DOI:
10.1039/D4ME00010B
(Review Article)
Mol. Syst. Des. Eng., 2024,
9, 800-813
Fabrication of a photothermal antibacterial platform for bacterial infectious skin wound healing: a review
Received
12th January 2024
, Accepted 11th April 2024
First published on 15th April 2024
Abstract
Antibiotics are currently the main strategy to treat bacterial infections, but they can cause antimicrobial resistance. Thus, it is urgent to solve this problem. The emergence of photothermal therapy provides a new opportunity for the prevention and control of bacterial infection. In recent years, photothermal agents have been widely used in infection control and wound healing due to their strong antibacterial properties and low drug resistance. Photothermal agents (PTAs) are nanomaterials themselves, or small molecules loaded in nanoparticles, and are the basic elements of PPT. In this review, we discuss the characteristics of wound dressings in skin wound healing, types and main functions of antibacterial photothermal therapy (PTA), and the fabrication and application of wound dressings. Finally, the current challenges and future development of PTAs as a photothermal antibacterial platform for wound healing are summarized and discussed.
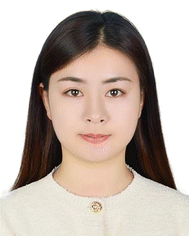 Mei Han | Mei Han is a Master's student at the School of Light Industry and Textile, Qiqihar University. She is working with Dr. Li's research group. Her research interests involve the synthesis of auxiliaries and their application in polymer materials. |
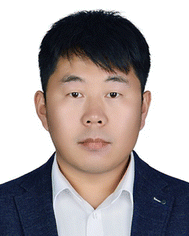 Hongbin Li | Hongbin Li received his Ph.D. degree in chemical engineering and technology at Harbin Institute of Technology in 2022. He is a Professor of Qiqihar University now. From 2018 to 2019, he worked as a visiting scholar at Harvard Medical School. His research interests are in polymeric biomaterials as scaffolds for in vitro tissue models. His current work focuses on the design of multi-function hydrogel bioinks for 3D DLP bioprinting in the field of tissue models. |
Design, System, Application
Antibiotics are currently the main strategy to treat bacterial infections, but they can cause bacterial resistance, and it is urgent to solve this problem. The emergence of photothermal therapy provides a new opportunity for the prevention and control of bacterial infection. The preparation of photothermal antibacterial platform for bacterial infection wound is very important. In recent years, photothermal agents have been widely used in infection control and wound healing due to their strong antibacterial properties and low drug resistance. PTAs are nanomaterials themselves, or small molecules loaded in nanoparticles, are the basic elements of PTT. In this review, we discuss the characteristics of wound dressings in skin wound healing, types and main functions of antibacterial photothermal therapy (PTA), fabrication and application of wound dressings. Finally, the current challenges and future development of PTAs as a photothermal antibacterial platform for wound healing are summarized and discussed.
|
Introduction
The skin is the first defense system and an important part of the human body, which is responsible for protecting various organs and tissues in the body from external factors, sensing external stimuli, and absorbing external material.1,2 However, in our daily life, the skin is exposed to the outside world for a long time, and it is easy to injure and cause wounds. Wound healing requires a complex and long-drawn process and can be categorized into four overlapping (but well-defined) stages: initial hemostasis, inflammation, hyperplasia, and wound remodeling.3,4 At the same time, it is also necessary to maintain sterile breathable, moist conditions and proper temperature during wound healing to facilitate the formation of wound epithelial tissue and promote the growth of granulation tissue and wound healing.5 If there is no effective treatment strategy for the wound, the wound will be prone to bacterial infection leading to a chronic inflammatory state, and even nonhealing.6 Therefore, the timely application of an effective antibacterial wound dressing is of great significance for promoted wound healing. Wound dressings provide protection and support for epithelialization or transition to permanent reconstruction by providing a moist, low-oxygenated, slightly acidic environment that temporarily acts as part of the skin's barrier during wound healing.7–9 An ideal wound dressing not only should maintain the physical environment of the wound but also protect the wound sites from bacterial infections.10–12
To date, a variety of wound dressings have been developed in clinical practice, which can be divided into traditional dressings and modern dressings. Traditional dressings, also known as inert dressings, include the use of gauze, cotton, and bandages.13,14 Modern dressings include foam, sponges, hydrogels, polymeric films, electrospun fibers, and other materials.15–20 Compared with the above passive wound dressings, wound dressing combined with antibiotics or antibacterial agents is more conducive to wound healing and more effective at combating bacterial infections.21 Common antibiotics are tetracycline, fluoroquinolone, cephalosporin, penicillin, and others, and the mechanisms by which they destroy bacteria include affecting the cell wall biosynthesis, enhancing the membrane permeability, preventing protein synthesis, and blocking DNA and RNA synthesis.22–25 However, due to the misuse and abuse of antibiotics, the emergence of multiple drug-resistant bacteria has brought great challenges to antibacterial agents.26,27 Therefore, it is urgent to develop novel technologies and platforms to accelerate the healing of wound infections. Inexplicably, an increasing number of researchers have begun to conduct synergistic antibacterial studies because of the low efficiency of single antibacterial modalities on the wound site.28,29 In past decades, some antibiotic alternatives in the fight against bacterial proliferation have been developed, including photothermal therapy (PPT) and photodynamic therapy (PDT).30–34 Among these antibiotic-independent methods, photothermal therapy (PPT) is considered a promising treatment model to combat bacterial infection and is widely used in the biomedical field.35–39 PPT sterilization has the advantages of simplicity, low cost, broad-spectrum, non-invasiveness, no drug resistance, minimal side effect, and high efficiency.40 PPT relies on photothermal agents (PTA) to convert the absorbed light energy into heat under near-infrared (NIR) light irradiation. The NIR wavelength range is generally from 700 to 1000 nm, providing a thermal effect that causes cell membrane rupture, denaturation of proteins and inactivation of bacteria. PDT is when the laser irradiates the photosensitizer. Singlet oxygen (1O2) can destroy essential bacterial components, such as proteins, lipids and nucleic acids, resulting in bacterial inactivation. However, PDT is often limited by factors such as poor water solubility of the photosensitizer, short half-life, and limited diffusion distance.41–45 At present, it is known through research that PPT treatment can resist the infection of bacteria, such as E. coli, S. aureus, P. aeruginosa, Candida albicans, Saccharomyces cerevisiae and others.46
Up to now, a variety of nanoplatforms with excellent photothermal conversion efficiency have been exploited and designed, such as carbon-based materials,47 noble metal nanostructures,48 conjugated polymers,49,50 and metal–organic frameworks.51 The ideal PTA needs to have biosafety, economy and high efficiency for photothermal conversion. Bacterial infection is an important problem in wound healing that can lead to the formation of chronic wounds. Thus, PPT has great application prospects for accelerating angiogenesis and controlling infection to synergistically heal wounds.52–55
To promote the advancement of photothermal antibacterial materials-based wound dressing, we herein present a comprehensive and current summary of the photothermal antibacterial platforms for bacterial infectious wound healing. This review covers wound dressings, overview of PTA, fabrication and application of wound dressings, conclusion and future perspectives.
