DOI:
10.1039/D1AN00255D
(Paper)
Analyst, 2021,
146, 3127-3136
Rapid, in situ detection of chemical warfare agent simulants and hydrolysis products in bulk soils by low-cost 3D-printed cone spray ionization mass spectrometry†
Received
9th February 2021
, Accepted 17th March 2021
First published on 18th March 2021
Abstract
Chemical warfare agents (CWAs) are toxic chemicals that have been used as disabling or lethal weapons in war, terrorist attacks, and assasinations. The Chemical Weapons Convention (CWC) has prohibited the use, development, production, and stockpiling of CWAs since its initiation in 1997, however, the threat of deployment still looms. Detection of trace CWAs post-deployment or post-remediation, in bulk matrices such as soil, often requires lengthy sample preparation steps or extensive chromatographic separation times. 3D-printed cone spray ionization (3D-PCSI), an ambient ionization mass spectrometric (MS) technique, provides a rapid, simple, and low-cost method for trace CWA analysis in soil matrices for both in-laboratory and in-field detection. Described here is the utilization of conductive 3D-printed cones to perform both rapid sampling and ionization for CWA simulants and hydrolysis products in eight solid matrices. The analysis of trace quantities of CWA simulants and hydrolysis products by 3D-PCSI-MS coupled to both a commercial benchtop system and a field-portable MS system is detailed. Empirical limits of detection (LOD) for CWA simulants on the benchtop MS ranged from 100 ppt to 750 ppb and were highly dependant on solid matrix composition, with the portable system yielding similar spectral data from alike matrices, albeit with lower sensitivity.
Introduction
Chemical warfare agents (CWAs) are toxic chemicals that can be disabling or fatal to humans. CWAs can be dispersed in a variety of forms including gases, liquids, aerosols, or powders made of agents adsorbed onto particles.1,2 Modern CWAs, first used in World War I, have been prohibited by the Chemical Weapons Convention (CWC), but the threat of these agents being weaponized by terrorist groups still exists.1,3,4 North Atlantic Treaty Organization (NATO) has classified CWAs into 5 classes including blister agents (i.e. sulfur mustard), nerve agents (G series and V series, i.e. sarin), choking agents (i.e. chlorine gas), asphyxiants, and incapacitating/behaviour altering agents.4
Since its initiation in 1997, the CWC and participating countries have agreed to eliminate CWAs through destruction of any chemical stockpiles, removal of any production facilities, and banning their development or production.5 Current methods for destruction of CWA stockpiles include incineration or neutralization by base hydrolysis.4,6,7 However, if an attack or exposure occurs, detection and analysis of the plume, the bulk supply, any human exposure, and environmental contamination are required. This necessitates the detection of CWAs and their degradation products in diverse matrices and at variable concentrations, ranging from bulk agent to traces at the part per billion (ppb) level.8 The fate of CWAs in the environment can depend on sorption, volatilization, hydrolysis, microbial degradation, and photolysis. Hydrolysis is the primary degradation pathway for many CWAs in aqueous environments, and the process depends on environmental factors such as temperature, pH, and water quality.9
Onsite testing is typically performed using colorimetric devices, portable sensors, or field laboratories.10 The selection of the detection system is dependent on many factors, including the time needed to get an identification, false positive rates, required sensitivity and selectivity, cost, and the nature of the samples collected; cognizant samples of interest are highly diverse, including bulk materials (e.g., clothing, paper, etc.), contaminated soil or water, vapors, and even bodily fluids from exposed victims.2 Colorimetric kits are an inexpensive way to detect CWAs, rapidly producing a color change if threats are present; however, they are marked by low specificity and high false-positive results.1,10 Portable and handheld instruments for point detection include ion mobility spectrometers (IMS), gas chromatography-mass spectrometers (GC-MS), and surface acoustic wave sensors.11 Laboratory-based instruments, with superior sensitivity and specificity, include GC-MS and liquid chromatography-tandem mass spectrometry (LC-MS/MS);12 these are typically employed when confirmation is needed.
GC-MS is the most reliable analytical technique used for the detection of CWAs, however, aqueous samples and polar CWAs need to be derivatized for analysis, which is an additional time consuming step.13,14 GC-MS has been used to detect CWAs and their degradation products in environmental samples (contaminated water and soil) and biological samples (blood and urine).14–19 LC-MS/MS can be used to detect CWAs without performing a derivatization step.20 Previous reports have used LC-MS/MS to detect CWAs and their hydrolysis products in contaminated soil,8,21,22 dried blood spot samples,23 urine, saliva,24 and water.20,21
While hyphenated techniques are currently the predominant methodology to analyze for CWAs post-exposure, as well as for oversight of recalcitrant governments and terrorist organizations, ambient ionization mass spectrometry has emerged in the last fifteen years and can provide results in a fraction of the time.25 Ambient ionization, which began with the development of desorption electrospray ionization (DESI)26 and direct analysis in real time (DART),27 ushered in a field of mass spectrometry where samples are analyzed in their native state with little to no sample preparation.28 Additionally, the sample is directly evaluated by the mass spectrometer, therefore chromatographic separations are not required.29 When sampling in the field for environmental and forensic studies, ambient ionization methods can speed up analyses that are usually rate-limited by sample transport, necessary preparation and chromatographic separation to near realtime.30,31 Previously, DESI,32–35 DART,36–38 and later stage ambient ionization sources like low temperature plasma (LTP)39,40 and atmospheric solids analysis probe (ASAP)41,42 have been demonstrated on a myriad of sample and substrate types for rapid, in situ detection of CWAs.
A subsection of ambient ionization techniques combines both sampling and ionization, where the substrate used for ionization also acts as the sampling device. An example is swab touch spray ionization (STSI), where a rayon tipped swab connected to a conductive handle is used as the sampling device, and then when solvent and a potential are applied to the handle, spray-based ionization occurs.43,44 STSI has been utilized to swab surfaces for the direct detection of CWA simulants in seconds.45 Paper spray ionization (PSI), which employs paper substrates for collection and ionization, has also been demonstrated for CWA analysis.46–51 PSI utilizes paper substrates cut into a triangular shape as the ionization source.52,53 The sample is deposited onto the paper substrate via swabbing, dipping, liquid deposition, or through wafting gaseous samples over the paper. Once high voltage and an appropriate spray solvent are applied, the solvent wicks through the paper, extracting analytes and creating an electrospray-like process at the tip.53
The Glaros and Manicke research groups have developed a PSI-MS method to detect CWA simulants in biological samples48 and from aerosols.47 Follow-up PSI-MS experiments were then applied to authentic CWAs but proved troublesome with traditional PSI substrates. Glaros et al. incorporated metal–organic frameworks (MOFs) on fiberglass substrates to increase adsorption during sampling and desorption of CWAs during PSI analysis.50 Another strategy to help with CWA detection using PSI is to perform online derivatization.49 The derivatization product has a decreased volatility, allowing CWAs to be captured and retained more readily. This process does not require any additional sample preparation due to the dopant being directly applied to the paper and dried prior to analysis, and the derivatization process occurs in near real-time. Manicke et al. have also developed a method for soil analysis using PSI-MS.51 This study analyzed four simulants and five hydrolysis products for G-series nerve agents in two different soil types. Using 25 mg of soil, their LOD for CWA simulants in soil was 50 ng g−1 and between 1–5 ng g−1 for the hydrolysis products.
