DOI:
10.1039/D0SC05495J
(Edge Article)
Chem. Sci., 2021,
12, 2515-2520
A singlet oxygen self-reporting photosensitizer for cancer phototherapy†
Received
5th October 2020
, Accepted 17th November 2020
First published on 18th November 2020
Abstract
Photodynamic cancer therapy has attracted great attention with the increasing threat of tumors, and improving its therapeutic efficacy is highly desirable. However, due to the highly efficient intersystem crossing potency to generate singlet oxygen (1O2), high-efficiency photosensitizers often suffer from weak fluorescence and excess injury to normal tissue. To overcome these obstacles, here we show a reliable self-reporting strategy for real-time monitoring of therapeutic progression. As a proof of concept, a molecular dyad is designed by connecting benzo[a]phenoselenazinium (NBSe) to rhodamine (Rh), namely Rh-NBSe, where the fluorescence of the Rh unit is initially suppressed by the fluorescence resonance energy transfer mechanism, but enabled to recover as feedback signal once the reaction with photosensitized 1O2 takes place. The observed fluorescence increases by irradiation in vitro and in vivo successfully reflect the real-time 1O2 generation speed in photodynamic therapy. In addition, the favorable therapeutic advantages of Rh-NBSe are also verified, for example, the high ΦΔ (0.8) and the low IC50 (0.2 μM, 6 J cm−2). Based on the therapeutic ability and real-time 1O2 self-reporting ability, Rh-NBSe demonstrates significant potential for self-regulating phototherapy.
Introduction
Cancer is currently one of the most lethal diseases in global healthcare, and it has been estimated that 18.1 million new cancer cases were diagnosed and 9.6 million cancer deaths occurred worldwide in 2018.1,2 With the development of clinical treatment, photodynamic therapy (PDT) has drawn more and more attention due to the unique advantages of noninvasiveness, spatiotemporal accuracy and synergism.3–7 The photosensitizers (PSs), as crucial elements, can transfer the endogenous oxygen to reactive oxygen species (ROS) for therapy.8–12 In particular, benzo[a]phenoselenazinium (NBSe) has potential for efficient cancer phototherapy, thanks to its ultrahigh singlet oxygen quantum yield (ΦΔ) in light and non-toxicity in the dark.13,14 However, the negligible fluorescence quantum yield (0.03) of NBSe has limited its use in PDT.13 Meanwhile, excessive light irradiation or PS dosages often lead to the risks of damage to normal tissue in PDT.15 To address these problems, self-reporting PSs can be a good choice, which report the feedback signals to visualize some elements (e.g. singlet oxygen (1O2), superoxide radical, and apoptosis) for diagnosis and control.11,16–19 Based on a self-reporting strategy, NBSe has the potential to realize visualization in PDT and offer feedback to the treatment process. As a vital therapeutic weapon, the 1O2 produced by PSs in light can destroy proteins or organelles in tumor cells.20 There is no doubt that the generation of 1O2in situ can affect the therapeutic intensity during irradiation.21 Therefore, the imaging and tracing of 1O2 are meaningful to monitor therapeutic processes and decrease the risk of damage to normal tissues. However, the short lifetime (∼40 ns) and limited spread distance (∼20 nm) result in the difficulty of real-time imaging of 1O2 during phototherapy, especially for targeted tumor tissues.22
To date, structures such as vinyl dithioether, aminoacrylates, and disulfanyl–ethyl carbonate have been found to have special response to ROS.17,23 Of which, vinyl dithioether can realize efficient 1O2 response by [2 + 2] cycloaddition reactions, and has better biological stability in vivo.24 Usually, self-reporting PSs are combined with two or more segments, realizing therapy and affording fluorescent feedback after the reaction with linker. Some strategies, such as fluorescence resonance energy transfer (FRET), intramolecular charge transfer and electronic energy transfer, can be good choices to realize fluorescence reporting in PDT.25–27 Using these 1O2 active structures as linker, the fluorescence can be gradually released as feedback to control the phototherapy process during the irradiation period. Unfortunately, self-reporting PSs have not been much researched in the past; instead, these 1O2 sensitive linkers are more commonly used for prodrug release.28,29 Moreover, focusing on the fluorescence reporting potency, the therapeutic efficacy of self-reporting PSs is always ignored. Thus, to develop 1O2 self-reporting PSs for high-efficiency therapy is still meaningful.