Overview of wounds dressing in skin wound healing
Properties of an ideal wound dressings
The ideal wound dressing should be sufficient for all aspects of the wound healing process (Fig. 1). Firstly, the raw material of the wound dressing should be selected with good biocompatibility, and will not cause immune rejection and biological toxicity.56 Secondly, wound dressing requires a lower cost, and should provide softness, comfort, conformability and flexibility to improve the patient quality of life. The conformability and flexibility can prevent skin tears and thus wound infection.57 Meanwhile, an ideal wound dressing also should have good water permeability, adherence to the tissue, resistance to shearing forces and mechanical performance.58 Furthermore, the dressing should maintain a moist environment, absorb the exudate to prevent maceration, maintain the temperature at 35–37 °C, and ensure the gas exchange between the wound dressing and outside world.59 Finally, the wound dressing should have certain antibacterial and bactericidal ability, and avoid skin stripping at removal and reapplication to promote a faster and more complete healing of the wound.60,61
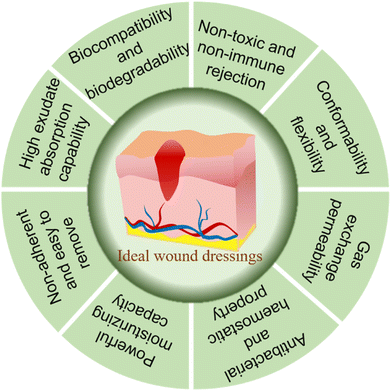 |
| Fig. 1 Characteristics of ideal wound dressings. | |
Types and functions of wounds dressings
As schematized in Table 1, wound dressing can be divided into traditional and modern wound dressings. Traditional wound dressings belong to passive dressings, also known as inert dressings, and are usually used to keep wounds dry and clean, protect wounds from contact with the outside world, and to stop bleeding.62,63 The traditional dressings examples are gauze, gauze-cotton composites, tulle and bandages, which are characterized by their low cost, high absorption capacity and simple production process. The main function of traditional dressings is to absorb exudates and fluids of wounds.64–66 However, owing to the difficulty in maintaining a moist wound environment, traditional dressings easily stick to the wound surface. This will cause secondary injury without an antibacterial effect.67,68
Table 1 Classification of the wound dressing materials
Type of wound dressings |
Examples |
Functions of wound management |
Ref. |
Traditional dressings |
Gauze, bandages, tulle, etc. |
Protect the wound tissue, stop bleeding and absorb wound exudate |
64
|
Interactive dressings (modern) |
Spray, films, sponges, hydrogels, foams, composites, etc. |
Prevent bacterial infection, low cost, provide a moist environment for the wound, long shelf life, enhance the water vapor transmission rate (WVTR) and well mechanical properties |
69
|
Biomaterial-based dressings (modern) |
Allograft, xenografts, and autografts |
They replace the damaged skin |
70
|
Bioactive dressings (modern) |
Hydrogels, wafers, sponges, films, nanofibers, foams, and membranes |
Encapsulated with bioactive agents, such as antimicrobials and growth factors, to improve the wound healing process |
71
|
Due to the shortcomings of traditional wounds, they have been superseded by modern dressings. Modern dressings are classified as interactive, biomaterial-based and bioactive dressings based on their applications.69 Interactive dressings, also known as artificial dressings, include spray, films, sponges, hydrogels, foams, composites, etc.70 The advantages of interactive wound dressings are that they can prevent bacterial infection, are low cost, provide a moist environment for the wound, have a long shelf life, and enhance the water vapor transmission rate (WVTR) and well mechanical properties.71 They are usually formulated from biopolymers and synthetic polymers. Among them, gelatin, chitosan and alginate are the most widely used.72
Biomaterial-based wound dressings are classified as allografts, xenografts and tissue derivatives. The survival time of allografts at the wound is short, and there is a possibility of infection and disease transmission.73 Fresh or dried fragments of the skin are typically collected from a donor. However, its use as a transplant is limited by the immune response, leading to exclusion by the body. Xenotransplantation refers to tissue transplants or organ transplants from donors of different species, such as animals to humans.74,75 Tissue derivatives are obtained from collagen, but their use is limited due to the risk of infection.
Bioactive dressings are the addition of growth factors and antibacterial agents to improve the wound healing process. Examples of bioactive wound dressings include tissue-engineered, nanoparticles loaded wound dressings, antimicrobial dressings, self-removal smart wound dressings, 3D bioprinted dressings, etc.76–78
Photothermal therapy antimicrobial mechanism
Compared with antibiotics, PPT has many advantages, including short treatment time and a wide range of antibacterial effects. Furthermore, bacterial resistance is not obvious. Because PTT is a non-invasive therapy, bacteria cannot develop resistance to PTT through drug excretion, metabolic promotion, and delayed absorption as they do with antibiotics. In clinical application, the PTA should have good biocompatibility and biodegradability, high photothermal conversion efficiency, excellent photothermal stability and simple preparation method.79,80 In accordance with the PTA used, there are different mechanisms for photothermal conversion. The photothermal antibacterial effect of noble metal-based materials depends on the absorption of visible light radiation and formation of a plasma. Then, the resulting heat is transferred to the surrounding bacteria at high speed to induce cell or bacterial death. There are strong resonance absorption peaks in the spectrum.81 The effect of photothermal sterilization is related to the size, shape and dielectric constant of the nanomaterial particles.82
The carbon-based nanomaterial and conjugated polymer materials produce photothermal antibacterial effects through the lattice vibration of molecules. 3D (metal–organic frameworks and covalent organic frameworks) materials are a new kind of crystalline porous coordination polymer material. Among them, the antibacterial mechanism of metal–organic frameworks and covalent organic framework (MOFs) is related to its chemical properties and structure, which can be divided into the following three categories: metal ion releasing system, oxidative stress and as a carrier of antibacterial drugs.83–85 Covalent organic frameworks (COFs) have durable antibacterial properties. COFs have a regulated pore size and structure, and can be designed as a material with specific functional groups that can interact with the bacterial cell membrane to destroy the bacterial cell structure or damage the cell membrane and play an antibacterial role. Under NIR irradiation, COFs can produce photothermal effects to kill Escherichia coli (E. coli) and Staphylococcus aureus (S. aureus).86
Types and main functions of antibacterial photothermal therapy (PTA)
As is known to all, PTAs plays an important role in PPT. PTAs should have excellent biocompatibility, ease of synthesis and modification, high photothermal conversion efficiency (PCE) and light stability, and the ability to be quickly cleared from the body after use, etc.87 It can be divided into metal-based nanomaterials, carbon-based nanomaterials, conjugated polymers (Cps) and 3D (covalent organic framework and metal–organic framework) materials, which have been extensively studied (Fig. 2).
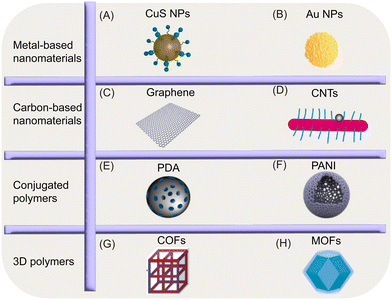 |
| Fig. 2 Illustrations of the nanostructures of various PTAs. (A–H). Reproduced from ref. 80, copyright 2023, Elsevier. | |
Metal-based nanomaterials
Transition metal oxide/sulfide nanomaterials.
Transition metal oxide/sulfide nanomaterials consist of transition metal elements and oxygen or sulfur elements, including CuS, MnO2, TiO2, ZnO, etc.88,89 Compared with the noble metal, the transition metal oxide/sulfide nanomaterials cost is lower.90 Transition metal oxide/sulfide nanomaterials have been widely studied because of their abundant resources, stable chemical properties, various configurations and valence states.91 Transition metal oxides/sulfides-based antibacterial nanomaterials have become the focus of research on photothermal antibacterial materials due to their advantages of environmental friendliness, low cost, controllable structure, various preparation methods and good photothermal conversion effect.92,93 The mechanism of the photothermal conversion of transition metal oxide/sulfide nanomaterials is still controversial. Some researchers believe that the photothermal conversion is caused by the localized surface plasmon resonance (LSPR). Some researchers believe that when NIR light is irradiated, it will produce photoinduced charge carriers. Their recombination will absorb a large amount of photon energy and convert it into heat energy, making the local temperature rise rapidly. However, it needs further research.94 Therefore, in PPT, the cell wall of the bacteria wrinkles or breaks due to high temperature, thus damaging the cell membrane, leaking the cytoplasm, and eventually causing the death of the bacteria.95,96
Noble metal nanoparticles.
