DOI:
10.1039/D2SC01264B
(Review Article)
Chem. Sci., 2022,
13, 7378-7391
State-of-the-art accounts of hyperpolarized 15N-labeled molecular imaging probes for magnetic resonance spectroscopy and imaging
Received
2nd March 2022
, Accepted 17th May 2022
First published on 17th May 2022
Abstract
Hyperpolarized isotope-labeled agents have significantly advanced nuclear magnetic resonance spectroscopy and imaging (MRS/MRI) of physicochemical activities at molecular levels. An emerging advance in this area is exciting developments of 15N-labeled hyperpolarized MR agents to enable acquisition of highly valuable information that was previously inaccessible and expand the applications of MRS/MRI beyond commonly studied 13C nuclei. This review will present recent developments of these hyperpolarized 15N-labeled molecular imaging probes, ranging from endogenous and drug molecules, and chemical sensors, to various 15N-tagged biomolecules. Through these examples, this review will provide insights into the target selection and probe design rationale and inherent challenges of HP imaging in hopes of facilitating future developments of 15N-based biomedical imaging agents and their applications.
1. Introduction
1.1. Magnetic resonance spectroscopy and imaging – general information and limitations
Magnetic resonance spectroscopy and imaging (MRS/MRI) are powerful non-invasive molecular imaging modalities that provide biochemical and anatomical information about the human body. MR imaging mainly concerns the generation of anatomical images translated into spatial maps to distinguish healthy tissues from diseased areas.1 MR spectroscopy, performed along with MRI, analyzes chemical processes and metabolic contents of the scanned tissue. MRS offers qualitative and quantitative assessments of various MR-active nuclei (i.e., 1H, 13C, 15N, and 31P) in metabolites using chemical shift assignments in the NMR spectra.2,3 Therefore, MRI and MRS have been routinely used in research and clinical practices as imperative diagnostic techniques that offer valuable biochemical and anatomical information. Despite these advancements, magnetic resonance spectroscopic technologies suffer from low sensitivity and clinical MRS/MRI are restricted to the most abundant proton (1H) resonances as the signal source.
All MR scans are evolved from nuclear magnetic resonance (NMR) and the imaging sensitivity mainly relies on the abundance and polarization levels of nuclear spins. As thermal polarization levels of nuclear spins are small, traditional 1H-MRI detects highly abundant 1H signals in the form of water and fat to provide sufficient sensitivity. Yet scanning other MR-active nuclei found in biomolecules is challenging due to their low natural abundances. For example, carbon and nitrogen are among the most common elements found in the structures of biomolecules. The natural abundance for 13C and 15N is only 1.1% and 0.37%, respectively, in comparison to 99.99% for 1H (Table 1).4 Other factors related to the MR signal intensity are the gyromagnetic ratio (γ) and concentration of the nuclei of interest. The γ value directly correlates with the NMR signal sensitivity; for instance, 13C has a low gyromagnetic ratio, which is less than 1/4 of γ(1H), and therefore has a lower relative sensitivity to 1H. Furthermore, the ultra-low γ of 15N (1/10 of γ(1H)) translates into a significant decrease in sensitivity.
Table 1 Nuclear magnetic properties of 1H, 13C and 15N nuclei4
Nucleus |
Natural abundance (%) |
γ (107 rad T−1 s−1) |
Relative sensitivitya |
Relative receptivityb |
At a constant magnetic field and equal number of nuclei.
The receptivity reflects the overall ease of acquiring an NMR signal relative to 1H at the same magnetic field.
|
1H |
99.99 |
26.75 |
1.000 |
1.00 |
13C |
1.11 |
6.73 |
0.016 |
1.70 × 10−4 |
15N |
0.37 |
−2.71 |
0.001 |
3.84 × 10−6 |
In addition to the low γ values, the sensitivity of isotope-enriched metabolites may suffer from the low concentration (sub-millimolar) of the interrogated metabolic species in vivo.5 Accordingly, it is challenging to observe these isotope signals, especially those of 15N nuclei, from biomolecules in vivo with the conventional MRS/MRI. Yet, several hyperpolarization techniques have emerged to tackle the challenge of MR sensitivity.
1.2. Hyperpolarization technique and current methods
The sensitivity of MR correlates with nuclear-spin polarization. The NMR signal intensity is governed by the population difference between two nuclear spin states, also referred to as the polarization level. The polarization is affected by the gyromagnetic ratio (γ) and the magnetic field strength (B0). At thermal equilibrium, polarization levels of MR-active nuclei are low (only 10−6 to 10−4), which is the reason for the low sensitivity of MRI/MRS.6
The hyperpolarization (HP) technique addresses the sensitivity problem and has revolutionized the field of MR spectroscopy and imaging. Hyperpolarization artificially induces a nonequilibrium polarization of nuclear spins for a period of time (Fig. 1). The HP technique can enhance signal sensitivity by several orders of magnitude by increasing the spin state population difference.6 The dramatic signal enhancements allow real-time detection of both introduced hyperpolarized imaging agents and their metabolic products. Thus, HP-MR scans of isotope-labeled probes provide unparalleled ability to monitor complex biological processes through advantageous features of the NMR spectroscopy combined with its high structural specificity, non-invasiveness, and quantitative analysis.
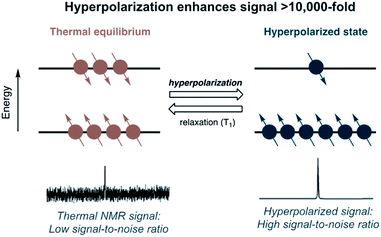 |
| Fig. 1 Hyperpolarization of the nuclear-spin population to enhance NMR signals. | |
Among several available hyperpolarization methods, two techniques have been used mainly for polarizing non-gaseous isotopes. The first technique is dynamic nuclear polarization (DNP), which is currently the most clinically advanced method that has been used for in vivo hyperpolarization studies.5,7 DNP relies on polarization transfer from electrons to the nuclei of interest dissolved in glass-forming solvents via microwave irradiation at low temperatures (1–2 K) for approximately 1–3 hours.8 After polarization build-up, the frozen pellet containing a hyperpolarized imaging agent is quickly dissolved with hot water (hence dissolution-DNP, d-DNP), generating a hyperpolarized solution ready for in vivo imaging. d-DNP is the most established polarization method used for preclinical and clinical imaging, as according to its principle, any molecule of interest can be hyperpolarized in water.
The second hyperpolarization method uses para-hydrogen as the polarization transfer source.9,10 For example, para-hydrogen-induced polarization (PHIP) can be achieved by catalytic hydrogenation of para-H2 across unsaturated bonds (e.g., alkene or alkyne) located near the MR-active isotope. Thus, the reduction of the unsaturated bond with a concomitant break of para-H2 symmetry enables polarization transfer from 1H to nearby 13C or 15N nuclei via networks of J-coupling.11 The PHIP method is not generally applicable as d-DNP as the substrate needs to have an unsaturated bond. Alternatively, para-H2 can be used to deliver polarization transfer by signal amplification by reversible exchange (SABRE) through reversible binding to a metal catalyst from both para-H2 and the substrate.12–14 Thus, SABRE can hyperpolarize a broader scope of substrates than the traditional PHIP method that relies on the irreversible hydrogenation reaction. The detailed mechanisms of these hyperpolarization techniques have been described in several review papers.15–17 Overall, these developments in polarization techniques have significantly advanced simple proof-of-concept ideas of hyperpolarized MRS/MRI into clinical applications.
1.3. Development of hyperpolarized MRI/MRS agents: considering factors and current progress
Hyperpolarized imaging studies rely on molecular probes, which are isotope-enriched chemical agents used to visualize, characterize, and quantify biological processes.18,19 These probes can be fine-tuned to characterize a specific molecular or cellular process of interest for diagnostic or therapeutic applications. Developing an effective hyperpolarized molecular imaging probe is challenging, particularly in addressing several important considerations that are specific to hyperpolarized imaging. First, the labeled nuclei should have a long longitudinal relaxation time, denoted as T1. The MR signal detection window strictly depends on the T1 value, which represents approximately 1/3 of polarization decay back to the thermal equilibrium of the spin population. Therefore, great efforts have been devoted to extending the polarized state in the hyperpolarization process and identifying isotope-labeled functional groups and centers with long T1 lifetimes. For example, 13C centers without directly attached protons, such as 13C centers in carbonyl groups, benefit from the decreased dipolar relaxation and commonly have longer T1 values.20
In addition to the dipolar contribution, the magnetic field strength also affects the T1 value. The magnetic field strength, commonly measured in tesla (T), correlates with the signal-to-noise ratio – a stronger magnetic field yields stronger signals over background noise and consequently, provides a better image. Routinely used clinical MRI scanners have field strengths of 1.5 and 3.0 T, while research MRI and laboratory NMR spectrometers commonly have field strengths of 7.0, 11.7, and 14.1 T. Generally, the T1 has an inverse correlation with the magnetic field, so the higher fields result in shorter T1 values.
Second, the design of HP-MR probes should consider the difference in the chemical shift between the probe and its reaction product (i.e., metabolite). A larger chemical shift difference in the NMR spectra will provide more distinguishable peak identification and quantification, especially in lower magnetic fields (for example, typically 5–40 ppm for 13C).21
In the current field of HP imaging, 13C tracers are the most explored for studying metabolic processes, largely because carbon serves as a backbone for nearly all organic biomolecules. Several comprehensive review papers delineate hyperpolarized 13C probes exploited for preclinical and clinical research,20–28 which is beyond the scope of this review paper. The success in hyperpolarized 13C imaging has validated the applicability of HP MRI/MRS technology in clinical settings for monitoring disease progression and therapy response. At the same time, 13C-labeled agents often manifest short polarization lifetimes, with T1 values of only tens of seconds, presenting a limitation for imaging slower biological processes beyond rapid metabolic systems.
