DOI:
10.1039/D2AN01719A
(Paper)
Analyst, 2023,
148, 262-268
Confined surface-enhanced indole cation-radical cyclization studied by mass spectrometry†
Received
20th October 2022
, Accepted 26th November 2022
First published on 12th December 2022
Abstract
Reactions in confined spaces exhibit unique reactivity, while how the confinement effect enhances reactions remains unclear. Herein, the reaction in the confined space of a nanopipette reactor was examined by in situ nano-electrospray mass spectrometry (nanoESI-MS). The indole cation-radical cyclization was selected as the model reaction, catalyzed by a common visible-light-harvesting complex Ru(bpz)3(PF6)2 (1% eq.) rather than traditional harsh reaction conditions (high temperature or pressure, etc.). As demonstrated by in situ nanoESI-MS, this reaction was readily promoted in the nanopipette under mild conditions, while it was inefficient in both normal flasks and microdroplets. Both experimental and theoretical evidence demonstrated the formation of concentrated Ru(II)-complexes on the inner surface of the nanopipette, which facilitated the accelerated reactions. As a result, dissociative reactive cation radicals with lower HOMO–LUMO gap were generated from the Ru(II)-complexes by ligand-to-metal charge transfer (LMCT). Furthermore, the crucial cation radical intermediates were captured and dynamically monitored via in situ nanoESI-MS, responsible for the electronically matched [4 + 2] cycloaddition and subsequent intramolecular dehydrogenation. This work inspires a deeper understanding of the unique reactions in confined spaces.
Introduction
Confined spaces afford the focusing of various energies to command single entities, which triggers unique physicochemical properties. In tiny domains of micro/meso-porous crystalline materials,1,2 microdroplets,3,4 nanochannels5–7 or nanopipettes,8,9 single entities can be accommodated for enhanced catalytic performance, electrochemical field, optical density, and mass spectrometric signals. Specifically, compared to the bulk, catalytic reactions in confined spaces exhibit unique reactivity and selectivity under the accommodation. Therefore, confined spaces have attracted much attention to enable facile reactions, including protein degradation,10 nanostructure formation,11 and organic synthesis. However, the application of confined space is still in the exploratory stage due to the restricted mechanism examinations by the following factors: (1) the solution volume of reactions in tiny confined spaces is usually small, which is hard to be characterized by general methods. (2) The in situ capture, transmission, and interpretation of important species (including reactive radicals, intermediates, and transient catalyst complexes) are challenging during the rapid processes. (3) Dynamic reaction monitoring and mechanism examinations in confined spaces are hindered by the conflict between the ultrasmall volume of samples and the requirement of continuous injections.
Nanopipette, a controllable sharp tip with a nanometer-sized confined space, has exhibited much stronger capability for sensing, imaging, and synthesis.12–14 As a kind of ambient mass spectrometry (AMS), nanoESI-MS was constructed by nanopipette, which has contributed to fast detection15 and substrate screening.8 Therefore, for reaction examinations in confined space, magic nanopipettes would exhibit at least two important roles: (1) allow in situ activation of substrates for reactions in confined spaces; (2) allow fast evaluation of reactions by MS detection not only in microdroplets produced by electrospray but also in confined spaces. However, most studies were concentrated on reaction acceleration in the sprayed microdroplets, while examinations of reactions in confined spaces are normally ignored. In addition, due to the difference in interface properties between microdroplets (gas–liquid) and confined nanopipette (solid–liquid), their reaction behavior would be different. Therefore, further efforts on in situ examination and dynamic monitoring of reactions in confined spaces are encouraged.
Herein, a generally inefficient indole cation-radical cyclization reaction was selected as a model for mechanistic studies of reactions in the confined nanopipette. Using electron-rich indole as the dienophile, this reaction was normally employed under high temperature and high pressure or modifying indole with electron-withdrawing groups.16,17 Although ruthenium(II) polypyridyl complexes are selective catalysts, ligand modifications or hydrogen-bonding anion binders were usually required to tune electrochemical properties and ion-pairing interferences.18,19 This makes traditional cyclization much more rigorous. In the present work, the challenging visible-light photocatalytic cyclization reaction without electro-withdrawing groups on the dienophile was commanded in a nanopipette under mild conditions (Fig. 1A). As expected, this reaction was inefficient in both normal flasks and confined microdroplets, while exhibiting enhanced reaction efficiency in the confined space. Thereby, both ambient mass spectrometric (AMS)20,21 and theoretical examinations were employed to reveal mechanisms of enhanced reaction in the confined space.
