DOI:
10.1039/D3QI00479A
(Research Article)
Inorg. Chem. Front., 2023,
10, 3230-3236
An ultra-stable CuI12 cluster built from a CuI6 precursor sandwiched by two CuI3-thiacalixarene units for efficient photothermal conversion†
Received
15th March 2023
, Accepted 24th April 2023
First published on 25th April 2023
Abstract
We report the synthesis, crystal structure, optical properties, and photothermal conversion properties of an ultra-stable cuprous Cu12 cluster, namely {[CuI3(HTC4A)]2[CuI6(2-PyS)6]}·H2O (Cu12, H4TC4A = p-tert-butylthiacalix[4]arene, 2-PySH = 2-pyridinethiol), which was built from a pre-synthesized CuI6(2-PyS)6 (Cu6) precursor and two CuI3-HTC4A polynuclear secondary building units (PSBUs). The Cu12 cluster features a sandwich-like framework in which the Cu6 core is double surface capped by forming six Cu–S bonds with two CuI3-HTC4A PSBUs. The “cluster–cluster” assembly strategy enables all the metal centers in the Cu12 cluster to be monovalent and efficient organic ligand protection makes the cuprous cluster stable in common solvents (alcohol, acetonitrile, acetone, CHCl3, N N-dimethylacetamide, etc.) as well as in strong acids (pH = 1) or bases (pH = 14). Band gap determination and photophysical analysis combined with density functional theory (DFT) calculations indicated that CuI3-HTC4A PSBUs can tune the electron and hole distribution of the Cu6 core, which makes Cu12 a stable and efficient photothermal conversion material both in the solid state and in water/N,N-dimethylformamide solvents.
1 Introduction
Coinage metal clusters with atomically precise structures are of great interest due to their fascinating structure evolution and promising applications in biological sensing, catalysis, and nanoscale optoelectronics.1–3 Coinage metal clusters can be synthesized by reduction growth, seed growth, alloying, and ligand exchange methods.4 A variety of huge coinage clusters, including Cu81,5 Au279,6 and Ag490,7 have been successfully synthesized and structurally determined. Compared with the achievements in synthesis, the utilization of coinage metal clusters is limited by their stability at ambient condition. Due to their unique potential applications in catalytic,8 luminescence,9 and biological processes10 and of course, due to their atom economy, cuprous clusters have received considerable attention from scientists. However, copper has a lower reduction potential than AuI and AgI and is not readily stable in air; thus, it is always challenging to fabricate a stable cuprous cluster that can be utilized under ambient or extreme conditions.11–13 The ligand modification and protection strategy has been proven to be an effective way to fabricate stable cuprous clusters, and ligands with rich electronic structures can also endow these clusters with a variety of applications.14–16
Cyclic calixarene compounds with high thermal and chemical stability as well as changeable conformations and abundant coordination sites have received extensive attention in coordination chemistry.17p-tert-Butylthiacalix[4]arene (H4TC4A) is the most utilized calixarene with sulfur bridging. It has been proven to be an especially effective ligand to form Mx (TC4A) (M = MnII, CoII, NiII, FeII, etc., x = 3 or 4) polynuclear secondary building units (PSBUs) by strongly combined coordination of bridging S atoms and phenoxo groups for the assembly of metal clusters.18–22 Recently, Wang and Sun used H4TC4A to assemble a series of AuI and AgI clusters, and the ligand protection to achieve stable clusters is evident.23–28 It is also familiar to the assembly of CuII-based metal clusters but there are limited examples of pure CuI clusters of H4TC4A that can be obtained. Liao and co-workers reported a two-dimensional (2D) polymer based on CuI2Cl2-(TC4A) units.29 However, this compound is easily turned into a CuII4-(TC4A) cluster in the mother liquid in air. Attempts at H4TC4A functionalization, e.g., t-butyl substituted by phenyl groups or –OH replaced by –SH, did not work to separate a stable CuI-(H4TC4A) compound at ambient condition.29,30 Therefore, the absence of CuII species in the initial synthesis system might be the determining factor in the fabrication of the stable CuI compound H4TC4A. Otherwise, H4TC4A tends to use its multidentate coordination sites to form a triangular coordination environment, leaving at least two active metal orbitals to be coordinated, as is favorable for forming CuII compounds.31–36 Very recently, two isomeric CuI13(TC4A)2 pairs were successfully isolated by using CuII and Cu0 sources, and the NaBH4 reduction agent was applied to avoid the reoxidation of the CuI species by O2 in air.26
In this work, we adopted a “cluster–cluster” assembly strategy to control the synthesis of a cuprous Cu12 cluster by pre-synthesis of a [CuI6(2-PyS)6] (2-PySH = 2-pyridinethiol) precursor with exposed S coordination sites and capped with CuI3-HTC4A PSBUs afterward. The Cu12 cluster was stable at ambient condition for six months and the broad absorbance makes it an excellent photothermal conversion material both in the solid state and in solvents.