Compared to 13C-based probes, hyperpolarized 15N agents have proved to offer much longer T1 lifetimes and are well suited for sensor designs.29 This review will present current accounts of hyperpolarized 15N-labeled biomolecular probes studied in the literature, the advantages and challenges associated with 15N-probes, and how 15N-agents can provide unique directions in the field of hyperpolarized imaging.
2. Hyperpolarized 15N probes
2.1. Introduction on 15N-labeled probes: unique properties and potential in molecular imaging
Nitrogen atoms are present ubiquitously in bioorganic molecules, and in principle, any nitrogen center can be isotope-enriched with 15N nuclei.30 As 15N has a gyromagnetic ratio lower than those of 1H and 13C, the 15N-NMR signal suffers from poorer sensitivity. However, the reduced interaction of 15N with an external magnetic field allows longer polarization lifetimes of 15N centers in the order of several minutes. Such long polarization lifetimes expand the imaging window of the hyperpolarized species and dramatically broaden the potential applications in biomedical imaging beyond rapid metabolism tracing restricted by the shorter T1 lifetime of 13C metabolites.
Besides potentially long hyperpolarized lifetimes of the 15N nucleus, 15N-NMR has a wider range of chemical shifts. This warrants a greater sensitivity of 15N chemical shift to its environment. The development of non-1H-based MRI and MRS agents has been partially motivated by the difficulty in deconvoluting many metabolite resonances in the narrow chemical shift range of ∼10 ppm of the 1H spectrum. In comparison, peaks corresponding to 13C metabolites of interest occur over a much wider range of approximately 200 ppm. A wider range of chemical shifts provides hyperpolarized 13C-based probes with greater qualitative analysis capability to trace complex biochemical processes. In this respect, the 15N spectrum provides an even more comprehensive range up to 900 ppm,29 thus providing hyperpolarized 15N probes with an even higher detection accuracy and an extended scope of chemical complexity. These favorable features of hyperpolarized 15N-probes offer promising biomedical and clinical imaging applications.
This review presents the up-to-date progress in the development of various 15N agents for hyperpolarized bioimaging. The HP 15N agents reported so far are classified into three main categories in this review: (1) 15N-enriched endogenous molecules and drugs, (2) 15N sensors designed for specific physiological parameters, and (3) biomolecules labeled with 15N molecular tags. The discussion on these probes generally covers the design principles, considerations, and imaging performances in each molecular probe category.
2.2.
15N-Enriched endogenous molecules and drugs
Isotope enrichment is the most straightforward approach in designing HP agents, including 15N-labeled endogenous metabolites and drug molecules. Ideally, HP agents should have low toxicity and high cellular uptake for in vivo imaging. Considering that the imaging agents are typically hyperpolarized ex vivo and injected intravenously into animals, high concentrations of HP agents (generally 10–100 mM) are often needed, taking into account the dilution in the blood, to produce sufficiently detectable NMR signals in vivo. The cytotoxicity profiles of endogenous metabolites and drug molecules are readily available, which expedited the in vivo applications of 15N-enriched hyperpolarized probes. So far, several successful probes in this regard have been reported, including 15N-choline, 15N-permethylated amino acids, 15N-carnitine, 15N-azidothymidine (AZT), and 15N-heterocycle-based drugs.
2.2.1.
15N-Choline.
Choline (Cho) is an endogenous molecule involved in phospholipid metabolism. Elevated metabolism of choline to phosphocholine (PCho) catalyzed by choline kinases is a known characteristic of cancer, making choline an ideal biomarker for tumor imaging.31,32 None of the carbon centers in the natural choline molecule (CH3)3N+CH2CH2OH would retain a long T1 lifetime if 13C-enriched. However, the quaternary amine is suitable for 15N-enrichment to achieve long-lasting polarization, benefiting from the absence of proton-based dipole relaxation.
15N-Enriched choline has been hyperpolarized and studied to monitor in vitro choline metabolism to 15N-PCho for the first time by Gabellieri et al. (Fig. 2A).33 The non-basic and symmetrical environment of the quaternary 15N center in choline led to exceptionally long T1 values of 285 ± 12 s in water and 120 ± 10 s in blood at 37 °C in a magnetic field of 11.7 T. A reduction of T1 in the blood is a documented phenomenon and can result from the increased relaxation caused by the viscosity of blood, presence of red blood cells, and hydrogen-bonding with biomolecules. This study monitored the in vitro enzymatic conversion of hyperpolarized 15N-Cho to 15N-PCho, with a maximum buildup of 15N-PCho observed at 114 s. The initial rate of 15N-PCho buildup was estimated to be 1.45 mM min−1 using choline kinase (2 μM). Such kinetics information obtained using the plotted hyperpolarization signal over time is vital for estimating substrate buildup rates and enzyme activity. Most encouragingly, the 15N signal remained after 10 min, substantially exceeding the longevity compared to deuterated 13C-choline with a T1 of ∼30 s (11.8 T).34 On the other hand, the 15N spectra of 15N-Cho and 15N-PCho showed a chemical shift difference of only ∼0.2 ppm. Such a small difference presents a challenge for practical in vivo imaging of choline kinase activity, especially with low sensitivity of the 15N nucleus at clinically relevant MRI (3 T) (Fig. 2B).33 Nonetheless, the exceptionally long relaxation time of 15N-choline in these earlier studies showed great promise in hyperpolarized 15N imaging and has drawn scientific attention to exploring a new range of biological applications.
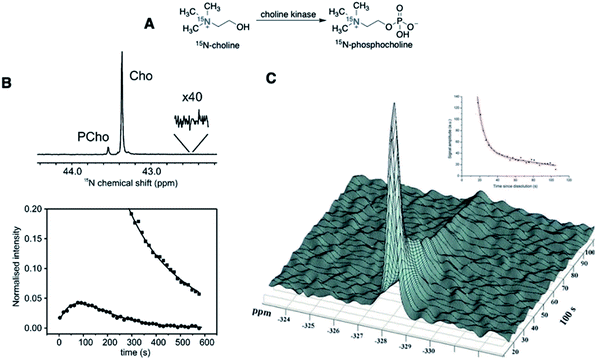 |
| Fig. 2 First hyperpolarization experiments of 15N-choline. (A) Schematic conversion of 15N-choline to 15N-phosphocholine. (B) (Top): Enzymatic conversion of hyperpolarized 15N-Cho to 15N-PCho, scanned at the maximum buildup PCho (t = 114 s, Δ15N = ∼0.2 ppm), and (bottom): peak integral plotted against imaging time in seconds, with squares = 15N-Cho and circles = 15N-PCho. (C) First in vivo polarization decay graph of 15N-Cho spectra, with the 15N peak referenced to nitromethane. (B) Adapted with permission from ref. 33. Copyright 2008, American Chemical Society. (C) Adapted with permission from ref. 35. Copyright 2010, The Royal Society of Chemistry. | |
In 2012, Cudalbu et al. performed MRS of HP 15N-Cho to monitor 15N-Cho build-up in a rat brain. This in vivo study has established the feasibility of detecting hyperpolarized 15N signals in the animal model for the first time.35 Injection of 15N-choline infusate at ∼90 mM was tolerated without severe toxicity, although previous work has reported that MRI of choline was problematic due to its toxicity at high doses.36 As shown in Fig. 2C, hyperpolarized 15N-Cho provided a T1 of 126 ± 15 s in vivo (9.4 T) and the 15N-Cho signals were detectable well over 100 s, possibly over 300 s based on the T1 value. However, the choline kinase activity was not observed in the animal model, possibly owing to the decreased sensitivity of the 15N signal in vivo and slow Cho uptake.
Promising potential of hyperpolarized choline in diverse applications has also attracted efforts to improve hyperpolarization efficiency and lifetimes of 15N-Cho, for example, by a deuteration strategy (Fig. 3). A study by Sarkar et al. showed that naturally abundant choline-d9 with deuterated methyl groups showed a T1(15N) of 390 ± 110 s, and 15N-Cho showed a T1 of 189 ± 2 s (both in D2O at 7 T).37 Note that compared to 285 ± 12 s (11.7 T) in Gabellieri et al.,33 the shorter relaxation of 15N-Cho shown in Sarkar's study was due to the addition of free radicals used for d-DNP hyperpolarization. In another study by Kumagai et al., the fully deuterated 15N-choline-d13 showed a T1 of 580 ± 10 s (9.4 T).38
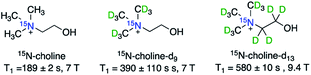 |
| Fig. 3 Structures of 15N-cholines with various degrees of deuteration, showing deuteration of the methyl and methylene groups of choline elongates the T1 lifetime. | |
Similarly, a 15N-choline analog has been hyperpolarized using PHIP in aqueous media, achieving a T1 of 348 ± 10 s and 494 ± 13 s for protonated and deuterated substrates, respectively (9.4 T).39 Longer relaxation times of hyperpolarized 15N signals in these deuterated choline analogs were rationalized by the reduced dipolar relaxation pathway with every neighboring proton (spin = ½) replaced with deuterium (spin = 1). These studies also demonstrate that the deuteration of nearby protons can increase the polarization lifetime of 15N ammonium centers up to 3-fold.
2.2.2. Permethylated, perdeuterated 15N-amino acids.
15N-Enriched derivatives of amino acids, another type of endogenous molecules, have been studied extensively for long-lasting hyperpolarized perfusion imaging. Specifically, the 15N-enriched amino acids can perform as tracers to study renal functions, such as filtration rates and tubular properties that are vital for diagnosing metabolic disorders.
