DOI:
10.1039/C5CS00285K
(Review Article)
Chem. Soc. Rev., 2015,
44, 5897-5914
A sustainable future for photonic colloidal nanocrystals
Received
5th April 2015
First published on 18th June 2015
Abstract
Colloidal nanocrystals – produced in a growing variety of shapes, sizes and compositions – are rapidly developing into a new generation of photonic materials, spanning light emitting as well as energy harvesting applications. Precise tailoring of their optoelectronic properties enables them to satisfy disparate application-specific requirements. However, the presence of toxic heavy metals such as cadmium and lead in some of the most mature nanocrystals is a serious drawback which may ultimately preclude their use in consumer applications. Although the pursuit of non-toxic alternatives has occurred in parallel to the well-developed Cd- and Pb-based nanocrystals, synthetic challenges have, until recently, curbed progress. In this review, we highlight recent advances in the development of heavy-metal-free nanocrystals within the context of specific photonic applications. We also describe strategies to transfer some of the advantageous nanocrystal features such as shape control to non-toxic materials. Finally, we present recent developments that have the potential to make substantial impacts on the quest to attain a balance between performance and sustainability in photonics.
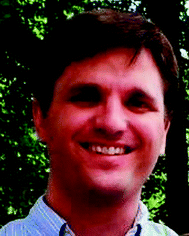
Joel Q. Grim
| Joel Grim joined the NanoCrystal Photonics Lab within the Nanochemistry Department at the Istituto Italiano di Tecnologia in September 2013. His postdoctoral research centers on investigating the optoelectronic properties of zero-, one-, and two-dimensional shape-controlled colloidal nanocrystals using ultrafast and nonlinear optical techniques. In addition to these fundamental studies, he is pursuing photonic applications such as low-threshold lasers. He obtained his PhD in Physics at Wake Forest University (USA) in 2012 where he studied nonlinear recombination processes in materials used for high-energy detection. He has BSc degrees in Mathematics, and Ag. and Biological Engineering from the Pennsylvania State University (USA). |
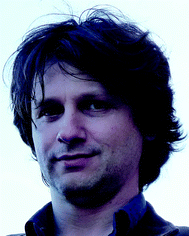
Liberato Manna
| Liberato Manna received his PhD in Chemistry in 2001 from the University of Bari (Italy) and worked at the University of California Berkeley as a visiting student and then at the Lawrence Berkeley Lab as a postdoc until 2003. He was then a scientist at the National Nanotechnology Lab in Lecce (Italy) and in 2009 he moved to the Istituto Italiano di Tecnologia (Genova) as the director of the Nanochemistry Department. Since 2010 he has been also working as a part-time professor at TU Delft (The Netherlands). His research interests are the synthesis and assembly of inorganic nanostructures for applications in various fields and the study of structural and chemical transformations in nanocrystals. |
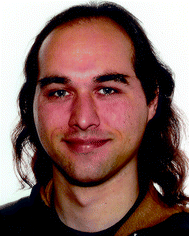
Iwan Moreels
| Iwan Moreels obtained his PhD degree in applied physics at Ghent University (Belgium) in 2009, studying near-infrared lead salt quantum dots. He then continued with a postdoc project on quantum dot emission dynamics at Ghent University and the IBM Zurich research lab (Switzerland). In 2012 he joined the Istituto Italiano di Tecnologia (Italy), where he now leads the NanoCrystal Photonics Lab in the Nanochemistry Department. He has published about 50 papers in peer-reviewed journals, with research that spans from the synthesis of novel fluorescent nanocrystals to ultrafast optical spectroscopy and photonic applications based on colloidal quantum dots. |
1. Introduction
As the global population continues to grow, and with it energy consumption and pressure on natural resources, sustainable development of technology has become a critical issue. Photonic and optoelectronic applications are particularly relevant in this regard, with lighting and personal electronics contributing to more than 20% of electricity consumed worldwide.1 The development of economically viable solutions requires scalable, inexpensive earth-abundant materials, with minimal energy required for the development and integration into applications. Material toxicity also plays a crucial role, both with regard to minimizing possible contact in consumer applications as well as reducing hazardous waste.
Colloidal quantum dots (QDs) are solution-processed semiconductor nanocrystals with diameters less than 100 nm. They are particularly suited to initiate a future generation of photonic devices that adequately respond to the requirements set above. The discovery of the quantum confinement effect2 now just over 30 years ago,3–6 and the first organic ‘hot-injection’ QD synthesis developed in 1993 (ref. 7) have led to a significant advance in the production of high-quality size-tunable, monodisperse nanocrystals with high photoluminescence quantum efficiency (PLQE). This new material class has received substantial attention ever since, with an increasing range of applications. Besides quantum dots, other colloidal geometries have also been developed including nanorods (NRs),8 nanoplatelets (NPLs),9–11 and heterostructures such as core–shell dot-in-dots,12,13 dot-in-rods,14 and more recently rod-in-rods,15 and dot-in-plates,16 all of which are referred to broadly throughout this review as colloidal nanocrystals (NCs). The diversity in NCs is achieved by numerous synthesis possibilities, including preparation in organic7 or aqueous media,17,18 polymer-assisted syntheses19,20 and cation exchange reactions21–23 in case direct growth procedures do not lead to the required NC size or shape. The facile, near-ambient solution synthesis conditions imply a substantial potential for NCs in photonic applications, which is further assisted by the high NC PLQE, and size- and shape-controlled optoelectronic properties. Fluorescent NCs are therefore well positioned to enable disruptive additions to applications such as light-emitting diodes (LEDs),24–27 lasers,28–32 bio-imaging,33–35 photovoltaics (PVs),36–39 or as novel quantum emitters.40,41
Although many NC applications are targeted at reducing energy consumption (for instance, more efficient lighting) or reliance on fossil fuels via solar energy harvesting, the principles of sustainability42 start before and extend beyond the end-application. The potential benefits at the moment still do not outweigh the most significant issue facing colloidal NCs, which is the presence of toxic heavy metals such as Cd, Pb and Hg. In this review, we will discuss the current status of NC-based photonic applications, and focus on the promising benign alternatives, contrasting their performance against the (generally) superior heavy-metal containing NCs and discuss potential pathways toward future developments.
1.1 Motivation for using NCs in photonic applications
The requirement for attaining a balance between performance and sustainability using NCs is multifaceted. Their low-energy, solution-based synthesis enables scalable and facile incorporation into devices, while tunable optoelectronic properties allow fine adjustments to optimize performance to meet application-specific requirements. Established semiconductor competitors for NCs in the photonic applications discussed in this review can be broadly categorized as inorganic bulk single-crystals (e.g. Si in PVs), epitaxially grown thin films, quantum wells and dots (e.g. GaN in LEDs), and organics (e.g. poly(9,9′-dioctylfluorene) polymers for OLEDs). NCs have a clear advantage over the inorganic competitors in terms of material and production costs with the potential to rival their performance. Conversely, the current cost of scaled-up NC production is about $10 per gram while mass-produced organic small molecules can be produced at 10–100 times lower cost.43,44 However, organic molecules and inorganic single-crystal or epitaxial semiconductors are unable to match the precisely controllable emission and absorption spectra provided by NCs. From a performance and sustainability point of view, NCs thus sit somewhere between inorganic bulk or epitaxially grown semiconductors and organic molecules.
1.2 Current state-of-the-art with heavy-metal NCs
Fig. 1 shows the emission wavelength range for a wide variety of NCs, from the bulk band gap down to the – to the best of our knowledge – shortest reported quantum confinement-induced emission wavelength. They are grouped into three categories. The first group is formed by NCs that only contain elements that are considered non-toxic (green points). The second group contains elements such as indium that are limited or geographically concentrated (orange squares), and the last group contains toxic elements which are banned by, for instance, the European Union's Restriction of Hazardous Substances Directive (RoHS), specifically cadmium, lead and mercury containing compounds (red triangles).
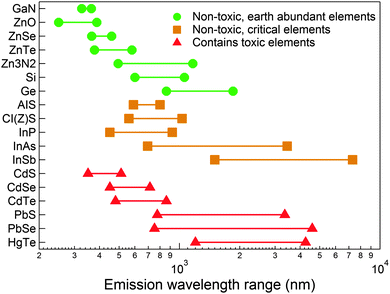 |
| Fig. 1 Emission tunability of selected semiconductor NCs. In most cases, the range varies from the bulk band gap down to the shortest confinement-induced, blue shifted emission. GaN,45 ZnO,46 ZnSe,47,48 ZnTe,49,50 Zn3N2,51 Si,52,53 Ge,54,55 AgInS2,56,57 CI(Z)S,58–61 InP,62–64 InAs,65,66 InSb,67,68 CdS,69 CdSe,70,71 CdTe,69 PbS,72,73 PbSe,74 and HgTe.75 HgTe is a semi-metal, so instead of the bulk band gap, emission from large NCs is reported from ref. 75. For AIS and CI(Z)S, we report the PL position, which falls significantly below the band gap due to defect-assisted emission. | |
It is immediately clear from Fig. 1 that the coverage of the technologically important visible and near-infrared (NIR) spectral windows is already feasible by heavy-metal-free NCs. However, Cd-based II–VI and Pb-based IV–VI NCs benefit from mature synthesis procedures that have enabled the preparation of highly monodisperse NCs with PLQEs that approach unity.7,76–78 Furthermore, the synthesis of these NCs extends well beyond the prototypical spherical quantum dots, to other structures that are highly relevant in photonic applications such as 1D NRs8 and 2D NPLs.10 Additionally, the development of core–shell heterostructures using II–VI and IV–VI core materials in which an inorganic wider-band gap material overcoats and passivates surface defects has led to highly efficient light emission, with PLQE in excess of 90% for CdSe/ZnS and CdSe/CdS NCs even in close-packed films.31 Similar enhancements can be achieved in NIR materials, with reported PLQEs in the range of 70–80% for core–shell PbSe/CdSe NCs,79 while core-only PbS passivated with Cl− ions have also shown QEs up to 90%.76
1.3 Synthesis of non-toxic alternatives
With the standard set at such a high level for heavy-metal containing NCs, developing less toxic alternatives is becoming an increasingly active area of research. There is already considerable potential without moving outside the group of II–VI semiconductors. Zinc-based NCs can cover UV to green wavelengths, and they are already a part of standard colloidal systems, with Zn(S, Se, Te) semiconductors being used as passivating shells for CdSe. Heavy-metal-free Zn-based NCs have shown promise with, for example, the development of highly monodisperse core–shell ZnSe/ZnS QDs with very narrow emission peaks (FWHM as low as 15 nm) and tunable blue emission.80,81 Emission beyond the bulk band gap can also be achieved by engineering Type II heterostructures in which carriers are spatially separated. For example, by varying the thickness of a ZnSe shell from one to five monolayers on a ZnTe core, emission can be red-shifted from 500 nm to 580 nm, albeit at the expense of a reduced oscillator strength and long exciton lifetime.49
Importantly, the shape control of Zn-based NCs has extended beyond spherical QDs to 1D quantum rods82–85 and 2D nanosheets.86,87 In the former case, ZnSe, ZnS and ZnTe rods with controllable aspect ratios have been synthesized using a phosphine-free technique via a ripening process through thermodynamically driven material diffusion.82 ZnSe/ZnS dot-in-rods88 and ZnS and ZnSe/ZnS core–shell NPLs89 have been synthesized with absorption and emission in the UV-blue via cation exchange, a reaction that replaces cations within an NC lattice with those in solution. ZnSe NPLs have also been produced with both wurtzite86 and zinc-blende87 structures, with a thickness of 1.4 nm for the former, but photoluminescence has yet to be reported. Further potential of zinc-based NCs has been demonstrated with zinc nitride (Zn3N2) NCs, which belongs to the II–V semiconductor group.51 In addition to tunable emission from green to the NIR as shown in Fig. 1, they possess QEs of over 50%,51 remarkable performance considering this was the first report on the synthesis of zinc nitride NCs.
Epitaxially grown III–V semiconductors have enjoyed considerable success in photonic applications for decades, forming the basis for state-of-the-art LEDs90 and lasers,91 for example. Not surprisingly, the development of colloidal III–V semiconductor NCs has been pursued nearly as long as the II–VIs.92–94 In addition to their relatively benign elements, direct band gaps and potential to cover the full visible to the NIR spectrum makes them a very attractive class of materials. However, finding appropriate precursors for colloidal synthesis has been hampered by the high covalency of these compounds,21,95,96 requiring high temperatures for both nucleation and growth stages, resulting in large NC size-dispersions.95 For example, early synthesis protocols for InP NCs were energy intensive and yielded poor-quality particles with broad size distributions.97–99 In spite of these challenges, remarkable progress has been made with InP and InAs, initiated with a low-temperature route developed by the group of X. Peng in 2002.100 In a later work, introducing fatty amines as activation reagents had dual benefits of lowering reaction temperatures (below 200 °C) and allowing for ZnS shell growth in a one-pot synthesis to produce high quality, monodisperse InP and InP/ZnS QDs with broad size tunability.62 Importantly, more recent progress has also enabled narrow emission linewidths and high PLQE from InP/ZnS NCs,101 in addition to the wide emission tunability shown in Fig. 1.
The search for appropriate precursors96 remains an important focus in the development of III–V NCs with narrow size distributions. Separating the nucleation and growth stages, as is the case in II–VI NC syntheses, is an outstanding problem for III–Vs. Toward this objective, it was found that using less reactive group-V sources, such as (trimethylgermyl)arsine, resulted in significantly improved InAs QD size distributions.96 Finding appropriate precursors for Ga-based NCs, on the other hand, has proven to be more difficult. A reaction has been proposed in which Ga(III) halides are transmetalated with n-butyllithium, followed by a reduction to gallium.102 GaAs NCs, for example, can be formed by reacting Ga with Mg3As2.102 In the same work, this reaction scheme was also demonstrated to make InP, InAs, and GaP QDs. With the exception of InP, however, size control remains an open challenge. An important extension to shape control has been demonstrated with the growth of 1D quantum rods of InAs,103 InP,103,104 and GaAs,103 with technologically important NIR emission from InAs rods.103
An alternative to the direct-synthesis procedures is given by cation exchange, circumventing the challenging direct synthetic protocols, as illustrated in Fig. 2. It has recently been used to produce GaAs, InAs, GaP, and InP with relatively narrow size dispersions, while avoiding the challenges associated with the traditional so-called hot-injection synthesis.21–23 This synthetic route also has the advantage of preserving the original NC conformation, opening up the control of shape in these zinc blende NCs, including 1D rods and 2D sheets. A potential drawback is the incomplete removal of Cu that is used in an intermediate stage, and often quenches the PL.105 Recent achievements on CdTe quantum disks,106 CdSe/CdS105 and ZnSe/ZnS dot-in-rods88 suggest that it can be alleviated, however, opening an avenue for fluorescent shape-controlled III–V NCs.