Paper cone spray ionization (PCSI) is a 3D variant on PSI that has been demonstrated in applications requiring bulk sample analysis.54–57 PCSI uses filter paper crafted into a pyramidal shape to easily allow the analysis of bulk samples. A recent variant of PCSI that features on-board filtration, filter cone spray ionization (FCSI),58 alleviates carryover events stemming from complex matrices. Spray solvent is added to the conical reservoir holding the sample of interest, and when high voltage is applied, extracted analytes flow to the tip where they undergo ESI-like ionization. This method removes rigorous preparative steps, as the bulk solid can be simply added into the cavity of the cone, and after solvent is added, spectra are rapidly obtained and can last up to 8 minutes, as reported.58
Recently, a 3D-printing method utilizing conductive plastics to perform an adaptive PCSI method known as 3D-printed cone spray ionization (3D-PCSI) has been developed.59 Per- and polyfluoroalkyl substances were detected and identified in a variety of soil types by 3D-PCSI-MS with LODs as low as 100 ppt. 3D-printing increases the rigidity of the cone and prevents damaging the tip, while providing utility for scooping. 3D-printing has seen an increase in analytical chemistry60,61 as printing enables rapid prototyping,62 increases open-source sharing,61 and increases reproducibility.63 Additionally, 3D-printing in chemical education laboratory curricula is increasing, lowering the knowledge barrier to its utilization.64,65 The larger sample sizes that 3D-PCSI-MS can provide, as well as the aforementioned benefits of rigidity, stability, and reproducibility, makes 3D-PCSI-MS a prime method for the analysis of CWAs in soil and solid matrices.
More importantly, 3D-PCSI-MS does not require pneumatic gas assistance, making it easier to couple with portable mass spectrometers. Portable MS systems have seen advances over the last few decades with improvements to size, weight, and power consumption.31,66–68 Recent studies have demonstrated the robustness and analytical validation of ambient ionization sources coupled with portable MS,69,70 as well as the legality of utilizing these instruments from a forensic point of view.71,72 With the need of on-site detection for monitoring the safe disposal of CWAs, the swift detection of CWA-based terrorism, and the oversite of government bodies to ensure compliance with the CWC, rapid analysis by fieldable MS systems is of increased interest.73,74 Presented here is the in situ analysis of CWA simulants and their hydrolysis products by 3D-PCSI-MS on both benchtop and portable systems, tested over a wide range of soil matrices to demonstrate the universality of the method.
Experimental
Supplies and materials
All CWA simulants and hydrolysis products (Table 1) (with the exception of diisopropyl methylphosphonate and cyclohexyl methyl methylphosphonate), HPLC-grade methanol, carbon tetrachloride, ammonium hydroxide, clean loam soil, clean clay #5, clean sand #4, clean sediment #2, and clean sandy soil were purchased from Millipore Sigma (St Louis, MO). Diisopropyl methylphosphonate and cyclohexyl methyl methylphosphonate were purchased from Fisher Scientific (Pittsburgh, PA). Gravel, Topsoil, and Silt were purchased from Ward's Science (Rochester, NY). All CWA simulants and CWA hydrolysis products were prepared via serial dilution in methanol, and 100 μL was dispensed onto ∼1 g of solid matrix (except gravel which ∼5 g was used to fill the cone), mixed, and allowed to dry. Upon analysis, no sample preparation was performed other than depositing the contaminated solid matrix into the 3D-printed cone for analysis. Table S1† includes the structure of each CWA simulant and hydrolysis product and also gives a brief description of which CWA class each compound is a simulant for.75–77
Table 1 Benchtop and portable ion trap MS parameters used for MSn experiments including precursor ion m/z, product ions used for identification, and collision energy used for CWA simulants or hydrolysis products
Chemical warfare agent simulants |
Name |
MW (g mol−1) |
[M + H]+ or [M + Na]+a (m/z) |
LTQ CEb |
CID fragments (m/z) |
AI-MS CEc (eV) |
CID fragments (m/z) |
Dimethyl methyl phosphonate (DMMP) |
124.08 |
125 |
20 |
111, 93 |
0.173 |
111 |
Trimethyl phosphate (TMP) |
140.07 |
141 |
30 |
127, 109, 95 |
0.190 |
127 |
Diethyl methyl phosphate (DEMP) |
152.13 |
153 |
30 (MS2) |
125 |
0.204 |
125, 97 |
20 (MS3) |
97 |
Diisopropyl methyl phosphonate (DIMP) |
180.18 |
203a |
35 (MS2) |
161 |
0.259 |
161, 119 |
20 (MS3) |
119 |
Triethyl phosphate (TEP) |
182.07 |
183 |
30 (MS2) |
155 |
0.237 |
155, 127, 99 |
30 (MS3) |
127, 99 |
Cyclohexyl methyl methylphosphonate (CMMP) |
192.19 |
215a |
30 |
133 |
0.272 |
133 |
Tripropyl phosphate (TPP) |
224.23 |
225 |
30 (MS2) |
183 |
0.283 |
183, 141, 99 |
30 (MS3) |
141, 99 |
Triisopropyl phosphate (TiPP) |
224.23 |
247 a |
20 (MS2) |
205 |
0.307 |
205, 163, 121 |
20 (MS3) |
163, 121 |
Profenofos |
373.63 |
373 |
35 (MS2) |
345 |
0.446 |
345 |
10 (MS3) |
303 |
Chemical warfare agent hydrolysis products |
Name |
MW (g mol−1) |
[M − H]− (m/z) |
LTQ CEa,b |
CID fragments (m/z) |
AI-MS CEc |
CID fragments (m/z) |
Sodiated adduct, [M + Na]+ observed as base peak.
Normalized collision energies.