Chiavazza et al. have first prepared permethylated, perdeuterated derivatives of glutamine, glutamate, and lysine.40 The design of perdeuterated glutamine compounds was based on the long relaxation time previously observed for deuterated ammonium centers that benefit from the reduced dipolar interaction with neighboring protons. The α-glutamine-15N, prepared by 15N-enrichment of α-amine (15NH2) in naturally occurring amino acids, had a T1 value of merely 8 s (14.1 T). In comparison, perdeuteromethylation of α-15N-amine in amino acids as a strategic approach dramatically increased the T1 values up to 220–250 s (Fig. 4A).
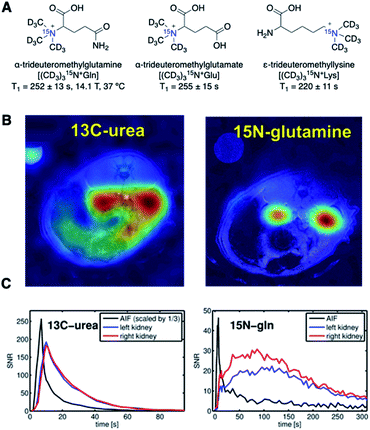 |
| Fig. 4 (A) Structures of perdeuteromethylated 15N glutamine, glutamate, and lysine analogs. T1 values of all three analogs were measured at 14.1 T and 37 °C. (B) HP-MRI of [13C, 15N2] urea (left) and (CD3)315N+Gln (right) at the peak of signal accumulation. Image laid over 1H MRI, demonstrating the localized 15N-glutamine signal in the kidneys. (C) Plot of the signal-to-noise ratio (SNR) of 13C-urea and 15N-glutamine signals over a time course from kidney and blood vessel regions. (B and C) Adapted with permission from ref. 41. Copyright 2016, John Wiley and Sons. | |
Durst et al. applied the amino acid derivative (CD3)315N+Gln to compare the hyperpolarized imaging performances of 15N-probes and 13C-urea in HP-MRI perfusion studies (Fig. 4B and C).41 The signal from the 15N-glutamine analog was localized to the kidney area and detectable for more than 5 minutes. In contrast, the 13C signal from [13C, 15N2]urea was delocalized around the tissue and disappeared within 90 s (Fig. 4B). In practice, the hyperpolarized signal of 15N had a lower SNR than that of 13C, as the SNR correlates with the gyromagnetic ratio (Fig. 4C). However, this was offset by the slow signal decay of the 15N-glutamine analog in the order of several minutes.
These permethylated, perdeuterated amino acid analogs had long signal retention and showed minimal toxicity in an animal model, meeting the requirements for in vivo imaging applications of hyperpolarized probes. The high T1 values and strong localization properties make perdeuteromethylated 15N probes promising candidates for perfusion imaging. These examples also reinforce design principles to increase T1(15N) by reducing the dipolar interaction with neighboring protons and installing a symmetrical environment of the 15N nucleus.
2.2.3.
15N-Carnitine.
The ideal HP properties of quaternary 15N centers are further illustrated by 15N-labeled L-carnitine,42 an endogenous metabolite involved in acetyl-coenzyme A and fatty acid metabolism (Fig. 5A). The T1 times of L-15N-carnitine-d9 were determined to be 210 s in water and 160 s in vivo (4.7 T) (Fig. 5B). Furthermore, the MR spectroscopic imaging of HP 15N-carnitine in the rat abdomen three minutes after injection showed 15N signals localized in the liver and kidney area, proving the feasibility of imaging the biodistribution of an 15N-agent for an extended period (Fig. 5C–E). However, no downstream 15N-acetyl-carnitine metabolites were detected in this study due to magnetic isolation of the 15N-quaternary atom.
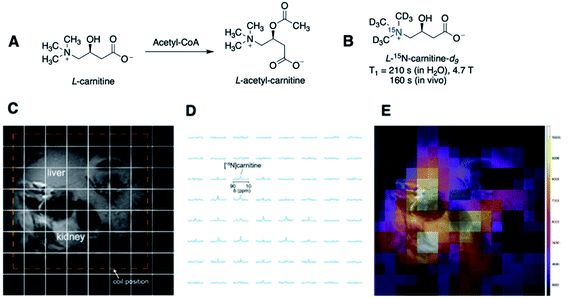 |
| Fig. 5 (A) Structures of endogenous L-carnitine and its acetylated product. (B) T1 lifetimes of L-15N-carnitine-d9 in water and in vivo. (C) Spectral grid used for MR imaging overlaid on the 1H anatomic image. (D) 15N spectra of each spectral grid (E) hyperpolarized 15N-carnitine signals in color overlaid on the anatomic image, illustrating the biodistribution of 15N-carnitine in the liver and kidney. (C–E) Adapted with permission from ref. 42. Copyright 2020, John Wiley and Sons. | |
The 15N-labeled choline, amino acids, and carnitine studies show several benefits of simple isotope-enrichment of endogenous molecules, such as ease of synthesis, high aqueous solubility, and low cytotoxicity. Nonetheless, 15N-labeled endogenous molecules do not present detectable chemical reactions and thus cannot capture real-time physicochemical activities. Discovery of imaging agents that undergo an enzymatic or chemical reaction with significant 15N chemical shift differences will provide even greater analytical appeal in terms of structure determination and quantification.
2.2.4.
15N-Azidothymidine (AZT).
15N-Enrichment of nitrogen-containing drug molecules may offer the capability of monitoring the drug's location and metabolism by HP imaging. A good example of this category is azidothymidine (AZT), an azide-containing antiviral drug that prevents reverse transcriptase from forming viral DNA.43 Shchepin et al. have reported the synthesis of AZT using sodium-15N14N2 azide to yield singly labeled 15N14N2-AZT as a mixture of 1-15N and 3-15N isotopomers. This mixture of 1-15N and 3-15N labeled AZT provided two distinct hyperpolarized 15N NMR peaks.44 SABRE hyperpolarization provided T1 values of 1-15N and 3-15N azides as 45 ± 1 and 37 ± 2 s, respectively (9.4 T) (Fig. 6A). In another study by Bae et al., triply labeled 15N3-AZT and singly labeled 15N14N2-AZT hyperpolarized by d-DNP showed T1 values of 2.5–5.3 min (1 T).45 In this study, the singly labeled 1-15N center was affected by the scalar relaxation with neighboring 14N (I = 1), leading to unmeasurable T1 at 1 T. These studies exemplify the synthesis of 15N-labeled drug molecules with the potential to monitor drug activities.
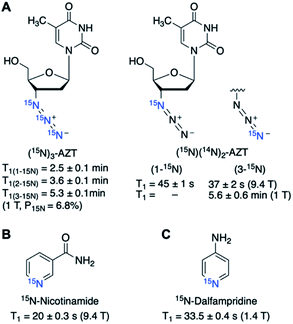 |
| Fig. 6 Structures and hyperpolarized lifetimes of (A) singly and triply labeled 15N-AZT, (B) 15N-nicotinamide, and (C) 15N-dalfampridine. | |
2.2.5.
15N-Nicotinamide and 15N-dalfampridine.
Hyperpolarized 15N-heterocycles have been explored as potential drug contrast agents. Nicotinamide, also known as vitamin B3 amide, is a drug that is used for the treatment of M. tuberculosis, HIV and cancer.46,47 Shchepin et al. have demonstrated an efficient synthesis of 15N-enriched nicotinamide with high isotopic purity.48 SABRE-SHEATH (SHield Enables Alignment Transfer to Heteronuclei) hyperpolarization of 15N-nicotinamide provided a T1 of 20.2 ± 0.3 s (9.4 T), presenting the possibilities of synthesized 15N-heterocycles as hyperpolarized drug contrast agents. Similarly, dalfampridine (4-aminopyridine) is another pyridine-based drug used to treat the symptoms of multiple sclerosis.49 In a study by Chukanov et al., 15N-enriched dalfampridine has been synthesized and hyperpolarized with SABRE-SHEATH to afford a T1 of 33.5 ± 0.4 s (1.4 T).50 These studies illustrate the significance of 15N-enrichment methodology development for biomedical applications.
The feasibility of hyperpolarized 15N-drug imaging is yet to be confirmed with in vivo studies. In addition to hyperpolarization efficiency, factors such as drug metabolism rate, cellular uptake, and cytotoxicity of the probe of interest need to be scrutinized to meet the criteria for preclinical applications.
2.2.6.
15N-Nitrate.
Hyperpolarized 15N-nitrates (15NO3−), bioactive ions that mediate physiological processes, have been explored as contrast agents for HP-MRI. D-DNP hyperpolarization of 15N-nitrate in D2O, H2O and saline provided T1 values of ∼100 s for each solvent at a temperature range of 34–44 °C, which was reduced to a T1 of 29 ± 1 s in blood samples. The metabolic conversion from 15N-nitrate to 15N-nitrite was undetectable in blood and saliva, making this molecular probe suitable as an MR tracer for perfusion or tissue retention imaging.51
2.3.
15N-Labeled molecular sensors for detecting the biological environment
Most nitrogen centers in biomolecules are proton-bound amines or amides, in which hyperpolarized 15N signals would suffer shortened lifetimes, owing to the dipole relaxation pathway. This challenge associated with short T1 has limited the range of HP 15N-labeled endogenous molecules to quaternary permethylated 15N-centers, such as the 15N-choline and amino acid derivatives. However, de novo15N molecular probes not restricted to endogenous biomolecules present great promise as chemical sensors. Several examples of 15N-labeled chemical sensors have been reported so far for the detection of intracellular pH, signaling molecules, and enzymatic activity as potential disease biomarkers.
2.3.1.
15N-Heteroatom bases as pH sensors.
Imbalanced intracellular pH is closely related to pathological processes and is a hallmark for diseases such as cancer.52 Developing pH sensors for effective cancer diagnosis has attracted continuous interest, including isotope-labeled hyperpolarized pH sensors. Several 13C-pH sensors have been developed, such as [1-13C]-bicarbonate53,54 and 13C2-zymonic acid,8 allowing for pH detection via the proton exchange of 13C-carboxylic acids. Alternatively, 15N-based pH sensors have been developed for direct 15N-protonation-based chemical shift imaging because sp2-hydridized, aromatic nitrogen centers can be protonated near physiological pH and cause significant electronic changes in the 15N atom.