Noble metal nanoparticle PTAs, including Au, Ag, and Cu, can absorb the energy of light and produce free electrons of specific wavelengths that oscillate at the surface. This photophysical phenomenon, known as local surface plasmon resonance (LSPR), is capable of generating heat and inducing bacterial death.97
Au NPs are most commonly used for their longitudinal surface plasmon resonance under near-infrared laser illumination. The property is related to their shape, structures and size, such as nanorods, nanostars, nanowires, nanobipyramids, nanoworms and nanoflowers.98 The chemical properties of Ag are similar to those of Au. They all have the same LSPR effect, large specific surface area and enhanced optical properties. However, the biological toxicity of Ag cannot be ignored. The outstanding properties of Ag NPs are photothermal and antibacterial, widely used in biomedical fields such as wound healing. When Ag NPs were used alone as antibacterial agents, Ag NPs could interact with phosphate and sulfur-containing proteins inside and outside the cells to inhibit bacterial growth. When Ag NPs combine with PTT and a hydrogel, the hydrogel coated on the surface of the wound is heated locally under near-infrared light, damaging the cell membrane and proteins of the bacteria, resulting in bacterial death.99 Cu is one of the important trace elements in the human body, and is responsible for regulating many cytokines and growth factors, so it is often used to stimulate wound healing. Meanwhile, the d–d band transition of Cu2+ can quickly and effectively eradicate the infection of pathogens. The Cu-containing nanomaterials also have excellent photothermal conversion properties. Therefore, people are interested in Cu as an antibacterial agent, and people have begun to design copper-containing metal composites and apply them to wound dressings. For example, the copper-based nanomaterials are embedded in hydrogels and their photothermal properties are used to destroy bacteria.100
In recent years, due to the shortcomings of single noble metal materials (such as their high cost and high biological toxicity), bimetallic nanoparticles have attracted wide attention due to their excellent physicochemical, catalytic and optical properties, as well as higher chemical and physical stability compared with single metal particles.101
Carbon-based nanomaterial.
Carbon-based nanomaterials include graphene-based nanomaterials (GBNs), carbon nanotubes (CNTs) and MXene, which has been widely studied as a new carbon-based material in recent years. Carbon-based nanomaterials have many advantages, including its excellent optical, thermal/electronic, low cost, high thermal conductivity and mechanical properties, so it is widely used as the PTA. However, its disadvantage as the PTA is poor biocompatibility and low antibacterial activity caused by photothermal effects compared with precious metals.102
Graphene and graphene-based nanocomposites showed strong optical absorbance in the near-infrared (NIR) region. Thus, it can be used as a photothermal agent, and it can also ablate tumors in cancer treatment and kill bacteria effectively.103 Carbon nanotubes (CNTs) are nano-biomaterials of small size and large surface area, which exhibit unique interaction patterns with biomolecules, cells and even natural tissues, thus enhancing the biological activity of wound dressings.104
Among these photothermal materials, MXene stands out for its high aspect ratio, atomically thin thickness, excellent photothermal properties, low toxicity, and ultra-high dispersion in water-based systems. MXene pertains to a 2D layered material composed of transitional metal carbides and nitrides or carbon nitrides. The chemical formula of MXene is Mn+1XnTX, where M represents the early transition metal (Sc, T, Zr, Hf, V, Nb, Ta, Cr, Mo), X is carbon or nitrogen (C/N), and TX is the surface functional group (–OH, OH, –O or –F), n = 1, 2, 3.105 MXene has strong light absorption properties throughout the ultraviolet (UV), visible light and near infrared (NIR) regions. From the UV-visible diffuse reflection of MXene, it can be seen that MXene samples exhibit strong absorption in the range of 250–800 nm.106 The UV-visible-near-infrared absorption spectra of MXene show unique absorption in the 750–850 nm region, showing LSPR effects. MXene's absorption range is just in the biological NIR range, and NIR light has minimal damage, spatial controllability and maximum tissue penetration, so MXene can be applied to photothermal antibacterial under the action of NIR light.107,108 The current research focuses on the application of 808 nm wavelength near-infrared light to trigger PPT, which is because 808 nm-NIR light shows lower water absorption and enhanced tissue penetration.109Table 2 shows some of the photothermal antibacterial properties of MXene. MXene is prepared by acid etching by selectively removing the “A” layer from its MAX or non-Max phase parent, where A is primarily a group 13 or 14 element in the periodic table. However, due to the high negative surface charge of MXenes, it is difficult to electrostatically adsorb MXenes to anionic bacterial membranes. This indicates that when heat is transferred from MXenes to bacteria, there is a pathway from MXene to the environment to the bacteria, which extends the heat transfer distance and reduces the efficiency of PPT. It takes 70 °C to play the antibacterial effect, which will increase the pain of patients, so structural modification is needed to improve these shortcomings.110,111
Table 2 Photothermal antibacterial properties of MXene-based materials
MXene-based materials |
Wavelength |
Type of bacteria |
Inhibition rate |
Ref. |
MXene@Fe3O4/Au/PDA |
808 nm |
E. coli and S. aureus |
100% |
108
|
Cu(II)@MXene |
808 nm |
E. coli and S. aureus |
80% |
112
|
MXene–Au |
808 nm |
E. coli and B. subtilis |
99.25% and 100% |
113
|
MXene–chitosan |
808 nm |
Against methicillin-resistant Staphylococcus aureus (MRSA) |
99.18% |
114
|
Ti3C2Tx@CuS |
808 nm |
E. coli and S. aureus |
99.6% and 99.1% |
115
|
MXene/ZIF-8/PLA |
808 nm |
E. coli and methicillin-resistant Staphylococcus aureus (MRSA) |
99.9% and 99.8% |
116
|
BiOI@Bi2S3/MXene |
808 nm |
P. aeruginosa and S. aureus |
99.7% and 99.8% |
117
|
Ag2S/Ti3C2 |
808 nm |
S. aureus
|
99.99% |
118
|
Conjugated polymers (Cps)
Cps are newly minted organic compounds, and contain large π-conjugated skeletons and delocalized electron structures, giving them excellent light capture and optical amplification capabilities. Currently, Cps are widely used in biological and medical fields for antimicrobial/antitumor therapy, biosensing, bioimaging, and gene/drug delivery due to their excellent photothermal properties. Cps include polydopamine (PDA), polyaniline (PANI), poly(3,4-ethylenedioxythiophene) (PEDOT) and polypyrrole (PPy).119,120
PDA, due to its mussel-inspired adhesive properties, can be easily coated onto almost any surface, and can confer photothermal properties on the nanoparticles it is coated with. PAIN is the most promising PTA due to its excellent biodegradability, electrical conductivity, stability, low cost, high photothermal conversion efficiency and mechanical flexibility.121 PEDOT has the advantages of satisfactory conductivity, environmental stability, biocompatibility and photothermal conversion performance, and has been widely used in the fields of flexible tensile sensors, energy storage devices, biosensors and photothermal therapy.122 PPy has attracted wide attention due to its advantages of good biocompatibility, photon stability, low cost, high photothermal conversion efficiency and simple preparation. However, pure PPy is less water-soluble. It must be doped/functionalized with other components to obtain better stability and water dispersion.123
3D (covalent organic framework and metal–organic framework) materials
Covalent organic frameworks (COFs)
Covalent organic frameworks (COFs) are crystalline porous polymers and newly developed multifunctional materials with excellent biocompatibility, inherent porosity, concurrently predesigned structure and tailorable function, and have been widely used in the biomedical field.124 COFs have the following advanced properties in anti-infection therapy: (1) high drug carrying capacity due to considerable specific surface area and pore volume; (2) biodegradability; (3) flexible structure and function; (3) high π electron density and photoelectric properties.125
Metal–organic frameworks (MOFs)
Metal–organic frameworks (MOFs) are a kind of inorganic–organic hybrid material consisting of an organic ligand and a metal node connected by a coordination bond. The zeolite-based imidazolyl skeleton (ZIF-8) is a subclass of MOF, which is a porous material comprising a zinc ion and 2-methylimidazole, and is expected to be an ideal nanocaller for effective chemical–photothermal combination therapy.126–129 MOFs have many excellent properties, such as high specific surface area, low skeleton density, adjustable uniform pore size, great thermal stability, more active sites and simple functionalization. In recent years, MOFs have been used to release bactericidal metal ions, load different small molecule drugs for wound chemotherapy, and produce large amounts of reactive oxygen species (ROS) or heat for photothermal sterilization, while demonstrating safe and efficient skin wound disinfection.130
Fabrication of wound dressings
To reduce the high risk of morbidity and mortality, researchers are working to develop antibacterial dressings to prevent contamination and speed wound healing. The above PTAs are integrated into different forms of biomaterials to accelerate wound healing. Different structures and forms of biomaterials include electrospinning scaffolds, three-dimension (3D) printed scaffolds for skin regeneration, hydrogels, films, sponges and foams.2,131 Various methods of preparing wound dressings are described below.