2. Experimental section
2.1 Materials and measurements
p-tert-Butylthiacalix[4]arene (H4TC4A) was synthesized according to the procedure in the literature.37 Other reagents were purchased commercially without further purification. Thermogravimetric analysis (TGA) was carried out at a ramp rate of 10 °C min−1 in an N2 flow with a TA Q600 TGA analyzer. Fourier transform infrared spectroscopy (FT-IR) using KBr pellets was performed on a PerkinElmer Spectrum GX spectrometer. Powder X-ray diffraction (PXRD) was performed using a Bruker D8 VENTURE diffractometer with Cu-Kα radiation. Ultraviolet–visible (UV-vis) spectra were recorded on an Agilent Cary5000 spectrometer. X-ray photoelectron spectroscopic (XPS) measurements were made with an ESCALAB 250Xi using a monochromic Al KαX-ray source (1486.6 eV).
2.2 Synthesis of a CuI6(2-PyS)6 (2-PySH = 2-pyridinethiol) precursor
CuI6(2-PyS)6 (Cu6) is a known compound and was synthesized by the solvothermal method in this report.38 X-ray diffraction measurements showed the same crystal parameters as those in the literature. Details of the structure solution and final refinements are given in Table S1.† The phase purity of the crystals was confirmed by powder X-ray diffraction (PXRD) analysis.
2.3 Synthesis of {[CuI3(HTC4A)]2[CuI6(2-PyS)6]}·H2O (Cu12)
A mixture of Cu6 (0.050 g, 0.05 mmol), CuCl (0.030 g, 0.3 mmol), H4TC4A (0.72 g, 0.1 mmol), three drops of triethylamine, methanol (MeOH, 5.0 mL), and N,N-dimethylacetamide (DMA, 5.0 mL) in a 20 mL Teflon-lined autoclave was kept at 130 °C for two days and then slowly cooled to 20 °C. Light brown block crystals were obtained in 34.7% yield (based on H4TC4A). The crystals were isolated by filtration and then washed with 1
:
1 MeOH–DMA and dried in air. FT-IR (KBr pellet, cm−1): 3440(m), 2962(s), 1636(w), 1577(s), 1451(s), 1358(m), 1311(m), 1257(s), 1124(s), 885(m), 831(m), 752(s), and 720(m).
2.4 Single crystal X-ray diffraction
Intensity data were collected at 296 K using Mo-Kα radiation on a Bruker D8 QUEST system (λ = 0.71073 Å). Direct methods were used to solve the crystal structures, and then full-matrix least squares on F2 (SHELXTL-2014) were used to refine them.39 All non-hydrogen atoms were polished anisotropically except for lattice water. The hydrogen atoms in the organic ligands were theoretically fixed on the particular atoms and refined isotropically with predetermined thermal factors. The hydrogen atoms on solvent H2O molecules were directly incorporated in the molecular formula. Details of the structure solution and final refinements for the compounds are given in Table S1.† CCDC2246463 contains the supplementary crystallographic data for this paper.†
3. Results and discussion
3.1 Synthesis and characterization
The Cu6 precursor was synthesized by the solvothermal method (Fig. 1). Cu12 was built from one Cu6 core with a similar arrangement to that of the Cu6 precursor and two CuI3-HTC4A (H4TC4A = p-tert-butylthiacalix[4]arene, Fig. S1†) polynuclear secondary building units (PSBUs) by the “cluster–cluster” assembly strategy. The phase purity of the samples is confirmed by the powder X-ray diffraction (PXRD) of the two clusters, which consisted of the simulated patterns obtained from single-crystal X-ray diffraction (SCXRD, Fig. S2†). The FT-IR results revealed the predicted peaks for the components of Cu12, showing the characteristic C–H vibration p-tert-butyl groups and phenolic groups of H4TC4A (Fig. S3†). TGA tests were performed to test the thermal stability and the TGA residue is also confirmed by PXRD (Fig. S4 and S5†).