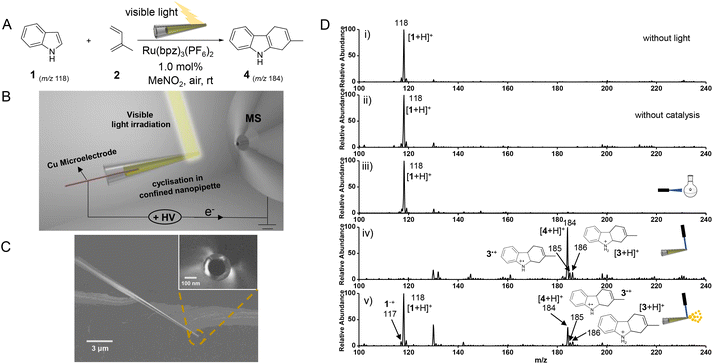 |
| Fig. 1 (A) Scheme of the indole cation-radical cyclization catalyzed by Ru(bpz)3(PF6)2 (1% eq.) under mild conditions. (B) Schematic diagram of nanopipette-based photocatalytic reactor and in situ monitoring system associated with in situ nanoESI-MS. (C) SEM images of nanopipette and the orifice (the inset). (D) Mass spectrometric analysis of Ru-catalyzed indole cation-radical cyclization under different conditions. Indole cation-radical cyclization in nanopipette without light irradiation (i) or without catalyst (ii), in normal flask (iii), nanopipette (iv) and microdroplets (v). All spectra were collected after reacting for 20 s. | |
Experimental
Regents
All reagents and solvents were purchased from commercial sources and were used without further purification unless otherwise noted. All chemicals were of analytical grade. Indole (98%, purity), Ru(bpz)3(PF6)2, and isoprene were purchased from Sigma. Nitromethane was obtained from Innochem. Glass capillaries (i.d. 0.86 mm) were produced by Sutter Instrument (Novato, CA). Laser point (450 nm, 50 mW) was purchased from Shenzhen Infrared Laser Technology Co., Ltd, and compact fluorescent light bulb (23 W) was produced by Royal Philips. 2-Deuterated indole-d1 (97% D) was synthesized according to the literature.22
Instruments and methods
All MS spectra were recorded in positive ion mode and carried out with an LTQ XL (Thermo Fisher Scientific, San Jose, CA, USA) instrument. The nanoESI emitters were constructed from glass capillaries, which were pulled to a tip using a P-1000 micropipette puller (Sutter Instrument, Novato, CA). NMR spectra were recorded on a JEOL-400 spectrometer.
Reaction preparation and procedure
In a dark hood, a solution of indole in MeNO2 (0.01 M) was prepared before mixing with isoprene (5 equiv.) and Ru(bpz)3(BArF)2 (0.01 equiv.). All working solutions were prepared freshly and preserved in the dark before use. The confined-space indole cation-radical cyclization was conducted in the nanopipette, which was irradiated via 540 nm visible light for 20 s. Then, with the light off, 0.6 kV of the spray voltage was applied to the solution for the nanoESI-MS detection. In the microdroplet reaction, MS spectra were recorded in real time when the light and high voltage of nanoESI turned on simultaneously. Besides, the bulk reaction was conducted in a flask, which was irradiated by a compact fluorescent light bulb for 20 s. Aliquots from the flask were taken and analyzed by traditional ESI-MS in the off-line mode.
Computational procedure
All the species were optimized by employing DFT UB3LYP/Def2-SVP method. The van der Waals effects were considered by Grimme D3 protocol. All the calculations were performed using Gaussian09 program.
Results and discussion
Construction of reaction system in confined space
As shown in Fig. 1B, a nanopipette-based photocatalytic reaction and in situ nanoESI-MS evaluation system were constructed. A nanopipette (140 nm, Fig. 1C) was prepared to load 1 μL dienophile indole (1) and diene (2) solution by adding Ru(bpz)3(PF6)2 catalyst at low loadings (1% eq.). For photoexcitation, a handheld laser source, hanging vertically (∼1.0 cm) on the nanopipette, offered high density and coherent visible light (450 nm, 50 mW). A Cu electrode (∼0.6 kV) was inserted into the nanopipette for electrospray and subsequent MS detections. The distance between the nanotip and the MS inlet is approximately 0.7 cm. Therefore, compared to other AMSs (equipped with Venturi or ultrasonic gas-flow extraction), this system is more convenient for dynamic monitoring of short-lived intermediates in a confined space.