Jiang et al. first illustrated hyperpolarized 15N-pyridine and pyridine derivatives as potential pH sensors (Fig. 7A and B).5515N-Pyridine demonstrated pH-sensitive chemical shift changes up to 90 ppm at a pH range of 2.1–8.5. Sharper chemical shift changes were observed in pH near a 15N-pyridine pKa of 5.17, and the pH sensitivity was further altered by adding substituents to the pyridine derivatives. Yet the 15N-pyridines suffered from a short hyperpolarization lifetime, with a T1 value of 41 s for non-protonated 15N-pyridine (pH 8.4) that decreased to 11 s in plasma (9.4 T). The reduced T1 is due to an added relaxation pathway from proton exchange between the 15N atom (H–15N+) and water.
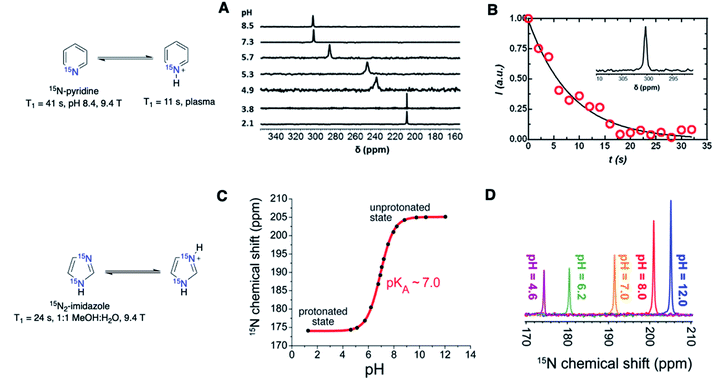 |
| Fig. 7 (A) pH-dependent 15N chemical shifts of free-base and protonated 15N-pyridine. (B) Hyperpolarization signal decay of 15N-pyridine in rat plasma with a T1 value of ∼11 s. (C) Determination of 15N2-imidazole pKa using 15N chemical shifts. (D) Chemical shifts of thermally polarized 15N2-imidazole in water at various pH values. (A and B) Adapted with permission from ref. 55. Copyright 2015, Springer Nature. (C and D) Adapted with permission from ref. 56. | |
A study by Shchepin et al. examined 15N2-imidazole as a pH sensor by SABRE-SHEATH hyperpolarization (Fig. 7C and D).5615N2-Imidazole, with a pKa of ∼7.0, showed higher sensitivity near physiological pH than that of 15N-pyridine, with a chemical shift change of ∼15 ppm within the range of 6.5–7.5 (1.5 ppm/0.1 pH unit). 15N2-Imidazole demonstrated a T1 value of only 24 s in 1
:
1 MeOH
:
H2O (9.4 T). Although the T1 measurements at the physiological pH were not disclosed, 15N2-imidazole is expected to have faster signal decay upon protonation, based on the results from 15N-pyridine. Similarly, a simultaneous hyperpolarization of cleavable 15N2-imidazole and 13C-acetate has been reported, exemplifying the possibility of dual 15N/13C-labeled HP agents for metabolic and pH sensing.57
These studies use isotope-enriched substrates because of the low natural abundance of 15N (0.37%). Notably, high levels of 15N polarization of naturally abundant substrates (i.e., pyridine, metronidazole and acetonitrile) up to P15N = 51% have been achieved using SABRE hyperpolarization in the presence of amines as coligands of the SABRE catalyst. Such a study will allow simple and efficient hyperpolarization of nitrogen-containing pH sensors and relevant biomolecules for 15N-MRI.58
2.3.2.
15N-TMPA for detection of ROS and enzyme activity.
Unlike the above-mentioned 15N-based pH sensors designed with an all-in-one 15N-sensing and signaling unit, the probes can be designed with a separate sensing unit and a signal unit. In this alternative design, the sensing unit surveys a biological system of interest while a remote 15N signaling unit provides chemical shift changes as a readout.
Nonaka et al. exemplified this design strategy in [15N]trimethylphenylammonium (15N-TMPA) as a versatile platform for developing 15N-based sensors that can potentially adapt any sensing of interest.59 At the same time, 15N-TMPA can provide a long polarization lifetime of the quaternary permethylated 15N center, with minimal influence on T1 from the environment. In this study, the 15N-TMPA imaging platform was examined for a reaction-based detection of H2O2 and carboxyl esterase, the representative reactive oxygen species and enzyme commonly elevated in diseases (Fig. 8A and C). Both probes showed H2O2 concentration or enzyme-activity-dependent 15N-chemical shift changes. Deuterated [15N, d9]-TMPA offered a T1 of over ∼7 min (9.4 T). Such a long polarization lifetime allowed for an extended 15N signal detection of up to 40 min, considering that the T1 value is approximately 37% of the total hyperpolarization decay. However, both H2O2 oxidation or carboxyl esterase reaction of [15N, d9]-TMPA resulted in a 15N shift difference of merely ∼1.5 ppm (Fig. 8B and D). Such a small chemical shift difference corresponds to a 15N frequency of only 60 Hz at 9.4 T and even smaller at clinically relevant magnetic fields, with 19 Hz at 3 T and 9.7 Hz at 1.5 T, which would be insufficient for signal distinction. These results suggest that a 15N chemical shift change of larger than 1.5 ppm is needed to distinguish the peaks for accurate analysis of the signals.
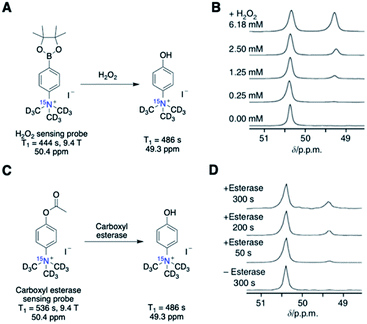 |
| Fig. 8 (A) Scheme of H2O2 detection probe reaction. (B) Scans of the hyperpolarized H2O2 detection probe in the presence of various concentrations of H2O2 (in PBS, 50 s after mixing). (C) Scheme of carboxyl esterase detection probe reaction. (D) Scans of the hyperpolarized carboxyl esterase detection probe in the presence of esterase (125 units mL−1 in PBS). (B and D) Adapted with permission from ref. 59. Copyright 2013, Springer Nature. | |
The excitingly long T1 values in these 15N-based probes significantly broaden the HP imaging possibilities for in vivo characterization of slower biochemical reactions, such as enzymatic reactions, redox activities, and cellular signaling pathways, which would be otherwise challenging with a short signal lifetime of HP 13C-probes.
2.3.3.
15N-Metronidazole and 15N-nimorazole as hypoxia sensors.
15N-Labeled probes for hypoxia sensing have been developed as an imaging model of the tumor microenvironment. Hypoxia, a condition with inadequate oxygen supply in tissues, is a common feature in solid tumors and a diagnostic marker for therapy-resistant tumors.60 Thus, non-invasive and reliable hyperpolarized hypoxia sensors offer valuable tools for cancer diagnosis and predicting therapy efficacy.
Nitroimidazoles have been widely used as hypoxia markers through immunohistochemistry and PET imaging. Under hypoxic conditions, the nitro group of these nitroimidazole compounds is expected to undergo sequential bioreduction to form nitroso, hydroxylamine, and amine derivatives (Fig. 9A). These hypoxia-based reactions can potentially provide significant 15N chemical shift changes and make 15N-nitroimidazoles suitable candidates for MRS/MRI probes. So far, two types of 15N-labeled nitroimidazoles have been investigated as hypoxia sensors.
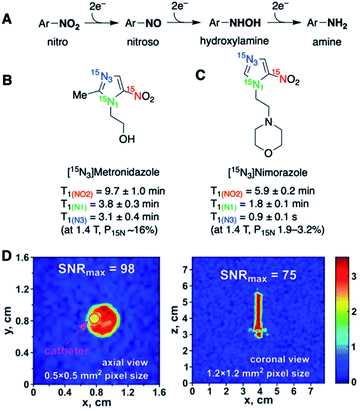 |
| Fig. 9 (A) Schematic illustration of sequential nitro reduction under hypoxic conditions. (B) T1 lifetimes of the three 15N centers in 15N-labeled metronidazole. (C) 15N-Labeled nimorazole as a hyperpolarized imaging agent of hypoxia. (D) 2D sub-second 15N MRI visualization of HP [15N3]nimorazole in a 5 mm NMR tube (9.4 T). Axial (left) and coronal (right) projections of the first scan of 15N MRI. (D) Adapted with permission from ref. 63. Copyright 2020, John Wiley and Sons. | |
Metronidazole is an FDA-approved nitroimidazole-type antibiotic drug. It can be administered safely at high doses, which well suits the use of hyperpolarized solution at high concentrations for HP-MR studies. Efficient hyperpolarization of naturally abundant metronidazole61 as well as 15N-enriched [15N3]-metronidazole62 has been demonstrated using SABRE-SHEATH. In the work by Shchepin et al., all three 15N sites had high polarizations of ∼16% and long polarization lifetimes (Fig. 9B).62 Among the three 15N centers, 15NO2 had an extraordinarily long T1 value of 9.7 min (1.4 T), and the two aromatic 15N-1 and 15N-3 centers in the imidazole ring had T1 values of 3.1 and 3.8 min, respectively. Nimorazole is another imidazole-based radiosensitizer drug for head and neck cancer. [15N3]-Nimorazole has also been studied as a potential HP sensor for tumor hypoxia. Salnikov et al. reported hyperpolarized [15N3]-nimorazole as a potential theranostic agent for dual therapy and imaging of tumor hypoxia (Fig. 9C).63 Hyperpolarization of [15N3]-nimorazole using SABRE-SHEATH provided long T1 lifetimes, especially for 15NO2 (5.9 min, 1.4 T). Such remarkably long-lasting polarizations open opportunities for hyperpolarized hypoxia MR imaging for over tens of minutes.