Herein, we designed a new 1O2 self-reporting PS based on the NBSe structure, which is expected to have both real-time fluorescence reporting ability and favorable therapeutic ability. With rhodamine (Rh) moiety as donor and NBSe moiety as acceptor, a FRET-based PS was constructed after connection via (Z)-1,2-bis(alkylthio)ethene (vinyl dithioether structure), named Rh-NBSe (Fig. 1a). As control, a molecule without the vinyl dithioether linker (direct click reaction) was also designed in the work, named Rh-NBSe-1 (Fig. 1b). In Rh-NBSe, the fluorescence of donor (Rh) can be initially quenched due to the FRET process (Scheme 1),17,30 while the linker would be cleaved upon irradiation, resulting in the release of fluorescence as feedback. Using Rh as donor, the high fluorescence quantum yield can provide stronger fluorescent differentiation reporting and overcome the imaging problem of NBSe.31,32 Both for Rh-NBSe and Rh-NBSe-1, the double-cationic donor–acceptor (D–A) structure has advantages of good solubility and dispersibility, which can efficiently decrease the self-aggregation in aqueous solution. Moreover, cationic PSs are more likely to accumulate in tumor sites because of the lower negative membrane potential of tumors, and the structure inherent targeting (SIT) ability has been identified in some cationic molecules, including Rh and NBSe.33,34 Therefore, the FRET-based PS with SIT ability and double cationic D–A structure has the potential to realize simultaneously integrated imaging, efficient therapy, and real-time 1O2 reporting both in vivo and in vitro.
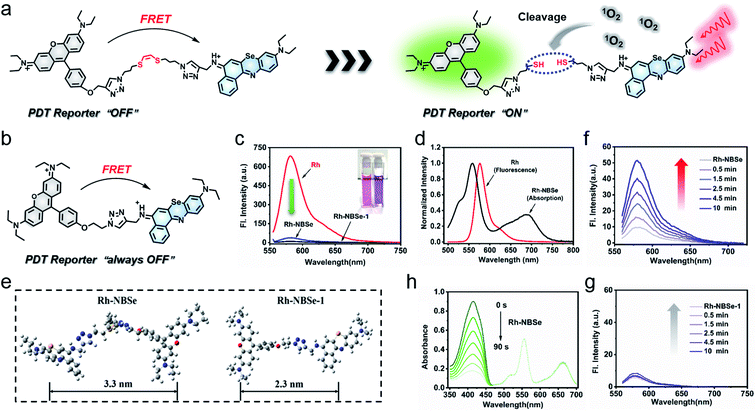 |
| Fig. 1 Schematic illustrations of (a) Rh-NBSe and (b) Rh-NBSe-1. (c) Fluorescence emission comparison of Rh-NBSe, Rh-NBSe-1 and Rh in PBS of 6 μM. (d) Spectral overlap between Rh emission and Rh-NBSe absorption in aqueous solution. (e) Molecular distance between Rh and NBSe moieties of Rh-NBSe or Rh-NBSe-1. Fluorescence recovery of (f) Rh-NBSe and (g) Rh-NBSe-1after irradiation for different times. (h) Singlet oxygen quantum evaluation of Rh-NBSe using 1,3-diphenylisobenzofuran (DPBF) as scavenger. The decay spectra were recorded at an interval of 15 s irradiation (3 mW cm−2). | |
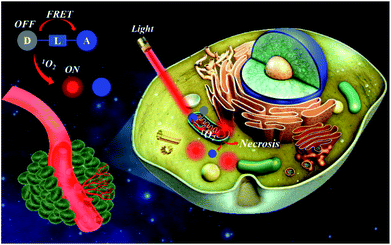 |
| Scheme 1 Sketch of therapy and fluorescence reporting of Rh-NBSe. | |
Results and discussion
Spectral properties