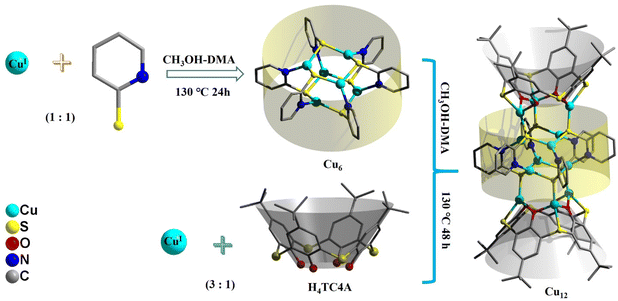 |
| Fig. 1 Synthesis and structure of Cu6 and Cu12. | |
3.2 Crystal structures
Single-crystal X-ray diffraction showed that 2-PyS− ligands in Cu6 show the same coordination mode (μ3-κN1:κS2) and copper centers are tri-coordinated with one N and two μ2-S atoms. Cu12 crystallizes in the tetragonal system with the space group P42/mbc. The Cu12 cluster is a sandwich-like structure with a Cu6(2-PyS)6 core double-surface capped by two Cu3-HTC4A PSBUs by forming six Cu–S bonds (Fig. 1). The Cu6(2-PyS)6 core in Cu12 is an inheritance from the Cu6 precursor except for the 2-PyS− ligands in a different coordination mode (μ4-κN1:κS3). Furthermore, each HTC4A3− ligand bonds with three Cu centers (Cu1, Cu2, and Cu1i) and each Cu is coordinated by two μ2 phenoxyl atoms and one bridging sulfur atom from the HTC4A3− ligand. The overall coordination of three Cu in PSBUs is four-coordination in a tetrahedral arrangement. All the Cu atoms both in Cu6 and Cu12 showed the low coordination (2, 3, 4) of a typical CuI.26,29,30,40,41 Both Cu6 and Cu12 contain a highly similar Cu6(2-PyS)6 core but differ in distortion. The average Cu⋯Cu distance in the “Cu6(2-PyS)6 core” of Cu12 being 3.251 Å is much larger than that for Cu6 (2.953 Å), which suggests that the presence of Cu3-HTC4A stretched the “Cu6(2-PyS)6 core” on both sides (Fig. 2). Additionally, the distance between two adjacent coppers (Cu1⋯Cu2, Cu1⋯Cu3iii, Cu2⋯Cu3iii) was 3.605, 3.437, and 3.622 Å, respectively (Fig. S6†). Combined with the Cu coordination, bond valence calculation, charge balance (Table S2†) and X-ray photoelectron spectroscopy (XPS) results (Fig. S7†), all the Cu in Cu12 being cuprous is evident. Additionally, no obvious interaction is observed between adjacent two metal clusters and isolated solvent waters are located in the crystal lattice of Cu12 (Fig. S8†).
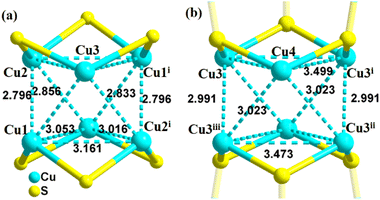 |
| Fig. 2 (a) Cu⋯Cu distance (Å) in Cu6 and (b) Cu⋯Cu distance (Å) in the “Cu6(2-PyS)6” core of Cu12. Symmetry codes: Cu6 (i) −x + 1, −y + 1, −z + 1; Cu12 (i) x, y, −z + 1; (ii) −x + 1, −y + 1, −z + 1; (iii) −x + 1, −y + 1, z. | |
3.2 Stability of Cu12
We tested the stability of Cu12 under various conditions. The crystal samples were immersed in water solutions with pH values of 1, 7, and 14 for 48 h, in 1 M H2O2 for 6 h, and in common solvents (or mixture) for 48 h. Then, PXRD patterns were obtained under ambient conditions. As shown in Fig. 3, the tested PXRD patterns are well-matched with the simulated one from SCXRD. Additionally, Cu12 can also keep its crystallinity well after exposing to air for more than six months. All those results suggested the outstanding structural integrity and stability of Cu12.
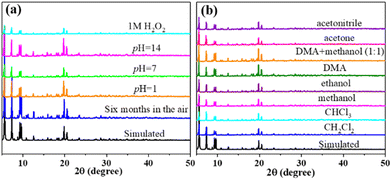 |
| Fig. 3 PXRD pattern of Cu12 showing the stability under different conditions: (a) in air and water solution; (b) in common organic solvents. | |
For most cluster coordination compounds, it is easy to lose the crystallinity under ambient conditions and their crystal structures are usually determined at low temperatures by liquid nitrogen protection, especially for those containing coinage metals.5–7,42 The high-resolution XPS spectrum of S in Cu12 is 1.3 eV bigger than that in Cu6, which suggests that the electron density of S is lower in Cu12 than in Cu6 (Fig. 4). It is more likely that the strong coordination and delocalization ability of HTC4A3− tunes the S electron density distribution from the Cu6(2-PyS)6 core to CuI3-HTC4A PSBUs, which corresponded to the high-resolution XPS spectra of Cu 2p (Fig. S7b†). That is, HTC4A3− contributes to the stability of Cu12 by not only shielding CuI from oxidation, but also changing the electron distribution to decrease the activity of the CuI12 core.