Reaction evaluation by in situ nanoESI-MS
Initially, the cyclization reactions of indole (1) and isoprene (2) were respectively employed in the flask, nanopipette, and microdroplets for comparison (Fig. 1D). The confined reaction was carried out for 20 s of light irradiation at the nanotip (Fig. 1D-iv). Thereafter, with the light off, the solution in the nanopipette was detected by in situ nanoESI-MS at a sample consumption rate of about 120 nL min−1. As expected, the confined-space reaction did not proceed in the absence of either catalysis or visible light, where only the significant reactant ion [1 + H]+ at m/z 118 was observed (Fig. 1D-i and ii). Similarly, after irradiating for 20 s, species in the flask were detected by traditional ESI-MS in the off-line mode. As a result, the open-chain 1,3-dienes without a rigid s-cis-conformation of double bonds underwent a very inefficient cyclization reaction in the normal flask (Fig. 1D-iii), consistent with the previous reports.23 While in the presence of both catalyst and visible light irradiation in the nanopipette, the reactants were almost exhausted, and no remarkable reactant ion of [1 + H]+ (at m/z 118) was observed after irradiating for 20 s (Fig. 1D-iv). Notably, the main ion peak was at m/z 184, and a low abundance of the desired ion at m/z 186 was observed. As deduced by collision-induced dissociation (CID) experiment, the ion at m/z 186 was attributed to the protonated [4 + 2] cycloaddition intermediate ion ([3 + H]+) (Fig. S1-A†). The ion at m/z 184 was identified as the dehydrogenation product generated from 3 by the CID experiment (Fig. S1-B†). Furthermore, the metal electrode has little effect on the reaction, confirmed by the detection via inductive nanoESI, without physical contact between the electrode and solution (Fig. S2†). Therefore, in the confined space, the electron-rich indole undergoes a rapid [4 + 2] cycloaddition followed by catalytic intramolecular dehydrogenation.
Nevertheless, microdroplets from electrospray could exhibit an acceleration effect for reactions as reported previously.15,24 To verify whether the reaction was mainly initiated by microdroplet acceleration, the reaction system was on-line detected without pre-irradiation. As shown in the inset of Fig. 1D-v, with light irradiation (on the tip) and ESI voltage simultaneously turned on, the MS spectrum was collected at 20 s. As demonstrated (Fig. 1D-v), the reaction efficiency is quite low, which exhibited the main peak of reactant indole ([1 + H]+) at m/z 118. Furthermore, the corresponding ions of product at m/z 184 ([4 + H]+) as well as cycloaddition intermediate ion at m/z 186 ([3 + H]+) were quite low. As expected, when the microdroplet reaction was employed via shooting the light on sprayed microdroplets, no significant product ion was recorded. These could be attributed to the short reaction time during microdroplets flying from the nanotip to the MS inlet (a short distance of approximately 0.7 cm).15 Therefore, although microdroplets were reported to exhibit high reactivities to accelerate reactions, they cannot support the satisfactory reaction efficiency for the present cyclization (Fig. 1D-iv).
Significantly, the in situ nanopipette-based nano-electrospray also facilitated the capture and interpretation of short-lived intermediates through MS detection. As demonstrated, other significant cationic radicals at m/z 117 (Fig. 1D-v) and m/z 185 (Fig. 1D-iv and v) were also observed. The ion at m/z 117 was ascribed to cationic radical indole˙+ (1˙+) generated from the photo-oxidation of 1, which evidenced the cation radical-induced cyclization. The formation of the cationic radical was confirmed by comparing the cyclization reaction with the one without light irradiation (Fig. S3†).25,26 The ion at m/z 185 was assigned to radical 3˙+ (Fig. S1-C†), which was afforded by the [4 + 2] cycloaddition of 1˙+ and 2. Further oxidation or reduction of 3˙+ would generate the dehydrogenation product 4 or cycloaddition intermediate 3, respectively. This indicated the generation of 4 and 3 was competitive, and the ratio of ion at m/z 184 to m/z 186 (I184/I186) could be used for evaluating reaction efficiencies.
