for biodegradation of polyaromatic hydrocarbons (PAH) in soil model system, Eur. J. Soil Biol., 2014, 60, 67–76 CrossRef CAS.
- G. L. Xu, H. Liu, M. J. Li, Z. M. Li, Z. H. Peng, L. M. Zuo, X. He, W. W. Liu and L. G. Cai, In situ bioremediation of crude oil contaminated site: A case study in Jianghan oil field, China, Pet. Sci. Technol., 2016, 34(1), 63–70 CrossRef CAS.
- J. Jacobsen, I. Venables, M. B. Wang, P. Matthews, M. Ayliffe and F. Gubler, Barley (Hordeum Vulgare l.), Agrobacterium protocols, 2006, pp. 171–184 Search PubMed.
- S. L. Kelley, E. W. Aitchison, M. Deshpande, J. L. Schnoor and P. J. Alvarez, Biodegradation of 1, 4-dioxane in planted and unplanted soil: effect of bioaugmentation with Amycolata sp. CB1190, Water Res., 2001, 35(16), 3791–3800 CrossRef CAS PubMed.
- J. A. Rentz, P. J. Alvarez and J. L. Schnoor, Benzo [a] pyrene co-metabolism in the presence of plant root extracts and exudates: Implications for phytoremediation, Energy Convers. Manag., 2005, 136(3), 477–484 CAS.
- Y. Hong, D. Liao, J. Chen, S. Khan, J. Su and H. Li, A comprehensive study of the impact of polycyclic aromatic hydrocarbons (PAHs) contamination on salt marsh plants Spartina alterniflora: implication for plant-microbe interactions in phytoremediation, Environ. Sci. Pollut. Res., 2015, 22, 7071–7081 CrossRef CAS PubMed.
- L. Bardi, C. Martini, F. Opsi, E. Bertolone, S. Belviso, G. Masoero, M. Marzona and F. A. Marsan, Cyclodextrin-enhanced in situ bioremediation of polyaromatic hydrocarbons-contaminated soils and plant uptake, J. Inclusion Phenom. Macrocyclic Chem., 2007, 57, 439–444 CrossRef CAS.
- E. Knez, K. Kadac-Czapska, K. Dmochowska-Ślęzak and M. Grembecka, Root vegetables—Composition, health effects, and contaminants, Int. J. Environ. Res. Public Health, 2022, 19(23), 15531 CrossRef CAS PubMed.
- M. Pawlik, B. Cania, S. Thijs, J. Vangronsveld and Z. Piotrowska-Seget, Hydrocarbon degradation potential and plant growth-promoting activity of culturable endophytic bacteria of Lotus corniculatus and Oenothera biennis from a long-term polluted site, Environ. Sci. Pollut. Res., 2017, 24, 19640–19652 CrossRef CAS PubMed.
- I. Lenoir, A. Lounes-Hadj Sahraoui and J. Fontaine, Arbuscular mycorrhizal fungal-assisted phytoremediation of soil contaminated with persistent organic pollutants: a review, Eur. J. Soil Sci., 2016, 67(5), 624–640 CrossRef.
- J. J. Dominguez, H. P. Bacosa, M. F. Chien and C. Inoue, Enhanced degradation of polycyclic aromatic hydrocarbons (PAHs) in the rhizosphere of sudangrass (Sorghum× drummondii), Chemosphere, 2019, 234, 789–795 CrossRef CAS PubMed.
- L. P. Singha and P. Pandey, Glutathione and glutathione-S-transferase activity in Jatropha curcas in association with pyrene degrader Pseudomonas aeruginosa PDB1 in rhizosphere, for alleviation of stress induced by polyaromatic hydrocarbon for effective rhizoremediation, Ecol. Eng., 2017, 102, 422–432 CrossRef.
- J. Liu, H. Su, X. Wei and J. Xue, Progress in bioremediation of pyrene, Recent Innovations Chem. Eng., 2020, 13(2), 138–155 CAS.
- A. K. Sivaram, S. R. Subashchandrabose, P. Logeshwaran, R. Lockington, R. Naidu and M. Megharaj, Rhizodegradation of PAHs differentially altered by C3 and C4 plants, Sci. Rep., 2020, 10(1), 16109 CrossRef CAS PubMed.
- F. Hussain, I. Hussain, A. H. Khan, Y. S. Muhammad, M. Iqbal, G. Soja, T. G. Reichenauer and S. Yousaf, Combined application of biochar, compost, and bacterial consortia with Italian ryegrass enhanced phytoremediation of petroleum hydrocarbon contaminated soil, Environ. Exp. Bot., 2018, 153, 80–88 CrossRef CAS.
- F. Hussain, I. Hussain, A. H. Khan, Y. S. Muhammad, M. Iqbal, G. Soja, T. G. Reichenauer and S. Yousaf, Combined application of biochar, compost, and bacterial consortia with Italian ryegrass enhanced phytoremediation of petroleum hydrocarbon contaminated soil, Environ. Exp. Bot., 2018, 153, 80–88 CrossRef CAS.
- M. Andreolli, S. Lampis, M. Poli, G. Gullner, B. Biró and G. Vallini, Endophytic Burkholderia fungorum DBT1 can improve phytoremediation efficiency of polycyclic aromatic hydrocarbons, Chemosphere, 2013, 92(6), 688–694 CrossRef CAS PubMed.
- Y. Sun and Q. Zhou, Uptake and translocation of benzo [a] pyrene (B [a] P) in two ornamental plants and dissipation in soil, Ecotoxicol. Environ. Saf., 2016, 124, 74–81 CrossRef CAS PubMed.
- J. Wojtera-Kwiczor, W. Żukowska, W. Graj, A. Małecka, A. Piechalak, L. Ciszewska, Ł. Chrzanowski, P. Lisiecki, I. Komorowicz, D. Barałkiewicz and I. Voss, Rhizoremediation of diesel-contaminated soil with two rapeseed varieties and petroleum degraders reveals different responses of the plant defense mechanisms, Int. J. Phytoremediation, 2014, 16(7–8), 770–789 CrossRef CAS PubMed.
- C. Tu, L. Ma, P. Guo, F. Song, Y. Teng, H. Zhang and Y. Luo, Rhizoremediation of a dioxin-like PCB polluted soil by alfalfa: Dynamic characterization at temporal and spatial scale, Chemosphere, 2017, 189, 517–524 CrossRef CAS PubMed.