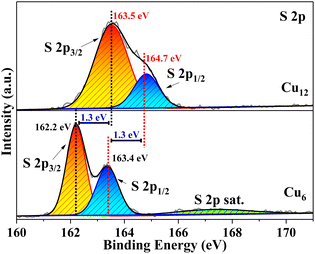 |
| Fig. 4 High-resolution XPS spectra of S 2p (Cu12 and Cu6). | |
3.3. UV–vis absorption and DFT calculation studies
The solid-state UV–vis spectra of Cu6 and Cu12 were tested in the range of 200–800 nm (Fig. 5). The absorption of Cu12 is weaker in the range of 400–500 nm and stronger between 200–400 nm compared with Cu6, the latter of which might be due to the presence of HTC4A3−, which enhances the π → π* transformation of Cu12.43,44 The broad absorption in 500–800 nm indicated that Cu12 might be a good candidate as a long-wavelength responsive light material. To better understand the relationship between the compound structures and their light absorption, DFT calculations using B3LYP were performed for Cu6 and Cu12 based on the models from single-crystal structures, respectively (Fig. 6). Both the HOMO and HOMO−1 of Cu12 are almost entirely located on the calixarene ligand (donor), while the LUMO is mainly located on the “Cu6(2-PyS)6” core (acceptor). This suggests that ligand-to-metal charge transfer (LMCT) and ligand-to-ligand charge transfer (LLCT) might occur and result in long-wavelength light absorption.45 Contrastingly, the HOMO and HOMO−1 of Cu6 are virtually totally found on the Cu center, whereas the LUMO and LUMO+1 are primarily placed on the ligand, indicating metal-to-ligand charge transfer (MLCT) being the main contribution that results in the 400–500 nm absorption.45 Simultaneously, the HOMO–LUMO gap with the B3LYP function and the def2-SVP basis group for Cu12 and Cu6 are calculated to be 2.96 eV and 3.61 eV, respectively, the former of which is quite accordant with that from the band gap determination (2.97 eV) while the latter of which is obviously different (2.85 eV). As for the DFT calculations performed on single clusters excluding “cluster–cluster” interactions, the difference between the HOMO–LUMO gap and band gap for Cu6 is contributed to the inter-cluster actions via, for example, π⋯π or C–H⋯π interactions of two 2-PyS− ligands from two clusters (Fig. S9†). The larger band gap energy and non-inter-cluster actions make Cu12 not only stable in the crystalline form, but also in a single cluster.
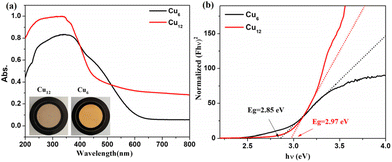 |
| Fig. 5 (a) UV-vis spectra and (b) band gaps of Cu6 and Cu12. | |
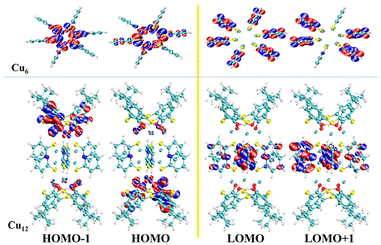 |
| Fig. 6 Frontier molecular orbitals of Cu6 (top) and Cu12 (bottom). | |
3.4 Photothermal studies
Photothermal studies on Cu-based coordination compounds mainly involved divalent46 and mixed valence41,47–49 clusters, and a pure CuI analogue is rarely involved. The larger band gaps originated from LMCT and LLCT as well as the ultra-stable nature of Cu12 prompted us to investigate the photothermal properties. An appropriate sample was placed on a 2 × 2 cm2 glass sheet, a 660 nm laser was used as a light source, and a thermal imager recorded the temperatures of the sample. The irradiation power was 0.4 W cm−2 (see the ESI† for details). The time–temperature plots were obtained as shown in Fig. 7a. The temperature of Cu12 sharply increased from room temperature (20 °C) to 70 °C within 5 s corresponding to a 9.2 °C s−1 heating rate. In stark contrast, the temperatures of Cu6 and the mixture (H4TC4A
:CuCl = 1
:
3) reached 27 °C and 26 °C, with the heating rate of 1 °C s−1 and 0.8 °C s−1, respectively, under the same conditions. The maximum temperature of Cu12 reached 124.5 °C (Fig. 7b) and was kept steady; however, the temperatures were significantly lower (below 73.1 °C) for both Cu6 and the mixture. No obvious decay was observed in four light on–off cycles for three compounds (150 s per cycle, Fig. 7c). A fast photothermal conversion rate and a visual photothermal delay were apparent for Cu12. It is indicated that Cu12 has efficient photothermal conversion properties compared with the contrasts. With combined absorption properties and DFT calculation, the superior photothermal conversion of Cu12 might originate from the larger absorption at 660 nm and LMCT nature, which results in electron–hole pairs relaxing to the band edges upon irradiation and then releasing phonon energy to transform into heat through a nonradioactive decay.50 For better comparison, some recent reports on Cu-based coordination compounds/representative composites for photothermal conversion are listed in Table S3.†
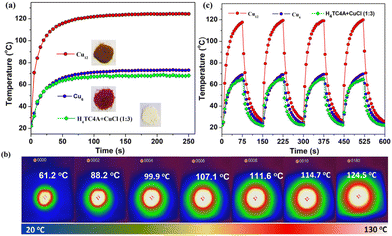 |
| Fig. 7 Photothermal experiment in the solid state: (a) time–temperature curves for Cu12, Cu6, and the mixture; (b) photothermal images for Cu12 (interval, 5 s); (c) light on–off cycles. | |
Cu12 is insoluble and stable in H2O or DMF as indicated by PXRD experiments. By placing the Cu12 sample at the bottom of a plastic pipe in different solvents and exposing the sample to a 0.4 W cm−2 660 nm laser, the solvent photothermal property for the metal cluster was evaluated (Fig. 8). Upon irradiation within 10 min, the solid sample temperature elevated quickly from room temperature to 65.7 °C in DMF and 56.5 °C in H2O. Meanwhile, the solvent also gradually warmed to 43.3 °C and 29.9 °C, respectively. The solid sample temperatures were similar while the solvent temperatures were different. The different photothermal conversion properties in H2O and DMF are due to the different solvent heat capacities. After the experiment, the samples were separated from the solvents by filtration and characterized by PXRD. The resulting PXRD patterns still matched the simulated one (Fig. S10†). The results suggest that Cu12 is stable even after the photothermal experiments involving the solvent.