Isotope labeling experiments and optimizations
To further clear the attribution of 3, isotope labeling experiments were employed. In the experiment, 2-deuterated indole-d1 (97% D) acted as the dienophile for the confined cyclization reaction, whose species were in situ monitored at interval time.22 The structure of 2-deuterated indole-d1 was confirmed by both NMR and ESI-MS (Fig. S4†). As shown in Fig. 2A, without light irradiation, only protonated ion of 2-deuterated indole-d1 [1-d1 + H]+ at m/z 119 was observed (Fig. 2A-i). After 1 min of visible-light irradiation, ion at m/z 187 was observed (Fig. 2A-ii), assigned to the 3-d1 intermediate of isotope labeled cyclization (Fig. S1-D†). Subsequently, as the reaction continued, ions at m/z 184 and 187 increased significantly, exhibiting a comparable abundance to reactant ion (m/z 119) at 2 min (Fig. 2-iii). Significantly, after 6 min of the reaction, the product ion at m/z 184 came to be the main peak, while other ions, including reactant ion (m/z 119) and [3-d1 + H]+ (m/z 187), dramatically decreased (Fig. 2A-iv).
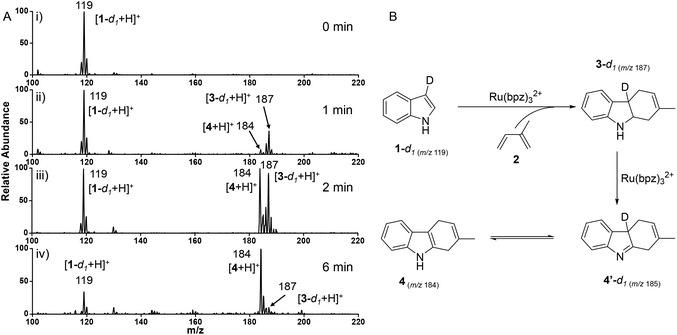 |
| Fig. 2 Isotope labelling experiment using 2-deuterated indole-d1 (97% D) as the dienophile. (A) The mass spectra of the reaction at different times. (B) The formation of product 4 (m/z 184) from the intermediate 3-d1 (m/z 187). | |
This result indicated the isotope labeled cyclization product of 3-d1 could be an intermediate to induce the formation of final product 4 (Fig. 2B). As demonstrated, 1-d1 (m/z 119) underwent the rapid [4 + 2] cycloaddition with isoprene (2), which afforded 3-d1 (m/z 187) generation catalyzed by Ru(bpz)32+. Subsequently, two hydrogen atoms were abstracted from 3-d1 to form intermediate 4-d1′ in the presence of Ru(bpz)32+. Finally, product 4 (m/z 184) could be generated by tautomerization of intermediate 4-d1′,8 in accordance with the observation of the main peak at m/z 184 (Fig. 2A-iv). It should be noted that the generation of 4 without deuteration could be attributed to the rapid hydrogen–deuterium exchange or the mediation of counteranion PF6−.27 The online extracted ion chromatograms (EICs) of deuterated ions also indicated the consumption of the reactant 1-d1 (Fig. S5-a†), along with the generation of product 4 (Fig. S5-c†). Significantly, the intensity of intermediate 3-d1 decreased after 1.5 min, which demonstrated its consumption for the generation of product 4 (Fig. S5-b†).
To obtain better reaction efficiency, the orifice size of the nanopipette and the volume of the reaction system were optimized. Herein, the ratios of I184/I186 (corresponding to the ratios of final product 4 to intermediate 3) at different conditions were calculated based on MS spectra obtained after reacting for 20 s. As shown in Fig. S6-A,† the I184/I186 value decreased with increasing the orifice size from nanometer to micron, consistent with the enhanced reaction efficiency in confined spaces.28–30 It should be noted that the nanoESI-MS detections were employed at high voltage from 0.6 to 0.9 kV when using nanopipettes with different orifice sizes (140 nm to 91 μm). In addition, a smaller volume of the reaction system was more convenient for obtaining higher reaction efficiency (Fig. S6-B†). This could be generated from the relatively higher light absorption efficiency and the shorter diffusion distance of the reagent in the tiny tip.31 Therefore, 1 μL of the reaction solution was finally adopted to conduct the confined cyclization with better reaction efficiency and less operating error.
Mechanism studies of confinement effect in nanopipette
To elucidate the confinement effect of the nanopipette on the rapid cyclization reaction, ions of Ru(II)-complex were examined by in situ nanoESI-MS. During the reaction, the ions of m/z 288 and 576 were recorded, corresponding to Ru(II)(bpz)32+ and the reduced catalyst of Ru(I)(bpz)32+ (Fig. 3a). Significantly, another two complex ions at m/z 346 and 380 were well detected by the in situ nano-electrospray MS. Confirmed by CID experiments (Fig. S7 and S8†), the two complex ions were generated from the incorporation of Ru (bpz)32+ with reactant 1 and intermediate 3.