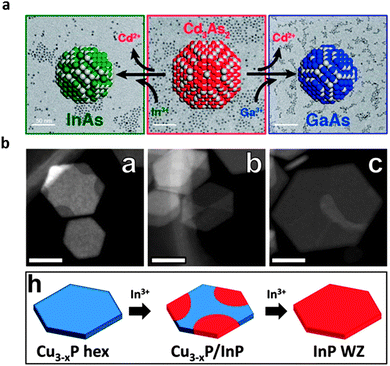 |
| Fig. 2 (a) Illustration of the cation exchange reaction used to produce monodisperse III–V NCs (including GaAs, InAs, GaP, and InP) by substitution of group 13 ions in cadmium pnictide NCs. Reproduced with permission from ref. 21. (b) Preparation of hexagonal WZ InP NPLs via a cation exchange of Cu3−xP with In3+. Adapted with permission from ref. 22. | |
Multinary compounds, such as ternary I–III–VI2 chalcogenides are recent additions to the colloidal semiconductor NC family, but they mark a shift from simple binary NCs to more complex systems with added control provided by compositional tunability. CuIn(S, Se)2 and AgIn(S, Se)2 are prominent examples that provide access to emission from the visible to the NIR as indicated in Fig. 1, with relatively high QEs of 80% for CIS/ZnS33 and 35% for core-only AgInS2 (ref. 57). Due to the wide range of stoichiometric variations and complex crystal structures, high density sub-band gap states are formed (Fig. 3(a)). This results in characteristic broad emission linewidths and long lifetimes.107,108Ref. 109 provides a comprehensive overview of the synthesis of ternary and quaternary chalcogenides, so it will not be discussed here.
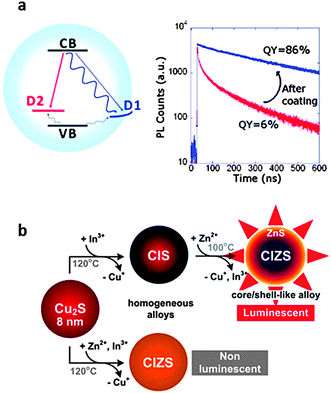 |
| Fig. 3 (a) Left: schematic of radiative (straight arrows) and nonradiative (wavy arrows) recombination pathways for CIS NCs, with an electron in a quantized conduction band (CB), and a hole trapped at a surface (D1) or internal (D2) defect. Right: elimination of the fast, nonradiative D1 decay channel by overcoating CIS NCs with ZnS shells results in a substantial increase in quantum efficiency. Adapted with permission from ref. 110. (b) Reaction scheme depicting the synthesis of homogeneously alloyed CIZS and luminescent CIZS/ZnS core–shell alloy NCs from pristine Cu2S NCs. Reproduced with permission from ref. 58. | |
Multinary chalcogenides can be synthesized using a route similar to the cation exchange approach used to produce III–V NCs.58,111,112Fig. 3(b) illustrates the partial cation exchange reaction that has been used to produce CuInS and CuInZnS (CIZS)111 with controllable size and composition starting from Cu2S NCs.58 Because the size and shape of the NCs remain unchanged from the Cu2S template, it should again enable the synthesis of geometries that already exist for Cu2S, including rods,113,114 nanodisks113,115 and nanosheets.113
While III–V and I–III–VI semiconductors present realistic alternatives to the heavy-metal based NCs, the presence of critical elements is also a concern for long-term sustainable application of colloidal NCs. Indium is frequently highlighted in this regard since it is considered to be in limited supply116 making it susceptible to price instability due to the demand in a variety of other micro-electronic applications and its geographical concentration of nearly 50% of the world's resources in China.117 In our specific case, the indium supply may not be a serious issue, particularly due to the small quantities required for NC-based applications.118 Nevertheless, in the long term we are bound to search for alternatives. This makes silicon and germanium NCs especially attractive for photonic applications, providing non-toxic solutions as well as seamless integration into existing Si-based micro-electronic applications. Although bulk Si and Ge have indirect band gaps, quantum confinement induces a direct-band gap transition in NCs of these materials, enabling efficient light emission.119,120 Relatively monodisperse Si QDs have been prepared via various bottom-up synthesis procedures53,121–124 with QEs as high as 75% with surface modification,124 but emission is limited to blue and green wavelengths, with red emission (as well as generally higher QE) only obtained with top-down preparation, such as electrochemical etching of bulk Si.125 Recently, free standing two-dimensional Si nanosheets with thicknesses controllable between 1 and 13 nm have been synthesized by chemical vapour deposition.126 Emission was shown to be tunable from blue to red (387 to 775 nm), and these Si nanosheets were also used as emitting layers in a blue-emitting LED, which may be considered a significant accomplishment that will be further discussed in Section 2.3.
The relatively small 4.2 nm exciton Bohr radius in bulk Si127 necessitates NCs of approximately that size or smaller to tune the absorption and emission spectra via quantum confinement. In contrast, the large 24 nm exciton Bohr radius128 combined with the small bulk band gap of 0.67 eV of Ge provide substantial room for quantum-confinement induced tuning of the optical properties, as reflected in the emission wavelength range in Fig. 1. However, the synthesis of high-QE Ge NCs is still an ongoing challenge due, in large part, to the strong covalent nature of Ge, entailing high crystallization temperatures.129,130 Similar to other NCs, growth of passivating shells substantially improves PLQE, with the epitaxial growth of ZnS and CdS on colloidal Ge QDs resulting in 1–3 orders of magnitude enhancement to NIR emission.131 Despite the present synthesis challenges, the shape control of Ge NCs has already expanded beyond spherical QDs to tetrahedral,132 triangles,133 nanocubes,134 nanowires135 and nanosheets.136,137
2. Light-emitting diodes, displays and lighting applications
2.1 Introduction
The 2014 Nobel Prize in Physics awarded for the invention of blue LEDs90 highlights broad impact LEDs have on providing energy efficient lighting. LED efficiency has been doubling roughly every 36 months, following what is known as Haitz's law,138 with efficiencies that are now double that of compact fluorescent lamps (CFLs).139–141 Efficiency, however, is an incomplete measure of the efficacy and sustainability of LEDs. Their cost and scalability are currently limited to a large extent by low-yield, high-energy epitaxial growth of, for example, InGaN for blue- and green-emitting LEDs.
Relatively new demands created by consumer technologies such as portable electronics and flat-panel displays have accelerated the need for facile, large area solutions. At present, LEDs are predominantly used in display applications, strongly motivating the search for efficient red, green, blue (RGB) emitters amenable to the large areas and thin dimensions required by these applications. Crucially, with already substantial but still growing demand for display technologies, special attention needs to be devoted to sustainability. Performance-wise, important metrics for LEDs are their external quantum efficiency (EQE; the number of emitted photons to the number of injected electrons), peak brightness (cd m−2), operating voltage (conversion of electrons to photons) and lifetime measured in hours, and these metrics will be used to discuss the viability of LEDs based on sustainable materials below. We only list EQE for NC-LEDs in Table 1 since the other metrics are not always reported in literature.
Table 1 Comparing NC materials used in LEDs in terms of external quantum efficiency (EQE)
Core material |
EQE (%) |
Colours |
Sustainability issue |
CdSe (ref. 142) |
20.5 |
Red |
Toxic metal |
Ref. 26
|
9.34 |
Green |
|
Ref. 26
|
1.7 |
Blue |
|
PbS147 |
2 |
Red |
Toxic metal |
InP158 |
3.46 |
Green |
Critical element |
CIS107 |
1.1 |
Yellow |
Critical element |
ZnSe163 |
7.83 |
Violet |
— |
ZnO/graphene164 |
— |
Blue/white |
— |
Silicon165 |
1.1 |
Red |
— |
2.2 State-of-the art colloidal NCs for LEDs
It was not long after the landmark synthesis of monodisperse QDs143 that an electrically injected NC-LED was first demonstrated in 1994.24 Since then, there have been significant improvements, including architectures employing all-inorganic as well as hybrid structures that utilize both organic and inorganic charge transport layers (CTLs).26,144–153 Notably, Kwak et al.26 developed NC-LEDs using CdSe/CdS/ZnS, CdSe@ZnS, and Cd1−xZnx@ZnS NCs to achieve peak brightness values of 23
040, 218
800, and 2250 cd m−2 for red, green, and blue emission, respectively, satisfying the requirements for both displays (100–1000 cd m−2) and general lighting (1000–10
000 cd m−2). Although the internal QE of CdSe-based NCs is close to 100% due to thermal mixing of dark and bright states, boosting the EQE has proved to be challenging. Recently, Mashford et al.146 developed a red-emitting NC-LED with an EQE of 18%, rivalling state-of-the-art OLEDs. As with other NC-LED structures,154–156 a film of ZnO NCs was used as an electron injection layer, and it was found that NC-LED performance was very sensitive to the NC emitting layer thickness. With further optimization, the performance of CdSe/CdS QD LEDs is now comparable to the state-of-the-art OLEDs, with an EQE of 20.5% and a lifetime of over 100
000 hours at 100 cd m−2.142 The device structure used in that work is shown in Fig. 4.
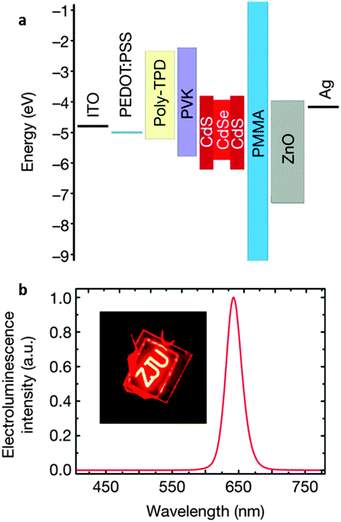 |
| Fig. 4 LED device structure used to achieve record efficiencies with CdSe/CdS core–shell QDs. Adapted with permission from ref. 142. | |
In addition to providing competition to OLEDs in terms of power conversion efficiency at technologically relevant brightness levels, operating lifetimes that were typically in the range of 102–104 hours26,144,145,154 (compared to 103–106 hours for OLEDs157) have now been increased to over 105 hours at 100 cd m−2. While achieving sufficient operating lifetimes was one of the greatest technical challenges for the incorporation of NCs in SSL applications, the presence of Cd-based NCs in these LEDs still precludes their use in consumer applications. Nevertheless, the performance of Cd-based NC-LEDs is both a confirmation that there is a future for NC-LEDs in general and a source of knowledge that can be transferred to non-toxic systems.
2.3 Heavy-metal-free NC LEDs
InP has notably emerged as a promising alternative due to its broad spectral tunability and maturing synthesis, which is now capable of yielding monodisperse QDs with linewidths as low as 38 nm FWHM.101 In the past few years there have been numerous reports of InP-based LEDs, with rapidly improving performance.27,62,158–161 For instance, green-emitting (500–520 nm) InP cores passivated by composition gradient ZnSeS shells were incorporated into an inverted device structure (Fig. 5) to obtain an EQE of 3.46% and a peak brightness of 3900 cd m−2.158 Although high NC QEs, in the range of 60–70% have been reported for blue emission,162 and even values above 90% for red emitting InP-based NCs,118 direct charge-injection blue LEDs have not yet been reported and red LEDs exhibit inferior performance (Table 1).
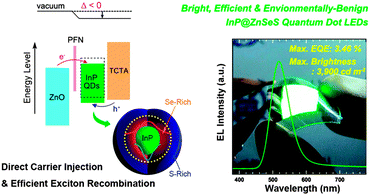 |
| Fig. 5 Architecture of InP/ZnSeS QD-based LEDs with ZnO and 4,4′,4′′-tris(N-carbazolyl)triphenylamine (TCTA) as electron and hole transport layers, respectively, used to achieve an EQE of 3.46%. Reproduced with permission from ref. 158. | |
In spite of the challenges associated with intra-band trap states in ternary chalcogenides, CIS-based NCs have also been successfully incorporated into direct-injection LEDs,107,166,167 albeit with modest results. The best performance to date has been achieved using yellow-emitting CIS/ZnS in a hybrid organic–inorganic architecture similar to that used for Cd-based LEDs (see Fig. 4), with EQE and peak luminance of 1.1% and 1564 cd m−2, respectively.107 Although green, yellow and red CIS-based LEDs have been reported,167 blue emission remains to be demonstrated. Another significant challenge for CIS LEDs is donor–acceptor pair-broadened emission, which is a critical issue for display applications that have high demands for colour purity. Steps toward solving this issue includes the development of CuInS2–ZnS (CIZS) alloyed cores where size-dependent shifts with concomitant short PL lifetimes (<100 ns) were observed.168 Similar CIZS cores were used with a double ZnSe/ZnS shell to obtain green, yellow and red emissions with lifetimes three orders of magnitude shorter than trap-emission lifetime,167 though emission linewidths remained broad.
Looking back at Fig. 1, there is an attractive possibility to cover the visible to the NIR spectrum using silicon NCs.119 Silicon is earth-abundant, non-toxic, and already dominates the microelectronic industry, making it a highly sustainable option. Efficient light emission from Si NCs (PLQEs in the range of 75%)124,169 is enabled by the indirect to direct band gap transition when translational symmetry is removed in the quantum confined regime. Moderate EQEs up to 1.1% have been demonstrated for red-emitting Si NC LEDs, at a noteworthy low turn-on voltage of 1.8 V.170 Broad linewidths and difficulty in achieving blue emission are among the challenges that Si NC-LEDs still face, particularly for integration into display applications.165 A promising route toward blue emission has recently been shown with two-dimensional Si nanosheets with emission tunable from 387 to 775 nm (corresponding to thicknesses ranging from 1 to 13 nm), which have been used to make a blue LED.126 Although the Si nanosheets in that work were prepared by chemical vapour deposition, it should motivate the development of colloidal analogues.