Energy setting for excitation waveform during CID MS2.
|
Thiodiglycol (TDG) |
122.04 |
121 |
20 |
77 |
— |
— |
Ethyl methyl phosphonic acid (EMPA) |
124.08 |
123 |
20 |
95 |
— |
— |
Pinacolyl methylphosphonate (PinMP) |
180.18 |
179 |
20 |
165, 149, 135, 121, 95 |
— |
— |
3D-printing parameters
All 3D-printed cones were constructed on a MakerGear M2 3D-printer (Beachwood, OH). The cone geometry was designed using Autodesk Inventor (San Rafael, CA) and converted to an STL file and sliced using Simplify3D (Cincinnati, OH). Previous work has provided the STL file for the cone design.59 The glass print platform was covered with Kapton tape and maintained at 95 °C for the duration of the print. The 3D-printer's stainless-steel extruder nozzle (0.35 mm) was heated to 250 °C. ESD-Safe PETG 3D-printing filament (3DXSTAT, Grand Rapids, MI) was utilized to construct the cones. The plastic is constructed with multi-wall carbon nanotubes embedded into the plastic to permit conductivity. PETG is a chemically-resistant material that does not react with methanol, therefore, the cone will not degrade or deform after the extraction and spray solvent is added. The dimensions of each cone printed was 30 mm × 30 mm × 29.3 mm and had an opening at the apex of the cone roughly 0.2 mm.
3D-printed cone spray ionization mass spectrometry conditions
All mass spectra were collected using a benchtop Thermo-Fisher LTQ ion trap mass spectrometer (San Jose, CA) or a field portable FLIR AI-MS 1.2 cylindrical ion trap mass spectrometer (West Lafayette, IN). All CWA simulants were identified in positive ionization mode, and CWA hydrolysis products were identified in negative ionization mode. Samples were prepared by placing approximately 1 g of contaminated solid matrix (with the exception of gravel where 5 g was utilized) into the cavity of the 3D printed cone. A 1 mL aliquot of 95
:
5 methanol
:
CCl4, with 0.01% ammonium hydroxide solution was deposited atop the solid matrix, which acted both as the extraction solvent and the spray solvent. This solvent system was selected to help promote ionization while maintaining a stable spray, as seen in other ambient ionization methods.48,51
A potential, ±5.75 kV on the benchtop system and +4.5 kV on the FLIR AI-MS 1.2, was applied to the 3D printed cone via a copper clip attached to the instrument's power supply for positive or negative ion mode, respectively. The CWA simulants and hydrolysis products were each identified by their characteristic MS2 (LTQ and AI-MS 1.2) or MS3 (LTQ) spectra (Table 1) The collision energies applied to the CWA simulants and hydrolysis products’ precursor ions can be found in Table 1. The optimization of cone positioning, instrumental setups, as well as detailed photographs and CAD files can be found in previous manuscripts.55,59 A depiction of the 3D-PCSI source coupled with the FLIR AI-MS 1.2 for sand analysis can be seen in Fig. S2.†
Results and discussion
Nine CWA simulants and three CWA hydrolysis products were selected for characterization by 3D-PCSI-MS. Simulants and hydrolysis products were identified based on their MS2 or MS3 transitions on both a benchtop ion trap and a portable ion trap instrument. Table 1 details the parent ion that was isolated, corresponding fragments, and fragmentation energies used for each CWA simulant and hydrolysis product. Simulants were analyzed in positive ion mode where the [M + H]+ ion was isolated, except for three standards (DIMP, CMMP, and TiPP), where the sodium adduct was isolated [M + Na]+. The hydrolysis products were detected in negative ion mode using the [M − H]− peak. For the benchtop instrument, MS3 was used to confirm six of the CWA simulants to increase confidence in the identification and alleviate interferences from isobaric compounds native to the soil.
The MS2 and MS3 spectra for the CWA simulants in sandy soil at their respective empirical limits of detection (LODs) by 3D-PCSI-MS on a benchtop system are shown in Fig. 1. As solid matrices can alter the LODs drastically, eight soil types were explored in this study to demonstrate the applicability of this technique in a variety of environments. Table 2 outlines the empirical LODs for each CWA simulant in all eight solid soil types. These empirical LODs were determined based on triplicate measurements. LODs for CWA simulants range from 100 ppt to 750 ppb depending on the analyte and matrix type, where sand, sandy soil, and gravel exhibited the lowest detection limits. Topsoil and silt consistently had higher LODs due to more isobaric compounds interfering with MS2 analysis. Blank soil samples were run to ensure that the indicative fragments originated from spiking CWAs into the clean soil, rather than the soil itself. Interfering compounds at the limits of detection were isobaric interferences, and no carry-over was detected. Fig. S1† shows a representative 3D-PCSI-MS spectra of neat soil without spiked CWA targets.