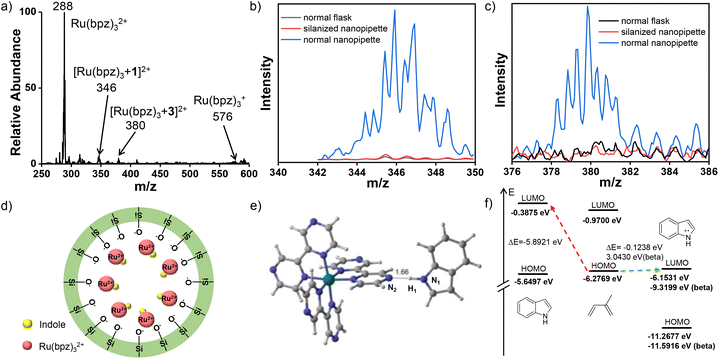 |
| Fig. 3 Mechanism studies of confinement effect in confined space. (a) Mass spectrum of Ru(II)-complexes. (b) Ion signal of complex [Ru(bpz)3 + 1]2+ in bulk, and nanopipette with and without surface Si–OH. (c) Ion signal of [Ru(bpz)3 + 3]2+ in bulk, and nanopipette with and without surface Si–OH. (d) Illustration of the concentration of Ru(bpz)32+ by surface Si–OH. (e) Calculated structure of complex [Ru(bpz)3 + 1]2+. (f) Frontier molecular orbitals of indole, indole˙+ and isoprene. | |
Subsequently, the formation of the above Ru-complexes on the inner surface of the nanopipette was studied. In fact, the nanopipette glass is not inert and could take roles during reactions,32,33 such as the electrostatic interactions from surface silanol groups.34 Therefore, the inner surface of the nanopipette was silanized by dichlorodimethylsilane,35 which would avoid the electrostatic interactions from the ionization of silanol groups. Afterwards, the ions of Ru(II)-complex in the bulk reaction system, normal nanopipette, and silanized nanopipette were respectively captured for comparison. As demonstrated in Fig. 3b and c, the significant Ru(II)-complex ions of [Ru(bpz)3 + 1]2+ (m/z 346) and [Ru(bpz)3 + 3]2+ (m/z 380) were observed in the normal nanopipette. While in the normal flask and silanized nanopipette, no significant complex ion was recorded. Besides, using the silanized nanopipette, only the reactant ion of [1 + H]+ was observed after the irradiation (Fig. S9†). Therefore, it can be concluded that the inner surface silanol of the nanopipette facilitated the formation of initial Ru-complexes with reactant and intermediate.
Actually, the inner glass surface of the nanopipette could be negatively charged due to the ionization of silanol groups (Fig. 3d), which was also mentioned in microchannels.36 Thereafter, the positively charged Ru(bpz)32+ diffusion layer is automatically formed to give an electric double layer under the electrostatic interactions. This undoubtedly resulted in the concentrated Ru(bpz)32+ catalyst on the inner surface of the nanopipette. Therefore, the locally increased catalyst concentration dramatically promoted the formation of Ru-complexes for facile oxidations, which were further examined by theoretical calculations. In addition, the present photocatalytic reaction was initiated by activating the Ru(bpz)32+ catalyst via light absorption (Fig. 1D-i and ii), in accordance with the reports.19 Therefore, via concentrating on inner surface of the nanopipette, the Ru(bpz)32+ with the maximum light absorption efficiency is more likely to be greatly activated.