NCs have potential to provide a versatile addition to the LED family (i.e. a single material capable of emitting from blue to red), but synthesis efforts have not yet achieved the required quality in the strong confinement regime that is needed for efficient blue LEDs. A solution used large band gap II–VI zinc-based semiconductors Zn(O, S, Se, Te), where efficient blue emission can be realized for larger NCs. Indeed, ZnO164,171,172 and ZnSe80,81,173 have been used to make LEDs emitting in the UV-blue region. ZnSe/ZnS has shown particular promise, with an EQE of 7.8% for violet emission,163 substantially better performance than Cd-based LEDs at a similar wavelength.26
3. Photovoltaics
The ease of processing and the possibility of light and flexible PV panels have driven the search for solution processed materials capable of competing with the state-of-the-art bulk PV materials such as silicon or CIGS. Added to the general benefits of solution processed PVs, tunable absorption spectra (extending into the NIR, beyond the Si band gap) and multiple exciton generation (MEG, the creation of more than one electron–hole pair with one incident photon) possibilities have triggered intense efforts for QD-PVs over the last decade. Stability in air during both module fabrication and implementation without the need for inert conditions provides a further advantage of QDs, especially in light of the stability issues of their organic competitors.174 Harvesting more of the solar spectrum with NCs and the prospect of MEG38 offering a route to exceed the Shockley–Queisser single-junction limit have provided good reasons for optimism. This is tempered somewhat considering that Pb-based QD-PV power conversion efficiencies (PCE) have risen from less than 1% in 2005 (ref. 175) to 9.2% in 2015 (ref. 176), still short of other emerging technologies such as dye-sensitized or perovskite-based solar cells (see Fig. 6).
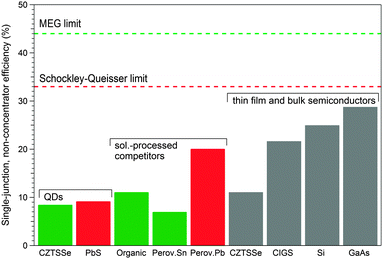 |
| Fig. 6 Comparison of record power conversion efficiencies for PVs based on CZTSSe177 and PbS QDs,176 organics, perovskites (Pb-based182 and Sn-based183,184), and more established CZTSSe and CIGS thin films and Si and GaAs bulk semiconductors (unless otherwise referenced, data were taken from the NREL Research Cell Efficiency chart185). Shockley–Queisser186 and MEG187 limits are shown using red and green dashed lines, respectively. | |
Interestingly, in contrast to the light-emitting applications discussed in Section 2, the performance of PV cells comprised of sustainable colloidal NCs is already comparable to the state-of-the-art in heavy-metal QD-PVs, with a PCE of 8.5% for CZTSSe NCs177 and 7.2% for CIS/ZnS QDs.37Fig. 6 compares record PCEs for QD-PVs with both solution-processed competitors and established thin film and bulk semiconductor PVs, all in single-junction mode.
Although quantum confinement and size-controlled optical properties offer tantalizing possibilities, they are not necessities for PV applications. For instance, excellent performance has been achieved by sintering all-inorganic CZTSSe NCs into thin films to create high NC packing densities for efficient charge transport,177,178 with the disadvantage of high temperatures required to add selenium lost (or replace sulphur with selenium). Strategies to increase NC packing density while avoiding energy-intensive sintering include using other NC geometries such as CuInSe2 nanowires179 (larger contact area) or organic to inorganic ligand exchange (smaller NC–NC distance).180 Another strategy to improve carrier transport is to link NCs together with composition matched inorganic ligands.181 Specifically, annealing chalcogenidometallates of Cd, Pb, and Bi with CdSe, PbSe, and Bi2Te3 NCs, respectively, at temperatures as low as 250 °C resulted in drastically enhanced electron mobilities.181 In addition to Bi2Te3, this technique can in principle be extended to other non-toxic NCs such as CZT(S, Se) and CIS. The prospect for sustainable NC-PVs is therefore multifaceted, with efforts to achieve bulk-like charge transport NCs providing an alternative means of benefitting from a solution-processed PV. With this approach, a facile preparation of bulk-like semiconductor PVs can be accomplished under relatively undemanding conditions, starting from solution-based NCs. Toward this objective, sustainable materials such as CZT(S, Se) and CIS are already well-positioned.
4. Colour converting phosphors
The traditional strengths of NCs as optically stable and highly fluorescent phosphors were recognized early on in their application as biolabels,33,34,188–190 where such properties form key requirements. The approaches discussed in the previous two sections for LEDs and solar cells relied on the efficient conversion of charge to light or vice versa, which can be circumvented for both applications by falling back on the primary use of NCs as bright and stable colour-converters.
4.1 Phosphors for PVs
A recent development is to use NCs in luminescent solar concentrators (LSCs) to amplify the collection efficiency of PV technologies with colour-converting phosphors. The principle is illustrated in Fig. 7, where a transparent planar waveguide slab is coated or doped with luminescent dyes or particles that absorb light and emit longer wavelength light that is channelled via total internal reflection to a solar cell.191–194 Up to a 10 fold concentration of solar light can be realized with LSCs,195 along the obvious advantage of relying on mature PV technologies for energy conversion, with an added advantage of substantially minimized area.
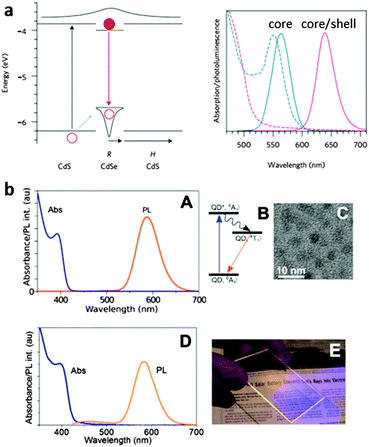 |
| Fig. 7 Successful strategies for the incorporation of NCs into luminescent solar concentrators. (a) A band diagram of NC heterostructures used to shift emission outside of the absorption band. Adapted with permission from ref. 193 and (b) using dopant emission to completely avoid reabsorption. Adapted with permission from ref. 196. | |
Given the inherently small Stokes shifts in QDs, reabsorption of colour-converted light is a significant challenge for QD-LSCs, becoming a substantial loss in large-area implementations that require long optical path lengths. Fig. 7 illustrates two strategies for obviating reabsorption, ‘Stokes-shift engineering’ with core–shell NCs193,197,198 and impurity-doped NCs,196 with the emission wavelength shifted outside the absorption band in both cases. The first approach is quite general, and in principle can be extended to heavy-metal-free NCs with a voluminous large-band gap shell, confirmed by red shifted emission from InP/thick shell ZnS QDs.162 Currently, the more effective approach is to introduce transition-metal ion dopants into a NC host.197,199–201 Here, emission from intraband states formed by the dopant ions is far removed from the absorption edge of the NC. Non-toxic ZnSe/ZnS QDs doped with Mn2+ ions have been shown to be effective in LSCs, avoiding all reabsorption losses (Fig. 8).196 Although promising both from sustainability and performance perspectives, expanding to smaller-gap materials is a necessary step to harness more of the solar spectrum. InP again provides good performance, with Cu+ dopant PLQEs as high as 35–40% and tunable NIR emission obtained in InP/ZnSe QDs,202 and the results clearly show their potential for enhancing PV performance as LSCs.
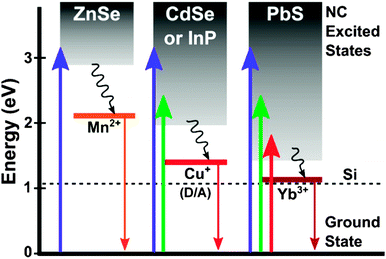 |
| Fig. 8 Possibilities for semiconductor-dopant combinations indicating potential for covering more of the solar spectrum with for instance InP doped with Cu+. Reproduced with permission from ref. 196. | |
Future directions should include extending the absorption deeper into the NIR, which can be accomplished by finding appropriate dopants for materials such as InAs, InSb, and Ge. Compositional tunability of multinary NCs provides another approach. In CIZS NCs for example, variations in starting ratios of Cu+
:
In3+
:
Zn2+ were capable of shifting the PL from 880 to 1030 nm.58 The large Stokes shift of Si NCs (absorption peak in the ultraviolet and emission possible from 300 to above 1000 nm)203 could also make them candidates for use in LSCs, though to the best of our knowledge no reports on their use in this context exists to date.
4.2 Phosphors for lighting
In a different sector, CFLs have been the primary competitor of the inefficient, yet aesthetically pleasing incandescent bulb invented by Thomas Edison in 1880. The presence of mercury in CFLs204 is a major concern, however, motivating the development of low-cost, heavy-metal-free white light sources. Using NCs as phosphors in combination with established InGaN blue-LEDs again relies on the intrinsic strengths of NCs while avoiding problems with inefficient charge transport.
In this regard, InP and CIS NCs in particular are beginning to offer viable solutions as colour-converters for lighting,118 where large-area implementations (50 × 50 cm) of InP/ZnS QDs have already been demonstrated.206 Their versatility for this application is further highlighted by recent demonstrations that combine intrinsic and dopant emission in a single system. For example, as illustrated in Fig. 9, a Cu-doped InP/ZnS/InP/ZnS multilayer structure yielded a WLED with a high colour-rendering index (CRI).205 Similarly, high quality white light was generated using Mn-doped CIS/ZnS NCs with bichromatic emission enabled by separating Mn2+ ions from the CIS core with a ZnS barrier.207 In both cases, the NCs down-converted blue light from commercially available InGaN LEDs that also provided the blue portion of the white-light spectrum.
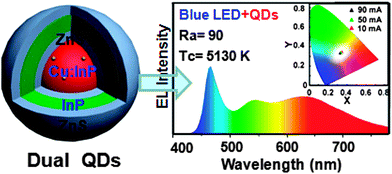 |
| Fig. 9 White light generation using dual emission (Cu dopant and InP layer) from a heavy-metal-free NC phosphor with blue light provided by the excitation LED. Reproduced with permission from ref. 205. | |
In spite of the promise demonstrated in the above examples for heavy-metal-free NCs, a substantial challenge remains in improving emission stability at elevated temperatures, as WLEDs for lighting can locally reach 100 °C under operating conditions.208 In this regard, the PL temperature-dependence of InP/ZnS QDs has been investigated,209–211 where the improved performance of the core–shell system over core-only InP was attributed to reduced electron trapping on the surface.210 In addition to core–shell heterostructures, replacing long organic ligands on core-only InP with short inorganic ligands (S2− as well as Sn2S64−), resulted in similar thermal stability, including PL recovery in cycling between 300 and 800 K.210
5. Lasers
Lasers are arguably one of the most important technological developments of last century, finding broad, integral use in consumer electronics, industrial manufacturing, medicine and scientific research. Emerging and next-generation applications (for instance, optical computing, quantum computing, lab-on-chip devices, etc.) are creating demand for lasers that can be integrated onto a diverse array of platforms, including the micro- and nano-scales.
Solution-processed materials provide a route toward flexible, substrate-independent gain media that can be incorporated into a wide variety of cavity geometries31,212–216 using deposition techniques such as inkjet printing and spray coating. However, moving beyond laboratory demonstrations of lasing – where large, powerful pulsed pump sources are available – has proven to be a significant challenge. Laser action under continuous wave (cw) excitation or electrical injection is a crucial feasibility achievement necessary for the transfer to practical applications. The former enables the use of small, inexpensive diode laser pump sources (recently demonstrated for CdSe nanosheets28), and the latter is generally considered the ultimate goal which would permit integration into microelectronic applications.
NCs have gained significant attention for their use as gain media in large part due to their confinement-induced quantization of energy spectra that results in discrete energy levels, leading to enhanced band-edge density of states as well as low threshold lasing.31,217,218 The delta-like density of states in quantum dots further predicts a temperature independent threshold when the separation of energy levels is greater than the thermal energy, avoiding thermal depopulation.217 The primary challenge in using NCs as gain media is overcoming the exact balance between absorption and stimulated emission. For example, the doubly degenerate electron ground state of CdSe QDs results in the complete reabsorption of SE if only one carrier is excited, reaching transparency but no net gain. Therefore, a solution to break this balance and enable gain is to excite, on average, multiple electron–hole pairs (multiexcitons) per QD. However, the resulting high carrier densities in the small volume of QDs results in highly efficient Auger recombination.219 Mitigating the effects of Auger recombination has therefore been a primary objective for the realization of practical NC-based lasers.
Another solution is to break the balance between (multi-exciton) absorption and emission, enabling single-exciton gain and thereby eliminating Auger recombination. This concept is shown schematically in Fig. 10(a). Klimov and co-authors first demonstrated this by engineering type II CdS/ZnSe core–shell NCs to spatially separate the electrons and holes, shifting the biexciton absorption to the blue of the emission line, leading to single-exciton optical gain and stimulated emission.220 Dang et al.31 have shown that low-threshold single-exciton stimulated emission and lasing can also be accomplished with CdSe/Cd0.5Zn0.5S type I core–shell QDs. Here, a graded core–shell interface likely resulted in a smooth confining potential, which has been shown to also reduce Auger rates.221,222 Yet, while heterostructure QD lasers operated under nanosecond optical pumping,214,223,224 CW-pumped lasing has remained elusive. Auger recombination can also be avoided by using 2D NPLs. This system provides the colloidal analogue to epitaxial quantum wells (Qwells), and is characterized by confinement in only one direction, with free motion in the plane of the NPL. A consequence of this is that, unlike QDs, momentum conservation rules apply more strictly in colloidal quantum wells (CQwells) which reduces nonradiative Auger recombination transition possibilities. A combination of supressed Auger and enhanced biexciton binding energies28 has resulted in ultralow stimulated emission thresholds on close-packed CdSe,28,32,225 and lasing under CW pumping conditions.28
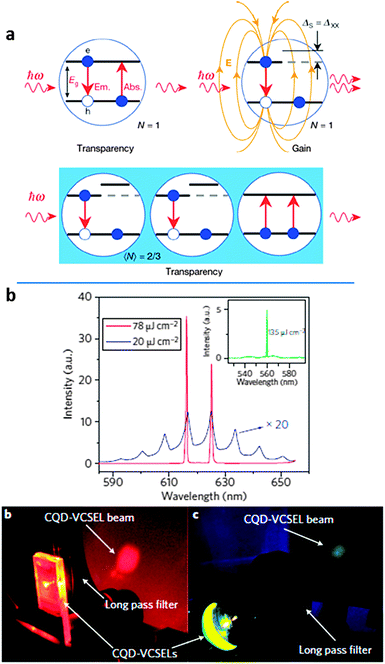 |
| Fig. 10 (a) Illustration of single exciton gain. Top left: optical transparency but no gain when stimulated emission and absorption overlap requires the excitation of multiple electron–hole pairs in each QD to achieve gain. Top right: shifting the position of the absorption energy with respect to the emission energy of core–shell QDs lifts the requirement of generating multiple excitons, enabling single-exciton gain. Reproduced with permission from ref. 220 (b) lasing using CdSe/Cd0.5Zn0.5S core–shell QDs imbedded between distributed Bragg reflectors. Adapted with permission from ref. 31. | |
While requirements for efficient lasing are clear from the results obtained on shape-controlled CdSe and their heterostructures, plotting some of the record low stimulated emission thresholds (under femtosecond pulsed optical pumping) for colloidal NCs versus emission wavelength in Fig. 11 highlights the scarcity of heavy-metal-free NC gain media. The few reports of lasing using more sustainable gain materials include InP/ZnS in a liquid crystal grating cavity213 and InGaP/ZnS in a VCSEL cavity.226 Although the SE threshold shown in for InP/ZnS NCs is significantly higher than other NCs at similar wavelengths (note the log–log scale), this work predated more recent improvements in the synthesis of InP NCs. Given the substantial improvements in the past few years for InP, there are good prospects for its use as a gain medium. For instance, nanosecond pumped lasing with emission at 657 nm has been demonstrated for InGaP/ZnS core–shell QDs in a flexible VCSEL configuration,226 enabling mechanical wavelength tuning (600–660 nm in that report). Although no SE threshold was provided, the stringent gain/loss requirements of VCSEL structures make them a particularly challenging configuration for any gain media. Combined with the demonstrated nanosecond lasing, the performance of InGaP NCs in this structure demonstrates their strong potential as a green alternative to Cd-based NC gain media.