Electrospinning scaffold
Electrospinning is a common, low-cost, adjustable process used to produce nanofibers with some unique properties. Due to the flexibility of selecting raw materials and the possibility of adjusting the final properties, electrospinning technology has been widely used in biomedical materials such as tissue-engineered stents, wound dressings, and drug delivery systems.132 Compared with other fibers, the internal pore structure of electrospun nanofibers can be controlled, larger areas can be covered for higher healing capacity, and the fiber composition and structure can be changed according to the performance requirements. Its high porosity provides a fuller contact surface for gas exchange and liquid absorption, resulting in excellent permeability, keeping the wound moist when used as a wound dressing, and acting as a barrier against microbial invasion. At the same time, the high flexibility of the nanofiber itself makes the wound dressing suitable for different parts and different shapes of the wound, promoting wound healing, and may have a certain anti-scar formation potential. However, the electrospinning process has drawbacks, and a large number of parameters can affect the final product, including solution, process conditions, and environmental conditions, but once the appropriate parameters are reached, nanofiber production can proceed smoothly.133,134 Different types of bioactive agents are incorporated into electrospinning nanofibers, such as growth factors, anti-inflammatory compounds, and medicinal plant extracts, to enhance the healing process by gradually releasing the medium. It can also provide high drug-loading capabilities, embedding photothermic agents into nanofibers to trigger drug release via near-infrared irradiation. The photothermal agent converts near-infrared light into heat, and the photothermal effect destroys microorganisms to achieve the purpose of an antibacterial agent. In addition, they allowed skin wounds to heal in bacteria-infected mice by anti-infective activity, reducing pro-inflammatory factors, stimulating collagen deposition, and promoting the formation of dermis and skin attachments.135 Tian et al. first prepared Au@carbon dots (Au@CDs) composite nanoparticles (Au@CD) by surface modification of AuNPs with CD, and then embedded the Au@CD composite nanoparticles with a polyvinyl alcohol (PVA) membrane by electrospinning (Fig. 3A).136 After 5 minutes of NIR irradiation, the temperature of the wound treated by the Au@CD membrane can reach 50 °C, which proves that the Au@CD membrane is an effective wound dressing.
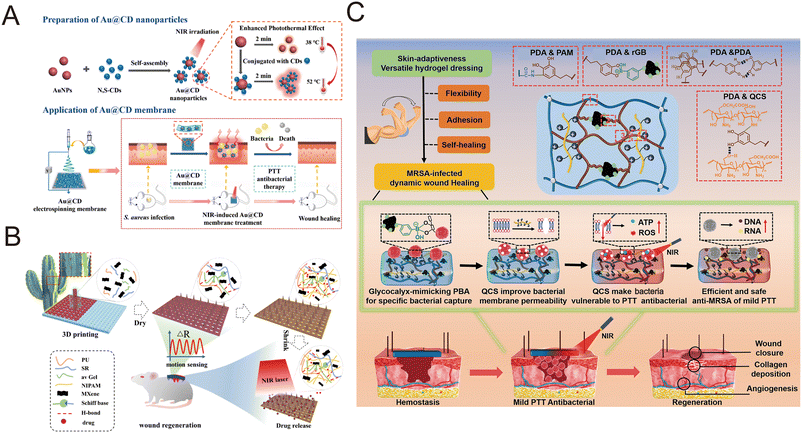 |
| Fig. 3 Fabrication of wound dressings. (A) Preparation of Au@CD nanoparticles and electrospinning scaffold for the preparation of wound dressings. Reproduced from ref. 136, copyright 2022, Elsevier. (B) Preparation of the 3D-printed MXene and spidroin-based microneedle scaffolds. Reproduced from ref. 139, copyright 2022, ACS Publication. (C) Preparation and application of the multifunctional hydrogel dressing. Reproduced from ref. 148, copyright 2023, Wiley. | |
3D printing scaffold
3D printing technology is widely regarded as the technology of the next global industrial and manufacturing revolution due to its high controllability and high resolution, and it is a key driver for the development of wound dressings.137 In skin repair, bioprinting technology can precisely match the geometry of wound healing materials and tissue defects, thus achieving rapid and effective wound healing. At present, bio-3D printing technology combined with a variety of functional materials can produce replicable, personalized 3D structures with antibacterial, anti-inflammatory, antioxidant, hemostatic, anti-tumor and other functions.138 Shao et al. extrudated hydrogel precursors composed of water-soluble polyurethane (PU), aloe vera gel (avGel), and recombinant spidroin doped with MXene solution into a 3D printer, and printed them onto the silicone rubber (Ecoflex) microneedle molds with an IO PC structure to prepare a hydrogel microneedle scaffold (MNS) (Fig. 3B).139 Xu et al. used a mixed bioink composed of sodium alginate (SA), gellan gum (GG), and polydopamine nanoparticles (PDA NPs) for 3D printing to prepare a porous scaffold SA-GG@PDA. The SA-GG@PDA hybrid hydrogel scaffold was then covered with a layer of hydrogel containing doxorubicin (DOX), which had excellent photothermal properties, good cellular compatibility, good mechanical properties, fast expansion rate and rich water content.140
Hydrogels
The main components of hydrogel wound dressings are three-dimensional macromolecular networks and water, of which the water content is 90%. The water content makes hydrogel dressings suitable for the treatment of dry and necrotic wounds, creating a moist environment for the wound.141–143 Three-dimensional polymer gels have a hydrophilic porous structure that can absorb large amounts of water, and studies have shown that each gram of dressing can absorb up to 1000 grams of wound exudate, depending on the composition of the hydrogel. Hydrogels are ideal dressings because of their non-adhesion, ductility, and similarity to living tissue. The permeable hydrogel structure promotes the exchange of CO2, O2 and H2, allowing the tissue to “breathe”.144–146 The crosslinking methods of hydrogels can be divided into physical crosslinking and chemical crosslinking. Physical crosslinking includes intermolecular interactions, such as ionic interaction, hydrogen bonding, hydrophobic association, and crystalline crosslinking with good biocompatibility and hypotoxicity. Chemical crosslinking is linked by covalent bonds between polymers, such as radical polymerization crosslinking and radiation crosslinking, with great mechanical properties.147 Zhao et al. designed a multifunctional hydrogel dressing based on the cross-linking system of polydopamine (PDA) and polyacrylamide (PAM), and added photothermal 3-aminobenzenoboric acid modified reduced graphene oxide (rGB) sheet and QAS modified carboxymethyl chitosan (QCS) to prepare a hydrogel (rGB/QCS/PDA-PAM) (Fig. 3C).148 The hydrogel of rGB/QCS/PDA-PAM can reach 49.6 °C in 600 s at 0.8 W cm−2. This temperature is below 50 °C, meeting the basic biosafety requirements of mild antibacterial PTAs. Zhang et al. incorporated Mn3O4 into an antibacterial chitin hydrogel to obtain bioactive chitin/Mn3O4 composite hydrogels for healing infected wounds. It showed excellent photothermal antibacterial and antibiofilm activities with the aid of near-infrared irradiation.149