As demonstrated, [Ru(bpz)3 + 1]2+ was delicately obtained by covalent bonding between indole and Ru(bpz)32+via hydrogen bond between H1 and N2 (Fig. 3e). This was also confirmed by the CID experiment (Fig. S7†). Interestingly, calculations indicated that one β electron is transferred from 1 to Ru(bpz)32+, which resulted in an approximate +1 of the spin population summed on 1. This result indicated that dissociative radical cations of 1˙+ could be generated from the photoexcited conversion of the complex to LMCT states.37 This is crucial for the cation-radical initiated cyclization. The formation of radical cations 1˙+ was further supported by studying the kinetic properties of substrates and catalyst under the theoretical level of UB3LYP/Def2-SVP.38 Firstly, the electronic states of indole and Ru(bpz)32+ were calculated to evaluate electron transformation among different valence states. As calculated, −0.377 Ha energy was released from the reduction of Ru 4d6 (Ru2+) that was triggered by one electron transformation. This is much higher than the required energy of indole (0.279 Ha) oxidation (Table S1†), which indicates the ready generation of radical ions of 1˙+ (m/z 117). In fact, the formation of 1˙+ is crucial for the cyclization reaction under mild conditions.38 As verified (Fig. 3f), the calculated HOMO–LUMO gap between diene and 1˙+ is much lower than that between diene and indole, exhibiting electronically matched states under mild conditions. Similarly, intermediate 3 is also covalently bonded to Ru(bpz)32+via hydrogen bond (Fig. S10†). This promoted the formation of radical cations 3˙+ (m/z 185) via LMCT (Table S1†), which facilitated the intramolecular dehydrogenation to generate final product 4.
On-line monitoring of important reaction species in nanopipette
To further examine dynamic changes of the indole cation-radical cyclization in confined space, time-dependent profiles were obtained via in situ nanoESI-MS. Fig. 4 exhibits the online extracted ion chromatograms (EICs) of the reactant ion at m/z 118, the product ion at m/z 184, as well as intermediate ions at m/z 117, m/z 185 and m/z 186. As expected, upon visible light irradiation, the intensity of the reactant ion decreased gradually (Fig. 4a), accompanied by the momentary increase of cationic radical indole˙+ (1˙+ at m/z 117) before 1 min (Fig. 4b). Along with the consumption of 1˙+, cationic radical of (3˙+) at m/z 185 increased gradually within 1 min (Fig. 4c). In addition, the intermediate ion of [3 + H]+ at m/z 186 increased after approximately 1 min (Fig. 4d), accompanied by the simultaneous decrease of 3˙+ (Fig. 4c). Subsequently, intermediate 3 was consumed, and the intensity decreased gradually after approximately 1.5 min (Fig. 4d). This result also strongly verified that 3 is a relatively stable intermediate for the generation of product 4. Undoubtedly, the product signal of [4 + H]+ at m/z 184 kept increasing and reached the highest intensity at approximately 6 min (Fig. 4e). This time dependence property was different from the reported accelerated reactions in microdroplets within milliseconds.10 This further excluded the possiblity of employing the present indole cation-radical cyclization in microdroplets. Therefore, the dynamic changes of different species were succesfully monitored to propose the mechanism.
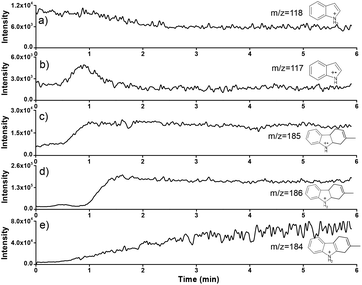 |
| Fig. 4 Dynamic studies of different ions in confined spaces. EICs of [1 + H]+ at m/z 118 (a), 1˙+ at m/z 117 (b), 3˙+ at m/z 185 (c), [3 + H]+ at m/z 186 (d), and [4 + H]+ at m/z 184 (e). | |
Mechanism of confined cyclization reaction in nanopipette