As a proof of concept, both Rh-NBSe and Rh-NBSe-1 were synthesized using the routes shown in Scheme S1,† and the intermediates and products were characterized (Fig. S13–S30†) by mass spectra and nuclear magnetic resonance. To study the D–A structure in Rh-NBSe or Rh-NBSe-1, UV-visible absorption spectra were used to identify the components. As shown in Fig. S1,† the spectra of Rh-NBSe and Rh-NBSe-1 are both consistent with the overlap of Rh and NBSe in DCM, and show little spectral difference in water. The spectral results verify the combined D–A construction, and express the independence of absorption of donor/acceptor. Though Rh and Rh-NBSe both show lower absorption and molar extinction coefficient (ε) of maximum absorption in water compared with Rh in DCM (Fig. S2†), Rh-NBSe can still retain 76% maximum absorption, suggesting the sustaining of solubility after the combination with NBSe. Moreover, the step-up absorption spectra (6–36 μM, at intervals of 6 μM) showed good linearity at maximum absorption peak (R = 0.9999), representing favorable dispersing performance in aqueous solution.
By connection with NBSe, both Rh-NBSe and Rh-NBSe-1 showed weak fluorescence compared with Rh at the same concentration in aqueous solution (Fig. 1c), in which the fluorescence intensity dropped to 36.77 and 9.71 (that of Rh was 684 a.u.), respectively. The results verified the fluorescence quenching, because of the FRET between Rh and NBSe moieties. From the normalized intensity shown in Fig. 1d, the fluorescence spectrum of Rh and the absorption spectrum of NBSe exhibit obvious spectral overlap at 580–650 nm, which is a prerequisite for FRET. Also, FRET needs a suitable molecular distance (1–10 nm) to transfer energy; the distances between Rh and NBSe moieties of Rh-NBSe/Rh-NBSe-1 were calculated to be 3.3/2.3 nm using Gauss software, respectively (Fig. 1e). Both the spectral overlap and molecular distance meet the demands of FRET, and thus Rh-NBSe can realize fluorescence reporting by the breakage of linker due to the off state of FRET.
Under irradiation, the Rh fluorescence of Rh-NBSe will recover when the linker is cut by 1O2, leading to the separation of donor and acceptor. Here we tested the fluorescence spectra of Rh-NBSe in PBS when irradiated by 660 nm LED light (20 mW cm−2), and the fluorescence increase was recorded after a series of irradiation times (0.5, 1.5, 2.5, 4.5, 10 min). As shown in Fig. 1f, the maximum fluorescence increase was from 9.7 to 51.1 a.u. after 10 min irradiation (5.3-fold). By contrast, Rh-NBSe-1 exhibits negligible signal changes after irradiation (Fig. 1g), demonstrating the property of (Z)-1,2-bis(alkylthio)ethene. These results revealed the self-reporting ability of Rh-NBSe, which showed a correlation between fluorescence and irradiation time. Indeed, the fluorescence change can reflect the real-time 1O2, expecting to feedback therapy according the visual signals.