Films
The film dressing consists of an adhesive, porous and thin transparent polyurethane.13 Films are thin and flexible polymers that are often used as semi-permeable dressings because they are able to protect the wound from the external environment and can adapt to different body structures, with oxygen, carbon dioxide and water vapor in the wound passing through the dressing, while liquids and bacteria do not penetrate it.2,150 Moreover, the film dressing has the characteristics of autolysis debridement, suitable for epithelialized wounds and superficial wounds with little exudate. The films dressing allows the wound to be examined without removing the dressing.57 Li et al. prepared a wound dressing CS/CuS/PCA/Gent film that combines diagnostic function and synergistic therapy (Fig. 4A).151 Firstly, bovine serum albumin (BSA) was used as a template to synthesize copper sulfide (CuS NPs), and then purple cabbage anthocyanins (PCA), CuS NPs and gentamicin (Gent) were introduced into the CS solution to prepare the dressing. Under NIR laser irradiation (0.75 W cm−2) at 808 nm, the temperature of the CS/CuS/PCA/Gent film increased to 45.51 °C. Furthermore, the temperature of the CS/CuS/PCA/Gent film can reach 50 °C under NIR laser irradiation (0.75 W cm−2) at 1064 nm. Chen et al. adopted a very simple method to prepare piezoelectric and photothermal bifocal films as wound dressing. Firstly, the films were prepared by air-drying chitosan solution, and then the chitosan films were immersed in dopamine solution to cover a layer of polydopamine (PDA) to obtain the polydopamine coating film (CM@DA). Wound healing is promoted through a pathway involving heat shock protein 90 (Hsp90) and hypoxia-inducing factor 1α (HIF-1α).152
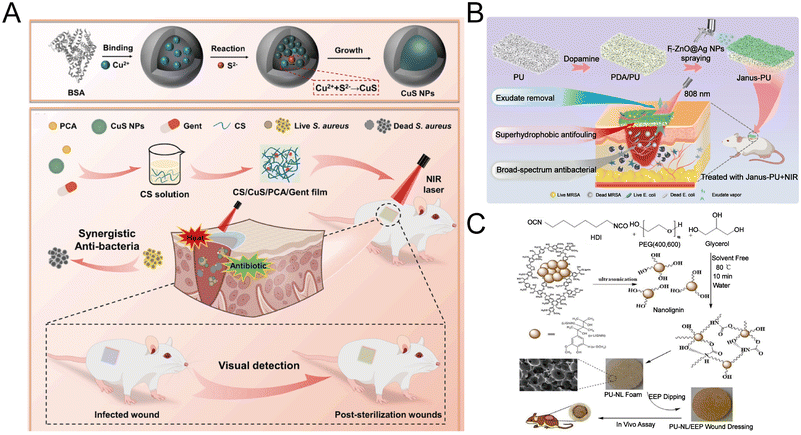 |
| Fig. 4 Fabrication of wound dressings. (A) Preparation of the CuS NPs and CS/CuS/PCA/Gent film and their application. Reproduced from ref. 151, copyright 2024, Elsevier. (B) Preparation and application of Janus-PU sponges. Reproduced from ref. 156, copyright 2023, Elsevier. (C) Preparation and application of PU-NL/EEP foams wound dressing. Reproduced from ref. 161, copyright 2021, MDPI. | |
Sponges
Dressings with shape recovery or shape adaptability have been shown to be more beneficial for hemostasis, as they can better adapt to the shape of the wound to increase the contact area while reducing damage. So, sponges might be an ideal wound dressing.153 The sponge has thermal insulation properties, and it has an interconnected porous structure that can absorb large amounts of wound exudates, so it has moisturizing properties.2,154 However, sponge dressings are not suitable for wounds with severe burns or dry eschar. A variety of sponge-based dressing systems have been developed to add to skin wound healing.155 In a study by Chen et al., silver nanoparticles (Ag NPs) were firstly in situ formed on ZnO and fluorinated to produce F-ZnO@Ag NPs hydrophobic nanoparticles. Then, F-ZnO@Ag NPs were coated on a dopamine-modified polyurethane sponge (PDA/PU) to obtain Janus-PU (Fig. 4B).156 Under NIR irradiation, the outer layer of Janus-PU rapidly heats up to 85.7 °C within 30 s, and the inner layer of Janus-PU can heat up to 49.7 °C and remain constant at 50.3 °C, proving that Janus-PU has a constant and powerful photothermal capacity.
Foams
Foam dressings are semi-permeable, hydrophilic or hydrophobic, have a bacterial barrier, insulation, and can fit on the body surface.13 Foam dressings are composed of polyurethane or silicone-based materials that are similar to sponges, and are another moisturizing material used to hold liquids that can absorb exudate and prevent maceration around the wound. At the same time, foam dressings also maintain hydration, allowing moisture to promote epithelialization and healing by promoting cell migration.157,158 However, foam dressings usually do not adhere to the skin, and a secondary dressing is needed as a cushion to prevent displacement. Furthermore, if the dressing is not replaced for a long time, the regenerated tissue will grow into the foam, which may cause secondary damage to the tissue, so its performance needs to be improved.159 Polyurethane (PU) foam is the most commonly used dressing, and is manufactured by a foaming process.160 Pahlevanneshan et al. prepared a composite polyurethane (PU) foam, which was synthesized with polyethylene glycol, glycerol, nanolignin (NL), 1,6-diisocyanate hexane and water as blowing agents, and then immersed in propolis ethanol extract (EEP) to obtain the PU-NL/EEP foam dressing (Fig. 4C).161 However, there are few research studies on the combination of foam and photothermal agent as wound dressing.
Application of the photothermal antibacterial platform
At present, photothermal therapy is widely used in various fields, such as bacterial infectious skin wound healing area, antibacterial water evaporation and purification, and flexible antibacterial textiles.31 This article mainly introduces the photothermal antibacterial platform for bacterial infectious skin wound healing.