- X. Wang, Y. Teng, C. Tu, Y. Luo, C. Greening, N. Zhang, S. Dai, W. Ren, L. Zhao and Z. Li, Coupling between nitrogen fixation and tetrachlorobiphenyl dechlorination in a rhizobium–legume symbiosis, Environ. Sci. Technol., 2018, 52(4), 2217–2224 CrossRef CAS PubMed.
- J. Li, C. Luo, D. Zhang, X. Zhao, Y. Dai, X. Cai and G. Zhang, The catabolic pathways of in situ rhizosphere PAH degraders and the main factors driving PAH rhizoremediation in oil-contaminated soil, Environ. Microbiol., 2021, 23(11), 7042–7055 CrossRef CAS PubMed.
- P. Sharma, P. Bakshi, K. Khanna, J. Kour, D. Kapoor, A. D. Singh, T. Bhardwaj, R. Kaur, A. Sharma and R. Bhardwaj, Plant and microbe association for degradation of xenobiotics focusing transgenic plants, Handbook of Assisted and Amendment: Enhanced Sustainable Remediation Technology, 2021, pp. 501–516 Search PubMed.
- S. G. Ibáñez, M. I. Medina and E. Agostini, Phenol tolerance, changes of antioxidative enzymes and cellular damage in transgenic tobacco hairy roots colonized by arbuscular mycorrhizal fungi, Chemosphere, 2011, 83(5), 700–705 CrossRef PubMed.
- K. Huang, C. Chen, Q. Shen, B. P. Rosen and F. J. Zhao, Genetically engineering Bacillus subtilis with a heat-resistant arsenite methyltransferase for bioremediation of arsenic-contaminated organic waste, Appl. Environ. Microbiol., 2015, 81(19), 6718–6724 CrossRef CAS PubMed.
- S. Taghavi, T. Barac, B. Greenberg, B. Borremans, J. Vangronsveld and D. van der Lelie, Horizontal gene transfer to endogenous endophytic bacteria from poplar improves phytoremediation of toluene, Appl. Environ. Microbiol., 2005, 71(12), 8500–8505 CrossRef CAS PubMed.
- X. Wang, N. Wu, J. Guo, X. Chu, J. Tian, B. Yao and Y. Fan, Phytodegradation of organophosphorus compounds by transgenic plants expressing a bacterial organophosphorus hydrolase, Biochem. Biophys. Res. Commun., 2008, 365(3), 453–458 CrossRef CAS PubMed.
- R. P. Ryan, D. Ryan and D. N. Dowling, Plant protection by the recombinant, root-colonizing Pseudomonas fluorescens F113rifPCB strain expressing arsenic resistance: improving rhizoremediation, Lett. Appl. Microbiol., 2007, 45(6), 668–674 CrossRef CAS PubMed.
- T. Barac, S. Taghavi, B. Borremans, A. Provoost, L. Oeyen, J. V. Colpaert, J. Vangronsveld and D. Van Der Lelie, Engineered endophytic bacteria improve phytoremediation of water-soluble, volatile, organic pollutants, Nat. Biotechnol., 2004, 22(5), 583–588 CrossRef CAS PubMed.
- M. L. De Souza, J. Seffernick, B. Martinez, M. J. Sadowsky and L. P. Wackett, The atrazine catabolism genes atzABC are widespread and highly conserved, J. Bacteriol., 1998, 180(7), 1951–1954 CrossRef CAS PubMed.
- D. Barriault, M. M. Plante and M. Sylvestre, Family shuffling of a targeted bphA region to engineer biphenyl dioxygenase, J. Bacteriol., 2002, 184(14), 3794–3800 CrossRef CAS PubMed.
- B. Mitter, N. Pfaffenbichler and A. Sessitsch, Plant–microbe partnerships in 2020, Microb. Biotechnol., 2016, 9(5), 635–640 CrossRef PubMed.
- F. Abbasian, T. Palanisami, M. Megharaj, R. Naidu, R. Lockington and K. Ramadass, Microbial diversity and hydrocarbon degrading gene capacity of a crude oil field soil as determined by metagenomics analysis, Biotechnol. Prog., 2016, 32(3), 638–648 CrossRef CAS PubMed.
- Y. Nie, C. Q. Chi, H. Fang, J. L. Liang, S. L. Lu, G. L. Lai, Y. Q. Tang and X. L. Wu, Diverse alkane hydroxylase genes in microorganisms and environments, Sci. Rep., 2014, 4(1), 4968 CrossRef CAS PubMed.
- T. Y. Izmalkova, O. I. Sazonova, M. O. Nagornih, S. L. Sokolov, I. A. Kosheleva and A. M. Boronin, The organization of naphthalene degradation genes in Pseudomonas putida strain AK5, Res. Microbiol., 2013, 164(3), 244–253 CrossRef CAS PubMed.
- P. Pandey, S. C. Kang, C. P. Gupta and D. K. Maheshwari, Rhizosphere competent Pseudomonas aeruginosa GRC 1 produces characteristic siderophore and enhances growth of Indian mustard (Brassica campestris), Curr. Microbiol., 2005, 51, 303–309 CrossRef CAS PubMed.
- B. Wang, Y. Teng, H. Yao and P. Christie, Detection of functional microorganisms in benzene [a] pyrene-contaminated soils using DNA-SIP technology, J. Hazard. Mater., 2021, 407, 124788 CrossRef CAS PubMed.
- S. Bisht, P. Pandey, A. Sood, S. Sharma and N. S. Bisht, Biodegradation of naphthalene and anthracene by chemo-tactically active rhizobacteria of Populus deltoides, Braz. J. Microbiol., 2010, 41, 922–930 CrossRef CAS PubMed.
- E. Pandolfo, A. Barra Caracciolo and L. Rolando, Recent advances in bacterial degradation of hydrocarbons, Water, 2023, 15(2), 375 CrossRef CAS.
- G. Kebede, T. Tafese, E. M. Abda, M. Kamaraj and F. Assefa, Factors influencing the bacterial bioremediation of hydrocarbon contaminants in the soil: mechanisms and impacts, J. Chem., 2021, 2021(1), 9823362 Search PubMed.
- D. Kour, T. Kaur, R. Devi, A. Yadav, M. Singh, D. Joshi, J. Singh, D. C. Suyal, A. Kumar, V. D. Rajput and A. N. Yadav, Beneficial microbiomes for bioremediation of diverse contaminated environments for environmental sustainability: present status and future challenges, Environ. Sci. Pollut. Res., 2021, 28, 24917–24939 CrossRef CAS PubMed.