PDT efficacy evaluation in cells
Since the 1O2 generation ability of PSs generally determines the therapeutic efficacy in PDT,35 we calculated the ΦΔ of the molecules. As shown in Fig. 1h, DPBF (1O2 scavenger) absorption spectra show rapid decay at an interval of 15 s irradiation when added to Rh-NBSe. And the control molecule Rh-NBSe-1 or Rh + NBSe (two molecules at the same concentration) shows similar linear decay (Fig. S3†). Using methyl blue (MB) as reference (ΦΔ = 0.57), the ΦΔ of Rh-NBSe, Rh-NBSe-1 and Rh + NBSe were estimated to be 0.8 by a reference method shown in ESI.† These results revealed the similar ΦΔ value for these three groups, suggesting the consistent 1O2 generation ability of NBSe moiety with/without linker. Besides, to confirm the generation of 1O2 in cells, 2,7-dichlorodihydrofluorescein diacetate (DCFH-DA) was selected, which can hydrolyze to DCFH in cells and then ROS oxide DCFH to DCF with bright green fluorescence.36 As shown in Fig. S4,† only the group of PDT shows bright green fluorescence, suggesting the potent intracellular ROS generation ability of Rh-NBSe and Rh-NBSe-1.
Furthermore, to directly evaluate the therapeutic efficacy of Rh-NBSein vitro, live/dead cell imaging in 4T1 cells was conducted using a calcein-AM/PI kit, whereby calcein-AM can be hydrolyzed into calcein and emit green fluorescence in living cells and PI can selectively embed into DNA double helix in dead cells to give red fluorescence.22 As shown in Fig. 2a, Rh-NBSe exhibits almost all green fluorescence, meaning satisfactory biosafety in the dark. In contrast, strong red fluorescence of PI occurs in the group of PDT, which shows strong phototoxicity. Besides, we also tested the cell viability of different concentrations of Rh-NBSe in dark/light using MTT assays.37 Under different exposure times (0, 5, 10 min), the cell viability was measured (Fig. 2b), which revealed the obvious phototoxicity. And the slight difference of cell viability between 5/10 min groups suggested the low light dose demand. Meanwhile, the phototoxicity in vitro was also compared with that of Chlorin e6 (Ce6), as shown in Fig. 2c; both Rh-NBSe and Ce6 keep >90% viability in the dark, but dramatically decreased in light, especially for Rh-NBSe. The IC50 of Rh-NBSe was calculated to be 0.2 μM, while that of Ce6 was 1.1 μM at the same conditions.
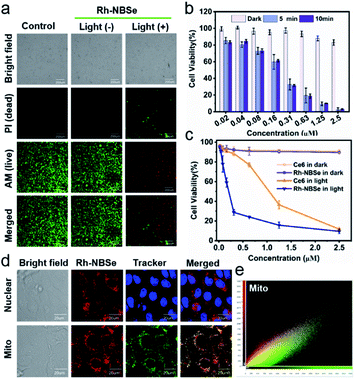 |
| Fig. 2 (a) Live/dead cell imaging using calcein-AM/PI kit after 5 min irradiation (20 mW cm−2). Ex: 488 nm, Em: 505–545 nm (calcein-AM); Em: 620–700 nm (PI). (b) MTT assays of Rh-NBSe with 0, 5 and 10 min irradiation (20 mW cm−2). (c) Cell viability comparison of Ce6 and Rh-NBSe both in dark and light (5 min). (d) Mitochondria colocalization imaging. (e) Colocalization analysis of Mito using CLSM. Data are shown as mean ± SD (n = 6). | |
Subcellular localization and therapeutic mechanism
Subcellular organelle localization generally decides the mechanism of cell death, and influences the therapeutic efficacy. To identify the actual localization, colocalization assays were conducted using commercial dyes. Rh-NBSe showed better mitochondria (Mito) colocalization (Fig. 2d) and good localization ratio with Mito Tracker Green (Fig. 2e). Meanwhile, the Pearson coefficient in Mito was calculated to be 0.896; as a comparison, that of lysosome was 0.473 and nucleus was 0. To further analyze the therapeutic process, we labelled the cellular membrane with DiO (membrane dye), and used PI to indicate the cell viability.38 As shown in Fig. S5,† obvious cell death but intact membrane were observed after 30 min post-irradiation. Then the cellular membrane starts to break after 50 min, and is almost completely broken at 1 h. Taken together, we suspected that the mitochondria could be quickly destroyed after phototherapy, resulting in cellular necrosis, but not apoptosis. By Annexin V-FITC/PI kit staining, in which FITC-labelled recombinant human Annexin V particularly binds to phosphatidylserine of outer surface of apoptotic cells,39 the PDT group showed red fluorescence in cellular nucleus and negligible green fluorescence (Fig. S6†), demonstrating the necrosis mechanism in PDT for Rh-NBSe. In addition, the group with PDT and NaN3 (10 mM, 1O2 scavenger) showed negligible fluorescence both for FITC and PI, indicating the ROS.