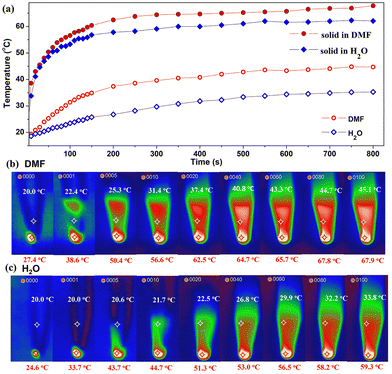 |
| Fig. 8 Photothermal experiment in solvent for Cu12 (a) time–temperature curves; photothermal images in DMF (b) and H2O (c). Red and white labels represent sample and solvent temperatures. | |
4. Conclusions
An ultra-stable cuprous Cu12 cluster has been fabricated by the “cluster–cluster” assembly strategy from pre-synthesized CuI6(2-PyS)6 and two CuI3-HTC4A polynuclear secondary building units (PSBUs). The modification of CuI6(2-PyS)6 with two CuI3-HTC4A PSBUs not only makes the obtained sandwich-like Cu12 cluster stable in air, in strong acids, or bases as well as in common solvents, but also significantly tunes the light absorption. Cu12 showed improved photothermal conversion efficiency and stability both in the solid state and in solvents, which is quite correlated with the band gap structure as evidenced by DFT calculations. This work provides a new view of fabricated stable cuprous materials and may shed light on the utilization of solar energy for storage and conversion.
Author contributions
Y. F. Bi and B. K. Chen conceived and designed the project. Z. H. Zhou, L. M. Xu, G. Y. Zhao, and K. Zhou performed the experiments and characterization. Z. H. Zhou performed the DFT calculations and wrote the original draft. G. Y. Zhao and Y. F. Bi provided supervision, validated the experimental results, and reviewed and edited the manuscript. B. K. Chen and Y. F. Bi provided funding support.
Conflicts of interest
There are no conflicts to declare.
Acknowledgements
This work was supported by the National Natural Science Foundation of China (no. 91961110, 22171122).
References
- J. P. Wilcoxon and B. L. Abrams, Synthesis, structure and properties of metal nanoclusters, Chem. Soc. Rev., 2006, 35, 1162 RSC
.
- S. Yamazoe, K. Koyasu and T. Tsukuda, Nonscalable Oxidation Catalysis of Gold Clusters, Acc. Chem. Res., 2014, 47, 816–824 CrossRef CAS PubMed
.
- R. Jin, Atomically precise metal nanoclusters: stable sizes and optical properties, Nanoscale, 2015, 7, 1549–1565 RSC
.
- Q.-F. Yao, T.-K. Chen, X. Yuan and J.-P. Xie, Toward Total Synthesis of Thiolate-Protected Metal Nanoclusters, Acc. Chem. Res., 2018, 51, 1338–1348 CrossRef CAS PubMed
.
- R.-W. Huang, J. Yin, C. Dong, A. Ghosh, M. J. Alhilaly, X. Dong, M. N. Hedhili, E. Abou-Hamad, B. Alamer, S. Nematulloev, Y. Han, O. F. Mohammed and O. M. Bakr, [Cu81(PhS)46(tBuNH2)10(H)32]3+ Reveals the Coexistence of Large Planar Cores and Hemispherical Shells in High-Nuclearity Copper Nanoclusters, J. Am. Chem. Soc., 2020, 142, 8696–8705 CrossRef PubMed
.
- N. A. Sakthivel, S. Theivendran, V. Ganeshraj, A. G. Oliver and A. Dass, Crystal Structure of Faradaurate-279: Au279(SPh- tBu)84 Plasmonic Nanocrystal Molecules, J. Am. Chem. Soc., 2017, 139, 15450–15459 CrossRef CAS PubMed
.
- C. E. Anson, A. Eichhöfer, I. Issac, D. Fenske, O. Fuhr, P. Sevillano, C. Persau, D. Stalke and J. Zhang, Synthesis and Crystal Structures of the Ligand-Stabilized Silver Chalcogenide Clusters [Ag154Se77(dppxy)18], [Ag320(StBu)60S130(dppp)12], [Ag352S128(StC5H11)96], and [Ag490S188(StC5H11)114], Angew. Chem., Int. Ed., 2008, 47, 1326–1331 CrossRef CAS PubMed
.