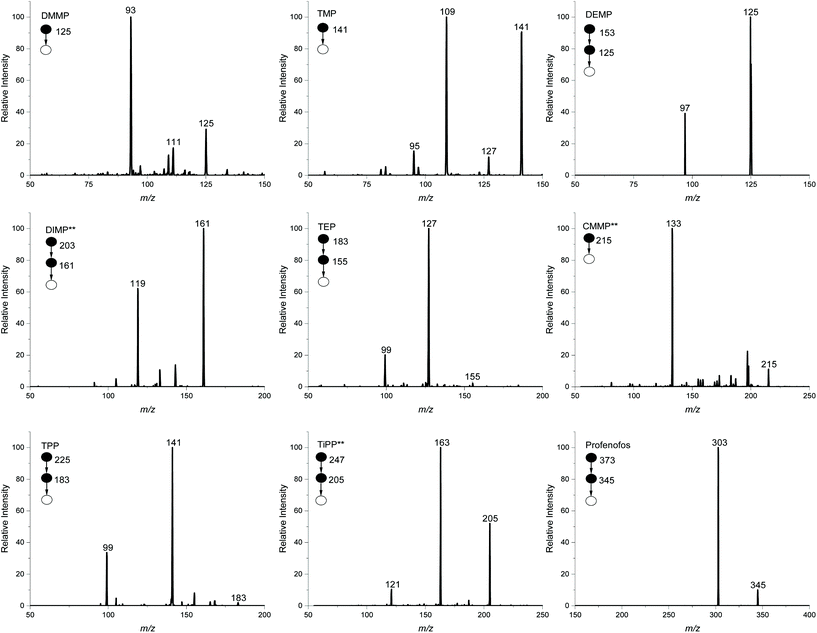 |
| Fig. 1 MSn spectra of CWA simulants at their respective empirical LODs on the benchtop ion trap instrument. The [M + H]+ peak was isolated for all CWA simulants except DIMP, CMMP, and TiPP which the sodium adduct [M + Na]+ was isolated and fragmented. The use of the sodium adduct is indicated by ** next to the compound name. | |
Table 2 The empirical limits of detection based on triplicate measurements, for the nine CWA simulants and the three CWA hydrolysis products in eight different solid matrix types on the benchtop ion trap mass spectrometer. LOD concentrations reported in parts per billion (ppb)
|
Chemical warfare agent simulant |
Name |
Clay |
Gravel |
Loam |
Sand |
Sandy soil |
Sediment |
Silt |
Top soil |
DMMP |
1 |
0.5 |
1 |
0.5 |
0.5 |
1 |
1 |
1 |
TMP |
50 |
10 |
50 |
10 |
10 |
50 |
50 |
50 |
DEMP |
5 |
1 |
5 |
1 |
1 |
5 |
100 |
5 |
DIMP |
5 |
0.5 |
5 |
0.5 |
0.5 |
5 |
5 |
5 |
TEP |
10 |
0.5 |
50 |
1 |
1 |
50 |
50 |
50 |
CMMP |
10 |
5 |
5 |
1 |
5 |
5 |
750 |
500 |
TPP |
50 |
5 |
50 |
10 |
10 |
50 |
100 |
50 |
TiPP |
1 |
0.1 |
0.1 |
0.1 |
0.1 |
5 |
50 |
50 |
Profenofos |
1 |
5 |
1 |
0.5 |
1 |
50 |
5 |
1 |
|
Chemical warfare agent hydrolysis product |
Name |
Clay |
Gravel |
Loam |
Sand |
Sandy soil |
Sediment |
Silt |
Top soil |
TDG |
0.1 |
0.1 |
0.1 |
1 |
1 |
0.1 |
0.1 |
0.1 |
EMPA |
0.1 |
5 |
1 |
5 |
5 |
0.5 |
5 |
0.5 |
PinMP |
100 |
5 |
10 |
5 |
5 |
100 |
100 |
100 |
For hydrolysis products, the LODs range from 100 ppt to 100 ppb. For TDG and EMPA, LODs were higher in sand and sandy soil compared to the other solid matrix type, which was counter to the CWA simulants. PinMP has the highest detection limits, ranging from 5–100 ppb, mostly affected by interference from isobaric compounds from the more complex soil types in MS2. The MS2 spectra for the hydrolysis products in sandy soil at their LODs are shown in Fig. 2. For EMPA and PinMP, the main fragment is m/z 95, corresponding to the methyl phosphonate backbone after losing the ethyl or pinacoyl group, respectively. Peaks can also be seen for the fragmentation of the pinacoyl group in PinMP but depending on the fragmentation energy applied, this may change across different instrument types. The LOD for PinMP was therefore based on the m/z 95 fragment.
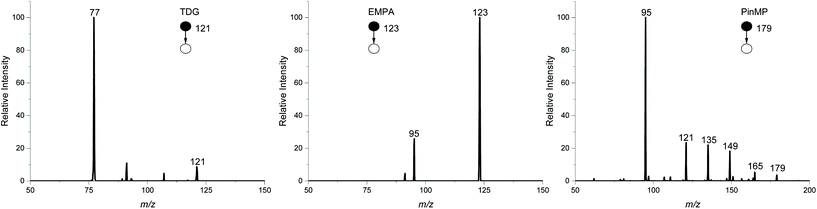 |
| Fig. 2 MS/MS spectra for CWA hydrolysis products at their respective empirical LODs on the benchtop ion trap instrument. The [M − H]− peak was isolated and fragmented in MS2 for all CWA hydrolysis products. | |
Soil-borne mixture analysis via 3D-PCSI-MS on a portable MS system
3D-PCSI-MS was easily coupled to the FLIR AI-MS portable system, which features a direct, atmospheric pressure inlet, allowing the screening of target CWA simulants in the test set of soil matrices. As seen in Fig. S2,† all that is needed for coupling is application of the on-board high voltage via a clamping, “alligator”-style electrode. Both MS and MS2 data collected on the AI-MS 1.2 were analogous to the commercial system, as seen in Table 1, with the exception of known in-source fragments seen in base MS spectra; these signatures predominately match those collected via MS2 of the target analyte.78
The utility of 3D-PCSI-MS coupled to portable MS units towards multi-target CWA screening from complex, soil-borne matrices was demonstrated. Fig. 3 depicts results collected from sand containing 5 ppm each of DMMP, TMP, DEMP, DIMP, TEP, CMMP, TPP, and TiPP. After addition of spray solvent and initial establishment of spray-based ionization, base MS spectra (seen in Fig. 3A) are marked by the appearance of protonated molecules and sodiated adducts; for some CWA simulant targets (e.g., DIMP, TiPP), both ion signatures are seen. Of note, 3D-PCSI-MS on the AI-MS 1.2 demonstrated extended signal durations, with some sample aliquots yielding spectra for durations approaching 25 minutes, allowing ample time for unknown identification via MS2 fragmentation spectra. For longer analysis times, it was observed that sodiated ions diminished over time, as the repeated application of solvent extracts and removes alkali earth metals innate to soil matrices. As seen in Fig. 3B, 3D-PCSI-MS spectra collected after ∼7 min of analysis are dominated by protonated molecular signatures. Corresponding MS/MS spectra utilized to confirm the target CWA simulants from this study can be seen in Fig. S3.†
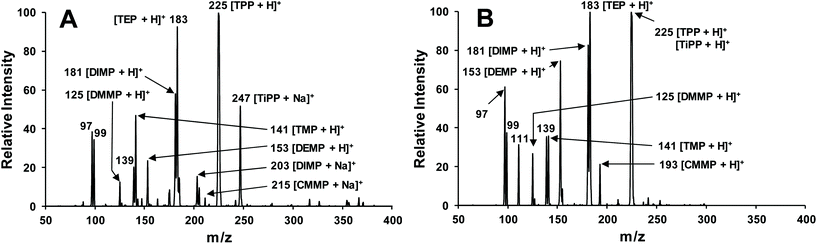 |
| Fig. 3 3D-PCSI-MS collected on the portable AI-MS from a mixture of DMMP, TMP, DEMP, DIMP, TEP, CMMP, TPP, and TiPP (all at 5 ppm each) in sand. Spectra (A) collected at 1 min of analysis time yield both protonated and sodiated adducts for some target CWA simulants, where spectra (B) from ∼7 min predominately show only the protonated forms, as salt leaves the sand matrix. | |
Detection limits for soil-borne, CWA simulants collected on the AI-MS 1.2 ranged from high ppb to low ppm, as seen in Table S2† for sand. While LODs were appreciably higher than those collected on a benchtop system, this is typical for MS instruments featuring miniaturized vacuum systems,35 and still supports the capability of trace screening of CWA targets in soil.