Although neither of these two studies have reported metabolic imaging of 15N-nitroimidazoles, the ab initio calculations revealed that the sequential hypoxic reduction processes shown in Fig. 9A were expected to provide significant 15N chemical shift differences, with nearly 800 ppm difference for the 15N-nitro center.63 Such a dynamic chemical shift range of the 15N-sites bodes well for future in vivo imaging of nitroimidazole metabolism. One challenge is that the 15NH2 metabolite from hypoxic reduction would deliver a short T1 because of the proton-coupled relaxation pathway. A possible alternative readout to monitor hypoxia is the other two sp2-15N atoms that may also lead to chemical shift changes upon 15NO2 reduction.
While in vivo imaging has not been demonstrated in these studies, the 2D 15N MRI visualization of [15N3]-metronidazole64 and [15N3]-nimorazole63 displayed high spatial and temporal resolution (Fig. 9D), highlighting the prospects of high-resolution 15N-imaging.
2.3.4. Coordination-based detection of Ca2+ and Zn2+ metal ions.
MR probes have also been designed for sensing biologically important metal ions. Free metal ions, such as calcium and zinc, participate in essential cellular ionic signaling cascades and oxidative balance. The importance of metal ion homeostasis suggests the promise of in vivo metal ion concentrations as diagnostic markers for analyzing diseases associated with metal ion imbalance. So far, hyperpolarized sensors for metal ions have been developed by designing chelators that can coordinate to metal ions to induce electron localization and chemical shift changes.
[15N, d9]-TMPA has been studied as a potential sensor for calcium ions (Ca2+). Calcium ions are ubiquitous signaling molecules that control various cellular functions, and abnormal Ca2+ concentrations are responsible for several pathological processes.65 The design of [15N, d9]-TMPA used (CD3)315N+ as the signaling unit and triacetic acid branches as the Ca2+ chelator. Unfortunately, small 15N chemical shift changes up to 1.5 ppm were inadequate for unambiguous Ca2+ detection (Fig. 10A).59 To address this limitation, another Ca2+ sensor 15N-o-aminophenol-N,N,O-triacetic acid (15N-APTRA) was designed with the 15N center positioned close to the Ca2+ coordination site.66 Encouragingly, 15N-APTRA provided chemical shift changes up to 5.2 ppm with the addition of 2 equivalence of Ca2+. On the downside, 15N-APTRA showed a T1 of only 37 s (pH 7.4, 9.4 T), a 3.5-fold decrease from T1 = 130 s of [15N, d9]-TMPA, presumably from protonation of 15N-aniline (Fig. 10B).
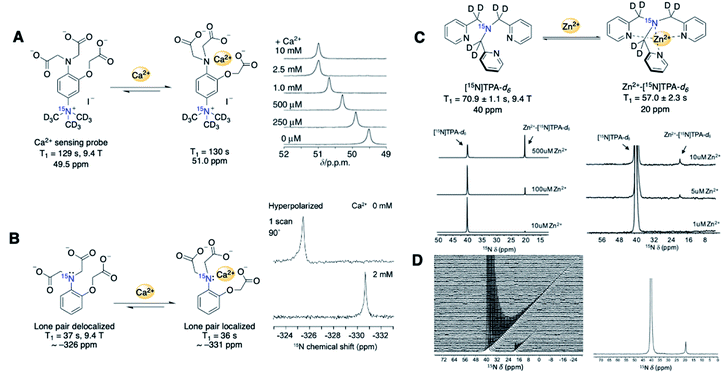 |
| Fig. 10 (A) 15N-TMPA based Ca2+ detection probe and Ca2+ level-dependent 15N chemical shifts (measured in HEPES buffer, 40 s after mixing). (B) 15N-APTRA based Ca2+ detection probe and 15N NMR spectra with and without Ca2+. (C) [15N]TPA-d6 based Zn2+ detection probe and 15N NMR spectra of hyperpolarized [15N]TPA-d6 (1.2 mM) with various concentrations of Zn2+ (1–500 μM). (D) Time-dependent 15N spectra collected using intact PNT1A cells after addition of 2.8 mM of HP-[15N]TPA-d6 (left) and its first 15N spectrum showing the detection of in vitro Zn2+ (right) (pH 7.4, 9.4 T). (A) Adapted with permission from ref. 59. Copyright 2013, Springer Nature. (B) Adapted with permission from ref. 66. Copyright 2015, The Royal Society of Chemistry. (C–E) Adapted with permission from ref. 69. Copyright 2020, Springer Nature. | |
15N-Labeled sensors for Zn2+ metals have also been reported. Elevated cellular Zn2+ levels are highly toxic and linked to cancer and neurodegenerative disorders. Imaging labile zinc ions as biomarkers presents a promising approach for diagnosing these diseases.67,6815N-labeled tris(2-pyridylmethyl)amine (TPA) was developed by Suh et al. using chemical shift changes resulting from pyridine–Zn2+ coordination for the detection and quantification of free Zn2+ metal (Fig. 10C).6915N-TPA showed several promising spectral features, including a favorable 15N signal linewidth, a large chemical shift of 20 ppm, and a linear relationship of peak area to zinc concentration. T1 values for [15N]TPA-d6 and Zn2+-[15N]TPA-d6 were 71 s and 57 s, respectively (9.4 T). Excitingly, the hyperpolarized [15N]TPA-d6 probe was able to measure physiological levels of Zn2+ (0–200 μM) in human prostate tissue homogenate and intact human prostate epithelial cells (Fig. 10D).
These studies show versatile 15N design principles through metal–ligand coordination-based chemical shift changes for hyperpolarized imaging of labile metal ions. Although a limited number of 15N probes have been developed so far, these studies of exogenous 15N sensors provide valuable lessons, including the effects of 15N center placement on chemical shifts and T1 values. These principles will expedite the design of more effective 15N-molecular imaging probes in future studies.
2.4.
15N-Molecular tags and biomolecules
An alternative to isotope enrichment, an attractive new strategy in designing HP 15N-labeled agents, is to install biocompatible and long-lasting polarized 15N-molecular tags onto biologically relevant molecules. Such a molecular tagging strategy can potentially introduce a 15N-signaling moiety into any target of interest. In the probes mentioned above, long-lived 15N signals rely on permethylated 15N-ammonium or 15N-heterocycles as the common 15N-centers. In comparison, the 15N-molecular tags can constitute various nitrogen-containing functional groups that are non-proton bound and symmetrical, selected for optimal polarization efficiency and long-lived polarization states.
2.4.1.
15N2-Diazirine tags.
15N2-Diazirines are one of the first 15N-based molecular tags explored for HP MRS/MRI.70 Structurally, 15N2-diazirines are three-membered rings containing a nitrogen–nitrogen double bond (Fig. 11). Diazirines have desirable physicochemical properties for a molecular tag, including small size, biocompatibility and stability under physiological conditions, and minimal effects on the physicochemical properties of biomolecules.71,72 Particularly attractive for HP-MR detection, 15N2-diazirines have a unique symmetrical molecular structure that stores polarization for an extended period through a singlet state. The singlet state (Ts) has a zero magnetic moment, so the symmetry has to be broken to be NMR-detectable. This also means that the singlet spin order is immune to many relaxation mechanisms and polarization is long-lived. In particular, SABRE-SHEATH hyperpolarization of 15N2-diazirine-labeled compounds had a long singlet relaxation of Ts = 23 min.70 Furthermore, several 15N2-diazirine-labeled biomolecules have been hyperpolarized by SABRE-SHEATH73 and d-DNP74 methods. Examples include the 15N2-diazirine tagged analogs of amino acids, glucose, and drug molecules. Hyperpolarization by d-DNP showed that all provided T1 values in the 3–4 min range (1 T) (Fig. 11). The study showed the considerable influence of the solubility of the 15N-tagged molecules on their hyperpolarization efficiencies. High solubility of the hyperpolarized probes in the aqueous glassing solvent (at least 100 mM) is crucial for effective hyperpolarization of non-polar endogenous or drug molecules for practical applications.
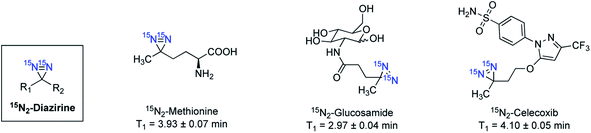 |
| Fig. 11 Selected examples of 15N2-diazirine-tagged endogenous and drug molecules. Hyperpolarized with d-DNP and all T1 lifetimes were measured at 1 T. | |
2.4.2.
15N3-Azide tags.
Azides, unique linear species containing three nitrogen atoms, have been known as bioorthogonal reactive partners and possess desired features for a molecular tag.75,7615N-Azides have been demonstrated as another class of 15N-molecular tags for hyperpolarized imaging by Bae et al.45 Triply labeled 15N3-azides have been incorporated into choline, glucose, and tyrosine analogs for investigation. Hyperpolarization of all these 15N3-tagged molecules by d-DNP demonstrated long lifetimes up to 9.8 min (1 T) (Fig. 12). The terminal nitrogen, 15Nγ, retained the longest HP signal, followed by 15Nβ and 15Nα, in which the long T1 corresponds to increased distance from the nearest protons. The 15N3-azide tag is especially interesting as three distinct 15N signals can be monitored simultaneously. Additionally, the extended imaging time window opens possibilities for 15N3-azide bioconjugation reaction in vivo (i.e., azide–alkyne cycloaddition) for hyperpolarized secondary labelling.