- Y. Zhang, L.-Z. Dong, S. Li, X. Huang, J.-N. Chang, J.-H. Wang, J. Zhou, S.-L. Li and Y.-Q. Lan, Coordination environment dependent selectivity of single-site-Cu enriched crystalline porous catalysts in CO2 reduction to CH4, Nat. Commun., 2021, 12, 6390 CrossRef CAS PubMed
.
- W.-T. Wei, Y.-Z. Lu, W. Chen and S.-W. Chen, One-Pot Synthesis, Photoluminescence, and Electrocatalytic Properties of Subnanometer-Sized Copper Clusters, J. Am. Chem. Soc., 2011, 133, 2060–2063 CrossRef CAS PubMed
.
- A. Wüst, L. Schneider, A. Pomowski, W. G. Zumft, P. M. H. Kroneck and O. Einsle, Nature's way of handling a greenhouse gas: the copper-sulfur cluster of purple nitrous oxide reductase, Biol. Chem., 2012, 393, 1067–1077 CrossRef PubMed
.
- T. A. D. Nguyen, Z. R. Jones, B. R. Goldsmith, W. R. Buratto, G. Wu, S. L. Scott and T. W. Hayton, A Cu25 Nanocluster with Partial Cu(0) Character, J. Am. Chem. Soc., 2015, 137, 13319–13324 CrossRef CAS PubMed
.
- A. Ghosh, R.-W. Huang, B. Alamer, E. Abou-Hamad, M. Nejib, O. F. Mohammed and O. M. Bakr, [Cu61(StBu)26S6Cl6H14]+: A Core–Shell Superatom Nanocluster with a Quasi-J36 Cu19 Core and an “18-crown-6” Metal-Sulfide-like Stabilizing Belt, ACS Mater. Lett., 2019, 1, 297–302 CrossRef CAS
.
- S. G. Bratsch, Standard Electrode Potentials and Temperature Coefficients in Water at 298.15 K, J. Phys. Chem. Ref. Data, 1989, 18, 1–21 CrossRef CAS
.
- X. Kang and M.-Z. Zhu, Intra-cluster growth meets inter-cluster assembly: The molecular and supramolecular chemistry of atomically precise nanoclusters, Coord. Chem. Rev., 2019, 394, 1–38 CrossRef CAS
.
- Q. Tang, G.-X. Hu, V. Fung and D. Jiang, Insights into Interfaces, Stability, Electronic Properties, and Catalytic Activities of Atomically Precise Metal Nanoclusters from First Principles, Acc. Chem. Res., 2018, 51, 2793–2802 CrossRef CAS PubMed
.
- L.-C. Liu and A. Corma, Metal Catalysts for Heterogeneous Catalysis: From Single Atoms
to Nanoclusters and Nanoparticles, Chem. Rev., 2018, 118, 4981–5079 CrossRef CAS PubMed
.
-
M. W. Hosseini, Thia-, Mercapto-, and Thiamercapto-Calix[4]arenes in Calixarenes 2001, ed. Z. Asfari, V. Böhmer, J. Harrowfield, J. Vicens and M. Saadioui, Kluwer Academic Publishers, Dordrecht, 2002, pp. 110–129 Search PubMed
.
- Y.-F. Bi, S.-C. Du and W.-P. Liao, Thiacalixarene-based nanoscale polyhedral coordination cages, Coord. Chem. Rev., 2014, 276, 61–72 CrossRef CAS
.
- X.-X. Hang and Y.-F. Bi, Thiacalix[4]arene-supported molecular clusters for catalytic applications[J], Dalton Trans., 2021, 50, 3749–3758 RSC
.
- X.-X. Hang, B. Liu, X.-F. Zhu, S.-T. Wang, H.-T. Han, W.-P. Liao, Y.-L. Liu and C.-H. Hu, Discrete {Ni40} Coordination Cage: A Calixarene-Based Johnson-Type (J17) Hexadecahedron, J. Am. Chem. Soc., 2016, 138, 2969–2972 CrossRef CAS PubMed
.
- H.-T. Han, L. Kan, P. Li, G.-S. Zhang, K.-Y. Li, W.-P. Liao, Y.-L. Liu, W. Chen and C.-H. T. Hu, 4.8 nm Concave {M72} (M=Co, Ni, Fe) metal-organic polyhedra capped by 18 calixarenes, Sci. China: Chem., 2021, 64, 426–431 CrossRef CAS
.
- D.-T. Geng, X. Han, Y.-F. Bi, Y.-C. Qin, Q. Li, L.-L. Huang, K. Zhou, L.-J. Song and Z.-P. Zheng, Chem. Sci., 2018, 9, 8535–8541 RSC
.
- Z. Wang, H.-F. Su, Y.-W. Gong, Q.-P. Qu, Y.-F. Bi, C.-H. Tung, D. Sun and L.-S. Zheng, A hierarchically assembled 88-nuclei silver-thiacalix[4]arene nanocluster, Nat. Commun., 2020, 11, 308 CrossRef CAS PubMed
.