Conclusions
3D-PCSI-MS is a new ambient ionization technique that has been developed for the analysis of contaminants in bulk matrices. 3D-printing has allowed for disposable ionization sources to be quickly and reproducibly generated using conductive plastics to create a rigid cone for in-field analysis. Nine CWA simulants and three CWA hydrolysis products were detected in various soil types, producing characteristic MS and MSn spectra useful for rapid screening and identification. The reported method was marked by high sensitivity, yielding detection limits as low as 100 ppt (depending on the soil type), with sand, sandy soil, and gravel exhibiting some of the lowest LOD across analytes when utilizing a benchtop MS.
3D-PCSI-MS was easily adapted to a portable MS system, producing analogous spectra, albeit in a fieldable form factor. Screening of soil-borne CWA simulants was demonstrated, with signal duration routinely over 10 min, which in turn allows thorough investigation of unknown targets via MS2 fragmentation spectra. Rapid, trace-level screening of CWA simulants afforded by 3D-PCSI-MS employed on fieldable MS systems naturally applies to both the identification and remediation of CWA events alike, eliminating the bottleneck of off-site sample analysis. By pre-screening samples at the site of contamination, future application of 3D-PCSI-MS, coupled to a portable MS, will reduce the number of samples transported to the laboratory for confirmatory analysis. The simplicity in analysis and, especially, solid sample collection afforded by 3D-PCSI-MS could naturally adapt to the rigors of hot zone screening, where operators require the use of Level A encapsulating suits and gloves. Here, a “scoop and screen” procedure applied to portable MS systems could prove valuable to robust and rapid assessment of CWA-related events.
Author contributions
HMB and TJM contributed to the investigation, data collection, validation, and writing of the manuscript. KRD provided support with the investigation and data collection. PWF and CCM contributed to conceptualization, funding acquisition, project administration, supervision, and writing-review and editing.
Conflicts of interest
There are no conflicts to declare.
Acknowledgements
HMB, KRD, and PWF acknowledge financial support provided by the Naval Air Warfare Center Weapons Division Ignites program. HMB acknowledges the National Research Council Fellowship. TJM and CCM acknowledge support in part by NIJ Award No. 2017-R2-CX-0022, from the National Institute of Justice, Office of Justice Programs, U.S. Department of Justice. The opinions, findings, and conclusions or recommendations expressed in this publication are those of the authors and do not necessarily reflect those of the Department of Justice. All authors thank Dr. Ian Fallis for intellectual discussions and Dr. Stephen Ayrton of SciPix for artistic contributions to the graphical abstract.
References
- K. Ganesan, S. Raza and R. Vijayaraghavan, Chemical warfare agents, J. Pharm. BioAllied Sci., 2010, 2(3), 166–178 CrossRef CAS PubMed.
-
G. M. Murray, Detection and Screening of Chemicals Related to the Chemical Weapons Convention, in Encyclopedia of Analytical Chemistry, 2013 Search PubMed.
- R. T. Delfino, T. S. Ribeiro and J. D. Figueroa-Villar, Organophosphorus compounds as chemical warfare agents: a review, J. Braz. Chem. Soc., 2009, 20, 407–428 CrossRef CAS.
- S. Chauhan, S. Chauhan, R. D'Cruz, S. Faruqi, K. K. Singh, S. Varma, M. Singh and V. Karthik, Chemical warfare agents, Environ. Toxicol. Pharmacol., 2008, 26(2), 113–122 CrossRef CAS PubMed.
-
O. f. t. P. o. C. Weapons, Convention on the Prohibition of the Development, Production, Stockpiling and Use of Chemical Weapons and on their Destruction, 2020 Search PubMed.
- K. Kim, O. G. Tsay, D. A. Atwood and D. G. Churchill, Destruction and Detection of Chemical Warfare Agents, Chem. Rev., 2011, 111(9), 5345–5403 CrossRef CAS PubMed.
- Y. J. Jang, K. Kim, O. G. Tsay, D. A. Atwood and D. G. Churchill, Update 1 of: Destruction and Detection of Chemical Warfare Agents, Chem. Rev., 2015, 115(24), PR1–PR76 CrossRef PubMed.
- M. R. Gravett, F. B. Hopkins, M. J. Main, A. J. Self, C. M. Timperley, A. J. Webb and M. J. Baker, Detection of the organophosphorus nerve agent VX and its hydrolysis products in white mustard plants grown in contaminated soil, Anal. Methods, 2013, 5(1), 50–53 RSC.
- S. L. Bartelt-Hunt, D. R. U. Knappe and M. A. Barlaz, A Review of Chemical Warfare Agent Simulants for the Study of Environmental Behavior, Crit. Rev. Environ. Sci. Technol., 2008, 38(2), 112–136 CrossRef CAS.
-
Institute of Medicine, Chemical and Biological Terrorism: Research and Development to Improve Civilian Medical Response, The National Academies Press, Washington, DC, 1999, DOI:10.17226/6364.
- E. J. Pacsial-Ong and Z. P. Aguilar, Chemical warfare agent detection: a review of current trends and future perspective, Front. Biosci., Scholar Ed., 2013, 5, 516–543 CrossRef PubMed.
-
P. A. D'Agostino and C. L. Chenier, Analysis of Chemical Warfare Agents: General Overview, LC-MS Review, InHouse LC-ESI-MS Methods and Open Literature Bibliography. DRDC Suffield TR 2006-022, 2006.
-
A. Weissberg, M. Madmon and S. Dagan, Derivatization and Modification of Chemical Warfare Agents and Their Related Compounds for LC–MS–Based Analytical Applications, in Encyclopedia of Analytical Chemistry, 2020 Search PubMed.
- R. Subramaniam, C. Åstot, L. Juhlin, C. Nilsson and A. Östin, Direct Derivatization and Rapid GC-MS Screening of Nerve Agent Markers in Aqueous Samples, Anal. Chem., 2010, 82(17), 7452–7459 CrossRef CAS PubMed.
- S.-Å. Fredriksson, L.-G. Hammarström, L. Henriksson and H.-Å. Lakso, Trace determination of alkyl methylphosphonic acids in environmental and biological samples using gas chromatography/negative-ion chemical ionization mass spectrometry and tandem mass spectrometry, J. Mass Spectrom., 1995, 30(8), 1133–1143 CrossRef CAS.