- N. Das, R. Kotoky, A. P. Maurya, B. Bhuyan and P. Pandey, Paradigm shift in antibiotic-resistome of petroleum hydrocarbon contaminated soil, Sci. Total Environ., 2021, 757, 143777 CrossRef CAS PubMed.
- M. A. Malla, A. Dubey, A. Kumar, S. Yadav, A. Hashem and E. F. Abd_Allah, Exploring the human microbiome: the potential future role of next-generation sequencing in disease diagnosis and treatment, Front. Immunol., 2019, 9, 2868 CrossRef PubMed.
- C. Djemiel, S. Dequiedt, B. Karimi, A. Cottin, W. Horrigue, A. Bailly, A. Boutaleb, S. Sadet-Bourgeteau, P. A. Maron, N. Chemidlin Prévost-Bouré and L. Ranjard, Potential of meta-omics to provide modern microbial indicators for monitoring soil quality and securing food production, Front. Microbiol., 2022, 13, 889788 CrossRef PubMed.
- N. Haleyur, E. Shahsavari, S. S. Jain, E. Koshlaf, V. B. Ravindran, P. D. Morrison, A. M. Osborn and A. S. Ball, Influence of bioaugmentation and biostimulation on PAH degradation in aged contaminated soils: response and dynamics of the bacterial community, J. Environ. Manag., 2019, 238, 49–58 CrossRef CAS PubMed.
- D. R. Leadbeater, N. C. Bruce and T. Tonon, In silico identification of bacterial seaweed-degrading bioplastic producers, Microb. Genomics, 2022, 8(9), 000866 CAS.
- Z. Song, S. Chen, F. Zhao and W. Zhu, Whole metagenome of injected and produced fluids reveal the heterogenetic characteristics of the microbial community in a water-flooded oil reservoir, J. Petrol. Sci. Eng., 2019, 176, 1198–1207 CrossRef CAS.
- K. Moon, J. H. Jeon, I. Kang, K. S. Park, K. Lee, C. J. Cha, S. H. Lee and J. C. Cho, Freshwater viral metagenome reveals novel and functional phage-borne antibiotic resistance genes, Microbiome, 2020, 8, 1–5 CrossRef PubMed.
- X. Wang, C. Liang, J. Mao, Y. Jiang, Q. Bian, Y. Liang, Y. Chen and B. Sun, Microbial keystone taxa drive succession of plant residue chemistry, ISME J., 2023, 17(5), 748–757 CrossRef PubMed.
- F. Zhu, Y. Ju, W. Wang, Q. Wang, R. Guo, Q. Ma, Q. Sun, Y. Fan, Y. Xie, Z. Yang and Z. Jie, Metagenome-wide association of gut microbiome features for schizophrenia, Nat. Commun., 2020, 11(1), 1612 CrossRef CAS PubMed.
- S. Correa-Garcia, P. Constant and E. Yergeau, The forecasting power of the microbiome, Trends Microbiol., 2023, 31(5), 444–452 CrossRef CAS PubMed.
- L. K. Redfern, C. M. Gardner, E. Hodzic, P. L. Ferguson, H. Hsu-Kim and C. K. Gunsch, A new framework for approaching precision bioremediation of PAH contaminated soils, J. Hazard Mater., 2019, 378, 120859 CrossRef CAS PubMed.
- N. Premnath, K. Mohanrasu, R. G. Rao, G. H. Dinesh, G. S. Prakash, V. Ananthi, K. Ponnuchamy, G. Muthusamy and A. Arun, A crucial review on polycyclic aromatic Hydrocarbons-Environmental occurrence and strategies for microbial degradation, Chemosphere, 2021, 280, 130608 CrossRef CAS PubMed.
- V. N. Kristensen, O. C. Lingjærde, H. G. Russnes, H. K. Vollan, A. Frigessi and A. L. Børresen-Dale, Principles and methods of integrative genomic analyses in cancer, Nat. Rev. Cancer, 2014, 14(5), 299–313 CrossRef CAS PubMed.
- T. O. Elufisan, I. C. Rodríguez-Luna, O. O. Oyedara, A. Sánchez-Varela, A. Hernández-Mendoza, E. D. Gonzalez, A. D. Paz-González, K. Muhammad, G. Rivera, M. A. Villalobos-Lopez and X. Guo, The Polycyclic Aromatic Hydrocarbon (PAH) degradation activities and genome analysis of a novel strain Stenotrophomonas sp. Pemsol isolated from Mexico, PeerJ, 2020, 8, e8102 CrossRef PubMed.
- Q. Zhao, S. Yue, M. Bilal, H. Hu, W. Wang and X. Zhang, Comparative genomic analysis of 26 Sphingomonas and Sphingobium strains: dissemination of bioremediation capabilities, biodegradation potential and horizontal gene transfer, Sci. Total Environ., 2017, 609, 1238–1247 CrossRef CAS PubMed.
- Z. Zhou, Y. Jiang, Z. Wang, Z. Gou, J. Lyu, W. Li, Y. Yu, L. Shu, Y. Zhao, Y. Ma and C. Fang, Resequencing 302 wild and cultivated accessions identifies genes related to domestication and improvement in soybean, Nat. Biotechnol., 2015, 33(4), 408–414 CrossRef CAS PubMed.
- A. E. Hesham, A. M. Mawad, Y. M. Mostafa and A. Shoreit, Biodegradation ability and catabolic genes of petroleum-degrading Sphingomonas koreensis strain ASU-06 isolated from Egyptian oily soil, BioMed Res. Int., 2014, 2014(1), 127674 Search PubMed.
- H. Cao, H. Wu, R. Luo, S. Huang, Y. Sun, X. Tong, Y. Xie, B. Liu, H. Yang, H. Zheng and J. Li, De novo assembly of a haplotype-resolved human genome, Nat. Biotechnol., 2015, 33(6), 617–622 CrossRef CAS PubMed.
- R. Kotoky, J. Rajkumari and P. Pandey, The rhizosphere microbiome: Significance in rhizoremediation of polyaromatic hydrocarbon contaminated soil, J. Environ. Manag., 2018, 217, 858–870 CrossRef CAS PubMed.
- S. Bazsefidpar, B. Mokhtarani, R. Panahi and H. Hajfarajollah, Overproduction of rhamnolipid by fed-batch cultivation of Pseudomonas aeruginosa in a lab-scale fermenter under tight DO control, Biodegradation, 2019, 30, 59–69 CrossRef CAS PubMed.