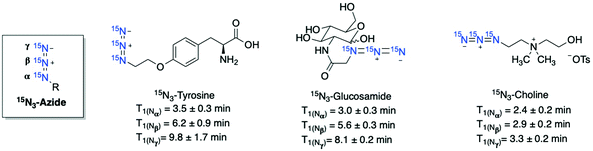 |
| Fig. 12 Selected examples of 15N3-azide-tagged endogenous and drug molecules. Hyperpolarized with d-DNP and all T1 lifetimes were measured at 1 T. | |
The 15N-tagging strategy demonstrated in 15N-azide and 15N-diazirine compounds will broaden the application of HP 15N imaging beyond nitrogen centers in heteroarenes and permethylated amines. Moreover, these 15N-tag motifs can be readily introduced into a broad range of biomolecules, allowing for preparing a variety of hyperpolarized imaging probes with a long polarization lifetime. Of note, in some examples where the 15N-tags are generally installed several bonds away from the metabolic sites, significant 15N chemical shift changes may not be observed upon metabolism. Nevertheless, the 15N-azide and 15N-diazirine-tagged molecules are of great interest for future studies on their applications in monitoring cellular uptake and accumulation.
3. Summary and outlook
This review provides the current state of development of HP 15N-probes, including their hyperpolarization performances in relation to design principles. As an emerging molecular imaging technique, hyperpolarized 15N MRS/MRI shows promising potential for biomedical applications. Several 15N-labeled endogenous and de novo molecular probes delivered long hyperpolarization lifetimes in the order of several minutes. Such substantial hyperpolarization lifetimes allow an extended imaging period to capture slower biochemical reactions that are useful for disease diagnosis. At the same time, long T1 lifetimes of HP 15N agents can compensate for the low sensitivity issues, as shown in the MRS/MRI scans of 15N-amino acids and 15N-carnitine acquired over several minutes.
Despite recent progress and increased interest in 15N-based imaging in the past decade, hyperpolarized 15N MR has not gained widespread use to enter the preclinical stage. As reflected in the analysis of currently studied HP 15N-probes in this review, advancing 15N MRS/MRI into a practical imaging tool requires advancements in multiple aspects such as new probe design, extensive animal imaging studies, and improved MR technology.
Fundamental considerations for the design of novel 15N-probes include the factors of T1 lifetime, chemical shift differences, and toxicity. First, the discussion on the reported 15N probes in this review reveals that the 15N signal lifetime can be greatly extended by the probe design to reduce dipole-relaxation pathways (i.e., deuteration of neighboring protons). Compared to commonly observed 13C carbonyl centers, the 15N centers of the HP probes in the literature have greater structural diversity, such as quaternary amine, diazirine, and azides. All these 15N centers warrant a long T1 lifetime. So far, most studies have presented polarization lifetimes at high B0 (7–11.7 T). Future work on demonstrating T1 in clinically relevant magnetic fields (1–3 T) will be important to accurately predict the performances of HP 15N probes in in vivo imaging. Second, accurate measurement of chemical reactions would require significant chemical shift differences. A serviceable chemical shift difference needed for HP imaging is affected by the magnetic field, polarization levels, and spectral resolution. Finally, the probe candidates must be biocompatible and non-toxic in living systems. The cytotoxicity profiling is critical for exogenous 15N-molecular agents, especially at high concentrations (mM range). The current exogenous 15N-probes solely demonstrate spectroscopic analysis, and only endogenous compounds (i.e., 15N-choline) have advanced to in vivo MRI studies.
Extensive characterization of 15N-labeled agents must be performed to understand the potential use of hyperpolarized 15N imaging in clinical studies. Cellular experiments of 15N-labeled HP-NMR agents can provide information on the membrane permeability of probes and cellular reaction kinetics. Additionally, in vivo imaging should be conducted to validate the hyperpolarization measurements and sensitivity threshold of the 15N probes. So far, most studies have demonstrated MRS experiments. The conjunction of MRS with MRI in small animal model imaging is desirable, which will provide not only pharmacokinetic data to quantify the rate of substrate buildup and metabolite conversion but also anatomical distribution of 15N signals for accurate and quantitative analysis in preclinical studies.
Developing hyperpolarized 15N imaging for preclinical studies requires addressing several technical challenges of MR scanners' technical challenges. For instance, 15N imaging requires dedicated 15N radiofrequency coils, which are not widely available in conventional MR scanners.69 Parallel efforts in improving pulse sequences and multichannel coils may be crucial. Advances in hyperpolarization techniques can increase polarization efficiency and address the sensitivity issues associated with 15N imaging.
Overall, the insights into the chemical and physical properties of 15N-molecular probes gained through the up-to-date examples will assist in more effective designs for future hyperpolarized 15N-based probes. Along with the advancement in MRI/MRS techniques, emerging next-generation probes are expected to foster hyperpolarized 15N-sensors as widespread molecular imaging technology in the future.
Author contributions
Both H. P. and Q. W. contribute to the writing of this manuscript.
Conflicts of interest
There are no conflicts to declare.
Acknowledgements
This work was supported by NIH (R21EB024824 and P30CA014236), and the Camille and Henry Dreyfus Foundation. The content is solely the responsibility of the authors and does not necessarily represent the official views of the NIH.
Notes and references
-
B. L. Hou and J. Hu, in Tumor Biomarker Discovery: Methods and Protocols, ed. M. A. Tainsky, Humana Press, Totowa, NJ, 2009, pp. 297–314, DOI: DOI:10.1007/978-1-60327-811-9_21
.
- J. H. Hwang and C. S. Choi, Use of in vivo magnetic resonance spectroscopy for studying metabolic diseases, Exp. Mol. Med., 2015, 47, e139 CrossRef CAS PubMed
.
- M. van der Graaf,
In vivo magnetic resonance spectroscopy: basic methodology and clinical applications, Eur. Biophys., 2010, 39, 527–540 CrossRef CAS PubMed
.
- R. K. Harris, E. D. Becker, S. M. Cabral de Menezes, R. Goodfellow and P. Granger, NMR Nomenclature: Nuclear Spin Properties and Conventions for Chemical Shifts: IUPAC Recommendations, 2001, Solid State Nucl. Magn. Reson., 2002, 22, 458–483 CrossRef CAS PubMed
.
- H. Gutte, A. E. Hansen, H. H. Johannesen, A. E. Clemmensen, J. H. Ardenkjær-Larsen, C. H. Nielsen and A. Kjær, The use of dynamic nuclear polarization 13C-pyruvate MRS in cancer, Am. J. Nucl. Med. Mol. Imaging, 2015, 5, 548–560 CAS
.
- P. Nikolaou, B. M. Goodson and E. Y. Chekmenev, NMR Hyperpolarization Techniques for Biomedicine, Chem.–Eur. J, 2015, 21, 3156–3166 CrossRef CAS PubMed
.
- A. Comment, Dissolution DNP for in vivo preclinical studies, J. Magn. Reson., 2016, 264, 39–48 CrossRef CAS PubMed
.
- J. H. Ardenkjaer-Larsen, B. Fridlund, A. Gram, G. Hansson, L. Hansson, M. H. Lerche, R. Servin, M. Thaning and K. Golman, Increase in signal-to-noise ratio of > 10,000 times in liquid-state NMR, Proc. Natl. Acad. Sci., 2003, 100, 10158–10163 CrossRef CAS PubMed
.
- J. B. Hövener, A. N. Pravdivtsev, B. Kidd, C. R. Bowers, S. Glöggler, K. V. Kovtunov, M. Plaumann, R. Katz-Brull, K. Buckenmaier, A. Jerschow, F. Reineri, T. Theis, R. V. Shchepin, S. Wagner, P. Bhattacharya, N. M. Zacharias and E. Y. Chekmenev, Parahydrogen-Based Hyperpolarization for Biomedicine, Angew. Chem., Int. Ed., 2018, 57, 11140–11162 CrossRef PubMed
.
- S. Glöggler, J. Colell and S. Appelt, Para-hydrogen perspectives in hyperpolarized NMR, J. Magn. Reson., 2013, 235, 130–142 CrossRef PubMed
.
- S. B. Duckett and R. E. Mewis, Application of Parahydrogen Induced Polarization Techniques in NMR Spectroscopy and Imaging, Acc. Chem. Res., 2012, 45, 1247–1257 CrossRef CAS PubMed
.
- R. W. Adams, J. A. Aguilar, K. D. Atkinson, M. J. Cowley, P. I. P. Elliott, S. B. Duckett, G. G. R. Green, I. G. Khazal, J. López-Serrano and D. C. Williamson, Reversible Interactions with para-Hydrogen Enhance NMR Sensitivity by Polarization Transfer, Science, 2009, 323, 1708 CrossRef CAS PubMed
.
- M. L. Truong, T. Theis, A. M. Coffey, R. V. Shchepin, K. W. Waddell, F. Shi, B. M. Goodson, W. S. Warren and E. Y. Chekmenev,
15N Hyperpolarization by Reversible Exchange Using SABRE-SHEATH, J. Phys. Chem. C, 2015, 119, 8786–8797 CrossRef CAS PubMed
.
- T. Theis, M. L. Truong, A. M. Coffey, R. V. Shchepin, K. W. Waddell, F. Shi, B. M. Goodson, W. S. Warren and E. Y. Chekmenev, Microtesla SABRE enables 10% nitrogen-15 nuclear spin polarization, J. Am. Chem. Soc., 2015, 137, 1404–1407 CrossRef CAS PubMed
.
- K. V. Kovtunov, E. V. Pokochueva, O. G. Salnikov, S. F. Cousin, D. Kurzbach, B. Vuichoud, S. Jannin, E. Y. Chekmenev, B. M. Goodson, D. A. Barskiy and I. V. Koptyug, Hyperpolarized NMR Spectroscopy: d-DNP, PHIP, and SABRE Techniques, Asian J. Chem., 2018, 13, 1857–1871 CrossRef CAS PubMed
.