Consequently, based on the experimental and theoretical studies, the mechanism of confined photocatalytic indole cation-radical cyclization was proposed. In the confined space (Fig. 5), the negatively charged Si–O− layer was formed on the inner surface of the nanopipette by the ionization of silanol groups. This induced a diffusion layer of concentrated positively charged Ru(bpz)32+ to form an electric double layer. The confined space of the nanopipette significantly limited the mass transfer of indole (1),1 which was favorable for the formation of complex [Ru(bpz)3 + 1]2+ (m/z 346). Under photoexcitation, LMCT was initiated to form dissociative Ru(bpz)3+ (m/z 576) and a cationic radical 1˙+ (m/z 117) with lower HOMO–LUMO gap for subsequent reactions.37 Simultaneously, oxygen acts as the charge carrier to trigger the charge transfer for converting Ru(bpz)3+ to Ru(bpz)32+.19,38 Thereafter, in the presence of isoprene (2), radical 1˙+ can rapidly undergo an electronically matched Diels–Alder reaction to generate the cationic radical 3˙+ (m/z 185). The generated 3˙+ could abstract one electron from another equivalent of 1via the chain-propagation step (the dashed line in Fig. 5) to form 1˙+ (m/z 117) and 3 (m/z 186).19,26 Besides, intermediate 3 (m/z 186) could also be obtained by the reduction of 3˙+via O2˙−.39 Similarly, the confined space also promotes the formation of complex [Ru(bpz)3 + 3]2+ (m/z 380), followed by the photoinduced LMCT to afford dissociative 3˙+. Furthermore, O2˙− is active in abstracting two hydrogen atoms from 3˙+ to generate intermediate 4′ and H2O2. Finally, product 4 (m/z 184) was obtained via the tautomerization of intermediate 4′.8,40
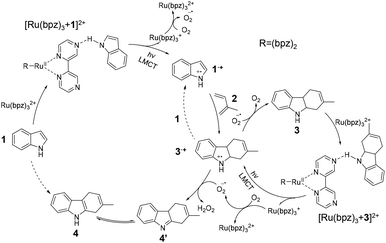 |
| Fig. 5 Possible mechanism of the photocatalytic indole cation-radical cyclization reaction in confined space. 1–4: reactant, intermediates and product detected by in situ nano-electrospray MS. | |
Conclusions
In summary, the enhanced indole cation-radical cyclization in the confined nanopipette was demonstrated and examined by mass spectrometry detections. As examined by the in situ monitoring with nanoESI-MS, new insights on the mechanism, including [4 + 2] cycloaddition and intramolecular dehydrogenation, were demonstrated. Both experimental and theoretical examinations demonstrated that an electric double layer on the inner surface of the nanopipette induces the concentration of catalysts to promote the reaction. This work has broken through the limitations on in situ capture, interpreting and dynamic monitoring of reactive intermediates in tiny geometric spaces. As an innovative effort to apply AMS to in situ examinations of the confined space, this work would inspire a deeper understanding of confinement effects.
Conflicts of interest
There are no conflicts to declare.
Acknowledgements
The authors gratefully acknowledge the financial support provided by the National Key Research and Development Program of China (No. 2019YFC1805600) and the National Natural Science Foundation of China (22274012, 21874012, 21974010).
References
- J. Dai and H. Zhang, Small, 2021, 17, 2005334 CrossRef PubMed.
- T. Dwars, E. Paetzold and G. Oehme, Angew. Chem., Int. Ed., 2005, 44, 7174–7199 CrossRef.
- D. B. Eremin and V. V. Fokin, J. Am. Chem. Soc., 2021, 143, 18374–18379 CrossRef PubMed.
- X. Yan, R. M. Bain and R. G. Cooks, Angew. Chem., Int. Ed., 2016, 55, 12960–12972 CrossRef PubMed.
- X. Wang, L. Liu, Q. Pu, Z. Zhu, G. Guo, H. Zhong and S. Liu, J. Am. Chem. Soc., 2012, 134, 7400–7405 CrossRef PubMed.
- K. Qiu, T. P. Fato, B. Yuan and Y.-T. Long, Small, 2019, 15, 1805426 CrossRef PubMed.
- H. Song, D. L. Chen and R. F. Ismagilov, Angew. Chem., Int. Ed., 2006, 45, 7336–7356 CrossRef CAS PubMed.
- S. Chen, Q. Wan and A. K. Badu-Tawiah, Angew. Chem., Int. Ed., 2016, 55, 9345–9349 CrossRef CAS.
- E. T. Jansson, Y.-H. Lai, J. G. Santiago and R. N. Zare, J. Am. Chem. Soc., 2017, 139, 6851–6854 CrossRef CAS PubMed.
- X. Zhong, H. Chen and R. N. Zare, Nat. Commun., 2020, 11, 1049 CrossRef CAS.
- J. K. Lee, D. Samanta, H. G. Nam and R. N. Zare, Nat. Commun., 2018, 9, 1562 CrossRef.
- Y. Zhang, Y. Tang, C. Tan and W. Xu, ACS Cent. Sci., 2020, 6, 1001–1008 CrossRef CAS PubMed.
- R.-J. Yu, Y.-L. Ying, R. Gao and Y.-T. Long, Angew. Chem., Int. Ed., 2019, 58, 3706–3714 CrossRef CAS PubMed.
- S.-M. Lu, Y.-Y. Peng, Y.-L. Ying and Y.-T. Long, Anal. Chem., 2020, 92, 5621–5644 CrossRef CAS PubMed.
- R. M. Bain, S. T. Ayrton and R. G. Cooks, J. Am. Soc. Mass Spectrom., 2017, 28, 1359–1364 CrossRef CAS PubMed.