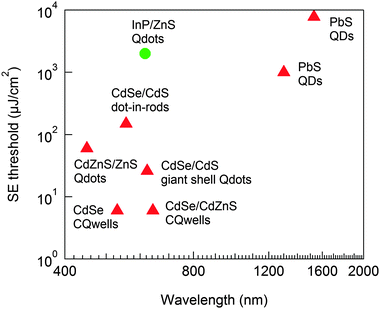 |
| Fig. 11 Stimulated emission thresholds as a function of wavelength for InP/ZnS QDs,213 PbS QDs (1.3 μm, ref. 227 and 1.52 μm, ref. 218), CdSe/CdS dot-in-rods,217 CdZnSe/ZnS QDs,31 giant shell CdSe/CdS QDs,228 CdSe/CdZnS CQwells,225 and CdSe CQwells,28 highlighting both the lack of heavy-metal-free and the high threshold and scarce reports of NIR NC gain media. | |
Low-threshold, high gain ‘green’ NC lasers will ultimately depend on solving the related problems of minimizing Auger recombination, circumventing issues associated with multiple exciton generation and achieving high quantum efficiency. As discussed above, three approaches to solving these problems include enabling single-exciton lasing by engineering Type II heterostructures, smoothing confinement potentials to reduce Auger rates and most recently eliminating Auger recombination with CQwells. Auger rates in InP QDs were found to decrease as a function of CdS shell thickness (determined from increasing multiexciton lifetimes), with a simultaneous suppression of blinking.229 Furthermore, the rising QE of InP/ZnS QDs (now as high as 90% for red emission118) also places this ‘green’ material among the best NCs for this metric. Progress toward shape-controlled green materials (with a viable route offered by cation exchange) may also pave the way to low-threshold CQwell III–V NC lasers capable of, at minimum, sustaining population inversion under CW pumping.
6. Sustainable future quantum technology with colloidal NCs
So far, applications in LEDs, lasers and photovoltaics have been feasible with novel, heavy-metal-free, low-toxicity materials. However, the quantum nature of the colloidal NC emission also makes them attractive candidates in fields including quantum cryptography, communication and computation.41 Efficient single photon and entangled photon pair emission represent core achievements to make these new technologies a reality. Producing non-classical light from colloidal NCs hinges on a thorough understanding of their band-edge fine structure, complete suppression of blinking, and elimination of Auger recombination. In this regard, CdSe NCs are again particularly well-developed.
Considered ‘artificial atoms’ because of their discrete energy levels, the fine structure of colloidal NCs has been the subject of both theoretical230–234 and experimental235–239 work. CdSe QDs have long served as the model system, with an eightfold degenerate band-edge exciton, and each state having a specific angular momentum projection, energy, and transition oscillator strength.230 Control over these states is crucial for successful application of CdSe QDs as quantum emitters, and understanding the underlying mechanisms such as the electron–hole exchange interaction and crystal field and shape anisotropy is therefore required. The exchange interaction, for example, is a parameter that can be manipulated by adjusting the overlap of the electron and hole wavefunctions with quasi-Type II CdSe/CdS QDs where substantially reduced fine structure splitting was observed for increasing shell thickness (i.e. reduced e–h overlap).240 Similarly, changing the core and/or rod diameter in CdSe/CdS dot-in-rods has been shown to be effective in tuning fine structure splitting with additional control provided by anisotropy.236 Experimental efforts have recently culminated in the observation of the entire 8-state band-edge fine structure of CdSe NCs (with zinc blende crystal structure), revealed by asymmetry-induced splitting by the group of B. Lounis, as shown in Fig. 12.237
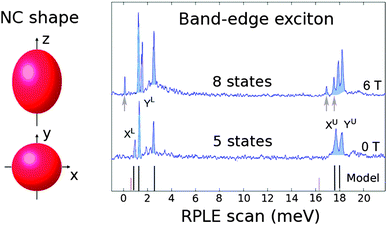 |
| Fig. 12 Asymmetry-induced splitting of the 8-state band-edge fine structure of zinc blende CdSe. Reproduced with permission from ref. 237. | |
With these well-developed properties, room temperature single photon emission has already been demonstrated with colloidal CdSe/ZnS QDs40,241,242 and CdSe/CdS dot-in-rods243 with advantages of higher PL yield and better photostability compared to other single photon sources that include single atoms244 and single molecules.245 Tuning the anisotropy in Cd-based dot-in-rods provides a further advantage. For instance, the dipole moment in quantum rods is oriented along the rod axis,246 allowing manipulation of polarization by orienting rods at different angles.41 Obstacles that can severely diminish the stability and reliability of quantum emission in the form of blinking and Auger recombination are now well-controlled in CdSe NCs, with the development of giant shell QDs, demonstrated for CdSe QDs and CdS shell thicknesses up to 8 nm.78,247,248 More recently, a new strategy to eliminate blinking completely has been demonstrated by encapsulating CdSe/CdS core–shell NCs in silica shells, which are in turn coated with thin gold layers, as illustrated in Fig. 13.249
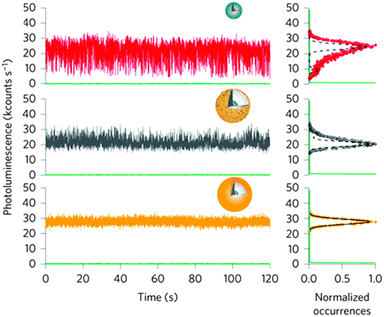 |
| Fig. 13 A strategy to eliminate blinking and strongly enhance stability is realized by encapsulating core–shell NCs in gold-coated silica shells. Adapted with permission from ref. 249. | |
Where do non-toxic NCs stand with respect to this high level of maturity in understanding and advanced functionality for Cd-based NCs? In some respects, they collectively sit where Cd-based NCs were about 10 years ago. Recent strides have been made, however, in developing an understanding of the challenges that are of particular relevance to future quantum technologies. Importantly, both experimental and theoretical efforts have begun to explore the fine structure of sustainable materials. On the experimental side, the nature of dark and bright exciton states corresponding to spin-allowed and forbidden transitions, respectively, has been investigated with temperature-dependent PL decay measurements for InP/ZnS,250 ZnSe251 and Si252 NCs. Meanwhile, atomistic pseudopotential calculations have investigated the relationship between the magnitude of the bright/dark exciton splitting and the size of GaAs, InAs and Si QDs.253,254
Similarly, fluorescence intermittency has been investigated in sustainable NCs, with early work conducted more than a decade ago for InP QDs.255 With respect to blinking suppression, many of the same techniques used for Cd-based NCs have been shown to be effective with non-toxic NCs. For example, overcoating InP QDs with ZnS shells was also found to strongly suppress blinking.256 In conjunction with tuning of other factors such as the particle size and the molar ratio of myristic acid/indium (MA
:
In), an on-time fraction up to 80% was achieved. A somewhat alternative strategy has been demonstrated with InP/CdS heterostructures.229 Here, the type II band alignment was posited for the suppression of blinking even for thin shells. However, avoiding the CdS shell necessitates the development of alternative type-II heterostructures. Investigations on PL intermittency in NIR InAs QDs with CdZnS shells have revealed blinking behaviour similar to that seen in CdSe,257 indicating that strategies to eliminate blinking are transferrable. Finally, the in principle general approach shown in Fig. 13 to eliminate blinking249 is independent of the core material, and thus provides a direction that can be applied to non-toxic NCs.
Strategies to mitigate Auger recombination in non-toxic NCs can also be taken from the substantial body of work in Cd-based NCs (see Section 5). Two of the approaches run parallel to blinking suppression (giant shell Type II QDs and alloyed shells for ‘wavefunction smoothing’). The third is provided via shape control with the strong suppression in Auger recombination observed in CdSe nanosheets.258 With regard to this third strategy, synthesis procedures have begun to develop for nanosheets of non-toxic materials such as Zn(S, Se, Te), which would form attractive new materials.86
There are interesting prospects for sustainable, solution processed NCs in future quantum technologies, bolstered by the knowledge gained on CdSe NCs. With maturing syntheses of green materials, the strategies already developed for CdSe NCs should accelerate their role in the future of quantum computing and communication. A key remaining question, however, is whether colloidal NCs can reach the material quality of their epitaxial analogues, which are significantly further developed as quantum emitters. For instance, electrically driven single photon emission has been demonstrated for InP grown on Si,259 and fine-structure splitting below 2 μeV for InAsP dots in InP nanowires for efficient polarized photon entanglement.260,261 To achieve such levels, clearly more work remains to be done.
7. Conclusions and perspective
Since their inception just over 30 years ago, the extraordinary progress in optimizing the synthesis, assembly and application of colloidal nanocrystals has uniquely positioned them to impact a wide array of photonic areas. Ultimate success in these applications, however, hinges as much on the sustainability of the base materials as it does on their performance. For NCs, moving beyond the well-developed Cd- and Pb-based materials therefore forms a crucial requirement.
In this review, we have highlighted some significant steps that have been taken towards sustainable NCs, including demonstrations of their use in photonic applications. The pursuit of these green materials has in many cases run in parallel to the seminal work with heavy-metal-containing NCs. For some sustainable material classes, such as III–V and IV group semiconductors, progress has lagged because of the difficulty in achieving the same material quality as the II–VIs, hindered by more demanding syntheses.21,52,95,96,262 Other heavy-metal-free options, such as ternary chalcogenides, and even Zn-based II–VIs, are by comparison in early stages of development, but are receiving considerable attention in response to the need for non-toxic NCs.
With regard to III–V NCs, notable progress in direct synthesis has been accompanied with the use of cation exchange, a method that has been generalized to other material classes. First, the synthesis of high-PL InP QDs62 with a spectral coverage that rivals CdSe, and converging performance indicates that these materials are accessible via traditional means. Cation exchange,21,23,58,83 on the other hand, is an emerging route used to obtain III–V NCs,21,22 Zn-based II–VI89 and even ternary chalcogenides.58 A prominent advantage of this approach is that it preserves the narrow size distributions as well as the shape of initial NCs, providing the possibility to obtain shape-controlled NCs that are difficult to grow via direct routes. Although the presence of toxic metals during synthesis might still form an issue, they do not remain in discernible quantities in the end-applications, and are contained in a controlled environment at the fabrication stage with appropriate waste disposal, satisfying the requirements for sustainable development of colloidal NCs.
Beyond the more traditional group IV, IV–VI, III–V, II–VI and I–III–VI NCs, there is also considerable excitement for other classes of solution processed materials. Some notable examples are represented by upconverting rare-earth doped NCs,263–267 lead halide perovskite NCs268–271 and 2D NCs from transition metal chalcogenides (TMCs).272–276 Recent work on rare-earth doped NCs has presented bright, tunable, blink-free, photostable upconverted emission from, for example NaYF4 (ref. 277), opening up possibilities for use in various photonic areas, as already demonstrated in biolabeling278 and single molecule sensors.279 Furthermore, amplified spontaneous emission (ASE) and lasing have been accomplished for red, green and blue wavelengths with upconverting core–shell NaYF4:Yb/Er@NaYF4 NCs.280 Due to the demanding conditions required to achieve population inversion for ASE and lasing, this work clearly demonstrates the substantial potential of this material class in advanced photonic applications. Considering the absence of toxic elements, these NCs should receive increasing attention over the coming years.
The meteoric rise of power conversion efficiencies using organic–inorganic lead halide perovskites (CH3NH3PbX3, X = Cl, Br or I) as solar cell absorbing layers has already positioned them as viable competitors to state-of-the-art PV technology.182,281–283 Another prominent development is the synthesis of monodisperse cesium lead halide perovskite NCs (CsPbX3, X = Cl, Br and I) with PLQEs up to 90%.268 Here, both quantum confinement and compositional adjustments produced spectrally narrow emission from 410–700 nm. Despite their potential, developing Pb-free perovskites remains a substantial challenge. One strategy to do so is to substitute Sn – another group 14 element – for Pb.183,184
In another area, the extraordinary properties of graphene have led to a resurgence of interest in other layered materials. TMCs such as Mo(S, Se)2 and W(S, Se)2, for example, are not new, but remarkable optoelectronic possibilities have emerged for individual 2D layers of these materials.272–276 For example, indirect TMC bulk band gaps become direct for monolayers,284 and coupled with a very large exciton binding energy in 2D MoS2 due to reduced dielectric screening,285 their prospect as light emitters is bright. Initial synthetic schemes for TMCs have mirrored those used for graphene, utilizing both top-down exfoliation276 and bottom-up chemical vapour deposition286 yielding atomically thin sheets. Progress in colloidal synthesis has been made with the synthesis of ZrS2287 and other TMC nanosheets.288 Nevertheless, uniform nanosheets with an extended lateral size at constant thickness remains a prominent ongoing challenge.287–289 From a sustainability perspective, since TMCs are free of toxic heavy metals and thus provide an attractive route toward green photonics with 2D materials, we expect substantially increased synthesis efforts in this direction.