- A. A. Ivanova, O. I. Sazonova, A. N. Zvonarev, Y. A. Delegan, R. A. Streletskii, L. A. Shishkina, A. G. Bogun and A. A. Vetrova, Genome analysis and physiology of Pseudomonas sp. strain OVF7 degrading naphthalene and n-dodecane, Microorganisms, 2023, 11(8), 2058 CrossRef CAS PubMed.
- K. Yu and T. Zhang, Metagenomic and metatranscriptomic analysis of microbial community structure and gene expression of activated sludge, PloS one, 2012, 7(5), e38183 CrossRef CAS PubMed.
- S. Mukhtar, N. Rashid, M. F. Haque and K. A. Malik, Metagenomic approach for the isolation of novel extremophiles, in Microbial Extremozymes, Academic Press, 2022, pp. 55–66 Search PubMed.
- R. Xu, M. Kolton, W. Tao, X. Sun, P. Su, D. Huang, M. Zhang, Z. Yang, Z. Guo, H. Gao and Q. Wang, Anaerobic selenite-reducing bacteria and their metabolic potentials in Se-rich sediment revealed by the combination of DNA-stable isotope probing, metagenomic binning, and metatranscriptomics, J. Hazard. Mater., 2023, 457, 131834 CrossRef CAS PubMed.
- J. Peng, C. E. Wegner, Q. Bei, P. Liu and W. Liesack, Metatranscriptomics reveals a differential temperature effect on the structural and functional organization of the anaerobic food web in rice field soil, Microbiome, 2018, 6, 1–6 CrossRef PubMed.
- A. de Menezes, N. Clipson and E. Doyle, Comparative metatranscriptomics reveals widespread community responses during phenanthrene degradation in soil, Environ. Microbiol., 2012, 14(9), 2577–2588 CrossRef CAS PubMed.
- M. Tartaglia, F. Bastida, R. Sciarrillo and C. Guarino, Soil metaproteomics for the study of the relationships between microorganisms and plants: a review of extraction protocols and ecological insights, Int. J. Mol. Sci., 2020, 21(22), 8455 CrossRef CAS PubMed.
- M. E. Guazzaroni, F. A. Herbst, I. Lores, J. Tamames, A. I. Peláez, N. Lopez-Cortés, M. Alcaide, M. V. Del Pozo, J. M. Vieites, M. Von Bergen and J. L. Gallego, Metaproteogenomic insights beyond bacterial response to naphthalene exposure and bio-stimulation, ISME J., 2013, 7(1), 122–136 CrossRef CAS PubMed.
- F. Bastida, N. Jehmlich, I. F. Torres and C. García, The extracellular metaproteome of soils under semiarid climate: A methodological comparison of extraction buffers, Sci. Total Environ., 2018, 619, 707–711 CrossRef PubMed.
- C. Liu, H. Huang, X. Duan and Y. Chen, Integrated metagenomic and metaproteomic analyses unravel ammonia toxicity to active methanogens and syntrophs, enzyme synthesis, and key enzymes in anaerobic digestion, Environ. Sci. Technol., 2021, 55(21), 14817–14827 CrossRef CAS PubMed.
- S. Wang, F. J. van Schooten, H. Jin, D. Jonkers and R. Godschalk, The involvement of intestinal tryptophan metabolism in inflammatory bowel disease identified by a meta-analysis of the transcriptome and a systematic review of the metabolome, Nutrients, 2023, 15(13), 2886 CrossRef CAS PubMed.
- R. Rabus, Fifteen years of physiological proteo (geno) mics with (marine) environmental bacteria, Arch. Physiol. Biochem., 2014, 120(5), 173–187 CrossRef CAS PubMed.
- D. Martins, A. Giacomel, S. C. Williams, F. Turkheimer, O. Dipasquale and M. Veronese, Imaging transcriptomics: Convergent cellular, transcriptomic, and molecular neuroimaging signatures in the healthy adult human brain, Cell Rep., 2021, 37(13), 110173 CrossRef CAS PubMed.
- D. S. Wishart, Metabolomics for investigating physiological and pathophysiological processes, Physiol. Rev., 2019, 99(4), 1819–1875 CrossRef CAS PubMed.
- R. W. Brown, D. R. Chadwick, H. Zang and D. L. Jones, Use of metabolomics to quantify changes in soil microbial function in response to fertiliser nitrogen supply and extreme drought, Soil Biol. Biochem., 2021, 160, 108351 CrossRef CAS.
- X. Bao, W. Xu, J. Cui, Z. Yan, J. Wang, X. Chen and Z. Meng, NMR-based metabolomics approach to assess the ecotoxicity of prothioconazole on the earthworm (Eisenia fetida) in soil, Pestic. Biochem. Physiol., 2023, 190, 105320 CrossRef CAS PubMed.
- Y. Li, C. Wang and M. Chen, Metabolomics-based study of potential biomarkers of sepsis, Sci. Rep., 2023, 13(1), 585 CrossRef CAS PubMed.
- H. Wang, J. Hua, Q. Yu, J. Li, J. Wang, Y. Deng, H. Yuan and Y. Jiang, Widely targeted metabolomic analysis reveals dynamic changes in non-volatile and volatile metabolites during green tea processing, Food Chem., 2021, 363, 130131 CrossRef CAS PubMed.
- J. D. Molina, S. Avila, G. Rubio and F. López-Muñoz, Metabolomic connections between schizophrenia, antipsychotic drugs and metabolic syndrome: a variety of players, Curr. Pharm. Des., 2021, 27(39), 4049–4061 CrossRef CAS PubMed.
- G. Kebede, T. Tafese, E. M. Abda, M. Kamaraj and F. Assefa, Factors influencing the bacterial bioremediation of hydrocarbon contaminants in the soil: mechanisms and impacts, J. Chem., 2021, 2021(1), 9823362 Search PubMed.
- A. Segura, S. Rodríguez-Conde, C. Ramos and J. L. Ramos, Bacterial responses and interactions with plants during rhizoremediation, Microb. Biotechnol., 2009, 2(4), 452–464 CrossRef CAS PubMed.
- X. Zhao, R. Miao, M. Guo, X. Shang, Y. Zhou and J. Zhu, Biochar enhanced polycyclic aromatic hydrocarbons degradation in soil planted with ryegrass: bacterial community and degradation gene expression mechanisms, Sci. Total Environ., 2022, 838, 156076 CrossRef CAS PubMed.