Zhou et al. developed a system of CuS nanoparticle complex (CuS NC) hydrogels with various functions by incorporating polyethylene glycol (PEG)-functionalized CuS NPs with a surface amino group into a three-dimensional network of oxidized dextran (ODex) and PEG with amino groups.162 CuS NC hydrogels formed in situ not only have excellent self-healing and injection ability, but also have good biocompatibility. In their work, the morphologies of E. coli and S. aureus were observed by scanning electron microscopy. It was proved that the presence of CuS NPs greatly improved the antibacterial properties of hydrogels (Fig. 5A-i and -ii). The histological images of the isolated 16F10 tumors shown in Fig. 5A-iii showed that tumor cells in the G-CuS1(+) group had less proliferation and better anti-tumor effects than those in the other groups. The in vivo wound healing performance of G-CuS0.5 groups can better repair skin wounds (Fig. 5A-iv). Finally, in H&E staining images, the wound was completely covered by a newly formed epidermal layer, indicating that the introduction of CuS NPs gives the hydrogel excellent photothermal/photodynamic properties under near-infrared laser irradiation, giving it multiple capabilities to eradicate melanoma cells and colonize bacteria (Fig. 5A-v).
 |
| Fig. 5 Wound dressing for skin repair. (A) Photothermal hydrogel for skin wound repair prepared by CuS. (i and ii) SEM images of S. aureus and E. coli. (iii) H&E and TUNEL staining images of isolated B16F10 tumors in the antitumor experiment. (iv) Photographs of skin wounds treated with 3 M dressing and G-CuS0.5. (v) H&E, Masson and CD31 staining images of skin wounds at the 14th day. Reproduced from ref. 162, copyright 2021, Elsevier. (B) Hydrogel with the synergistic effect of PPT and PDT for the skin wound. (i) Bacterial survival of S. aureus in the absence or presence of near-infrared irradiation. (ii) Bacterial survival of E. coli in the absence or presence of near-infrared irradiation. (iii) Representative photographs of different groups of in vivo healing experiments. (iv) Stained images of skin wound sections of different groups. Reproduced from ref. 163, copyright 2024, Elsevier. | |
When PTT acts alone, the photothermal agent will generate local heat under the irradiation of NIR, but it will cause damage to neighboring healthy tissues. In order to solve this problem, PPT should be used in combination with photodynamic therapy (PDT) to achieve a synergistic antibacterial effect. Sun et al. successfully constructed NIR-responsive BC composite membranes functionalized by PDA and CuS NPs, which showed excellent bactericidal properties and reduced the risk of bacterial drug resistance due to the synergistic effect of PPT and PDT. The PDA coating was formed by self-polymerization of DA on the surface of the BC fiber, and CuS NPs were synthesized in situ based on the modified film. Under 808 nm NIR laser irradiation, heat generated by PDA and CuS NPs and ROS produced by CuS NPs acted simultaneously to eliminate bacteria. In addition, the release of Cu2+ in wound dressings can be controlled by a NIR laser, which can promote the formation and growth of blood vessels and kill bacteria.163 In their work, firstly, the antibacterial performance was analyzed, and it was known that the BC/PDA membrane did not have an obvious antibacterial effect in the absence of light. The antibacterial rate of S. aureus and E. coli under 808 nm laser irradiation was 17.8% and 24.6%, respectively. The bactericidal efficiency of BC/PDA/CuS5 samples against S. aureus and E. coli under NIR irradiation was 99.7% and 88.0%, respectively. It can be seen that PPT and PDT have the best synergistic antibacterial effect (Fig. 5B-i and ii). Then, in the in vivo wound healing experiment, the wound in the BC/PDA/CuS5 + NIR group was completely closed after 14 days, and the new skin tissue was regenerated, and the wound repair effect was the best (Fig. 5B-iii). Finally, in Fig. 5B-iv, H&E, Masson and CD31 staining showed that the BC/PDA/CuS5 + NIR group had the best wound healing effect, which was related to the synergistic effect induced by NIR.
Conclusions, challenges and outlook
PPT is an emerging wound healing treatment method which has been widely used in recent years because of its non-invasive and low drug resistance. This review first introduces the properties of ideal wound dressings, types and functions of wounds dressings and the mechanism of photothermal therapy antimicrobial, then reviews the types and main functions of photothermal agents, namely precious noble metal-based nanomaterials, carbon-based nanomaterials, carbon-based nanomaterials and 3D materials. Finally, the fabrication of wound dressings has been discussed. We should note that the wound is exposed, without full skin protection. The biosafety of the product must be considered before use, as nanomaterials come into direct contact with tissues not covered by the skin. Meanwhile, nanomaterials can also cause DNA damage and reduce gene methylation, which can lead to cancerous cell changes. Nanomaterials can enter broken blood vessels at the site of the wound, which can cause hemolysis or follow the blood to spread to various organs. Metal nanoparticles have potential toxicity and long-term potential safety, so later studies should improve this problem.
Noble metal nanoparticles materials and carbon-based nanomaterial have demonstrated excellent photothermal antibacterial wound healing effects, but they have disadvantages such as high cost and insufficient photothermal properties, which limit their application. PDA has the characteristics of rapid hemostasis, good anti-inflammatory effect, no compression, strong adhesion, antibacterial effect, and can promote wound healing by photothermal effect, which is a promising PTA. In recent years, some small molecular photothermic agents (such as PB) have high bioactivity and biodegradability, and are easily loaded into various nanomaterials, which have great potential in the field of wound healing.
With the rapid development of PTA, PPT has shown great potential. However, its non-specific heat damage to the nearby tissues is one of the most important issues. Because PPT sterilization requires high temperatures (≥60 °C), this can damage surrounding normal cells and tissues. So, we offer several ways to improve this problem now. For example, PPT collaboration with PDT has been proved to be an effective method, which not only reduces the temperature induced by PPT, but also enhances the antibacterial effect.
In summary, PTAs still face many challenges, such as finding low-cost materials and synthesizing or discovering new PTAs. Its development direction is to use a variety of biological resources to achieve a greener synthesis of traditional nanomaterials (or the use of FDA-approved materials to develop new nanomaterials) to produce more pure, non-toxic, safe and degradable biological materials.
Author contributions
Conceptualization: M. H. and H. L. Supervision: H. L. writing – original draft: M. H. and H. L. writing – review and editing: M. H., W. S., Y. C. and H. L.
Conflicts of interest
The authors have no conflicts to declare.
Acknowledgements
This work was supported by the Fundamental Research Funds for Undergraduate Universities of Heilongjiang Province (Grant No. 145309507) and College Students' Innovative Entrepreneurial Training Project of Qiqihar University (x202310232063).
Notes and references
- Z. Y. Zhang, Z. P. Qi, W. J. Kong, R. F. Zhang and C. L. Yao, Front. Bioeng. Biotechnol., 2023, 11, 18 Search PubMed
.
- Y. Q. Liang, Y. P. Liang, H. L. Zhang and B. L. Guo, Asian J. Pharm. Sci., 2022, 17, 353–384 CrossRef
.
- Q. Pang, Z. L. Jiang, K. H. Wu, R. X. Hou and Y. B. Zhu, Antibiotics, 2023, 12, 31 Search PubMed
.
- T. Lehmann, A. E. Vaughn, S. Seal, K. W. Liechty and C. Zgheib, Pharmaceutics, 2022, 14, 19 CrossRef
.
- V. Brumberg, T. Astrelina, T. Malivanova and A. Samoilov, Biomedicines, 2021, 9, 15 CrossRef PubMed
.
- S. Cheng, M. Pan, D. R. Hu, R. X. Han, L. Li, Z. W. Bei, Y. C. Li, A. Sun and Z. Y. Qian, Chin. Chem. Lett., 2023, 34, 9 Search PubMed
.
- C. R. Ustundag and M. B. Piskin, Mater. Technol., 2023, 38, 14 Search PubMed
.
- M. Pita-Vilar, A. Concheiro, C. Alvarez-Lorenzo and L. Diaz-Gomez, Carbohydr. Polym., 2023, 321, 15 CrossRef
.
- A. Shaabani, D. Bizari and H. Khoshmohabat, Carbohydr. Polym., 2023, 321, 16 CrossRef PubMed
.
- X. Y. Hu, Q. Kong, R. Li, C. E. Zhou and Z. G. Li, Fibers Polym., 2023, 24, 3373–3384 CrossRef CAS
.
- M. X. Li, W. Z. Xia, Y. M. Khoong, L. J. Huang, X. Huang, H. Liang, Y. Zhao, J. Y. Mao, H. J. Yu and T. Zan, Biomater. Res., 2023, 27, 32 CrossRef
.