Acknowledgements
The research leading to these results has received funding from the European Union 7th Framework Programme under grant agreement no. 604391 GRAPHENE Flagship, and no. 614897 (ERC consolidator grant TRANS-NANO, L.M.). The present publication is further realized with the support of the Ministero degli Affari Esteri e della Cooperazione Internazionale (grant IONX-NC4SOL, I.M.).
Notes and references
- B. Wessler and U. Tober, SPIE Eco-Photonics, 2011, 806515 CrossRef PubMed
.
- A. I. Ekimov and A. A. Onushchenko, JETP Lett., 1981, 34, 345 Search PubMed
.
- R. Rossetti, J. L. Ellison, J. M. Gibson and L. E. Brus, J. Chem. Phys., 1984, 80, 4464 CrossRef CAS PubMed
.
- L. Brus, J. Phys. Chem., 1986, 90, 2555 CrossRef CAS
.
- H. Weller, H. M. Schmidt, U. Koch, A. Fojtik, S. Baral, A. Henglein, W. Kunath, K. Weiss and E. Dieman, Chem. Phys. Lett., 1986, 124, 557 CrossRef CAS
.
- L. Spanhel, M. Haase, H. Weller and A. Henglein, J. Am. Chem. Soc., 1987, 109, 5649 CrossRef CAS
.
- C. B. Murray, D. J. Norris and M. G. Bawendi, J. Am. Chem. Soc., 1993, 115, 8706 CrossRef CAS
.
- X. Peng, L. Manna, W. Yang, J. Wickham, E. Scher, A. Kadavanich and A. Alivisatos, Nature, 2000, 404, 59 CrossRef CAS PubMed
.
- S. Ithurria, M. D. Tessier, B. Mahler, R. P. S. M. Lobo, B. Dubertret and A. L. Efros, Nat. Mater., 2011, 10, 936 CrossRef CAS PubMed
.
- S. Ithurria and B. Dubertret, J. Am. Chem. Soc., 2008, 130, 16504 CrossRef CAS PubMed
.
- J. Joo, J. S. Son, S. G. Kwon, J. H. Yu and T. Hyeon, J. Am. Chem. Soc., 2006, 128, 5632 CrossRef CAS PubMed
.
- M. A. Hines and P. Guyot-Sionnest, J. Phys. Chem., 1996, 100, 468 CrossRef CAS
.
- S. Kim, B. Fisher, H.-J. Eisler and M. Bawendi, J. Am. Chem. Soc., 2003, 125, 11466 CrossRef CAS PubMed
.
- L. Carbone, C. Nobile, M. De Giorgi, F. Della Sala, G. Morello, P. Pompa, M. Hytch, E. Snoeck, A. Fiore, I. R. Franchini, M. Nadasan, A. F. Silvestre, L. Chiodo, S. Kudera, R. Cingolani, R. Krahne and L. Manna, Nano Lett., 2007, 7, 2942 CrossRef CAS PubMed
.
- G. Jia, A. Sitt, G. B. Hitin, I. Hadar, Y. Bekenstein, Y. Amit, I. Popov and U. Banin, Nat. Mater., 2014, 13, 301 CrossRef CAS PubMed
.
- E. Cassette, B. Mahler, J.-M. Guigner, G. Patriarche, B. Dubertret and T. Pons, ACS Nano, 2012, 6, 6741 CrossRef CAS PubMed
.
- N. Gaponik, D. V. Talapin, A. L. Rogach, K. Hoppe, E. V. Shevchenko, A. Kornowski, A. Eychmüller and H. Weller, J. Phys. Chem. B, 2002, 106, 7177 CrossRef CAS
.
- V. Lesnyak, N. Gaponik and A. Eychmüller, Chem. Soc. Rev., 2013, 42, 2905 RSC
.
- X. Pang, L. Zhao, W. Han, X. Xin and Z. Lin, Nat. Nanotechnol., 2013, 8, 426 CrossRef CAS PubMed
.
- H. Xu, X. Pang, Y. He, M. He, J. Jung, H. Xia and Z. Lin, Angew. Chem., 2015, 127, 4719 CrossRef PubMed
.
- B. J. Beberwyck and A. P. Alivisatos, J. Am. Chem. Soc., 2012, 134, 19977 CrossRef CAS PubMed
.
- L. De Trizio, R. Gaspari, G. Bertoni, I. Kriegel, L. Moretti, F. Scotognella, L. Maserati, Y. Zhang, G. C. Messina, M. Prato, S. Marras, A. Cavalli and L. Manna, Chem. Mater., 2015, 27, 1120 CrossRef CAS PubMed
.
- B. J. Beberwyck, Y. Surendranath and A. P. Alivisatos, J. Phys. Chem. C, 2013, 117, 19759 CAS
.
- V. L. Colvin, M. C. Schlamp and A. P. Alivisatos, Nature, 1994, 370, 354 CrossRef CAS PubMed
.
- M. Achermann, M. A. Petruska, D. D. Koleske, M. H. Crawford and V. I. Klimov, Nano Lett., 2006, 6, 1396 CrossRef CAS PubMed
.
- J. Kwak, W. K. Bae, D. Lee, I. Park, J. Lim, M. Park, H. Cho, H. Woo, D. Y. Yoon, K. Char, S. Lee and C. Lee, Nano Lett., 2012, 12, 2362 CrossRef CAS PubMed
.
- Y. Kim, C. Ippen, T. Greco, M. S. Oh, C. J. Han, J. Lee, A. Wedel and J. Kim, J. Nanosci. Nanotechnol., 2014, 14, 8636 CrossRef CAS PubMed
.
- J. Q. Grim, S. Christodoulou, F. Di Stasio, R. Krahne, R. Cingolani, L. Manna and I. Moreels, Nat. Nanotechnol., 2014, 9, 891 CrossRef CAS PubMed
.
- V. I. Klimov, A. A. Mikhailovsky, S. Xu, A. Malko, J. A. Hollingsworth, C. A. Leatherdale, H.-J. Eisler and M. G. Bawendi, Science, 2000, 290, 314 CrossRef CAS
.
- J. Xu and M. Xiao, Appl. Phys. Lett., 2005, 87, 173117 CrossRef PubMed
.
- C. Dang, J. Lee, C. Breen, J. S. Steckel, S. Coe-Sullivan and A. Nurmikko, Nat. Nanotechnol., 2012, 7, 335 CrossRef CAS PubMed
.
- B. Guzelturk, Y. Kelestemur, M. Olutas, S. Delikanli and H. V. Demir, ACS Nano, 2014, 8, 6599 CrossRef CAS PubMed
.
- D. Deng, Y. Chen, J. Cao, J. Tian, Z. Qian, S. Achilefu and Y. Gu, Chem. Mater., 2012, 24, 3029 CrossRef CAS
.
- E. Cassette, M. Helle, L. Bezdetnaya, F. Marchal, B. Dubertret and T. Pons, Adv. Drug Delivery Rev., 2013, 65, 719 CrossRef CAS PubMed
.
- K.-T. Yong, H. Ding, I. Roy, W.-C. Law, E. J. Bergey, A. Maitra and P. N. Prasad, ACS Nano, 2009, 3, 502 CrossRef CAS PubMed
.
- P. V. Kamat, Acc. Chem. Res., 2012, 45, 1906 CrossRef CAS PubMed
.
- Z. Pan, I. Mora-Seró, Q. Shen, H. Zhang, Y. Li, K. Zhao, J. Wang, X. Zhong and J. Bisquert, J. Am. Chem. Soc., 2014, 136, 9203 CrossRef CAS PubMed
.
- O. E. Semonin, J. M. Luther, S. Choi, H.-Y. Chen, J. Gao, A. J. Nozik and M. C. Beard, Science, 2011, 334, 1530 CrossRef CAS PubMed
.
- E. H. Sargent, Nat. Photonics, 2009, 3, 325 CrossRef CAS PubMed
.
- X. Brokmann, E. Giacobino, M. Dahan and J. P. Hermier, Appl. Phys. Lett., 2004, 85, 712 CrossRef CAS PubMed
.
-
F. Pisanello, L. Martiradonna, P. Spinicelli, A. Fiore, J. P. J. P. Hermier, L. Manna, R. Cingolani, E. Giacobino, A. Bramati and M. De Vittorio, IEEE Proc. 11th Int. Conf. Transparent Opt. Networks, 2009, 1.
-
P. T. Anastas and J. C. Warner, Green Chemistry: Theory and Practice, Oxford University Press, New York, 1998 Search PubMed
.
-
J. Oliver, BCC Res. Pap. NAN027C, 2011.
- Y. Shirasaki, G. J. Supran, M. G. Bawendi and V. Bulović, Nat. Photonics, 2012, 7, 13 CrossRef PubMed
.
- O. I. Micic, S. P. Ahrenkiel, D. Bertram and A. J. Nozik, Appl. Phys. Lett., 1999, 75, 478 CrossRef CAS PubMed
.
- S. Monticone and R. Tufeu, J. Phys. Chem. B, 1998, 102, 2854 CrossRef CAS
.
- M. A. Hines and P. Guyot-Sionnest, J. Phys. Chem. B, 1998, 102, 3655 CrossRef CAS
.
- L. S. Li, N. Pradhan, Y. Wang and X. Peng, Nano Lett., 2004, 4, 2261 CrossRef CAS
.
- J. Bang, J. Park, J. H. Lee, N. Won, J. Nam, J. Lim, B. Y. Chang, H. J. Lee, B. Chon, J. Shin, J. B. Park, J. H. Choi, K. Cho, S. M. Park, T. Joo and S. Kim, Chem. Mater., 2010, 22, 233 CrossRef CAS
.
- Y. Jun, C.-S. Choi and J. Cheon, Chem. Commun., 2001, 101 RSC
.
- P. N. Taylor, M. A. Schreuder, T. M. Smeeton, A. J. D. Grundy, J. A. R. Dimmock, S. E. Hooper, J. Heffernan and M. Kauer, J. Mater. Chem. C, 2014, 2, 4379 RSC
.
- M. L. Mastronardi, F. Maier-Flaig, D. Faulkner, E. J. Henderson, C. Kübel, U. Lemmer and G. A. Ozin, Nano Lett., 2012, 12, 337 CrossRef CAS PubMed
.
- D. S. English, L. E. Pell, Z. Yu, P. F. Barbara and B. A. Korgel, Nano Lett., 2002, 2, 681 CrossRef CAS
.
- D. A. Ruddy, J. C. Johnson, E. R. Smith and N. R. Neale, ACS Nano, 2010, 4, 7459 CrossRef CAS PubMed
.
- D. C. Lee, J. M. Pietryga, I. Robel, D. J. Werder, R. D. Schaller and V. I. Klimov, J. Am. Chem. Soc., 2009, 131, 3436 CrossRef CAS PubMed
.
- S. P. Hong, H. K. Park, J. H. Oh, H. Yang and Y. R. Do, J. Mater. Chem., 2012, 22, 18939 RSC
.
- J.-Y. Chang, G.-Q. Wang, C.-Y. Cheng, W.-X. Lin and J.-C. Hsu, J. Mater. Chem., 2012, 22, 10609 RSC
.
- Q. A. Akkerman, A. Genovese, C. George, M. Prato, I. Moreels, A. Casu, S. Marras, A. Curcio, A. Scarpellini, T. Pellegrino, L. Manna and V. Lesnyak, ACS Nano, 2014, 9, 521 CrossRef PubMed
.
- J. Zhang, R. Xie and W. Yang, Chem. Mater., 2011, 23, 3357 CrossRef CAS
.
- H. Nakamura, W. Kato, M. Uehara, K. Nose, T. Omata, S. Otsuka-Yao-Matsuo, M. Miyazaki and H. Maeda, Chem. Mater., 2006, 18, 3330 CrossRef CAS
.
- R. Xie, M. Rutherford and X. Peng, J. Am. Chem. Soc., 2009, 131, 5691 CrossRef CAS PubMed
.
- R. Xie, D. Battaglia and X. Peng, J. Am. Chem. Soc., 2007, 129, 15432 CrossRef CAS PubMed
.
- D. V. Talapin, N. Gaponik, H. Borchert, A. L. Rogach, M. Haase and H. Weller, J. Phys. Chem. B, 2002, 106, 12659 CrossRef CAS
.
- S. Xu, J. Ziegler and T. Nann, J. Mater. Chem., 2008, 18, 2653 RSC
.
- J. P. Zimmer, S.-W. Kim, S. Ohnishi, E. Tanaka, J. V. Frangioni and M. G. Bawendi, J. Am. Chem. Soc., 2006, 128, 2526 CrossRef CAS PubMed
.
- A. Aharoni, T. Mokari, I. Popov and U. Banin, J. Am. Chem. Soc., 2006, 128, 257 CrossRef CAS PubMed
.
- M. Yarema and M. V. Kovalenko, Chem. Mater., 2013, 25, 1788 CrossRef CAS
.
- W. Liu, A. Y. Chang, R. D. Schaller and D. V. Talapin, J. Am. Chem. Soc., 2012, 134, 20258 CrossRef CAS PubMed
.
- G. Pellegrini, G. Mattei and P. Mazzoldi, J. Appl. Phys., 2005, 97, 073706 CrossRef PubMed
.
- P. O. Anikeeva, J. E. Halpert, M. G. Bawendi and V. Bulović, Nano Lett., 2009, 9, 2532 CrossRef CAS PubMed
.
- Z. Tan, F. Zhang, T. Zhu, J. Xu, A. Y. Wang, J. D. Dixon, L. Li, Q. Zhang, S. E. Mohney and J. Ruzyllo, Nano Lett., 2007, 7, 3803 CrossRef CAS PubMed
.
- M. J. Fernee, P. Jensen and H. Rubinsztein-Dunlop, J. Phys. Chem. C, 2007, 111, 4984 CAS
.
- M. J. Fernée, E. Thomsen, P. Jensen and H. Rubinsztein-Dunlop, Nanotechnology, 2006, 17, 956 CrossRef PubMed
.