- E. Wenkert, P. D. R. Moeller and S. R. Piettre, J. Am. Chem. Soc., 1988, 110, 7188–7194 CrossRef CAS.
- A. Chretien, I. Chataigner, N. L'Helias and S. R. Piettre, J. Org. Chem., 2003, 68, 7990–8002 CrossRef CAS PubMed.
- E. P. Farney, S. J. Chapman, W. B. Swords, M. D. Torelli, R. J. Hamers and T. P. Yoon, J. Am. Chem. Soc., 2019, 141, 6385–6391 CrossRef.
- S. Lin, M. A. Ischay, C. G. Fry and T. P. Yoon, J. Am. Chem. Soc., 2011, 133, 19350–19353 CrossRef.
- J. Sun, Y. Yin, W. Li, O. Jin and N. Na, Mass Spectrom. Rev., 2022, 41, 70–99 CrossRef.
- Y. Wang, M. Sun, J. Qiao, J. Ouyang and N. Na, Chem. Sci., 2018, 9, 594–599 RSC.
- S. A. de Keczer, T. S. Lane and M. R. Masjedizadeh, J. Labelled Compd. Radiopharm., 2004, 47, 733–740 CrossRef.
- T. Peglow, S. Blechert and E. Steckhan, Chem. Commun., 1999, 433–434, 10.1039/a900078j.
- N. Sahota, D. I. AbuSalim, M. L. Wang, C. J. Brown, Z. Zhang, T. J. El-Baba, S. P. Cook and D. E. Clemmer, Chem. Sci., 2019, 10, 4822–4827 RSC.
- J. Sun, X. Fan, H. Lu, H. Tan, Y. Zhang, Y. Wang, Y. Zhao, J. Ouyang and N. Na, Chem. Commun., 2021, 57, 3921–3924 RSC.
- Y. Cai, J. Wang, Y. Zhang, Z. Li, D. Hu, N. Zheng and H. Chen, J. Am. Chem. Soc., 2017, 139, 12259–12266 CrossRef CAS PubMed.
- M. Talavera, J. Bravo, L. Gonsalvi, M. Peruzzini, C. Zuccaccia and S. Bolano, Eur. J. Inorg. Chem., 2014, 6268–6274, DOI:10.1002/ejic.201402882.
- J. Xiao, X. Pan, S. Guo, P. Ren and X. Bao, J. Am. Chem. Soc., 2015, 137, 477–482 CrossRef PubMed.
- X. Pan and X. Bao, Chem. Commun., 2008, 6271–6281, 10.1039/b810994j.
- S. A. Miners, G. A. Rance and A. N. Khlobystov, Chem. Sci., 2016, 45, 4727–4746 Search PubMed.
- Q. Wan, S. Chen and A. K. Badu-Tawiah, Chem. Sci., 2018, 9, 5724–5729 RSC.
- Y. Li, T. Mehari, Z. Wei, Y. Liu and R. G. Cooks, Angew. Chem., Int. Ed., 2021, 60, 2929–2933 CrossRef.
- Y. Li, K.-H. Huang, N. M. Morato and R. G. Cooks, Chem. Sci., 2021, 12, 9816–9822 RSC.
- N. O. Pretorius, C. M. Willemse, A. de Villiers and H. Pasch, J. Chromatogr., A, 2014, 1330, 74–81 CrossRef CAS.
-
B. Seed, Current protocols in cell biology, 2001, Appendix 3, 3E-Appendix 3E Search PubMed.
- A. Datta, S. Gangopadhyay, H. Temkin, Q. S. Pu and S. R. Liu, Talanta, 2006, 68, 659–665 CrossRef CAS PubMed.
- Q. Y. Li, S. N. Gockel, G. A. Lutovsky, K. S. DeGlopper, N. J. Baldwin, M. W. Bundesmann, J. W. Tucker, S. W. Bagley and T. P. Yoon, Nat. Chem., 2022, 14, 94–100 CrossRef CAS.
- Q. Liu, L.-J. Cheng and K. Wang, RSC Adv., 2017, 7, 30618–30625 RSC.
- Y. Zhao and M. Antonietti, Angew. Chem., Int. Ed., 2017, 56, 9336–9340 CrossRef CAS.
- S. Jayaraj and A. K. Badu-Tawiah, Sci. Rep., 2019, 9, 11280 CrossRef.
|
This journal is © The Royal Society of Chemistry 2023 |
Click here to see how this site uses Cookies. View our privacy policy here.