Fluorescence reporting in cells and in vivo
Aiming to demonstrate the fluorescence recovery in bio-system followed by the PDT process, the fluorescence variations of Rh-NBSe and Rh-NBSe-1 were compared before/after irradiation using Hoechst 33342 as indicator. As shown in Fig. 3a, Rh-NBSe exhibits obvious fluorescence increase after 5 min irradiation, while Rh-NBSe-1 shows negligible fluorescence change. Analyzing by CLSM, Rh-NBSe showed 3.6-fold fluorescence increase (Fig. 3b) after irradiation; however, only a slight fluorescence increase (1.1-fold) was obtained for Rh-NBSe-1 (Fig. 3c), suggesting the photo-active ability of Rh-NBSe in cells. To better show the fluorescence variations of Rh-NBSe in real time, fluorescence imaging with different irradiation times was also recorded. As shown in Fig. 3d, the fluorescence imaging exhibits a continuous increase during 10 min, and keeps a rapid increase during 3 min (Fig. S7†). The results demonstrate a real-time feedback following the irradiation time, which has potential to regulate the PDT progress.
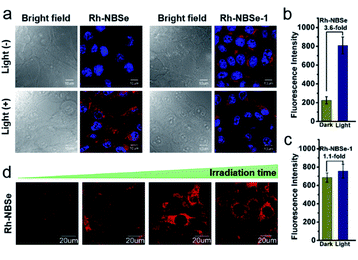 |
| Fig. 3 (a) Fluorescence reporting of Rh-NBSe and Rh-NBSe-1 in 4T1 cells before and after irradiation (660 nm, 20 mW cm−2 × 5 min). Quantitative fluorescence comparison before and after 5 min irradiation of (b) Rh-NBSe and (c) Rh-NBSe-1 calculated by CLSM. (d) Fluorescence increase of Rh-NBSe at different times post-irradiation. | |
To better study self-reporting performance in vivo, we also detected the fluorescence changes in BALB/c mice of subcutaneous 4T1 tumor models. For direct observation in tumors, the mice were peritumorally injected with Rh-NBSe or Rh-NBSe-1, and then the fluorescence images before/after irradiation were compared. As shown in Fig. 4a, the mice treated with Rh-NBSe show a ∼2-fold fluorescence increase upon irradiation, identifying the photo-active ability in mice. In contrast, Rh-NBSe-1 shows a slight fluorescence change in Fig. 4b. The results further reveal the light-dependent fluorescence increase potency of Rh-NBSein vivo.