- W. Ma, S. L. Swisher, T. Ewers, J. Engel, V. E. Ferry, H. A. Atwater and A. P. Alivisatos, ACS Nano, 2011, 5, 8140 CrossRef CAS PubMed
.
- S. Keuleyan, E. Lhuillier and P. Guyot-Sionnest, J. Am. Chem. Soc., 2011, 133, 16422 CrossRef CAS PubMed
.
- I. Moreels, Y. Justo, B. De Geyter, K. Haustraete, J. C. Martins and Z. Hens, ACS Nano, 2011, 5, 2004 CrossRef CAS PubMed
.
- F. García-Santamaría, Y. Chen, J. Vela, R. D. Schaller, J. A. Hollingsworth and V. I. Klimov, Nano Lett., 2009, 9, 3482 CrossRef PubMed
.
- S. Christodoulou, G. Vaccaro, V. Pinchetti, F. De Donato, J. Q. Grim, A. Casu, A. Genovese, G. Vicidomini, A. Diaspro, S. Brovelli, L. Manna and I. Moreels, J. Mater. Chem. C, 2014, 2, 3439 RSC
.
- J. M. Pietryga, D. J. Werder, D. J. Williams, J. L. Casson, R. D. Schaller, V. I. Klimov and J. A. Hollingsworth, J. Am. Chem. Soc., 2008, 130, 4879 CrossRef CAS PubMed
.
- C. Ippen, T. Greco, Y. Kim, J. Kim, M. S. Oh, C. J. Han and A. Wedel, Org. Electron., 2014, 15, 126 CrossRef CAS PubMed
.
- W. Ji, P. Jing, W. Xu, X. Yuan, Y. Wang, J. Zhao and A. K.-Y. Jen, Appl. Phys. Lett., 2013, 103, 053106 CrossRef PubMed
.
- G. Jia and U. Banin, J. Am. Chem. Soc., 2014, 136, 11121 CrossRef CAS PubMed
.
- H. Li, M. Zanella, A. Genovese, M. Povia, A. Falqui, C. Giannini and L. Manna, Nano Lett., 2011, 11, 4964 CrossRef CAS PubMed
.
- Y. Li, Y. Ding and Z. Wang, Adv. Mater., 1999, 11, 847 CrossRef CAS
.
- P. D. Cozzoli, L. Manna, M. L. Curri, S. Kudera, C. Giannini, M. Striccoli and A. Agostiano, Chem. Mater., 2005, 17, 1296 CrossRef CAS
.
- H. Park, H. Chung and W. Kim, Mater. Lett., 2013, 99, 172 CrossRef CAS PubMed
.
- Y. Sun, Z. Sun, S. Gao, H. Cheng, Q. Liu, J. Piao, T. Yao, C. Wu, S. Hu, S. Wei and Y. Xie, Nat. Commun., 2012, 3, 1057 CrossRef PubMed
.
- H. Li, R. Brescia, R. Krahne, G. Bertoni, M. J. P. Alcocer, C. D'Andrea, F. Scotognella, F. Tassone, M. Zanella, M. De Giorgi and L. Manna, ACS Nano, 2012, 6, 1637 CrossRef CAS PubMed
.
- C. Bouet, D. Laufer, B. Mahler, B. Nadal, H. Heuclin, S. Pedetti, G. Patriarche and B. Dubertret, Chem. Mater., 2014, 26, 3002 CrossRef CAS
.
- S. Nakamura, T. Mukai and M. Senoh, Appl. Phys. Lett., 1994, 64, 1687 CrossRef CAS PubMed
.
- Z. I. Alferov, V. M. Andreev, D. Z. Garbuzov, Y. V. Zhilyaev, E. P. Morozov, E. L. Portnoi and V. G. Trofim, Semiconductors, 1971, 4, 1573 Search PubMed
.
- M. A. Olshavsky, A. N. Goldstein and A. P. Alivisatos, J. Am. Chem. Soc., 1990, 112, 9438 CrossRef CAS
.
- X. Peng, J. Wickham and A. P. Alivisatos, J. Am. Chem. Soc., 1998, 120, 5343 CrossRef CAS
.
- Y. W. Cao and U. Banin, J. Am. Chem. Soc., 2000, 122, 9692 CrossRef CAS
.
- J. R. Heath, Chem. Soc. Rev., 1998, 27, 65 RSC
.
- D. K. Harris and M. G. Bawendi, J. Am. Chem. Soc., 2012, 134, 20211 CrossRef CAS PubMed
.
- O. I. Micic, C. J. Curtis, K. M. Jones, J. R. Sprague and A. J. Nozik, J. Phys. Chem., 1994, 98, 4966 CrossRef CAS
.
- O. I. Micic, J. R. Sprague, C. J. Curtis, K. M. Jones, J. L. Machol, A. J. Nozik, H. Giessen, B. Fluegel, G. Mohs and N. Peyghambarian, J. Phys. Chem., 1995, 99, 7754 CrossRef CAS
.
- A. A. Guzelian, J. E. B. Katari, A. V. Kadavanich, U. Banin, K. Hamad, E. Juban, A. P. Alivisatos, R. H. Wolters, C. C. Arnold and J. R. Heath, J. Phys. Chem., 1996, 100, 7212 CrossRef CAS
.
- D. Battaglia and X. Peng, Nano Lett., 2002, 2, 1027 CrossRef CAS
.
- X. Yang, D. Zhao, K. S. Leck, S. T. Tan, Y. X. Tang, J. Zhao, H. V. Demir and X. W. Sun, Adv. Mater., 2012, 24, 4180 CrossRef CAS PubMed
.
- J. Lauth, T. Strupeit, A. Kornowski and H. Weller, Chem. Mater., 2013, 25, 1377 CrossRef CAS
.
- S. Kan, A. Aharoni, T. Mokari and U. Banin, Faraday Discuss., 2004, 125, 23 RSC
.
- S. P. Ahrenkiel, O. I. Mićić, A. Miedaner, C. J. Curtis, J. M. Nedeljković and A. J. Nozik, Nano Lett., 2003, 3, 833 CrossRef CAS
.
- P. K. Jain, B. J. Beberwyck, L.-K. Fong, M. J. Polking and A. P. Alivisatos, Angew. Chem., 2012, 124, 2437 CrossRef PubMed
.
- H. Li, R. Brescia, M. Povia, M. Prato, G. Bertoni, L. Manna and I. Moreels, J. Am. Chem. Soc., 2013, 135, 12270 CrossRef CAS PubMed
.
- J.-H. Kim and H. Yang, Opt. Lett., 2014, 39, 5002 CrossRef PubMed
.
- W. Li, Z. Pan and X. Zhong, J. Mater. Chem. A, 2014, 3, 1649 Search PubMed
.
- D. Aldakov, A. Lefrançois and P. Reiss, J. Mater. Chem. C, 2013, 1, 3756 RSC
.
- L. Li, A. Pandey, D. J. Werder, B. P. Khanal, J. M. Pietryga and V. I. Klimov, J. Am. Chem. Soc., 2011, 133, 1176 CrossRef CAS PubMed
.
- L. De Trizio, M. Prato, A. Genovese, A. Casu, M. Povia, R. Simonutti, M. J. P. Alcocer, C. D'Andrea, F. Tassone and L. Manna, Chem. Mater., 2012, 24, 2400 CrossRef CAS
.
- J. Park and S.-W. Kim, J. Mater. Chem., 2011, 21, 3745 RSC
.
- M. B. Sigman, A. Ghezelbash, T. Hanrath, A. E. Saunders, F. Lee and B. A. Korgel, J. Am. Chem. Soc., 2003, 125, 16050 CrossRef CAS PubMed
.
- Z. Zhuang, Q. Peng, B. Zhang and Y. Li, J. Am. Chem. Soc., 2008, 130, 10482 CrossRef CAS PubMed
.
- W. Bryks, M. Wette, N. Velez, S.-W. Hsu and A. R. Tao, J. Am. Chem. Soc., 2014, 136, 6175 CrossRef CAS PubMed
.
- T. Todorov, O. Gunawan, S. J. Chey, T. G. de Monsabert, A. Prabhakar and D. B. Mitzi, Thin Solid Films, 2011, 519, 7378 CrossRef CAS PubMed
.
-
http://minerals.usgs.gov/minerals/pubs/commodity/indium/indiumcs04.pdf
.
- M. J. Anc, N. L. Pickett, N. C. Gresty, J. A. Harris and K. C. Mishra, ECS J. Solid State Sci. Technol., 2012, 2, R3071 CrossRef PubMed
.
- W. L. Wilson, P. F. Szajowski and L. E. Brus, Science, 1993, 262, 1242 CAS
.
- B. Delley and E. Steigmeier, Phys. Rev. B: Condens. Matter Mater. Phys., 1993, 47, 1397 CrossRef CAS
.
- J. Wilcoxon, G. Samara and P. Provencio, Phys. Rev. B: Condens. Matter Mater. Phys., 1999, 60, 2704 CrossRef CAS
.
- J. R. Heath, Science, 1992, 258, 1131 CAS
.
- R. D. Tilley and K. Yamamoto, Adv. Mater., 2006, 18, 2053 CrossRef CAS PubMed
.
- Q. Li, Y. He, J. Chang, L. Wang, H. Chen, Y.-W. Tan, H. Wang and Z. Shao, J. Am. Chem. Soc., 2013, 135, 14924 CrossRef CAS PubMed
.
- J. L. Heinrich, C. L. Curtis, G. M. Credo, M. J. Sailor and K. L. Kavanagh, Science, 1992, 255, 66 CAS
.
- S. W. Kim, J. Lee, J. H. Sung, D. Seo, I. Kim, M.-H. Jo, B. W. Kwon, W. K. Choi and H.-J. Choi, ACS Nano, 2014, 8, 6556 CrossRef CAS PubMed
.
- L. T. Canham, Appl. Phys. Lett., 1990, 57, 1046 CrossRef CAS PubMed
.
- Y. Maeda, Phys. Rev. B: Condens. Matter Mater. Phys., 1995, 51, 1658 CrossRef CAS
.
- D. Gerion, N. Zaitseva, C. Saw, M. F. Casula, S. Fakra, T. Van Buuren and G. Galli, Nano Lett., 2004, 4, 597 CrossRef CAS
.
- N. Zaitseva, Z. R. Dai, C. D. Grant, J. Harper and C. Saw, Chem. Mater., 2007, 19, 5174 CrossRef CAS
.
- Y. Guo, C. E. Rowland, R. D. Schaller and J. Vela, ACS Nano, 2014, 8, 8334 CrossRef CAS PubMed
.
- L. J. Hope-Weeks, Chem. Commun., 2003, 2980 RSC
.
- J. H. Warner and R. D. Tilley, Nanotechnology, 2006, 17, 3745 CrossRef CAS
.
- N. H. Chou, K. D. Oyler, N. E. Motl and R. E. Schaak, Chem. Mater., 2009, 21, 4105 CrossRef CAS
.
- T. J. Boyle, L. J. Tribby, L. A. M. Ottley and S. M. Han, Eur. J. Inorg. Chem., 2009, 5550 CAS
.
- A. O'Hare, F. V. Kusmartsev and K. I. Kugel, Nano Lett., 2012, 12, 1045 CrossRef PubMed
.
- Z. Ni, Q. Liu, K. Tang, J. Zheng, J. Zhou, R. Qin, Z. Gao, D. Yu and J. Lu, Nano Lett., 2012, 12, 113 CrossRef CAS PubMed
.
- R. Haitz and J. Y. Tsao, Phys. Status Solidi, 2011, 208, 17 CrossRef CAS PubMed
.
- S. Reineke, M. Thomschke, B. Lüssem and K. Leo, Rev. Mod. Phys., 2013, 85, 1245 CrossRef CAS
.
- Y. Narukawa, M. Sano, T. Sakamoto, T. Yamada and T. Mukai, Phys. Status Solidi, 2008, 205, 1081 CrossRef CAS PubMed
.
- Y. Narukawa, J. Narita, T. Sakamoto, K. Deguchi, T. Yamada and T. Mukai, Jpn. J. Appl. Phys., 2006, 45, L1084 CrossRef CAS
.
- X. Dai, Z. Zhang, Y. Jin, Y. Niu, H. Cao, X. Liang, L. Chen, J. Wang and X. Peng, Nature, 2014, 515, 96 CrossRef CAS PubMed
.
- C. B. Murray, M. Nirmal, D. J. Norris and M. G. Bawendi, Z. Phys. D: At., Mol. Clusters, 1993, 26, 231 CrossRef CAS
.
- Q. Sun, Y. A. Wang, L. S. Li, D. Wang, T. Zhu, J. Xu, C. Yang and Y. Li, Nat. Photonics, 2007, 1, 717 CrossRef CAS PubMed
.
- K.-S. Cho, E. K. Lee, W.-J. Joo, E. Jang, T.-H. Kim, S. J. Lee, S.-J. Kwon, J. Y. Han, B.-K. Kim, B. L. Choi and J. M. Kim, Nat. Photonics, 2009, 3, 341 CrossRef CAS PubMed
.
- B. S. Mashford, M. Stevenson, Z. Popovic, C. Hamilton, Z. Zhou, C. Breen, J. Steckel, V. Bulovic, M. Bawendi, S. Coe-Sullivan and P. T. Kazlas, Nat. Photonics, 2013, 7, 407 CrossRef CAS PubMed
.
- L. Sun, J. J. Choi, D. Stachnik, A. C. Bartnik, B.-R. Hyun, G. G. Malliaras, T. Hanrath and F. W. Wise, Nat. Nanotechnol., 2012, 7, 369 CrossRef CAS PubMed
.
- S. Coe, W.-K. Woo, M. Bawendi and V. Bulović, Nature, 2002, 420, 800 CrossRef CAS PubMed
.
- A. H. Mueller, M. A. Petruska, M. Achermann, D. J. Werder, E. A. Akhadov, D. D. Koleske, M. A. Hoffbauer and V. I. Klimov, Nano Lett., 2005, 5, 1039 CrossRef CAS PubMed
.
- K.-H. Lee, J.-H. Lee, W.-S. Song, H. Ko, C. Lee, J.-H. Lee and H. Yang, ACS Nano, 2013, 7, 7295 CrossRef CAS PubMed
.
- B. O. Dabbousi, M. G. Bawendi, O. Onitsuka and M. F. Rubner, Appl. Phys. Lett., 1995, 66, 1316 CrossRef CAS PubMed
.