- N. Rodríguez-Berbel, R. Soria, A. B. Villafuerte, R. Ortega and I. Miralles, Short-term dynamics of bacterial community structure in restored abandoned agricultural soils under semi-arid conditions, Agronomy, 2022, 13(1), 86 CrossRef.
- G. Wu, C. Kechavarzi, X. Li, H. Sui, S. J. Pollard and F. Coulon, Influence of mature compost amendment on total and bioavailable polycyclic aromatic hydrocarbons in contaminated soils, Chemosphere, 2013, 90(8), 2240–2246 CrossRef CAS PubMed.
- N. B. Islam, D. L. Whalen, H. Yagi and D. M. Jerina, pH dependence of the mechanism of hydrolysis of benzo [a] pyrene-cis-7, 8-diol 9, 10-epoxide catalyzed by DNA, poly (G), and poly (A), J. Am. Chem. Soc., 1987, 109(7), 2108–2111 CrossRef CAS.
- Z. Vipotnik, M. Michelin and T. Tavares, Ligninolytic enzymes production during polycyclic aromatic hydrocarbons degradation: effect of soil pH, soil amendments and fungal co-cultivation, Biodegradation, 2021, 32, 193–215 CrossRef CAS PubMed.
- Y. Qi, Y. Wu, Q. Zhi, Z. Zhang, Y. Zhao and G. Fu, Effects of polycyclic aromatic hydrocarbons on the composition of the soil bacterial communities in the tidal flat wetlands of the Yellow River Delta of China, Microorganisms, 2024, 12(1), 141 CrossRef CAS PubMed.
- Y. Wang, M. Li, Z. Liu, J. Zhao and Y. Chen, Interactions between pyrene and heavy metals and their fates in a soil-maize (Zea mays L.) system: Perspectives from the root physiological functions and rhizosphere microbial community, Environ. Pollut., 2021, 287, 117616 CrossRef CAS PubMed.
- T. W. Chen, L. Cabrera-Bosquet, S. Alvarez Prado, R. Perez, S. Artzet, C. Pradal, A. Coupel-Ledru, C. Fournier and F. Tardieu, Genetic and environmental dissection of biomass accumulation in multi-genotype maize canopies, J. Exp. Bot., 2019, 70(9), 2523–2534 CrossRef CAS PubMed.
- Z. Q. Du, Y. W. Xing and T. S. Han, Effects of environment and genotype-by-environment interaction on phenotype of Rorippa elata (Brassicaceae), an endemic alpine plant in the Hengduan mountains, J. Plant Ecol., 2024, 17(4), rtae048 CrossRef.
- S. Cadot, H. Guan, M. Bigalke, J. C. Walser, G. Jander, M. Erb, M. G. van der Heijden and K. Schlaeppi, Specific and conserved patterns of microbiota-structuring by maize benzoxazinoids in the field, Microbiome, 2021, 9(1), 103 CrossRef CAS PubMed.
- K. M. Robertson, E. Simonson, N. Ramirez-Bullon, B. Poulter and R. Carter, Effects of spatial resolution, mapping window size, and spectral species clustering on remote sensing of plant beta diversity using biodivMapR and hyperspectral imagery, J. Geophys. Res.:Biogeosci., 2023, 128(7), e2022JG007350 CrossRef.
- O. Pritchina, C. Ely and B. F. Smets, Effects of PAH-contaminated soil on rhizosphere microbial communities, Water, Air, Soil Pollut., 2011, 222, 17–25 CrossRef CAS.
- K. Huang, M. Guan, J. Chen, J. Xu, H. Xia and Y. Li, Biochars modify the degradation pathways of dewatered sludge by regulating active microorganisms during gut digestion of earthworms, Sci. Total Environ., 2022, 828, 154496 CrossRef CAS PubMed.
- Y. H. Su and X. Y. Yang, Interactions between selected PAHs and the microbial community in rhizosphere of a paddy soil, Sci. Total Environ., 2009, 407(3), 1027–1034 CrossRef CAS PubMed.
- C. Lu, Y. Hong, E. S. Odinga, J. Liu, D. C. Tsang and Y. Gao, Bacterial community and PAH-degrading genes in paddy soil and rice grain from PAH-contaminated area, Appl. Soil Ecol., 2021, 158, 103789 CrossRef.
- L. J. Ding, H. L. Cui, S. A. Nie, X. E. Long, G. L. Duan and Y. G. Zhu, Microbiomes inhabiting rice roots and rhizosphere, FEMS Microbiol. Ecol., 2019, 95(5), fiz040 CAS.
- M. Eriksson, E. Sodersten, Z. Yu, G. Dalhammar and W. W. Mohn, Degradation of polycyclic aromatic hydrocarbons at low temperature under aerobic and nitrate-reducing conditions in enrichment cultures from northern soils, Appl. Environ. Microbiol., 2003, 69(1), 275–284 CrossRef CAS PubMed.
- N. Y. Amponsah, J. Wang and L. Zhao, Modelling PAH Degradation in Contaminated Soils in Canada using a Modified Process-Based Model (DNDC), Soil Sci. Soc. Am. J., 2019, 83(3), 605–613 CrossRef CAS.
- C. T. Chiou, S. E. McGroddy and D. E. Kile, Partition characteristics of polycyclic aromatic hydrocarbons on soils and sediments, Environ. Sci. Technol., 1998, 32(2), 264–269 CrossRef CAS.
- C. Lors, D. Damidot, J. F. Ponge and F. Périé, Comparison of a bioremediation process of PAHs in a PAH-contaminated soil at field and laboratory scales, Environ. Pollut., 2012, 165, 11–17 CrossRef CAS PubMed.
- B. Maliszewska-Kordybach, Dissipation of polycyclic aromatic hydrocarbons in freshly contaminated soils–the effect of soil physicochemical properties and aging, Water, Air, Soil Pollut., 2005, 168, 113–128 CrossRef CAS.
- W. L. Straube, C. C. Nestler, L. D. Hansen, D. Ringleberg, P. H. Pritchard and J. Jones-Meehan, Remediation of polyaromatic hydrocarbons (PAHs) through landfarming with biostimulation and bioaugmentation, Acta Biotechnol., 2003, 23(2-3), 179–196 CrossRef CAS.