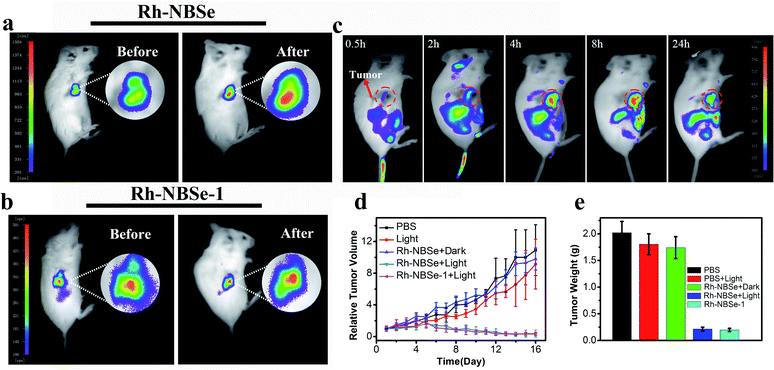 |
| Fig. 4 The self-reporting imaging of (a) Rh-NBSe and (b) Rh-NBSe-1 in mice by intratumoral injection (100 μL × 0.5 μM), before and after 5 min irradiation (660 nm, 50 mW cm−2). (c) The targeted imaging of Rh-NBSe by intravenous injection (100 μL × 0.5 μM). (d) Relative tumor volume record. Groups: PBS, PBS + light (50 mW cm−2, 8 min), Rh-NBSe + dark, Rh-NBSe + light, Rh-NBSe-1 + light. (e) The end tumor weight of different groups. Data are shown as mean ± SD (n = 5). | |
Imaging and therapy in mice
To verify the SIT ability of Rh-NBSein vivo, mice were imaged after tail vein injection of Rh-NBSe. Though the fluorescence of Rh was restricted, it can still realize tumor imaging after amplification of fluorescence. As shown in Fig. 4c, Rh-NBSe showed obvious accumulation at the tumor site, especially after 4 h post-injection. Also the tissues and tumor imaging offered evidence for the ideal tumor targeting capability of Rh-NBSe (Fig. S8†). The therapeutic efficacy of Rh-NBSe was evaluated when the tumor volume reached 100 mm3. Both PBS and PSs were injected intravenously. As control, tumors treated only with PBS showed a rapid increase of ∼10-fold at the end, and ∼9-fold increase was also observed when treated with 660 nm LED light (50 mW cm−2, 8 min) or the group of Rh-NBSe + dark (Fig. 4d), excluding the cytotoxicity of red light or PS in dark. In comparison, tumors treated with Rh-NBSe and irradiation showed significant tumor growth inhibition, as the tumor volume shrank by ∼0.3-fold. Similar to Rh-NBSe, we also evaluated the phototherapeutic ability of Rh-NBSe-1, for which ∼0.25-fold decrease of tumor volume was obtained during 16 days, suggesting the satisfactory suppression of tumors. The final average tumor weight of the 5 groups and corresponding tumor stereogram are depicted in Fig. 4e, and the tumors are shown in Fig. S9.† The histological analysis of tumors stained by H&E also identified apoptosis and necrosis in PDT (Fig. S10†). Also, histological analysis of major organs (heart, liver, spleen, lung and kidney) (Fig. S11†) and stable mice weight changes during treatment (Fig. S12†) showed the non-toxicity of our molecules.
Conclusions
In summary, we have developed a FRET-based 1O2 self-reporting PS, namely Rh-NBSe, which can simultaneously realize therapy, imaging and real-time therapeutic feedback. Using (Z)-1,2-bis(alkylthio)ethene as active linker, the Rh-NBSe dyad can be cleaved by 1O2, restoring the fluorescence of Rh as feedback. The therapeutic ability has been identified in the results reported, which show satisfactory 1O2 generation (ΦΔ = 0.8) and low half maximal inhibitory concentration (IC50 = 0.2 μM) with 5 min irradiation (20 mW cm−2). Also, a 5.3-fold fluorescence recovery in PBS and 3.6-fold in cells reveal the feasible reporting ability of Rh-NBSe. Moreover, the therapy and self-reporting ability were also verified in mice. Therefore, Rh-NBSe can realize efficient phototherapy and 1O2 self-reporting ability in vitro and in vivo, and we believe that the FRET-based self-reporting PS can have potential for theranostics and monitoring.
Ethical statement
All animal operations were in accordance with institutional animal use and care regulations approved by the Model Animal Research Center of Dalian Medical University.
Conflicts of interest
There are no conflicts to declare.
Acknowledgements
This research was supported by the National Natural Science Foundation of China (project no. 21421005, 21576037) and the NSFC-Liaoning United Fund (project nos. U1608222, U1908202). We also thank the Korea Research Fellowship Program funded by the Ministry of Science, ICT and Future Planning through the National Research Foundation of Korea (KRF grant no. 2020H1D3A1A02080172, M. L. L.)