- V. Wood, M. J. Panzer, J. E. Halpert, J.-M. Caruge, M. G. Bawendi and V. Bulović, ACS Nano, 2009, 3, 3581 CrossRef CAS PubMed
.
- Y. Li, A. Rizzo, M. Mazzeo, L. Carbone, L. Manna, R. Cingolani and G. Gigli, J. Appl. Phys., 2005, 97, 113501 CrossRef PubMed
.
- L. Qian, Y. Zheng, J. Xue and P. H. Holloway, Nat. Photonics, 2011, 5, 543 CrossRef CAS PubMed
.
- B. S. Mashford, T.-L. Nguyen, G. J. Wilson and P. Mulvaney, J. Mater. Chem., 2010, 20, 167 RSC
.
- L. Qian, Y. Zheng, K. R. Choudhury, D. Bera, F. So, J. Xue and P. H. Holloway, Nano Today, 2010, 5, 384 CrossRef CAS PubMed
.
- R. Meerheim, S. Scholz, S. Olthof, G. Schwartz, S. Reineke, K. Walzer and K. Leo, J. Appl. Phys., 2008, 104, 014510 CrossRef PubMed
.
- J. Lim, M. Park, W. K. Bae, D. Lee, S. Lee, C. Lee and K. Char, ACS Nano, 2013, 7, 9019 CrossRef CAS PubMed
.
- S. Kim, T. Kim, M. Kang, S. K. Kwak, T. W. Yoo, L. S. Park, I. Yang, S. Hwang, J. E. Lee, S. K. Kim and S.-W. Kim, J. Am. Chem. Soc., 2012, 134, 3804 CrossRef CAS PubMed
.
-
Y. Kim, T. Greco, C. Ippen, A. Wedel and J. Kim, 2013 IEEE 5th Int. Nanoelectron. Conf., 2013, 425.
- J. Lim, W. K. Bae, D. Lee, M. K. Nam, J. Jung, C. Lee, K. Char and S. Lee, Chem. Mater., 2011, 23, 4459 CrossRef CAS
.
- U. T. D. Thuy, P. Reiss and N. Q. Liem, Appl. Phys. Lett., 2010, 97, 193104 CrossRef PubMed
.
- A. Wang, H. Shen, S. Zang, Q. Lin, H. Wang, Q. Lei, J. Niu and L. S. Li, Nanoscale, 2015, 7, 2951 RSC
.
- D. I. Son, B. W. Kwon, D. H. Park, W.-S. Seo, Y. Yi, B. Angadi, C.-L. Lee and W. K. Choi, Nat. Nanotechnol., 2012, 7, 465 CrossRef CAS PubMed
.
- F. Maier-Flaig, J. Rinck, M. Stephan, T. Bocksrocker, M. Bruns, C. Kübel, A. K. Powell, G. A. Ozin and U. Lemmer, Nano Lett., 2013, 13, 475 CrossRef CAS PubMed
.
- B. Chen, H. Zhong, W. Zhang, Z. Tan, Y. Li, C. Yu, T. Zhai, Y. Bando, S. Yang and B. Zou, Adv. Funct. Mater., 2012, 22, 2081 CrossRef CAS PubMed
.
- Z. Tan, Y. Zhang, C. Xie, H. Su, J. Liu, C. Zhang, N. Dellas, S. E. Mohney, Y. Wang, J. Wang and J. Xu, Adv. Mater., 2011, 23, 3553 CrossRef CAS PubMed
.
- K. Nose, N. Fujita, T. Omata, S. Otsuka-Yao-Matsuo, W. Kato, M. Uehara, H. Nakamura, H. Maeda, H. Kamioka and H. Hosono, J. Phys.: Conf. Ser., 2009, 165, 012028 CrossRef
.
- D. Jurbergs, E. Rogojina, L. Mangolini and U. Kortshagen, Appl. Phys. Lett., 2006, 88, 233116 CrossRef PubMed
.
- F. Priolo, T. Gregorkiewicz, M. Galli and T. F. Krauss, Nat. Nanotechnol., 2014, 9, 19 CrossRef CAS PubMed
.
- Q. Qiao, B. H. Li, C. X. Shan, J. S. Liu, J. Yu, X. H. Xie, Z. Z. Zhang, T. B. Ji, Y. Jia and D. Z. Shen, Mater. Lett., 2012, 74, 104 CrossRef CAS PubMed
.
- J. Chen, J. Pan, D. Qingguo, G. Alagappan, W. Lei, Q. Li and J. Xia, Appl. Phys. A: Mater. Sci. Process., 2014, 117, 589 CrossRef CAS
.
- W. Ji, P. Jing, Y. Fan, J. Zhao, Y. Wang and X. Kong, Opt. Lett., 2013, 38, 7 CrossRef CAS PubMed
.
- K. A. Mazzio and C. K. Luscombe, Chem. Soc. Rev., 2014, 44, 78 RSC
.
- S. A. McDonald, G. Konstantatos, S. Zhang, P. W. Cyr, E. J. D. Klem, L. Levina and E. H. Sargent, Nat. Mater., 2005, 4, 138 CrossRef CAS PubMed
.
- A. J. Labelle, S. M. Thon, S. Masala, M. M. Adachi, H. Dong, M. Farahani, A. H. Ip, A. Fratalocchi and E. H. Sargent, Nano Lett., 2014, 15, 1101 CrossRef PubMed
.
- Y. Cao, M. S. Denny, J. V. Caspar, W. E. Farneth, Q. Guo, A. S. Ionkin, L. K. Johnson, M. Lu, I. Malajovich, D. Radu, H. D. Rosenfeld, K. R. Choudhury and W. Wu, J. Am. Chem. Soc., 2012, 134, 15644 CrossRef CAS PubMed
.
- Q. Guo, S. J. Kim, M. Kar, W. N. Shafarman, R. W. Birkmire, E. A. Stach, R. Agrawal and H. W. Hillhouse, Nano Lett., 2008, 8, 2982 CrossRef CAS PubMed
.
- C. Steinhagen, V. A. Akhavan, B. W. Goodfellow, M. G. Panthani, J. T. Harris, V. C. Holmberg and B. A. Korgel, ACS Appl. Mater. Interfaces, 2011, 3, 1781 CAS
.
- A. de Kergommeaux, A. Fiore, J. Faure-Vincent, F. Chandezon, A. Pron, R. de Bettignies and P. Reiss, Mater. Chem. Phys., 2012, 136, 877 CrossRef CAS PubMed
.
- D. S. Dolzhnikov, H. Zhang, J. Jang, J. S. Son, M. G. Panthani, T. Shibata, S. Chattopadhyay and D. V. Talapin, Science, 2015, 347, 425 CrossRef CAS PubMed
.
- H. Zhou, Q. Chen, G. Li, S. Luo, T.-b. Song, H.-S. Duan, Z. Hong, J. You, Y. Liu and Y. Yang, Science, 2014, 345, 542 CrossRef CAS PubMed
.
- F. Hao, C. C. Stoumpos, D. H. Cao, R. P. H. Chang and M. G. Kanatzidis, Nat. Photonics, 2014, 8, 489 CrossRef CAS PubMed
.
- N. K. Noel, S. D. Stranks, A. Abate, C. Wehrenfennig, S. Guarnera, A. Haghighirad, A. Sadhanala, G. E. Eperon, S. K. Pathak, M. B. Johnston, A. Petrozza, L. Herz and H. Snaith, Energy Environ. Sci., 2014, 7, 3061 CAS
.
- NREL: National Center for Photovoltaics Home Page: http://www.nrel.gov/ncpv/, 2015.
- W. Shockley and H. J. Queisser, J. Appl. Phys., 1961, 32, 510 CrossRef CAS PubMed
.
- A. J. Nozik, Nano Lett., 2010, 10, 2735 CrossRef CAS PubMed
.
- X. Cheng, S. B. Lowe, P. J. Reece and J. J. Gooding, Chem. Soc. Rev., 2014, 43, 2680 RSC
.
- S.-W. Kim, J. P. Zimmer, S. Ohnishi, J. B. Tracy, J. V. Frangioni and M. G. Bawendi, J. Am. Chem. Soc., 2005, 127, 10526 CrossRef CAS PubMed
.
- L. Li, T. J. Daou, I. Texier, T. T. Kim Chi, N. Q. Liem and P. Reiss, Chem. Mater., 2009, 21, 2422 CrossRef CAS
.
- M. J. Currie, J. K. Mapel, T. D. Heidel, S. Goffri and M. A. Baldo, Science, 2008, 321, 226 CrossRef CAS PubMed
.
- F. Purcell-Milton and Y. K. Gun'ko, J. Mater. Chem., 2012, 22, 16687 RSC
.
- F. Meinardi, A. Colombo, K. A. Velizhanin, R. Simonutti, M. Lorenzon, L. Beverina, R. Viswanatha, V. I. Klimov and S. Brovelli, Nat. Photonics, 2014, 8, 392 CrossRef CAS PubMed
.
- J. Bomm, A. Büchtemann, A. Fiore, L. Manna, J. H. Nelson, D. Hill and W. G. J. H. M. van Sark, Beilstein J. Nanotechnol., 2010, 1, 94 CrossRef CAS PubMed
.
- D. Chemisana, Renewable Sustainable Energy Rev., 2011, 15, 603 CrossRef PubMed
.
- C. S. Erickson, L. R. Bradshaw, S. McDowall, J. D. Gilbertson, D. R. Gamelin and D. L. Patrick, ACS Nano, 2014, 8, 3461 CrossRef CAS PubMed
.
- L. R. Bradshaw, K. Knowles, S. McDowall and D. R. Gamelin, Nano Lett., 2015, 1315 CrossRef CAS PubMed
.
- I. Coropceanu and M. G. Bawendi, Nano Lett., 2014, 14, 4097 CrossRef CAS PubMed
.
- S. C. Erwin, L. Zu, M. I. Haftel, A. L. Efros, T. A. Kennedy and D. J. Norris, Nature, 2005, 436, 91 CrossRef CAS PubMed
.
- R. N. Bhargava, D. Gallagher, X. Hong and A. Nurmikko, Phys. Rev. Lett., 1994, 72, 416 CrossRef CAS
.
- J. F. Suyver, S. F. Wuister, J. J. Kelly and A. Meijerink, Nano Lett., 2001, 1, 429 CrossRef CAS
.
- R. Xie and X. Peng, J. Am. Chem. Soc., 2009, 131, 10645 CrossRef CAS PubMed
.
- B. Ghosh, Y. Masuda, Y. Wakayama, Y. Imanaka, J. Inoue, K. Hashi, K. Deguchi, H. Yamada, Y. Sakka, S. Ohki, T. Shimizu and N. Shirahata, Adv. Funct. Mater., 2014, 24, 7151 CAS
.
- P. Principi and R. Fioretti, J. Cleaner Prod., 2014, 83, 96 CrossRef PubMed
.
- Z. Zhang, D. Liu, D. Li, K. Huang, Y. Zhang, Z. Shi, R. Xie, M.-Y. Han, Y. Wang and W. Yang, Chem. Mater., 2015, 27, 1405 CrossRef CAS
.
- E. Mutlugun, P. L. Hernandez-Martinez, C. Eroglu, Y. Coskun, T. Erdem, V. K. Sharma, E. Unal, S. K. Panda, S. G. Hickey, N. Gaponik, A. Eychmüller and H. V. Demir, Nano Lett., 2012, 12, 3986 CrossRef CAS PubMed
.
- B. Huang, Q. Dai, N. Zhuo, Q. Jiang, F. Shi, H. Wang, H. Zhang, C. Liao, Y. Cui and J. Zhang, J. Appl. Phys., 2014, 116, 094303 CrossRef PubMed
.
-
M. Arik, Fourteenth Intersoc. Conf. Therm. Thermomechanical Phenom. Electron. Syst., 2014, 260.
- A. Narayanaswamy, L. F. Feiner and P. J. VanderZaag, J. Phys. Chem. C, 2008, 112, 6775 CAS
.
- C. E. Rowland, W. Liu, D. C. Hannah, M. K. Y. Chan, D. V. Talapin and R. D. Schaller, ACS Nano, 2014, 8, 977 CrossRef CAS PubMed
.
- A. Narayanaswamy, L. F. Feiner, A. Meijerink and P. J. van der Zaag, ACS Nano, 2009, 3, 2539 CrossRef CAS PubMed
.
- C. Grivas, C. Li, P. Andreakou, P. Wang, M. Ding, G. Brambilla, L. Manna and P. Lagoudakis, Nat. Commun., 2013, 4, 2376 Search PubMed
.
- S. Gao, C. Zhang, Y. Liu, H. Su, L. Wei, T. Huang, N. Dellas, S. Shang, S. E. Mohney, J. Wang and J. Xu, Opt. Express, 2011, 19, 5528 CrossRef CAS PubMed
.
- B. Guilhabert, C. Foucher, A.-M. Haughey, E. Mutlugun, Y. Gao, J. Herrnsdorf, H. D. Sun, H. V. Demir, M. D. Dawson and N. Laurand, Opt. Express, 2014, 22, 7308 CrossRef CAS PubMed
.
- F. Di Stasio, J. Q. Grim, V. Lesnyak, P. Rastogi, L. Manna, I. Moreels and R. Krahne, Small, 2015, 11, 1328 CrossRef CAS PubMed
.
- M. Kazes, D. Y. Lewis, Y. Ebenstein, T. Mokari and U. Banin, Adv. Mater., 2002, 14, 317 CrossRef CAS
.
- I. Moreels, G. Rainò, R. Gomes, Z. Hens, T. Stöferle and R. F. Mahrt, Adv. Mater., 2012, 24, OP231 CrossRef CAS PubMed
.
- S. Hoogland, V. Sukhovatkin, I. Howard, S. Cauchi, L. Levina and E. H. Sargent, Opt. Express, 2006, 14, 3273 CrossRef CAS
.
- V. I. Klimov, A. A. Mikhailovsky, D. W. McBranch, C. A. Leatherdale and M. G. Bawendi, Science, 2000, 287, 1011 CrossRef CAS
.
- V. I. Klimov, S. A. Ivanov, J. Nanda, M. Achermann, I. Bezel, J. A. McGuire and A. Piryatinski, Nature, 2007, 447, 441 CrossRef CAS PubMed
.
- J. I. Climente, J. L. Movilla and J. Planelles, Small, 2012, 8, 754 CrossRef CAS PubMed
.