- M. E. Halse, Perspectives for hyperpolarisation in compact NMR, TrAC, Trends Anal. Chem., 2016, 83, 76–83 CrossRef CAS
.
- G. Zhang and C. Hilty, Applications of dissolution dynamic nuclear polarization in chemistry and biochemistry, Magn. Reson. Chem., 2018, 56, 566–582 CrossRef CAS PubMed
.
- K. Chen and X. Chen, Design and development of molecular imaging probes, Curr. Top. Med. Chem., 2010, 10, 1227–1236 CrossRef CAS PubMed
.
- R. Weissleder and U. Mahmood, Molecular imaging, Radiology, 2001, 219, 316–333 CrossRef CAS PubMed
.
- K. R. Keshari and D. M. Wilson, Chemistry and biochemistry of 13C hyperpolarized magnetic resonance using dynamic nuclear polarization, Chem. Soc. Rev., 2014, 43, 1627–1659 RSC
.
- Z. J. Wang, M. A. Ohliger, P. E. Z. Larson, J. W. Gordon, R. A. Bok, J. Slater, J. E. Villanueva-Meyer, C. P. Hess, J. Kurhanewicz and D. B. Vigneron, Hyperpolarized 13C MRI: State of the Art and Future Directions, Radiology, 2019, 291, 273–284 CrossRef PubMed
.
- R. E. Hurd, Y. F. Yen, A. Chen and J. H. Ardenkjaer-Larsen, Hyperpolarized 13C metabolic imaging using dissolution dynamic nuclear polarization, J. Magn. Reson. Imaging, 2012, 36, 1314–1328 CrossRef PubMed
.
- A. Comment and M. E. Merritt, Hyperpolarized Magnetic Resonance as a Sensitive Detector of Metabolic Function, Biochem, 2014, 53, 7333–7357 CrossRef CAS PubMed
.
- K. M. Brindle, Imaging Metabolism with Hyperpolarized 13C-Labeled Cell Substrates, J. Am. Chem. Soc., 2015, 137, 6418–6427 CrossRef CAS PubMed
.
- J. Kurhanewicz, D. B. Vigneron, J. H. Ardenkjaer-Larsen, J. A. Bankson, K. Brindle, C. H. Cunningham, F. A. Gallagher, K. R. Keshari, A. Kjaer, C. Laustsen, D. A. Mankoff, M. E. Merritt, S. J. Nelson, J. M. Pauly, P. Lee, S. Ronen, D. J. Tyler, S. S. Rajan, D. M. Spielman, L. Wald, X. Zhang, C. R. Malloy and R. Rizi, Hyperpolarized 13C MRI: Path to Clinical Translation in Oncology, Neoplasia, 2019, 21, 1–16 CrossRef PubMed
.
- Y. Kondo, H. Nonaka, Y. Takakusagi and S. Sando, Design of Nuclear Magnetic Resonance Molecular Probes for Hyperpolarized Bioimaging, Angew. Chem., Int. Ed., 2021, 60, 14779–14799 CrossRef CAS PubMed
.
- R. L. Hesketh and K. M. Brindle, Magnetic resonance imaging of cancer metabolism with hyperpolarized 13C-labeled cell metabolites, Curr. Opin. Chem. Biol., 2018, 45, 187–194 CrossRef CAS PubMed
.
- S. Meier, P. R. Jensen, M. Karlsson and M. H. Lerche, Hyperpolarized NMR Probes for Biological Assays, Sensors, 2014, 14, 1576–1597 CrossRef CAS PubMed
.
- M. Witanowski, Nitrogen nmr spectroscopy, Pure Appl. Chem., 1974, 37, 225–233 CAS
.
- N. V. Chukanov, R. V. Shchepin, S. M. Joshi, M. S. H. Kabir, O. G. Salnikov, A. Svyatova, I. V. Koptyug, J. G. Gelovani and E. Y. Chekmenev, Synthetic Approaches for 15N-Labeled Hyperpolarized Heterocyclic Molecular Imaging Agents for 15N NMR Signal Amplification by Reversible Exchange in Microtesla Magnetic Fields, Chem.–Eur. J., 2021, 27, 9727–9736 CrossRef CAS PubMed
.
- E. Ackerstaff, K. Glunde and Z. M. Bhujwalla, Choline phospholipid metabolism: A target in cancer cells, J. Cell. Biochem., 2003, 90, 525–533 CrossRef CAS PubMed
.
- F. Podo, Tumour phospholipid metabolism, NMR Biomed., 1999, 12, 413–439 CrossRef CAS PubMed
.
- C. Gabellieri, S. Reynolds, A. Lavie, G. S. Payne, M. O. Leach and T. R. Eykyn, Therapeutic Target Metabolism Observed Using Hyperpolarized 15N Choline, J. Am. Chem. Soc., 2008, 130, 4598–4599 CrossRef CAS PubMed
.
- H. Allouche-Arnon, A. Gamliel, C. M. Barzilay, R. Nalbandian, J. M. Gomori, M. Karlsson, M. H. Lerche and R. Katz-Brull, A hyperpolarized choline molecular probe for monitoring acetylcholine synthesis, Contrast Media Mol. Imaging, 2011, 6, 139–147 CrossRef CAS PubMed
.
- C. Cudalbu, A. Comment, F. Kurdzesau, R. B. van Heeswijk, K. Uffmann, S. Jannin, V. Denisov, D. Kirik and R. Gruetter, Feasibility of in vivo15N MRS detection of hyperpolarized 15N labeled choline in rats, Phys. Chem. Chem. Phys., 2010, 12, 5818–5823 RSC
.
- L. J. Friesen-Waldner, T. P. Wade, K. Thind, A. P. Chen, J. M. Gomori, J. Sosna, C. A. McKenzie and R. Katz-Brull, Hyperpolarized choline as an MR imaging molecular probe: Feasibility of in vivo imaging in a rat model, J. Magn. Reson. Imaging, 2015, 41, 917–923 CrossRef PubMed
.
- R. Sarkar, A. Comment, P. R. Vasos, S. Jannin, R. Gruetter, G. Bodenhausen, H. Hall, D. Kirik and V. P. Denisov, Proton NMR of 15N-Choline Metabolites Enhanced by Dynamic Nuclear Polarization, J. Am. Chem. Soc., 2009, 131, 16014–16015 CrossRef CAS PubMed
.
- K. Kumagai, K. Kawashima, M. Akakabe, M. Tsuda, T. Abe and M. Tsuda, Synthesis and hyperpolarized 15N NMR studies of 15N-choline-d13, Tetrahedron, 2013, 69, 3896–3900 CrossRef CAS
.
- L. B. Bales, K. V. Kovtunov, D. A. Barskiy, R. V. Shchepin, A. M. Coffey, L. M. Kovtunova, A. V. Bukhtiyarov, M. A. Feldman, V. I. Bukhtiyarov, E. Y. Chekmenev, I. V. Koptyug and B. M. Goodson, Aqueous, Heterogeneous para-Hydrogen-Induced 15N Polarization, J. Phys. Chem. C, 2017, 121, 15304–15309 CrossRef CAS PubMed
.
- E. Chiavazza, A. Viale, M. Karlsson and S. Aime,
15N-Permethylated amino acids as efficient probes for MRI-DNP applications, Contrast Media Mol. Imaging, 2013, 8, 417–421 CrossRef CAS PubMed
.
- M. Durst, E. Chiavazza, A. Haase, S. Aime, M. Schwaiger and R. F. Schulte, alpha-trideuteromethyl[15N]glutamine: A long-lived hyperpolarized perfusion marker, Magn. Reson. Med., 2016, 76, 1900–1904 CrossRef CAS PubMed
.
- C. von Morze, J. A. Engelbach, G. D. Reed, A. P. Chen, J. D. Quirk, T. Blazey, R. Mahar, C. R. Malloy, J. R. Garbow and M. E. Merritt,
15N-carnitine, a novel endogenous hyperpolarized MRI probe with long signal lifetime, Magn. Reson. Med., 2021, 85, 1814–1820 CrossRef CAS PubMed
.
- H. Mitsuya, K. J. Weinhold, P. A. Furman, M. H. St Clair, S. N. Lehrman, R. C. Gallo, D. Bolognesi, D. W. Barry and S. Broder, 3'-Azido-3'-deoxythymidine (BW A509U): an antiviral agent that inhibits the infectivity and cytopathic effect of human T-lymphotropic virus type III/lymphadenopathy-associated virus in vitro, PNAS, 1985, 82, 7096–7100 CrossRef CAS PubMed
.
- R. V. Shchepin and E. Y. Chekmenev, Toward hyperpolarized molecular imaging of HIV: synthesis and longitudinal relaxation properties of 15N-Azidothymidine, J. Labelled Compd. Radiopharm., 2014, 57, 621–624 CrossRef CAS PubMed
.
- J. Bae, G. Zhang, H. Park, W. S. Warren and Q. Wang,
15N-Azides as practical and effective tags for developing long-lived hyperpolarized agents, Chem. Sci., 2021, 12, 14309–14315 RSC
.
- M. F. Murray, Nicotinamide: An Oral Antimicrobial Agent with Activity against Both Mycobacterium tuberculosis and Human Immunodeficiency Virus, Clin. Infect. Dis., 2003, 36, 453–460 CrossRef CAS PubMed
.
- A. C. Chen, A. J. Martin, B. Choy, P. Fernández-Peñas, R. A. Dalziell, C. A. McKenzie, R. A. Scolyer, H. M. Dhillon, J. L. Vardy, A. Kricker, G. St. George, N. Chinniah, G. M. Halliday and D. L. Damian, A Phase 3 Randomized Trial of Nicotinamide for Skin-Cancer Chemoprevention, N. Engl. J. Med., 2015, 373, 1618–1626 CrossRef CAS PubMed
.