- D. Dong, P. Li, X. Li, Q. Zhao, Y. Zhang, C. Jia and P. Li, Investigation on the photocatalytic degradation of pyrene on soil surfaces using nanometer anatase TiO2 under UV irradiation, J. Hazard. Mater., 2010, 174(1–3), 859–863 CrossRef CAS PubMed.
- K. Kidd, A. Mercer and R. A. Curry, Methods and Preliminary Results for Metals, Polycyclic Aromatic Hydrocarbons, Polychlorinated Biphenyls and Chlorinated Pesticides in Surface Sediments of the Mactaquac Headpond, Mactaquac Aquatic Ecosystem Study Report Series 2015-018, 2016 Search PubMed.
- L. A. Msimbira and D. L. Smith, The roles of plant growth promoting microbes in enhancing plant tolerance to acidity and alkalinity stresses, Front. Sustain. Food Syst., 2020, 4, 106 CrossRef.
- S. Haldar and A. Ghosh, Microbial and plant-assisted heavy metal remediation in aquatic ecosystems: a comprehensive review, 3 Biotech., 2020, 10(5), 205 CrossRef PubMed.
- O. V. Mavrodi, J. R. McWilliams, J. O. Peter, A. Berim, K. A. Hassan, L. D. Elbourne, M. K. LeTourneau, D. R. Gang, I. T. Paulsen, D. M. Weller and L. S. Thomashow, Root exudates alter the expression of diverse metabolic, transport, regulatory, and stress response genes in rhizosphere Pseudomonas, Front. Microbiol., 2021, 12, 651282 CrossRef PubMed.
- F. Alotaibi, M. Hijri and M. St-Arnaud, Overview of approaches to improve rhizoremediation of petroleum hydrocarbon-contaminated soils, Appl. Microbiol., 2021, 1(2), 329–351 CrossRef.
- R. Zainab, M. Hasnain, F. Ali, D. A. Dias, A. El-Keblawy and Z. Abideen, Exploring the bioremediation capability of petroleum-contaminated soils for enhanced environmental sustainability and minimization of ecotoxicological concerns, Environ. Sci. Pollut. Res., 2023, 30(48), 104933–104957 CrossRef CAS PubMed.
- K. Derz, B. Schmidt, S. Schwiening and I. Schuphan, Comparison of microbial pyrene and benzo [a] pyrene mineralization in liquid medium, soil slurry, and soil, J. Environ. Sci. Health, Part B, 2006, 41(5), 471–484 CrossRef PubMed.
- R. Sriprang, M. Hayashi, H. Ono, M. Takagi, K. Hirata and Y. Murooka, Enhanced accumulation of Cd2+ by a Mesorhizobium sp. transformed with a gene from Arabidopsis thaliana coding for phytochelatin synthase, Appl. Environ. Microbiol., 2003, 69(3), 1791–1796 CrossRef PubMed.
- M. Lemare, H. Puja, S. R. David, S. Mathieu, D. Ihiawakrim, V. A. Geoffroy and C. Rigouin, Engineering siderophore production in Pseudomonas to improve asbestos weathering, Microb. Biotechnol., 2022, 15(9), 2351–2363 CrossRef CAS PubMed.
- S. Yavari, A. Malakahmad and N. B. Sapari, A review on phytoremediation of crude oil spills, Water, Air, Soil Pollut., 2015, 226, 1–8 CrossRef CAS.
- S. Che and Y. Men, Synthetic microbial consortia for biosynthesis and biodegradation: promises and challenges, J. Ind. Microbiol. Biotechnol., 2019, 46(9–10), 1343–1358 CrossRef CAS PubMed.
- R. Czajkowski, T. Maciag, D. M. Krzyzanowska and S. Jafra, Biological control based on microbial consortia–from theory to commercial products, How Research Can Stimulate the Development of Commercial Biological Control against Plant Diseases, 2020, pp. 183–202 Search PubMed.
- D. Ghosal, S. Ghosh, T. K. Dutta and Y. Ahn, Current state of knowledge in microbial degradation of polycyclic aromatic hydrocarbons (PAHs): a review, Front. Microbiol., 2016, 7, 1369 Search PubMed.
- T. Sayara, E. Borràs, G. Caminal, M. Sarrà and A. Sánchez, Bioremediation of PAHs-contaminated soil through composting: Influence of bioaugmentation and biostimulation on contaminant biodegradation, Int. Biodeterior. Biodegrad., 2011, 65(6), 859–865 CrossRef CAS.
- S. Geng, W. Qin, W. Cao, Y. Wang, A. Ding, Y. Zhu, F. Fan and J. Dou, Pilot-scale bioaugmentation of polycyclic aromatic hydrocarbon (PAH)-contaminated soil using an indigenous bacterial consortium in soil-slurry bioreactors, Chemosphere, 2022, 287, 132183 CrossRef CAS PubMed.
- S. Wang, Z. Wang, M. Usman, Z. Zheng, X. Zhao, X. Meng, K. Hu, X. Shen, X. Wang and Y. Cai, Two microbial consortia obtained through purposive acclimatization as biological additives to relieve ammonia inhibition in anaerobic digestion, Water Res., 2023, 230, 119583 CrossRef CAS PubMed.
- P. Sarkar and A. Dey, 4-Nitrophenol biodegradation by an isolated and characterized microbial consortium and statistical optimization of physicochemical parameters by Taguchi Methodology, J. Environ. Chem. Eng., 2020, 8(5), 104347 CrossRef CAS.
- D. K. Chaudhary, R. Bajagain, S. W. Jeong and J. Kim, Development of a bacterial consortium comprising oil-degraders and diazotrophic bacteria for elimination of exogenous nitrogen requirement in bioremediation of diesel-contaminated soil, World J. Microbiol. Biotechnol., 2019, 35, 1 CrossRef CAS PubMed.
- S. Srivastava and S. Sharma, Metabolomic insight into the synergistic mechanism of action of a bacterial consortium in plant growth promotion, J. Biosci. Bioeng., 2022, 134(5), 399–406 CrossRef PubMed.
- M. H. Ali, M. I. Khan, M. Naveed and M. A. Tanvir, Microbe-assisted rhizodegradation of hydrocarbons and growth enhancement of wheat plants in hydrocarbons contaminated soil, Int. J. Environ. Sci. Technol., 2024, 21(3), 3169–3184 CrossRef CAS.