- J. Nawała, K. Czupryński, S. Popiel, D. Dziedzic and J. Bełdowski, Development of the HS-SPME-GC-MS/MS method for analysis of chemical warfare agent and their degradation products in environmental samples, Anal. Chim. Acta, 2016, 933, 103–116 CrossRef PubMed.
- P. A. D'Agostino and L. R. Provost, Determination of chemical warfare agents, their hydrolysis products and related compounds in soil, J. Chromatogr., A, 1992, 589(1), 287–294 CrossRef.
- G. A. Sega, B. A. Tomkins and W. H. Griest, Analysis of methylphosphonic acid, ethyl methylphosphonic acid and isopropyl methylphosphonic acid at low microgram per liter levels in groundwater, J. Chromatogr., A, 1997, 790(1), 143–152 CrossRef CAS PubMed.
- O. M. Primera-Pedrozo, C. G. Fraga, A. Breton-Vega, M. M. Zumbach, B. P. Wilkins, N. S. Mirjankar and Z. C. Kennedy, Sorption and desorption study of a nerve-agent simulant from office materials for forensic applications, Forensic
Chem., 2020, 20, 100260 CrossRef CAS.
- V. Tak, A. Purohit, D. Pardasani, D. R. Goud, R. Jain and D. K. Dubey, Simultaneous detection and identification of precursors, degradation and co-products of chemical warfare agents in drinking water by ultra-high performance liquid chromatography–quadrupole time-of-flight mass spectrometry, J. Chromatogr., A, 2014, 1370, 80–92 CrossRef CAS PubMed.
- B. Xu, C. Zong, Z. Nie, L. Guo and J. Xie, A novel approach for high sensitive determination of sulfur mustard by derivatization and isotope-dilution LC–MS/MS analysis, Talanta, 2015, 132, 245–251 CrossRef CAS PubMed.
- P. A. D'Agostino, J. R. Hancock and L. R. Provost, Determination of sarin, soman and their hydrolysis products in soil by packed capillary liquid chromatography–electrospray mass spectrometry, J. Chromatogr., A, 2001, 912(2), 291–299 CrossRef.
- L. Yishai Aviram, M. Magen, S. Chapman, A. Neufeld Cohen, S. Lazar and S. Dagan, Dry Blood Spot sample collection for post-exposure monitoring of chemical warfare agents - In vivo determination of phosphonic acids using LC-MS/MS, J. Chromatogr. B: Anal. Technol. Biomed. Life Sci., 2018, 1093–1094, 60–65 CrossRef CAS PubMed.
- T. L. Hayes, D. V. Kenny and L. Hernon-Kenny, Feasibility of Direct Analysis of Saliva and Urine for Phosphonic Acids and Thiodiglycol-Related Species Associated with Exposure to Chemical Warfare Agents using LC-MS/MS, J. Med. Chem. Def., 2004, 2(1), 1 Search PubMed.
-
Ambient Ionization Mass Spectrometry, ed. M. Domin and R. Cody, Royal Society of Chemistry, 2014 Search PubMed.
- Z. Takáts, J. M. Wiseman, B. Gologan and R. G. Cooks, Mass Spectrometry Sampling Under Ambient Conditions with Desorption Electrospray Ionization, Science, 2004, 306(5695), 471 CrossRef PubMed.
- R. B. Cody, J. A. Laramée and H. D. Durst, Versatile New Ion Source for the Analysis of Materials in Open Air under Ambient Conditions, Anal. Chem., 2005, 77(8), 2297–2302 CrossRef CAS PubMed.
- C. L. Feider, A. Krieger, R. J. DeHoog and L. S. Eberlin, Ambient Ionization Mass Spectrometry: Recent Developments and Applications, Anal. Chem., 2019, 91(7), 4266–4290 CrossRef CAS PubMed.
- T.-H. Kuo, E. P. Dutkiewicz, J. Pei and C.-C. Hsu, Ambient Ionization Mass Spectrometry Today and Tomorrow: Embracing Challenges and Opportunities, Anal. Chem., 2020, 92(3), 2353–2363 CrossRef CAS PubMed.
- R. Chen, J. Deng, L. Fang, Y. Yao, B. Chen, X. Wang and T. Luan, Recent applications of ambient ionization mass spectrometry in environmental analysis, Trends Environ. Anal. Chem., 2017, 15, 1–11 CrossRef CAS.
- H. M. Brown, T. J. McDaniel, P. W. Fedick and C. C. Mulligan, The current role of mass spectrometry in forensics and future prospects, Anal. Methods, 2020, 12(32), 3974–3997 RSC.
- P. A. D'Agostino and C. L. Chenier, Desorption electrospray ionization mass spectrometric analysis of organophosphorus chemical warfare agents using ion mobility and tandem mass spectrometry, Rapid Commun. Mass Spectrom., 2010, 24(11), 1617–1624 CrossRef PubMed.
- P. A. D'Agostino, C. L. Chenier, J. R. Hancock and C. R. J. Lepage, Desorption electrospray ionisation mass spectrometric analysis of chemical warfare agents from solid-phase microextraction fibers, Rapid Commun. Mass Spectrom., 2007, 21(4), 543–549 CrossRef.
- I. Cotte-Rodríguez and R. G. Cooks, Non-proximate detection of explosives and chemical warfare agent simulants by desorption electrospray ionization mass spectrometry, Chem. Commun., 2006,(28), 2968–2970 RSC.
- C. C. Mulligan, N. Talaty and R. G. Cooks, Desorption electrospray ionization with a portable mass spectrometer: in situ analysis of ambient surfaces, Chem. Commun., 2006,(16), 1709–1711 RSC.
- J. M. Nilles, T. R. Connell and H. D. Durst, Quantitation of Chemical Warfare Agents Using the Direct Analysis in Real Time (DART) Technique, Anal. Chem., 2009, 81(16), 6744–6749 CrossRef CAS PubMed.
- G. A. Harris, C. E. Falcone and F. M. Fernández, Sensitivity “Hot Spots” in the Direct Analysis in
Real Time Mass Spectrometry of Nerve Agent Simulants, J. Am. Soc. Mass Spectrom., 2012, 23(1), 153–161 CrossRef CAS PubMed.
- G. A. Harris, M. Kwasnik and F. M. Fernández, Direct Analysis in Real Time Coupled to Multiplexed Drift Tube Ion Mobility Spectrometry for Detecting Toxic Chemicals, Anal. Chem., 2011, 83(6), 1908–1915 CrossRef CAS PubMed.