- R. V. Shchepin, D. A. Barskiy, D. M. Mikhaylov and E. Y. Chekmenev, Efficient Synthesis of Nicotinamide-1-15N for Ultrafast NMR Hyperpolarization Using Parahydrogen, Bioconjugate Chem., 2016, 27, 878–882 CrossRef CAS PubMed
.
- J. Dunn and A. Blight, Dalfampridine: a brief review of its mechanism of action and efficacy as a treatment to improve walking in patients with multiple sclerosis, Curr. Med. Res. Opin., 2011, 27, 1415–1423 CrossRef CAS PubMed
.
- N. V. Chukanov, O. G. Salnikov, I. A. Trofimov, M. S. H. Kabir, K. V. Kovtunov, I. V. Koptyug and E. Y. Chekmenev, Synthesis and 15N NMR Signal Amplification by Reversible Exchange of [15N]Dalfampridine at Microtesla Magnetic Fields, Chemphyschem, 2021, 22, 960–967 CrossRef CAS PubMed
.
- A. Gamliel, S. Uppala, G. Sapir, T. Harris, A. Nardi-Schreiber, D. Shaul, J. Sosna, J. M. Gomori and R. Katz-Brull, Hyperpolarized [15N]nitrate as a potential long lived hyperpolarized contrast agent for MRI, J. Magn. Reson., 2019, 299, 188–195 CrossRef CAS PubMed
.
- I. F. Tannock and D. Rotin, Acid pH in Tumors and Its Potential for Therapeutic Exploitation, Cancer Res., 1989, 49, 4373 CAS
.
- F. A. Gallagher, M. I. Kettunen, S. E. Day, D.-E. Hu, J. H. Ardenkjær-Larsen, R. i. t. Zandt, P. R. Jensen, M. Karlsson, K. Golman, M. H. Lerche and K. M. Brindle, Magnetic resonance imaging of pH in vivo using hyperpolarized 13C-labelled bicarbonate, Nature, 2008, 453, 940 CrossRef CAS PubMed
.
- D. E. Korenchan, R. R. Flavell, C. Baligand, R. Sriram, K. Neumann, S. Sukumar, H. VanBrocklin, D. B. Vigneron, D. M. Wilson and J. Kurhanewicz, Dynamic nuclear polarization of biocompatible 13C-enriched carbonates for in vivo pH imaging, Chem. Commun., 2016, 52, 3030–3033 RSC
.
- W. Jiang, L. Lumata, W. Chen, S. Zhang, Z. Kovacs, A. D. Sherry and C. Khemtong, Hyperpolarized 15N-pyridine Derivatives as pH-Sensitive MRI Agents, Sci. Rep., 2015, 5, 9104 CrossRef PubMed
.
- R. V. Shchepin, D. A. Barskiy, A. M. Coffey, T. Theis, F. Shi, W. S. Warren, B. M. Goodson and E. Y. Chekmenev, N-15 Hyperpolarization of Imidazole-N-15(2) for Magnetic Resonance pH Sensing via SABRE-SHEATH, ACS Sens., 2016, 1, 640–644 CrossRef CAS PubMed
.
- B. E. Kidd, J. A. Mashni, M. N. Limbach, F. Shi, E. Y. Chekmenev, Y. Hou and B. M. Goodson, Toward Cleavable Metabolic/pH Sensing “Double Agents” Hyperpolarized by NMR Signal Amplification by Reversible Exchange, Chem.–Eur. J., 2018, 24, 10641–10645 CrossRef CAS PubMed
.
- M. Fekete, F. Ahwal and S. B. Duckett, Remarkable Levels of 15N Polarization Delivered through SABRE into Unlabeled Pyridine, Pyrazine, or Metronidazole Enable Single Scan NMR Quantification at the mM Level, J. Phys. Chem. B, 2020, 124, 4573–4580 CrossRef CAS PubMed
.
- H. Nonaka, R. Hata, T. Doura, T. Nishihara, K. Kumagai, M. Akakabe, M. Tsuda, K. Ichikawa and S. Sando, A platform for designing hyperpolarized magnetic resonance chemical probes, Nat. Commun., 2013, 4, 2411 CrossRef PubMed
.
- W. R. Wilson and M. P. Hay, Targeting hypoxia in cancer therapy, Nat. Rev. Cancer, 2011, 11, 393–410 CrossRef CAS PubMed
.
- D. A. Barskiy, R. V. Shchepin, A. M. Coffey, T. Theis, W. S. Warren, B. M. Goodson and E. Y. Chekmenev, Over 20% 15N Hyperpolarization in Under One Minute for Metronidazole, an Antibiotic and Hypoxia Probe, J. Am. Chem. Soc., 2016, 138, 8080–8083 CrossRef CAS PubMed
.
- R. V. Shchepin, J. R. Birchall, N. V. Chukanov, K. V. Kovtunov, I. V. Koptyug, T. Theis, W. S. Warren, J. G. Gelovani, B. M. Goodson, S. Shokouhi, M. S. Rosen, Y.-F. Yen, W. Pham and E. Y. Chekmenev, Hyperpolarizing Concentrated Metronidazole 15NO2 Group over Six Chemical Bonds with More than 15% Polarization and a 20 Minute Lifetime, Chem.–Eur. J., 2019, 25, 8829–8836 CrossRef CAS PubMed
.
- O. G. Salnikov, N. V. Chukanov, A. Svyatova, I. A. Trofimov, M. S. H. Kabir, J. G. Gelovani, K. V. Kovtunov, I. V. Koptyug and E. Y. Chekmenev,
15N NMR Hyperpolarization of Radiosensitizing Antibiotic Nimorazole by Reversible Parahydrogen Exchange in Microtesla Magnetic Fields, Angew. Chem., Int. Ed., 2021, 60, 2406–2413 CrossRef CAS PubMed
.
- J. R. Birchall, M. S. H. Kabir, O. G. Salnikov, N. V. Chukanov, A. Svyatova, K. V. Kovtunov, I. V. Koptyug, J. G. Gelovani, B. M. Goodson, W. Pham and E. Y. Chekmenev, Quantifying the effects of quadrupolar sinks via15N relaxation dynamics in metronidazoles hyperpolarized via SABRE-SHEATH, Chem. Commun., 2020, 56, 9098–9101 RSC
.
- H. P. Cheng, S. Wei, L. P. Wei and A. Verkhratsky, Calcium signaling in physiology and pathophysiology, Acta Pharmacol. Sin., 2006, 27, 767–772 CrossRef CAS PubMed
.
- R. Hata, H. Nonaka, Y. Takakusagi, K. Ichikawa and S. Sando, Design of a hyperpolarized 15N NMR probe that induces a large chemical-shift change upon binding of calcium ions, Chem. Commun., 2015, 51, 12290–12292 RSC
.
- C. J. Frederickson, J.-Y. Koh and A. I. Bush, The neurobiology of zinc in health and disease, Nat. Rev. Neurosci., 2005, 6, 449–462 CrossRef CAS PubMed
.
- L. De Leon-Rodriguez, A. J. M. Lubag and A. Dean Sherry, Imaging free zinc levels in vivo – What can be learned?, Inorg. Chim. Acta, 2012, 393, 12–23 CrossRef CAS PubMed
.
- E. H. Suh, J. M. Park, L. Lumata, A. D. Sherry and Z. Kovacs, Hyperpolarized 15N-labeled, deuterated tris(2-pyridylmethyl)amine as an MRI sensor of freely available Zn2+, Commun. Chem., 2020, 3, 185 CrossRef CAS PubMed
.
- T. Theis, G. X. Ortiz Jr, A. W. Logan, K. E. Claytor, Y. Feng, W. P. Huhn, V. Blum, S. J. Malcolmson, E. Y. Chekmenev, Q. Wang and W. S. Warren, Direct and cost-efficient hyperpolarization of long-lived nuclear spin states on universal 15N2-diazirine molecular tags, Sci. Adv., 2016, 2, e1501438 CrossRef PubMed
.
- J. R. Hill and A. A. B. Robertson, Fishing for drug targets: a focus on diazirine photoaffinity probe synthesis, J. Med. Chem., 2018, 61, 6945–6963 CrossRef CAS PubMed
.
- L. Dubinsky, B. P. Krom and M. M. Meijler, Diazirine based photoaffinity labeling, Bioorg. Med. Chem., 2012, 20, 554–570 CrossRef CAS PubMed
.
- K. Shen, A. W. J. Logan, J. F. P. Colell, J. Bae, G. X. Ortiz Jr, T. Theis, W. S. Warren, S. J. Malcolmson and Q. Wang, Diazirines as potential molecular imaging tags: probing the requirements for efficient and long-lived SABRE-induced hyperpolarization, Angew. Chem., Int. Ed., 2017, 56, 12112–12116 CrossRef CAS PubMed
.
- H. Park, G. Zhang, J. Bae, T. Theis, W. S. Warren and Q. Wang, Application of 15N2-Diazirines as a Versatile Platform for Hyperpolarization of Biological Molecules by d-DNP, Bioconjugate Chem., 2020, 31, 537–541 CrossRef CAS PubMed
.
- N. J. Agard, J. M. Baskin, J. A. Prescher, A. Lo and C. R. Bertozzi, A comparative study of bioorthogonal reactions with azides, ACS Chem. Biol., 2006, 1, 644–648 CrossRef CAS PubMed
.
- S. Brase, C. Gil, K. Knepper and V. Zimmermann, Organic azides: An exploding diversity of a unique class of compounds, Angew. Chem., Int. Ed., 2005, 44, 5188–5240 CrossRef CAS PubMed
.
|
This journal is © The Royal Society of Chemistry 2022 |
Click here to see how this site uses Cookies. View our privacy policy here.