- Z. Wang, F. Alkan, C. M. Aikens, M. Kurmoo, Z. Zhang, K. Song, C. Tung and D. Sun, An Ultrastable 155−Nuclei Silver Nanocluster Protected by Thiacalix[4]arene and Cyclohexanethiol for Photothermal Conversion, Angew. Chem., Int. Ed., 2022, 61, e202206742 CAS
.
- L.-L. Zhang, D.-W. Liang, Y. Wang, D. Li, J.-H. Zhang, L. Wu, M.-K. Feng, F. Yi, L.-Z. Xu, L.-D. Lei, Q. Du and X.-J. Tang, Caged circular siRNAs for photo-modulation of gene expression in cells and mice, Chem. Sci., 2018, 9, 44–51 RSC
.
- C.-K. Zhang, Z. Wang, W.-D. Si, L.-Y. Wang, J.-M. Dou, Z.-Y. Gao, C.-H. Tung and D. Sun, Solvent-Induced Isomeric Cu13 Nanoclusters: Chlorine to Copper Charge Transfer Boosting Molecular Oxygen Activation in Sulfide Selective Oxidation, ACS Nano, 2022, 16, 9598–9607 CrossRef CAS PubMed
.
- Z. Wang, L. Li, L. Feng, Z.-Y. Gao, C.-H. Tung, L.-S. Zheng and D. Sun, Solvent–Controlled Condensation of [Mo2O5(PTC4A)2]6− Metalloligand in Stepwise Assembly of Hexagonal and Rectangular Ag18 Nanoclusters, Angew. Chem., Int. Ed., 2022, 61, e202200823 CAS
.
- Z. Wang, H.-F. Su, L.-P. Zhang, J.-M. Dou, C.-H. Tung, D. Sun and L. Zheng, Stepwise Assembly of Ag42 Nanocalices Based on a MoVI-Anchored Thiacalix[4]arene Metalloligand, ACS Nano, 2022, 16, 4500–4507 CrossRef CAS PubMed
.
- Y.-F. Bi, W.-P. Liao, X. Wang, R.-P. Deng and H.-J. Zhang, Self–Assembly from Two–Dimensional Layered Networks to Tetranuclear Structures: Syntheses, Structures, and Properties of Four Copper–Thiacalix[4]arene Compounds, Eur. J. Inorg. Chem., 2009, 2009, 4989–4994 CrossRef
.
- N. Frank, A. Dallmann, B. Braun-Cula, C. Herwig and C. Limberg, Mercaptothiacalixarenes Steer 24 Copper(I) Centers to form a Hollow–Sphere Structure
Featuring Cu2S2 Motifs with Exceptionally Short Cu…Cu Distances, Angew. Chem., 2020, 132, 6801–6805 CrossRef
.
- Q.-L. Guo, W.-X. Zhu, S. Gao, S.-L. Ma, S.-J. Dong and M.-Q. Xu, A novel 2D coordination polymer based on a copper(II) tetramer with p-sulfonated thiacalix[4]arene, Inorg. Chem. Commun., 2004, 7, 467–470 CrossRef CAS
.
- G. Karotsis, S. Kennedy, S. J. Dalgarno and E. K. Brechin, Calixarene supported enneanuclear Cu(ii) clusters, Chem. Commun., 2010, 46, 3884 RSC
.
- F.-J. Li and R.-J. Sa, Formation of Cu3,4(TCA), making the TCA complex a highly selective probe for Cu2+ detection: a TDDFT study, J. Mater. Chem. C, 2019, 7, 2443–2456 RSC
.
- R. E. Fairbairn, R. McLellan, R. D. McIntosh, M. A. Palacios, E. K. Brechin and S. J. Dalgarno, Oxacalix[4]arene-supported di-, tetra- and undecanuclear copper(II) clusters, Dalton Trans., 2014, 43, 5292–5298 RSC
.
- T. Kajiwara, N. Kon, S. Yokozawa, T. Ito, N. Iki and S. Miyano, Synthesis, Structure, and Ferromagnetic Behavior of Decacopper(II) Cluster Complex Supported by Hexaanionic p - tert- Butylthiacalix[6]arene, J. Am. Chem. Soc., 2002, 124, 11274–11275 CrossRef CAS PubMed
.
- Y.-F. Bi, W.-P. Liao and H.-J. Zhang, Assembly of Supramolecular Compounds with Water-Soluble Calix[4]arenes, Cryst. Growth Des., 2008, 8, 3630–3635 CrossRef CAS
.
- N. Iki, C. Kabuto, T. Fukushima, H. Kumagai, H. Takeya, S. Miyanari, T. Miyashi and S. Miyano, Synthesis of p-tert-Butylthiacalix[4]arene and its Inclusion Property, Tetrahedron, 2000, 56, 1437–1443 CrossRef CAS
.