- N. Santamaria, K. Woo, D. Beeckman, P. Alves, B. Cullen, A. Gefen, J. L. Lázaro-Martínez, H. Lev-Tov, B. Najafi, A. Sharpe and T. Swanson, Int. Wound J., 2023, 20(9), 3467–3473 CrossRef
.
- C. Y. Shi, C. Y. Wang, H. Liu, Q. J. Li, R. H. Li, Y. Zhang, Y. Z. Liu, Y. Shao and J. C. Wang, Front. Bioeng. Biotechnol., 2020, 8, 17 CrossRef
.
- E. R. Ghomi, M. Niazi and S. Ramakrishna, Polym. Adv. Technol., 2022, 34(2), 520–530 CrossRef
.
- H. Q. Tran, S. M. S. Shahriar, Z. Yan and J. W. Xie, Adv. Wound Care, 2023, 12, 399–427 CrossRef
.
- K. Nuutila and E. Eriksson, Adv. Wound Care, 2021, 10, 685–698 CrossRef
.
- S. Chaiarwut, P. Ekabutr, P. Chuysinuan, T. Chanamuangkon and P. Supaphol, J. Polym. Res., 2021, 28, 19 CrossRef
.
- H. Cortes, I. H. Caballero-Florán, N. Mendoza-Muñoz, E. N. Córdova-Villanueva, L. Escutia-Guadarrama, G. Figueroa-González, O. D. Reyes-Hernández, M. González-Del Carmen, M. Varela-Cardoso, J. J. Magaña, B. Florán, M. L. Del Prado-Audelo and G. Leyva-Gómez, Cell. Mol. Biol., 2020, 66, 191–198 CrossRef
.
- S. O'Callaghan, P. Galvin, C. O'Mahony, Z. Moore and R. Derwin, J. Wound Care, 2020, 29, 394–406 CrossRef
.
- M. Ding, D. Zhao, R. Wei, Z. Duan, Y. Zhao, Z. Li, T. Lin and C. Li, Exploration, 2023, 20230057 Search PubMed
.
- T. I. Pavel, C. Chircov, M. Radulescu and A. M. Grumezescu, Cancers, 2020, 12, 22 CrossRef PubMed
.
- J. Z. Ye and X. H. Chen, Antibiotics, 2023, 12, 18 Search PubMed
.
- T. A. Wencewicz, J. Mol. Biol., 2019, 431, 3370–3399 CrossRef CAS PubMed
.
- T. Verma, A. Aggarwal, S. Singh, S. Sharma and S. J. Sarma, J. Mol. Struct., 2022, 1248, 13 CrossRef
.
- A. Buonavoglia, P. Leone, A. G. Solimando, R. Fasano, E. Malerba, M. Prete, M. Corrente, C. Prati, A. Vacca and V. Racanelli, Antibiotics, 2021, 10, 20 CrossRef
.
- Y. Woappi, P. Gabani, A. Singh and O. V. Singh, Crit. Rev. Microbiol., 2016, 42, 17–30 CrossRef CAS
.
- H. Liu, Y. Yu, A. Dong, M. Elsabahy, Y.-W. Yang and H. Gao, Exploration, 2024, 4, 20230092 CrossRef
.
- Y. W. Hu, Q. Zeng, Y. F. Hu, H. Y. Wang, C. Y. Deng and D. Li, Talanta, 2024, 266, 9 Search PubMed
.
- J. Zhang, H. Guo, M. Liu, K. Tang, S. Li, Q. Fang, H. Du, X. Zhou, X. Lin, Y. Yang, B. Huang and D. Yang, Exploration, 2023, 4(2), 20230087 CrossRef
.
- X. L. Qi, Y. J. Xiang, E. Cai, X. X. Ge, X. J. Chen, W. Zhang, Z. P. Li and J. L. Shen, Coord. Chem. Rev., 2023, 496, 27 CrossRef
.
- S. Y. Hao, H. C. Han, Z. Y. Yang, M. T. Chen, Y. Y. Jiang, G. X. Lu, L. Dong, H. L. Wen, H. Li, J. R. Liu, L. L. Wu, Z. Wang and F. L. Wang, Nano-Micro Lett., 2022, 14, 36 CrossRef
.
- J. Zhao, L. Duan, A. H. Wang, J. B. Fei and J. B. Li, Wiley Interdiscip. Rev.: Nanomed. Nanobiotechnol., 2020, 12, 20 Search PubMed
.
- D. H. Liao, J. F. Huang, C. Y. Jiang, L. Y. Zhou, M. B. Zheng, A. Nezamzadeh-Ejhieh, N. Qi, C. Y. Lu and J. Q. Liu, Pharmaceutics, 2023, 15, 32 Search PubMed
.
- Y. H. Duo, G. H. Luo, Z. H. Li, Z. D. Chen, X. M. Li, Z. Y. Jiang, B. L. Yu, H. Huang, Z. B. Sun and X. F. Yu, Small, 2021, 17, 19 Search PubMed
.
- Y. Y. Xu, Y. J. Cai, Y. Xia, Q. X. Wu, M. E. Li, N. Guo, Y. F. Tu, B. Yang and Y. Liu, Eur. Polym. J., 2023, 186, 12 Search PubMed
.
- B. Wang, Y. Xu, D. H. Shao, L. J. Li, Y. Q. Ma, Y. H. Li, J. W. Zhu, X. C. Shi and W. L. Li, Front. Bioeng. Biotechnol., 2022, 10, 15 Search PubMed
.
- W. D. Zhao, L. Jiang, H. Q. Yang, Z. Z. Yu, Z. H. Yang and Y. Zhou, Appl. Phys. A: Mater. Sci. Process., 2023, 129, 9 CrossRef
.
- Y. Liu, Q. Lan, J. X. Liu, Y. X. Shi, Q. X. Wu, Q. Wang, S. Yang and F. Cheng, J. Drug Delivery Sci. Technol., 2023, 88, 9 Search PubMed
.
- J. X. Hong, M. H. Chen, J. R. Xian, C. G. Li, X. F. Zheng, Q. Y. Deng, X. Q. Yin and M. T. Li, Mater. Lett., 2022, 317, 4 CrossRef
.
- Z. P. Li, S. Y. You, R. T. Mao, Y. J. Xiang, E. R. Y. Cai, H. Deng, J. L. Shen and X. L. Qi, Mater. Today Bio, 2022, 15, 15 Search PubMed
.
- C. C. Wang, Y. F. Liu, Y. H. Zhang, Y. X. Song, Q. Chen, A. Q. Cai, H. W. Guo and P. Zhang, Ceram. Int., 2023, 49, 11378–11392 CrossRef CAS
.
- X. Chen, G. Wu, J. Y. Tang, L. Zhou and S. H. Wei, Inorg. Chem. Commun., 2020, 114, 7 CrossRef
.
- L. Park, H. S. Kim, W. Jang, M. K. Ji, J. H. Ryu, H. Cho and H. P. Lim, Int. J. Mol. Sci., 2023, 24, 13 Search PubMed
.
- J. Han, Y. P. Feng, Z. X. Liu, Q. Y. Chen, Y. P. Shen, F. Feng, L. Z. Liu, M. Q. Zhong, Y. Zhai, M. Bockstaller and Z. P. Zhao, Polymer, 2021, 230, 11 Search PubMed
.
- Y. Q. Xu, K. Wang, S. L. Zhao, Q. S. Xiong, G. H. Liu, Y. Li, Q. L. Fang, X. L. Gong and S. H. Xuan, Chem. Eng. J., 2022, 437, 14 Search PubMed
.