- W. K. Bae, L. A. Padilha, Y.-S. Park, H. McDaniel, I. Robel, J. M. Pietryga and V. I. Klimov, ACS Nano, 2013, 7, 3411 CrossRef CAS PubMed
.
- Y. Wang, S. Yang, H. Yang and H. Sun, Adv. Opt. Mater., 2015, 3, 652 CrossRef CAS PubMed
.
- Y. Wang, K. S. Leck, V. D. Ta, R. Chen, V. Nalla, Y. Gao, T. He, H. V. Demir and H. Sun, Adv. Mater., 2015, 27, 169 CrossRef CAS PubMed
.
- C. She, I. Fedin, D. S. Dolzhnikov, A. Demortière, R. D. Schaller, M. Pelton and D. V. Talapin, Nano Lett., 2014, 14, 2772 CrossRef CAS PubMed
.
- V. M. Menon, M. Luberto, N. V. Valappil and S. Chatterjee, Opt. Express, 2008, 16, 19535 CrossRef CAS
.
- V. Sukhovatkin, S. Musikhin, I. Gorelikov, S. Cauchi, L. Bakueva, E. Kumacheva and E. H. Sargent, Opt. Lett., 2005, 30, 171 CrossRef CAS
.
- F. García-Santamaría, Y. Chen, J. Vela, R. D. Schaller, J. A. Hollingsworth and V. I. Klimov, Nano Lett., 2009, 9, 3482 CrossRef PubMed
.
- A. M. Dennis, B. D. Mangum, A. Piryatinski, Y.-S. Park, D. C. Hannah, J. L. Casson, D. J. Williams, R. D. Schaller, H. Htoon and J. A. Hollingsworth, Nano Lett., 2012, 12, 5545 CrossRef CAS PubMed
.
- A. Efros, M. Rosen, M. Kuno, M. Nirmal, D. Norris and M. Bawendi, Phys. Rev. B: Condens. Matter Mater. Phys., 1996, 54, 4843 CrossRef CAS
.
- A. Zunger, J. Phys. Chem. B, 1998, 102, 6449 CrossRef
.
- A. Franceschetti and A. Zunger, Phys. Rev. B: Condens. Matter Mater. Phys., 2000, 62, R16287 CrossRef CAS
.
- G. Bester, J. Phys.: Condens. Matter, 2009, 21, 023202 CrossRef PubMed
.
- M. Califano, A. Franceschetti and A. Zunger, Nano Lett., 2005, 5, 2360 CrossRef CAS PubMed
.
- M. J. Fernée, B. N. Littleton and H. Rubinsztein-Dunlop, ACS Nano, 2009, 3, 3762 CrossRef PubMed
.
- G. Rainò, T. Stöferle, I. Moreels, R. Gomes, Z. Hens and R. F. Mahrt, ACS Nano, 2012, 6, 1979 CrossRef PubMed
.
- C. Sinito, M. J. Fernée, S. V. Goupalov, P. Mulvaney, P. Tamarat and B. Lounis, ACS Nano, 2014, 8, 11651 CrossRef CAS PubMed
.
- O. Labeau, P. Tamarat and B. Lounis, Phys. Rev. Lett., 2003, 90, 257404 CrossRef
.
- N. Le Thomas, E. Herz, O. Schöps, U. Woggon and M. V. Artemyev, Phys. Rev. Lett., 2005, 94, 016803 CrossRef CAS
.
- S. Brovelli, R. D. Schaller, S. A. Crooker, F. García-Santamaría, Y. Chen, R. Viswanatha, J. A. Hollingsworth, H. Htoon and V. I. Klimov, Nat. Commun., 2011, 2, 280 CrossRef CAS PubMed
.
- P. Michler, A. Imamoglu, M. Mason, P. Carson, G. Strouse and S. Buratto, Nature, 2000, 406, 968 CrossRef CAS PubMed
.
- B. Lounis, H. A. Bechtel, D. Gerion, P. Alivisatos and W. E. Moerner, Chem. Phys. Lett., 2000, 329, 399 CrossRef CAS
.
- F. Pisanello, G. Leménager, L. Martiradonna, L. Carbone, S. Vezzoli, P. Desfonds, P. D. Cozzoli, J.-P. Hermier, E. Giacobino, R. Cingolani, M. De Vittorio and A. Bramati, Adv. Mater., 2013, 25, 1974 CrossRef CAS PubMed
.
- H. Kimble, M. Dagenais and L. Mandel, Phys. Rev. Lett., 1977, 39, 691 CrossRef CAS
.
- B. Lounis and W. E. Moerner, Nature, 2000, 407, 491 CrossRef CAS PubMed
.
- J. Hu, L. Ls, W. Yang, L. Manna, W. Lw and A. P. Alivisatos, Science, 2001, 292, 2060 CrossRef CAS PubMed
.
- B. Mahler, P. Spinicelli, S. Buil, X. Quelin, J.-P. Hermier and B. Dubertret, Nat. Mater., 2008, 7, 659 CrossRef CAS PubMed
.
- Y. Chen, J. Vela, H. Htoon, J. L. Casson, D. J. Werder, D. A. Bussian, V. I. Klimov and J. A. Hollingsworth, J. Am. Chem. Soc., 2008, 130, 5026 CrossRef CAS PubMed
.
- B. Ji, E. Giovanelli, B. Habert, P. Spinicelli, M. Nasilowski, X. Xu, N. Lequeux, J.-P. Hugonin, F. Marquier, J.-J. Greffet and B. Dubertret, Nat. Nanotechnol., 2015, 10, 170 CrossRef CAS PubMed
.
- R. Shirazi, O. Kopylov, A. Kovacs and B. E. Kardynał, Appl. Phys. Lett., 2012, 101, 091910 CrossRef PubMed
.
- J. Eilers, J. van Hest, A. Meijerink and C. de M. Donega, J. Phys. Chem. C, 2014, 118, 23313 CAS
.
- B. Julsgaard, Y.-W. Lu, P. Balling and A. N. Larsen, Appl. Phys. Lett., 2009, 95, 183107 CrossRef PubMed
.
- J. Luo, A. Franceschetti and A. Zunger, Phys. Rev. B: Condens. Matter Mater. Phys., 2009, 79, 201301 CrossRef
.
- J.-W. Luo, A. Franceschetti and A. Zunger, Nano Lett., 2009, 9, 2648 CrossRef CAS PubMed
.
- M. Kuno, D. P. Fromm, A. Gallagher, D. J. Nesbitt, O. I. Micic and A. J. Nozik, Nano Lett., 2001, 1, 557 CrossRef CAS
.
- F. Zan, C. Dong, H. Liu and J. Ren, J. Phys. Chem. C, 2012, 116, 3944 CAS
.
- T. S. Bischof, R. E. Correa, D. Rosenberg, E. A. Dauler and M. G. Bawendi, Nano Lett., 2014, 14, 6787 CrossRef CAS PubMed
.
- L. T. Kunneman, M. D. Tessier, H. Heuclin, B. Dubertret, Y. V. Aulin, F. C. Grozema, J. M. Schins and L. D. A. Siebbeles, J. Phys. Chem. Lett., 2013, 4, 3574 CrossRef CAS
.
- M. Wiesner, W.-M. Schulz, C. Kessler, M. Reischle, S. Metzner, F. Bertram, J. Christen, R. Roßbach, M. Jetter and P. Michler, Nanotechnology, 2012, 23, 335201 CrossRef CAS PubMed
.
- M. A. M. Versteegh, M. E. Reimer, K. D. Jöns, D. Dalacu, P. J. Poole, A. Gulinatti, A. Giudice and V. Zwiller, Nat. Commun., 2014, 5, 5298 CrossRef CAS PubMed
.
- T. Huber, A. Predojević, M. Khoshnegar, D. Dalacu, P. J. Poole, H. Majedi and G. Weihs, Nano Lett., 2014, 14, 7107 CrossRef CAS PubMed
.
- D. D. Vaughn and R. E. Schaak, Chem. Soc. Rev., 2013, 42, 2861 RSC
.
- G. Yi, B. Sun, F. Yang, D. Chen, Y. Zhou and J. Cheng, Chem. Mater., 2002, 14, 2910 CrossRef CAS
.
- K. Kömpe, H. Borchert, J. Storz, A. Lobo, S. Adam, T. Möller and M. Haase, Angew. Chem., Int. Ed., 2003, 42, 5513 CrossRef PubMed
.
- H. Meyssamy, K. Riwotzki, A. Kornowski, S. Naused and M. Haase, Adv. Mater., 1999, 11, 840 CrossRef CAS
.
- K. Riwotzki and M. Haase, J. Phys. Chem. B, 1998, 102, 10129 CrossRef CAS
.
- A. Huignard, T. Gacoin and J.-P. Boilot, Chem. Mater., 2000, 12, 1090 CrossRef CAS
.
- L. Protesescu, S. Yakunin, M. I. Bodnarchuk, F. Krieg, R. Caputo, C. H. Hendon, R. X. Yang, A. Walsh and M. V. Kovalenko, Nano Lett., 2015, 15, 3692 CrossRef CAS PubMed
.
- D. Di, K. P. Musselman, G. Li, A. Sadhanala, Y. Ievskaya, Q. Song, Z.-K. Tan, M. L. Lai, J. L. MacManus-Driscoll, N. C. Greenham and R. H. Friend, J. Phys. Chem. Lett., 2015, 6, 446 CrossRef CAS PubMed
.
- Z. Chen, H. Li, Y. Tang, X. Huang, D. Ho and C.-S. Lee, Mater. Res. Express, 2014, 1, 015034 CrossRef PubMed
.
- F. Zhu, L. Men, Y. Guo, Q. Zhu, U. Bhattacharjee, P. M. Goodwin, J. W. Petrich, E. A. Smith and J. Vela, ACS Nano, 2015, 9, 2948 CrossRef CAS PubMed
.
- Y. Gong, J. Lin, X. Wang, G. Shi, S. Lei, Z. Lin, X. Zou, G. Ye, R. Vajtai, B. I. Yakobson, H. Terrones, M. Terrones, B. K. Tay, J. Lou, S. T. Pantelides, Z. Liu, W. Zhou and P. M. Ajayan, Nat. Mater., 2014, 13, 1135 CrossRef CAS PubMed
.
- R. Cheng, D. Li, H. Zhou, C. Wang, A. Yin, S. Jiang, Y. Liu, Y. Chen, Y. Huang and X. Duan, Nano Lett., 2014, 14, 5590 CrossRef CAS PubMed
.
- J. S. Ross, P. Klement, A. M. Jones, N. J. Ghimire, J. Yan, D. G. Mandrus, T. Taniguchi, K. Watanabe, K. Kitamura, W. Yao, D. H. Cobden and X. Xu, Nat. Nanotechnol., 2014, 9, 268 CrossRef CAS PubMed
.
- A. Splendiani, L. Sun, Y. Zhang, T. Li, J. Kim, C.-Y. Chim, G. Galli and F. Wang, Nano Lett., 2010, 10, 1271 CrossRef CAS PubMed
.
- K. F. Mak, C. Lee, J. Hone, J. Shan and T. F. Heinz, Phys. Rev. Lett., 2010, 105, 136805 CrossRef
.
- F. Wang, Y. Han, C. S. Lim, Y. Lu, J. Wang, J. Xu, H. Chen, C. Zhang, M. Hong and X. Liu, Nature, 2010, 463, 1061 CrossRef CAS PubMed
.
- J. F. Suyver, A. Aebischer, D. Biner, P. Gerner, J. Grimm, S. Heer, K. W. Krämer, C. Reinhard and H. U. Güdel, Opt. Mater., 2005, 27, 1111 CrossRef CAS PubMed
.
- D. J. Gargas, E. M. Chan, A. D. Ostrowski, S. Aloni, M. V. P. Altoe, E. S. Barnard, B. Sanii, J. J. Urban, D. J. Milliron, B. E. Cohen and P. J. Schuck, Nat. Nanotechnol., 2014, 9, 300 CrossRef CAS PubMed
.
- H. Zhu, X. Chen, L. M. Jin, Q. J. Wang, F. Wang and S. F. Yu, ACS Nano, 2013, 7, 11420 CrossRef CAS PubMed
.
- J. Luo, J.-H. Im, M. T. Mayer, M. Schreier, M. K. Nazeeruddin, N.-G. Park, S. D. Tilley, H. J. Fan and M. Gratzel, Science, 2014, 345, 1593 CrossRef CAS PubMed
.
- L. Gil-Escrig, G. Longo, A. Pertegás, C. Roldan, A. Soriano Portillo, M. Sessolo and H. J. Bolink, Chem. Commun., 2014, 51, 569 RSC
.
- O. Malinkiewicz, A. Yella, Y. H. Lee, G. M. Espallargas, M. Graetzel, M. K. Nazeeruddin and H. J. Bolink, Nat. Photonics, 2013, 8, 128 CrossRef PubMed
.
- A. Kuc, N. Zibouche and T. Heine, Phys. Rev. B: Condens. Matter Mater. Phys., 2011, 83, 245213 CrossRef
.
- M. M. Ugeda, A. J. Bradley, S.-F. Shi, F. H. da Jornada, Y. Zhang, D. Y. Qiu, W. Ruan, S.-K. Mo, Z. Hussain, Z.-X. Shen, F. Wang, S. G. Louie and M. F. Crommie, Nat. Mater., 2014, 13, 1091 CrossRef CAS PubMed
.
- Y.-H. Lee, X.-Q. Zhang, W. Zhang, M.-T. Chang, C.-T. Lin, K.-D. Chang, Y.-C. Yu, J. T.-W. Wang, C.-S. Chang, L.-J. Li and T.-W. Lin, Adv. Mater., 2012, 24, 2320 CrossRef CAS PubMed
.
- J. Jang, S. Jeong, J. Seo, M.-C. Kim, E. Sim, Y. Oh, S. Nam, B. Park and J. Cheon, J. Am. Chem. Soc., 2011, 133, 7636 CrossRef CAS PubMed
.
- S. Jeong, D. Yoo, J. Jang, M. Kim and J. Cheon, J. Am. Chem. Soc., 2012, 134, 18233 CrossRef CAS PubMed
.
- R. Lv, J. A. Robinson, R. E. Schaak, D. Sun, Y. Sun, T. E. Mallouk and M. Terrones, Acc. Chem. Res., 2014, 48, 56 CrossRef PubMed
.
|
This journal is © The Royal Society of Chemistry 2015 |
Click here to see how this site uses Cookies. View our privacy policy here.