- S. Kitagawa, M. Munakata, H. Shimono, S. Matsuyama and H. Masuda, Synthesis and Crystal Structure of Hexanuclear Copper(I) Complexes of 3-Pyridine-2-thionate, J. Chem. Soc., Dalton Trans., 1990, 2105–2109 RSC
.
- G. M. Sheldrick, Crystal structure refinement with SHELXL, Acta Crystallogr., Sect. B: Struct. Sci., 2015, 71, 3–8 CrossRef PubMed
.
- Y. Fang, W.-X. Xie, K. Han, K. Zhou, L. Kang, J. Shi, B.-K. Chen and Y.-F. Bi, Diphosphine modified copper(I)-thiacalixarene supramolecular structure for effective photocurrent response and photodegradation of methylene blue, Polyhedron, 2022, 222, 115934 CrossRef CAS
.
- D.-N. Yu, Z.-X. Yao, F. Bigdeli, X.-M. Gao, X. Cheng, J.-Z. Li, J.-W. Zhang, W. Wang, Z.-J. Guan, Y. Bu, K.-G. Liu and A. Morsali, Synthesis and Study of Photothermal Properties of a Mixed-Valence Nanocluster CuI/CuII with Strong near-Infrared Optical Absorption Supported by 4-tert-Butylcalix[4]arene Ligand, Inorg. Chem., 2023, 62, 401–407 CrossRef CAS PubMed
.
- A. K. Das, S. Biswas, V. S. Wani, A. S. Nair, B. Pathak and S. Mandal, [Cu18H3(S-Adm)12(PPh3)4Cl2]: fusion of Platonic and Johnson solids through a Cu(0) center and its photophysical properties, Chem. Sci., 2022, 13, 7616–7625 RSC
.
- C.-F. Sun, N. Mammen, S. Kaappa, P. Yuan, G.-C. Deng, C.-W. Zhao, J.-Z. Yan, S. Malola, K. Honkala, H. Häkkinen, B. K. Teo and N.-F. Zheng, Thiolated Copper–Hydride Nanoclusters as Single-Site Hydrogenation Catalysts for Ketones in Mild Conditions, ACS Nano, 2019, 13, 5975–5986 CrossRef CAS PubMed
.
- H.-Y. Zhuo, H.-F. Su, Z.-Z. Cao, W. Liu, S.-A. Wang, L. Feng, G.-L. Zhuang, S.-C. Lin, M. Kurmoo, C.-H. Tung, D. Sun and L.-S. Zheng, High-Nuclear Organometallic Copper(I)-Alkynide Clusters: Thermochromic Near-Infrared Luminescence and Solution Stability, Chem. – Eur. J., 2016, 22, 17619–17626 CrossRef CAS PubMed
.
- C. Gao, J. Wang, H. Xu and Y. Xiong, Coordination chemistry in the design of heterogeneous photocatalysts, Chem. Soc. Rev., 2017, 46, 2799–2823 RSC
.
- T.-T. Zhuang, Y. Liu, Y. Li, Y. Zhao, L. Wu, J. Jiang and S.-H. Yu, Integration of Semiconducting Sulfides for Full-Spectrum Solar Energy Absorption and Efficient Charge Separation, Angew. Chem., Int. Ed., 2016, 55, 6396–6400 CrossRef CAS PubMed
.
- Z.-X. Yao, J.-Z. Li, H.-H. Wang, X. Cheng, L.-L. Hou, D.-N. Yu, D.-L. Chen, W.-Y. Dan and K.-G. Liu, Construction of eight mixed-valence pentanuclear CuI4CuII clusters using ligands with inhomogeneous electron density distribution: synthesis, characterization and photothermal properties, Dalton Trans., 2022, 51, 6053–6060 RSC
.
- X.-F. Chen, G.-H. Zhang, B. Li and L.-X. Wu, An integrated giant polyoxometalate complex for photothermally enhanced catalytic oxidation, Sci. Adv., 2021, 7, eabf8413 CrossRef CAS PubMed
.
- J.-W. Zhang, L.-X. Wang, X. Cheng, L.-L. Hou, J.-Z. Li, C.-F. Wang, X.-W. Yan and K.-G. Liu, Use chloride to assist in constructing of penta- and nano-nucleus mixed-valent Cu(I/II) clusters and their photo-thermal properties, Inorg. Chim. Acta, 2023, 545, 121270 CrossRef CAS
.
- D.-X. Xu, Z.-D. Li, L.-S. Li and J. Wang, Insights into the Photothermal Conversion of 2D MXene Nanomaterials: Synthesis, Mechanism, and Applications, Adv. Funct. Mater., 2020, 2000712 CrossRef CAS
.
Footnote |
† Electronic supplementary information (ESI) available: Experimental details, DFT calculations, and additional figures and tables. CCDC 2246463. For ESI and crystallographic data in CIF or other electronic format see DOI: https://doi.org/10.1039/d3qi00479a |
|
This journal is © the Partner Organisations 2023 |
Click here to see how this site uses Cookies. View our privacy policy here.