- S. Curiel-Alegre, D. de la Fuente-Vivas, A. H. Khan, J. García-Tojal, B. Velasco-Arroyo, C. Rumbo, G. Soja, C. Rad and R. Barros, Unveiling the capacity of bioaugmentation application, in comparison with biochar and rhamnolipid for TPHs degradation in aged hydrocarbons polluted soil, Environ. Res., 2024, 252, 118880 CrossRef CAS PubMed.
- T. B. Moorman, J. K. Cowan, E. L. Arthur and J. R. Coats, Organic amendments to enhance herbicide biodegradation in contaminated soils, Biol. Fertil. Soils, 2001, 33, 541–545 CrossRef CAS.
- W. L. Straube, C. C. Nestler, L. D. Hansen, D. Ringleberg, P. H. Pritchard and J. Jones-Meehan, Remediation of polyaromatic hydrocarbons (PAHs) through landfarming with biostimulation and bioaugmentation, Acta Biotechnol., 2003, 23(2-3), 179–196 CrossRef CAS.
- D. Singh, K. Malik, M. Sindhu, N. Kumari, V. Rani, S. Mehta, K. Malik, P. Ranga, K. Sharma, N. Dhull and S. Malik, Biostimulation of anaerobic digestion using iron oxide nanoparticles (IONPs) for increasing biogas production from cattle manure, Nanomaterials, 2022, 12(3), 497 CrossRef CAS PubMed.
- O. A. Udume, G. O. Abu, H. O. Stanley, I. F. Vincent-Akpu, Y. Momoh and M. O. Eze, Biostimulation of petroleum-contaminated soil using organic and inorganic amendments, Plants, 2023, 12(3), 431 CrossRef CAS PubMed.
- M. Arshad, M. Saleem and S. Hussain, Perspectives of bacterial ACC deaminase in phytoremediation, Trends Biotechnol., 2007, 25(8), 356–362 CrossRef CAS PubMed.
- I. Kuiper, E. L. Lagendijk, R. Pickford, J. P. Derrick, G. E. Lamers, J. E. Thomas-Oates, B. J. Lugtenberg and G. V. Bloemberg, Characterization of two Pseudomonas putida lipopeptide biosurfactants, putisolvin I and II, which inhibit biofilm formation and break down existing biofilms, Mol. Microbiol., 2004, 51(1), 97–113 CrossRef CAS PubMed.
- A. Praveen and V. C. Pandey, Pteridophytes in phytoremediation, Environ. Geochem. Health, 2020, 42(8), 2399–2411 CrossRef CAS PubMed.
- G. R. Dixon and U. F. Walsh, Suppressing Pythium ultimum induced damping-off in cabbage seedlings by biostimulation with proprietary liquid seaweed extracts, in XXVI International Horticultural Congress: Managing Soil-Borne Pathogens: A Sound Rhizosphere to Improve Productivity in 635, 2002, pp. 103–106 Search PubMed.
- K. Uchida, K. Sakuta, A. Ito, Y. Takahashi, Y. Katayama, T. Omatsu, T. Mizutani, T. Arie, K. Komatsu, T. Fukuhara and S. Uematsu, Two novel endornaviruses co-infecting a Phytophthora pathogen of Asparagus officinalis modulate the developmental stages and fungicide sensitivities of the host oomycete, Front. Microbiol., 2021, 12, 633502 CrossRef PubMed.
- H. Kawahigashi, Transgenic plants for phytoremediation of herbicides, Curr. Opin. Biotechnol., 2009, 20(2), 225–230 CrossRef CAS PubMed.
- https://www.biospace.com/article/bioremediation-market-size-to-reach-usd-334-70-billion-in-2027-industry-trend-rapid-advancements-in-remediation-technology-/.
- G. O. Adams, P. T. Fufeyin, S. E. Okoro and I. Ehinomen, Bioremediation, biostimulation and bioaugmention: a review, Int. J. Environ. Biorem. Biodegrad., 2015, 3(1), 28–39 CAS.
- A. Kumar, R. Gudiukaite, A. Gricajeva, M. Sadauskas, V. Malunavicius, H. Kamyab, S. Sharma, T. Sharma and D. Pant, Microbial lipolytic enzymes–promising energy-efficient biocatalysts in bioremediation, Energy, 2020, 192, 116674 CrossRef CAS.
- V. K. Gaur, K. Gautam, P. Sharma, P. Gupta, S. Dwivedi, J. K. Srivastava, S. Varjani, H. H. Ngo, S. H. Kim, J. S. Chang and X. T. Bui, Sustainable strategies for combating hydrocarbon pollution: Special emphasis on mobil oil bioremediation, Sci. Total Environ., 2022, 832, 155083 CrossRef CAS PubMed.
- https://www.grandviewresearch.com/industry-analysis/bioremediation-market-report.
- V. Poria, K. Dębiec-Andrzejewska, A. Fiodor, M. Lyzohub, N. Ajijah, S. Singh and K. Pranaw, Plant Growth-Promoting Bacteria (PGPB) integrated phytotechnology: A sustainable approach for remediation of marginal lands, Front. Plant Sci., 2022, 13, 999866 CrossRef PubMed.
- S. Bala, D. Garg, B. V. Thirumalesh, M. Sharma, K. Sridhar, B. S. Inbaraj and M. Tripathi, Recent strategies for bioremediation of emerging pollutants: a review for a green and sustainable environment, Toxics, 2022, 10(8), 484 CrossRef CAS PubMed.
- National Intelligence Council (US), Global Trends 2030: Alternative Worlds: a Publication of the National Intelligence Council, US Government Printing Office, 2012 Search PubMed.
- https://www.marketsandmarkets.com/Market-Reports/environmental-remediation-market-93290334.html#:%7E:text=TheEnvironmentalRemediationmarketis,8.4%25from2022to2027.
- https://eu.boell.org/en/europe-sustainable-development-report-2021#:%7E:text=TheEuropeSustainableDevelopmentReport,SustainableDevelopmentGoals(SDGs).
- S. Maitra, In situ bioremediation—An overview, Res. J. Life Sci., Bioinf., Pharm. Chem. Sci., 2018, 4, 576–598 CAS.
- https://www.adb.org/sites/default/files/publication/209511/sanitation-sustainable-dev-japan.pdf.
- H. Talvenmäki, N. Saartama, A. Haukka, K. Lepikkö, V. Pajunen and M. Punkari, et al., In situ bioremediation of Fenton's reaction–treated oil spill site, with a soil inoculum, slow release additives, and methyl-β-cyclodextrin, Environ. Sci. Pollut. Res., 2021, 28, 20273–20289, DOI:10.1007/s11356-020-11910-w.