- B. Li, J. Kong, L. Zhang, W. Fu, Z. Zhang and C. Li, The ionization process of chemical warfare agent simulants in low temperature plasma ionization, Eur. J. Mass Spectrom., 2020, 26(5), 341–350 CrossRef CAS PubMed.
- B. Li, J. Kong, L. Yang, L. Zhang, Z. Zhang and C. Li, Direct detection of chemical warfare agent simulants in soil by thermal desorption-low temperature plasma-mass spectrometry, Int. J. Mass Spectrom., 2020, 451, 116320 CrossRef CAS.
- E. J. Crevelin, F. H. Salami, M. N. R. Alves, B. S. De Martinis, A. E. M. Crotti and L. A. B. Moraes, Direct Analysis of Amphetamine Stimulants in a Whole Urine Sample by Atmospheric Solids Analysis Probe Tandem Mass Spectrometry, J. Am. Soc. Mass Spectrom., 2016, 27(5), 944–947 CrossRef CAS PubMed.
- F. Zydel, J. R. Smith, V. S. Pagnotti, R. J. Lawrence, C. N. McEwen and B. R. Capacio, Rapid screening of chemical warfare nerve agent metabolites in urine by atmospheric solids analysis probe-mass spectroscopy (ASAP-MS), Drug Test. Anal., 2012, 4(3–4), 308–311 CrossRef CAS PubMed.
- P. W. Fedick and R. M. Bain, Swab touch spray mass spectrometry for rapid analysis of organic gunshot residue from human hand and various surfaces using commercial and fieldable mass spectrometry systems, Forensic Chem., 2017, 5, 53–57 CrossRef CAS.
- R. M. Bain, P. W. Fedick, J. M. Dilger and R. G. Cooks, Analysis of Residual Explosives by Swab Touch Spray Ionization Mass Spectrometry, Propellants, Explos., Pyrotech., 2018, 43(11), 1139–1144 CrossRef CAS.
- D. T. Snyder, L. J. Szalwinski, R. L. Schrader, V. Pirro, R. Hilger and R. G. Cooks, Precursor and Neutral Loss Scans in an RF Scanning Linear Quadrupole Ion Trap, J. Am. Soc. Mass Spectrom., 2018, 29(7), 1345–1354 CrossRef CAS PubMed.
- P. W. Fedick, B. J. Bills, N. E. Manicke and R. G. Cooks, Forensic Sampling and Analysis from a Single Substrate: Surface-Enhanced Raman Spectroscopy Followed by Paper Spray Mass Spectrometry, Anal. Chem., 2017, 89(20), 10973–10979 CrossRef CAS PubMed.
- E. S. Dhummakupt, P. M. Mach, D. Carmany, P. S. Demond, T. S. Moran, T. Connell, H. S. Wylie, N. E. Manicke, J. M. Nilles and T. Glaros, Direct Analysis of Aerosolized Chemical Warfare Simulants Captured on a Modified Glass-Based Substrate by “Paper-Spray” Ionization, Anal. Chem., 2017, 89(20), 10866–10872 CrossRef CAS PubMed.
- J. McKenna, E. S. Dhummakupt, T. Connell, P. S. Demond, D. B. Miller, J. M. Nilles, N. E. Manicke and T. Glaros, Detection of chemical warfare agent simulants and hydrolysis products in biological samples by paper spray mass spectrometry, Analyst, 2017, 142(9), 1442–1451 RSC.
- P. M. Mach, E. S. Dhummakupt, D. O. Carmany, E. M. McBride, M. W. Busch, P. S. Demond, G. M. Rizzo, D. E. Hollinshead and T. Glaros, On-substrate derivatization for detection of highly volatile G-series chemical warfare agents via paper spray mass spectrometry, Rapid Commun. Mass Spectrom., 2018, 32(23), 1979–1983 CrossRef CAS PubMed.
- E. S. Dhummakupt, D. O. Carmany, P. M. Mach, T. M. Tovar, A. M. Ploskonka, P. S. Demond, J. B. DeCoste and T. Glaros, Metal–Organic Framework Modified Glass Substrate for Analysis of Highly Volatile Chemical Warfare Agents by Paper Spray Mass Spectrometry, ACS Appl. Mater. Interfaces, 2018, 10(9), 8359–8365 CrossRef CAS PubMed.
- S. Dowling, E. M. McBride, J. McKenna, T. Glaros and N. E. Manicke, Direct soil analysis by paper spray mass spectrometry: Detection of drugs and chemical warfare agent hydrolysis products, Forensic Chem., 2020, 17, 100206 CrossRef.
- B. S. Frey, D. E. Damon and A. K. Badu-Tawiah, Emerging trends in paper spray mass spectrometry: Microsampling, storage, direct analysis, and applications, Mass Spectrom. Rev., 2020, 39(4), 336–370 CrossRef CAS PubMed.
- E. M. McBride, P. M. Mach, E. S. Dhummakupt, S. Dowling, D. O. Carmany, P. S. Demond, G. Rizzo, N. E. Manicke and T. Glaros, Paper spray ionization: Applications and perspectives, TrAC, Trends Anal. Chem., 2019, 118, 722–730 CrossRef CAS.
- G. Jun, T.-M. Park and S. Cha, Fast and Simple Chemical Fingerprinting Analysis of Medicinal Herbs by Paper Cone Spray Ionization Mass Spectrometry (PCSI MS), Bull. Korean Chem. Soc., 2016, 37(8), 1337–1343 CrossRef CAS.
- W. P. Fedick, L. W. Fatigante, E. Z. Lawton, E. A. O'Leary, E. S. Hall, M. R. Bain, T. S. Ayrton, A. J. Ludwig and C. C. Mulligan, A Low-Cost, Simplified Platform of Interchangeable, Ambient Ionization Sources for Rapid, Forensic Evidence Screening on Portable Mass Spectrometric Instrumentation, Instruments, 2018, 2(2), 5 CrossRef.
- P. Kim and S. Cha, Paper cone spray ionization mass spectrometry (PCSI MS) for simple and rapid analysis of raw solid samples, Analyst, 2015, 140(17), 5868–5872 RSC.
- P. W. Fedick, N. M. Morato, F. Pu and R. G. Cooks, Raman spectroscopy coupled with ambient ionization mass spectrometry: A forensic laboratory investigation into rapid and simple dual instrumental analysis techniques, Int. J. Mass Spectrom., 2020, 452, 116326 CrossRef CAS.