Notes and references
- F. Bray, J. Ferlay, I. Soerjomataram, R. L. Siegel, L. A. Torre and A. C. A. Jemal, Cancer J. Clin., 2018, 68, 394–424 CrossRef
.
- C. G. Lam, S. C. Howard, E. Bouffet and K. P. Jones, Science, 2019, 363, 1182–1186 CrossRef CAS
.
- W. Fan, P. Huang and X. Chen, Chem. Soc. Rev., 2016, 45, 6488–6519 RSC
.
- H. M. Kim and B. R. Cho, Chem. Rev., 2015, 115, 5014–5055 CrossRef CAS
.
- A. P. Thomas, L. Palanikumar, M. T. Jeena, K. Kim and J. Ryu, Chem. Sci., 2017, 8, 8351–8356 RSC
.
- V. A. Ivanova, E. V. Verenikina, V. P. Nikitina, O. E. Zhenilo, P. A. Kruze, I. S. Nikitin and O. I. Kit, J. Clin. Oncol., 2020, 38, 6035 Search PubMed
.
- J. Yoon, Coord. Chem. Rev., 2020, 415, 213297 CrossRef CAS
.
- M. A. Rajora, J. W. H. Lou and G. Zheng, Chem. Soc. Rev., 2017, 46, 6433–6469 RSC
.
- B. M. Luby, C. D. Walsh and G. Zheng, Angew. Chem., Int. Ed., 2019, 58, 2558–2569 CrossRef CAS
.
- P. C. A. Swamy, G. Sivaraman, R. N. Priyanka, S. O. Raga, K. Ponnuvel, J. Shanmugpriya and A. Gulyani, Coord. Chem. Rev., 2020, 411, 213233 CrossRef
.
- T. Zhang, Y. Li, Z. Zheng, R. Ye, Y. Zhang, R. T. K. Kwok, J. W. Y. Lam and B. Z. Tang, J. Am. Chem. Soc., 2019, 141, 5612–5616 CrossRef CAS
.
- N. A. Pace, B. K. Rugg, C. H. Chang, O. G. Reid, K. J. Thorley, S. Parkin, J. E. Anthony and J. C. Johnson, Chem. Sci., 2020, 11, 7226–7238 RSC
.
- J. W. Foley, X. Song, T. N. Denidova, F. Jilal and M. R. Hamblin, J. Med. Chem., 2006, 49, 5291–5299 CrossRef CAS
.
- K. H. Gebremedhin, M. Li, F. Gao, B. Gurram, J. Fan, J. Wang, Y. Li and X. Peng, Dyes Pigm., 2019, 170, 107617 CrossRef CAS
.
- P. Wang, F. Zhou, K. Guan, X. Zhang and W. Tan, Chem. Sci., 2020, 11, 1299–1306 RSC
.
- M. Bio, G. Nkepang and Y. You, Chem. Commun., 2012, 48, 6517–6519 RSC
.
- S. Erbas-Cakmak and E. U. Akkaya, Org. Lett., 2014, 16, 2946–2949 CrossRef CAS
.
- X. Meng, Y. Yang, L. Zhou, L. Zhang, Y. Lv, S. Li, Y. Wu, M. Zheng, W. Li, G. Gao, G. Deng, T. Jiang, D. Ni, P. Gong and L. Cai, Theranostics, 2017, 7, 1781–1794 CrossRef CAS
.
- P. Thapa, M. Li, M. Bio, P. Rajaputra, G. Nkepang, Y. Sun, S. Woo and Y. You, J. Med. Chem., 2016, 59, 3204–3214 CrossRef CAS
.
- T. Xiong, M. Li, X. Zhao, Y. Zou, J. Du, J. Fan and X. Peng, Sens. Actuators, B, 2020, 304, 127310–127320 CrossRef CAS
.