- D. Yang and M. Sonmez, Integration and divergence of patent systems across national and international institutions, J. World Bus., 2013, 48(4), 527–538 CrossRef.
- S. Saraswat, Patent analysis on bioremediation of environmental pollutants, J. Biorem. Biodegrad., 2014, 5(251), 2 Search PubMed.
- P. Sahoo, D. K. Rai and R. Kumar, India-korea Trade and Investment Relations [Working Paper No. 242], Indian Council for Research on International Economic Relations, New Delhi, 2009 Search PubMed.
- Y. Sun, Y. Lu, T. Wang, H. Ma and G. He, Pattern of patent-based environmental technology innovation in China, Technol. Forecast. Soc. Change, 2008, 75(7), 1032–1042 CrossRef.
- https://www.oecd.org/mena/47563588.pdf.
- K. Sayed, L. Baloo and N. K. Sharma, Bioremediation of total petroleum hydrocarbons (TPH) by bioaugmentation and biostimulation in water with floating oil spill containment booms as bioreactor basin, Int. Res. J. Publ. Environ. Health, 2021, 18(5), 2226 CrossRef CAS PubMed.
- H. D. Villela, R. S. Peixoto, A. U. Soriano and F. L. Carmo, Microbial bioremediation of oil contaminated seawater: A survey of patent deposits and the characterization of the top genera applied, Sci. Total Environ., 2019, 666, 743–758 CrossRef CAS PubMed.
- S. Chandra and N. Singh, Enhanced bioremediation techniques for agricultural soils, Int. J. Curr. Res. Acad. Rev., 2015, 3(7), 166–173 Search PubMed.
- C. M. Quintella, A. M. Mata and L. C. Lima, Overview of bioremediation with technology assessment and emphasis on fungal bioremediation of oil contaminated soils, J. Environ. Manag., 2019, 241, 156–166 CrossRef CAS PubMed.
- https://inkwoodresearch.com/reports/bioremediation-technology-and-services-market/.
- L. C. Davis, S. Castro-Diaz, Q. Zhang and L. E. Erickson, Benefits of vegetation for soils with organic contaminants, Crit. Rev. Plant Sci., 2002, 21(5), 457–491 CrossRef CAS.
- N. Fiorentino, M. Mori, V. Cenvinzo, L. G. Duri, L. Gioia, D. Visconti and M. Fagnano, Assisted phytoremediation for restoring soil fertility in contaminated and degraded land, Crit. Rev. Plant Sci., 2018, 13, 34–44 Search PubMed.
- D. Ghosal, S. Ghosh, T. K. Dutta and Y. Ahn, Current state of knowledge in microbial degradation of polycyclic aromatic hydrocarbons (PAHs): a review, Front. Microbiol., 2016, 7, 1369 Search PubMed.
- N. Weyens, D. Van Der Lelie, T. Artois, K. Smeets, S. Taghavi, L. Newman, R. Carleer and J. Vangronsveld, Bioaugmentation with engineered endophytic bacteria improves contaminant fate in phytoremediation, Environ. Sci. Technol., 2009, 43(24), 9413–9418 CrossRef CAS PubMed.
- S. Rebello, V. K. Nathan, R. Sindhu, P. Binod, M. K. Awasthi and A. Pandey, Bioengineered microbes for soil health restoration: present status and future, Bioengineered, 2021, 12(2), 12839–12853 CrossRef PubMed.
- S. Rebello, V. K. Nathan, R. Sindhu, P. Binod, M. K. Awasthi and A. Pandey, Bioengineered microbes for soil health restoration: present status and future, Bioengineered, 2021, 12(2), 12839–12853 CrossRef PubMed.
- C. Liu, Q. Liu, S. Song, W. Li, Y. Feng, X. Cong, Y. Ji and P. Li, The association between internal polycyclic aromatic hydrocarbons exposure and risk of obesity—a systematic review with meta-analysis, Chemosphere, 2023, 329, 138669 CrossRef CAS PubMed.
- H. A. Yeheyo, A. M. Ealias, G. George and U. Jagannathan, Bioremediation potential of microalgae for sustainable soil treatment in India: a comprehensive review on heavy metal and pesticide contaminant removal, J. Environ. Manage., 2024, 363, 121409 CrossRef CAS PubMed.
- T. T. Ma, X. F. Shen, C. Yang, H. L. Qian, Y. H. Pang and X. P. Yan, Covalent immobilization of covalent organic framework on stainless steel wire for solid-phase microextraction GC-MS/MS determination of sixteen polycyclic aromatic hydrocarbons in grilled meat samples, Talanta, 2019, 201, 413–418 CrossRef CAS PubMed.
- L. Wang, D. Hou, Z. Shen, J. Zhu, X. Jia, Y. S. Ok, F. M. Tack and J. Rinklebe, Field trials of phytomining and phytoremediation: A critical review of influencing factors and effects of additives, Crit. Rev. Environ. Sci. Technol., 2020, 50(24), 2724–2774 CrossRef.
- T. Heimann, Bioeconomy and SDGs: Does the bioeconomy support the achievement of the SDGs?, Earth's Future, 2019, 7(1), 43–57 CrossRef.
- T. Setiadi, A. Harimawan, G. A. Sumampouw and A. Indarto, The mixture of agricultural pesticides and their impact on populations: bioremediation strategies, in Emerging Contaminants in the Environment, Elsevier, 2022, pp. 511–546 Search PubMed.
- M. D. Yuniati, Bioremediation of petroleum-contaminated soil: A Review, in IOP Conference Series: Earth and Environmental Science, IOP Publishing, 2018, vol. 118, p. 012063 Search PubMed.
- M. Mainardis, F. Magnolo, C. Ferrara, C. Vance, G. Misson, G. De Feo, S. Speelman, F. Murphy and D. Goi, Alternative seagrass wrack management practices in the circular bioeconomy framework: A life cycle assessment approach, Sci. Total Environ., 2021, 798, 149283 CrossRef CAS PubMed.
- K. Alshehri, Z. Gao, M. Harbottle, D. Sapsford and P. Cleall, Life cycle assessment and cost-benefit analysis of nature-based solutions for contaminated land remediation: A mini-review, Heliyon, 2023, 9(10), e20632 CrossRef PubMed.
|
This journal is © The Royal Society of Chemistry 2025 |
Click here to see how this site uses Cookies. View our privacy policy here.