- W. L. Fatigante, S. Mukta, Z. E. Lawton, A. M. Bruno, A. Traub, A. J. Gasa, A. R. Stelmack, C. R. Wilson-Frank and C. C. Mulligan, Filter Cone Spray Ionization Coupled to a Portable MS System: Application to On-Site Forensic Evidence and Environmental Sample Analysis, J. Am. Soc. Mass Spectrom., 2020, 31(2), 336–346 CrossRef CAS PubMed.
- H. M. Brown and P. W. Fedick, 3D-Printed Cone Spray Ionization Mass Spectrometry for the Rapid and Low-Cost Analysis of Per and Polyfluoroalkyl Substances in Soils and Sediments, Chemosphere, 2021, 272, 129708 CrossRef CAS.
- U. Kalsoom, P. N. Nesterenko and B. Paull, Current and future impact of 3D printing on the separation sciences, TrAC, Trends Anal. Chem., 2018, 105, 492–502 CrossRef CAS.
- A. J. Capel, R. P. Rimington, M. P. Lewis and S. D. R. Christie, 3D printing for chemical, pharmaceutical and biological applications, Nat. Rev. Chem., 2018, 2(12), 422–436 CrossRef.
- B. Gross, S. Y. Lockwood and D. M. Spence, Recent Advances in Analytical Chemistry by 3D Printing, Anal. Chem., 2017, 89(1), 57–70 CrossRef CAS PubMed.
- S. Martínez-Jarquín, A. Moreno-Pedraza, H. Guillén-Alonso and R. Winkler, Template for 3D Printing a Low-Temperature Plasma Probe, Anal. Chem., 2016, 88(14), 6976–6980 CrossRef PubMed.
- P. W. Fedick, R. L. Schrader, S. T. Ayrton, C. J. Pulliam and R. G. Cooks, Process Analytical Technology for Online Monitoring of Organic Reactions by Mass Spectrometry and UV–Vis Spectroscopy, J. Chem. Educ., 2019, 96(1), 124–131 CrossRef CAS.
- J. Pernaa and S. Wiedmer, A Systematic Review of 3D Printing in Chemistry Education – Analysis of Earlier Research and Educational Use through Technological Pedagogical Content Knowledge Framework, Chemistry Teacher International, 2019, 2(2), 1 Search PubMed.
- K. Evans-Nguyen, A. R. Stelmack, P. C. Clowser, J. M. Holtz and C. C. Mulligan, Fieldable Mass Spectrometry for Forensic Science, Homeland Security and Defense Applications, Mass Spectrom. Rev., 2020 DOI:10.1002/mas.21646.
- C. C. Mulligan, D. R. Justes, R. J. Noll, N. L. Sanders, B. C. Laughlin and R. G. Cooks, Direct monitoring of toxic compounds in air using a portable mass spectrometer, Analyst, 2006, 131(4), 556–567 RSC.
- D. T. Snyder, C. J. Pulliam, Z. Ouyang and R. G. Cooks, Miniature and Fieldable Mass Spectrometers: Recent Advances, Anal. Chem., 2016, 88(1), 2–29 CrossRef CAS PubMed.
- P. W. Fedick, F. Pu, N. M. Morato and R. G. Cooks, Identification and Confirmation of Fentanyls on Paper using Portable Surface Enhanced Raman Spectroscopy and Paper Spray Ionization Mass Spectrometry, J. Am. Soc. Mass Spectrom., 2020, 31(3), 735–741 CrossRef CAS PubMed.
- Z. E. Lawton, A. Traub, W. L. Fatigante, J. Mancias, A. E. O'Leary, S. E. Hall, J. R. Wieland, H. Oberacher, M. C. Gizzi and C. C. Mulligan, Analytical Validation of a Portable Mass Spectrometer Featuring Interchangeable, Ambient Ionization Sources for High Throughput Forensic Evidence Screening, J. Am. Soc. Mass Spectrom., 2017, 28(6), 1048–1059 CrossRef CAS PubMed.
- A. M. Bruno, S. R. Cleary, A. E. O'Leary, M. C. Gizzi and C. C. Mulligan, Balancing the utility and legality of implementing portable mass spectrometers coupled with ambient ionization in routine law enforcement activities, Anal. Methods, 2017, 9(34), 5015–5022 RSC.
- M. C. Gizzi, A. M. Bruno, C. C. Mulligan and R. C. Curtis, The fourth amendment and the potential use of field-portable mass spectrometry systems in law enforcement, J. Crim. Justice, 2019, 42(3), 316–330 Search PubMed.
-
Y. Seto, On-site detection of chemical warfare agents, in Handbook of Toxicology of Chemical Warfare Agents, ed. R. C. Gupta, Academic Press, Boston, 3rd edn, 2020, ch. 57, pp. 983–1003 Search PubMed.
- S. Giannoukos, B. Brkić, S. Taylor, A. Marshall and G. F. Verbeck, Chemical Sniffing Instrumentation for Security Applications, Chem. Rev., 2016, 116(14), 8146–8172 CrossRef CAS PubMed.
- M. R. Sambrook, J. C. Vincent, J. A. Ede, I. A. Gass and P. J. Cragg, Experimental and computational study of the inclusion complexes of β-cyclodextrin with the chemical warfare agent soman (GD) and commonly used simulants, RSC Adv., 2017, 7(60), 38069–38076 RSC.
- C. A. Valdez, R. N. Leif, S. Hok and B. R. Hart, Analysis of chemical warfare agents by gas chromatography-mass spectrometry: methods for their direct detection and derivatization approaches for the analysis of their degradation products, Rev. Anal. Chem., 2018, 37(1), 20170007 CAS.
- I. A. Fallis, P. C. Griffiths, T. Cosgrove, C. A. Dreiss, N. Govan, R. K. Heenan, I. Holden, R. L. Jenkins, S. J. Mitchell, S. Notman, J. A. Platts, J. Riches and T. Tatchell, Locus-Specific Microemulsion Catalysts for Sulfur Mustard (HD) Chemical Warfare Agent Decontamination, J. Am. Chem. Soc., 2009, 131(28), 9746–9755 CrossRef CAS PubMed.
- K. E. Vircks and C. C. Mulligan, Rapid screening of synthetic cathinones as trace residues and in authentic seizures using a portable mass spectrometer equipped with desorption electrospray ionization, Rapid Commun. Mass Spectrom., 2012, 26(23), 2665–2672 CrossRef CAS PubMed.
Footnote |
† Electronic supplementary information (ESI) available. See DOI: 10.1039/d1an00255d |
|
This journal is © The Royal Society of Chemistry 2021 |
Click here to see how this site uses Cookies. View our privacy policy here.