- D. v. Straten, V. Mashayekhi, H. S. de Bruijn, S. Oliveira and D. J. Robinson, Cancers, 2017, 9, 19–72 CrossRef
.
- M. Li, S. Long, Y. Kang, L. Guo, J. Wang, J. Fan, J. Du and X. Peng, J. Am. Chem. Soc., 2018, 140, 15820–15826 CrossRef CAS
.
- G. Nkepang, M. Bio, P. Rajaputra, S. G. Awuah and Y. You, Bioconjugate Chem., 2014, 25, 2175–2188 CrossRef CAS
.
- R. S. Murthy, M. Bio and Y. You, Tetrahedron Lett., 2009, 50, 1041–1044 CrossRef CAS
.
- S. Erbas-Cakmak, O. A. Bozdemir, Y. Cakmak and E. U. Akkaya, Chem. Sci., 2013, 4, 858–862 RSC
.
- J. Ong, C. Lim, H. Le and W. Ang, Angew. Chem., Int. Ed., 2019, 58, 164–167 CrossRef CAS
.
- Y. Yuan, C. J. Zhang, S. Xu and B. Liu, Chem. Sci., 2016, 7, 1862–1866 RSC
.
- G. Nkepang, M. Bio, P. Rajaputra, S. G. Awuah and Y. You, Bioconjugate Chem., 2014, 25, 2175–2188 CrossRef CAS
.
- M. Bio, P. Rajaputra, G. Nkepang, S. G. Awuah, A. M. L. Hossion and Y. You, J. Med. Chem., 2013, 56, 3936–3942 CrossRef CAS
.
- M. Bio, G. Nkepang and Y. You, Chem. Commun., 2012, 48, 6517–6519 RSC
.
- Y. Yang, S. Ko, I. Shin and J. Tae, Nat. Protoc., 2007, 2, 1740–1745 CrossRef CAS
.
- Z. Yang, M. She, S. Ma, B. Yin, B. Liu, B. Liu, S. Zhao and J. Li, Sens. Actuators, B, 2016, 242, 872–879 CrossRef
.
- M. Li, T. Xiong, J. Du, R. Tian, M. Xiao, L. Guo, S. Long, J. Fan, W. Sun, K. Shao, X. Song, J. W. Foley and X. Peng, J. Am. Chem. Soc., 2019, 141, 2695–2702 CrossRef CAS
.
- E. A. Owens, S. Lee, J. Choi, M. Henary and H. S. Choi, Wiley Interdiscip. Rev.: Nanomed. Nanobiotechnol., 2015, 7, 828–838 CAS
.
- M. Li and X. Peng, Acta Chim. Sin., 2016, 74, 959–968 CrossRef CAS
.
- M. Li, Y. Shao, J. H. Kim, Z. J. Pu, X. Zhao, H. Huang, T. Xiong, Y. Kang, G. Li, K. Shao, J. Fan, J. W. Foley, J. S. Kim and X. Peng, J. Am. Chem. Soc., 2020, 142, 5380–5388 CrossRef CAS
.
- Z. Li, J. Wang, J. Chen, W. Lei, X. Wang and B. Zhang, Sci. China: Chem., 2010, 53, 1994–1999 CrossRef CAS
.
- W. Liu, T. Liu, M. Zou, W. Yu, C. Li, Z. He, M. Zhang, M. Liu, Z. Li, J. Feng and X. Zhang, Adv. Mater., 2018, 30, e1802006 CrossRef
.
- W. Ou, J. H. Byeon, R. K. Thapa, S. K. Ku, C. S. Yong and J. O. Kim, ACS Nano, 2018, 12, 10061–10074 CrossRef CAS
.
Footnote |
† Electronic supplementary information (ESI) available. See DOI: 10.1039/d0sc05495j |
|
This journal is © The Royal Society of Chemistry 2021 |
Click here to see how this site uses Cookies. View our privacy policy here.