- H. Liu, F. Xing, Y. X. Zhou, P. Y. Yu, J. W. Xu, R. Luo, Z. Xiang, P. M. Rommens, M. Liu and U. Ritz, Mater. Des., 2023, 233, 112231 CrossRef CAS
.
- H. Kamyab, S. Chelliapan, O. Tavakkoli, M. Mesbah, J. K. Bhutto, T. Khademi, I. Kirpichnikova, A. Ahmad and A. A. Aljohani, Chemosphere, 2022, 308, 11 CrossRef
.
- H. H. Ye, Z. Xi, K. Magloire and X. H. Xia, ChemNanoMat, 2019, 5, 860–868 CrossRef CAS
.
- H. Y. Xia, C. Hu, T. K. Chen, D. Hu, M. R. Zhang and K. Xie, Polymers, 2019, 11, 15 Search PubMed
.
- J. Ma, N. Li, J. Wang, Z. Liu, Y. Han and Y. Zeng, Exploration, 2023, 20220161 CrossRef
.
- B. X. Jiang, H. Wang, Y. T. Zhang and S. B. Li, Polyhedron, 2023, 243, 15 CrossRef
.
- L. P. Qiao, Y. P. Liang, J. Y. Chen, Y. Huang, S. A. Alsareii, A. M. Alamri, F. A. Harraz and B. L. Guo, Bioact. Mater., 2023, 30, 129–141 CAS
.
- S. J. Cao, K. Zhang, Q. J. Li, S. K. Zhang and J. D. Chen, Carbohydr. Polym., 2023, 320, 12 CrossRef
.
- J. H. He, Z. L. Li, J. X. Wang, T. Y. Li, J. Y. Chen, X. L. Duan and B. L. Guo, Composites, Part B, 2023, 266, 16 Search PubMed
.
- Y. Wu, D. X. Jia, K. Y. Lu, H. X. Zhang, C. X. Liu, Y. C. Lin, J. J. Cheng, Y. Zou, H. Xu, H. Chen, Y. X. Zhang and Q. Yu, J. Mater. Sci. Technol., 2023, 160, 76–85 CrossRef CAS
.
- Z. Y. Zhang, Z. P. Qi, W. J. Kong, R. F. Zhang and C. L. Yao, Front. Bioeng. Biotechnol., 2023, 11, 1154301 CrossRef
.
- K. Ousey, M. G. Rippon, A. A. Rogers and J. P. Totty, J. Wound Care, 2023, 32, 334–347 CrossRef PubMed
.
- P. P. Patil, M. R. Reagan and R. A. Bohara, Int. J. Biol. Macromol., 2020, 164, 4613–4627 CrossRef CAS PubMed
.
- S. P. Ndlovu, K. Ngece, S. Alven and B. A. Aderibigbe, Polymers, 2021, 13(17), 2959 CrossRef CAS PubMed
.
- Z. B. Liang, P. Lai, J. Zhang, Q. Lai and L. He, Int. Wound J., 2023, 20(10), 4410–4421 CrossRef PubMed
.
- N. Santamaria, K. Woo, D. Beeckman, P. Alves, B. Cullen, A. Gefen, J. L. Lázaro-Martínez, H. Lev-Tov, B. Najafi, A. Sharpe and T. Swanson, Int. Wound J., 2023, 20(9), 3467–3473 CrossRef
.
- E. R. Ghomi, M. Niazi and S. Ramakrishna, Polym. Adv. Technol., 2022, 34(2), 520–530 CrossRef
.
- B. A. Aderibigbe and B. Buyana, Pharmaceutics, 2018, 10(2), 42 CrossRef
.
- Q. Pang, F. Yang, Z. L. Jiang, K. H. Wu, R. X. Hou and Y. B. Zhu, Mater. Des., 2023, 229, 111917 CrossRef CAS
.
- M. L. Shao, Z. Shi, X. F. Zhang, B. Zhai and J. S. Sun, Materials, 2023, 16(4), 1338 CrossRef
.
- A. Amirsadeghi, A. Jafari, S. S. Hashemi, A. Kazemi, Y. Ghasemi, A. Derakhshanfar, M. A. Shahbazi and S. V. Niknezhad, Mater. Today Commun., 2021, 27, 102225 CrossRef CAS
.
- M. Gruppuso, G. Turco, E. Marsich and D. Porrelli, Appl. Mater. Today, 2021, 24, 110048 Search PubMed
.
- S. Alven and B. A. Aderibigbe, Polymers, 2021, 13(13), 2104 CrossRef CAS PubMed
.
- V. Brumberg, T. Astrelina, T. Malivanova and A. Samoilov, Biomedicines, 2021, 9(9), 1235 CrossRef CAS
.
- C. D. Weller, V. Team and G. Sussman, Front. Pharmacol., 2020, 11, 155 CrossRef CAS
.
- B. G. Andryukov, N. N. Besednova, T. A. Kuznetsova, T. S. Zaporozhets, S. P. Ermakova, T. N. Zvyagintseva, E. A. Chingizova, A. K. Gazha and T. P. Smolina, Biomedicines, 2020, 8(9), 301 CrossRef CAS PubMed
.
- G. M. Guebitz and G. S. Nyanhongo, Trends Biotechnol., 2018, 36, 1040–1053 CrossRef CAS PubMed
.
- M. Georgescu, M. C. Chifiriuc, L. Marutescu, I. Gheorghe, V. Lazar, A. Bolocan and S. Bertesteanu, Curr. Org. Chem., 2017, 21, 53–63 CrossRef CAS
.
- F. Pallaske, A. Pallaske, K. Herklotz and J. Boese-Landgraf, J. Wound Care, 2018, 27, 692–702 CrossRef PubMed
.
- S. Tandukar and S. Hariharan, Organogenesis, 2018, 14, 159–162 CrossRef CAS PubMed
.
- N. Ahmad, Pharmaceutics, 2023, 15(1), 42 CrossRef CAS PubMed
.
- L. Khullar, K. Harjai and S. Chhibber, Future Microbiol., 2023, 18, 43–63 CrossRef CAS PubMed
.
- V. Vivcharenko and A. Przekora, Appl. Sci., 2021, 11, 4114 CrossRef CAS
.
- B. Wang, Y. Xu, D. H. Shao, L. J. Li, Y. Q. Ma, Y. H. Li, J. W. Zhu, X. C. Shi and W. L. Li, Front. Bioeng. Biotechnol., 2022, 10, 1047598 CrossRef PubMed
.
- L. N. He, D. H. Di, X. H. Chu, X. L. Liu, Z. Y. Wang, J. Y. Lu, S. L. Wang and Q. F. Zhao, J. Controlled Release, 2023, 363, 180–200 CrossRef CAS PubMed
.
- F. Ding, L. L. Zhang, X. Chen, W. L. Yin, L. Ni and M. Wang, Front. Bioeng. Biotechnol., 2022, 10, 1066617 CrossRef PubMed
.
- V. Dediu, J. Ghitman, G. G. Pircalabioru, K. H. Chan, F. S. Iliescu and C. Iliescu, Int. J. Mol. Sci., 2023, 24(11), 9375 CrossRef CAS PubMed
.
- M. Yang, J. Zhang, Y. H. Wei, J. Zhang and C. M. Tao, Nano Res., 2022, 15, 6220–6242 CrossRef CAS PubMed
.
- S. Y. Chen, J. Lu, T. H. You and D. P. Sun, Coord. Chem. Rev., 2021, 439, 213929 CrossRef CAS
.
- B. X. Jiang, H. Wang, Y. T. Zhang and S. B. Li, Polyhedron, 2023, 243, 116569 CrossRef CAS
.
- G. B. Wang, K. H. Xie, H. P. Xu, Y. J. Wang, F. Zhao, Y. Geng and Y. B. Dong, Coord. Chem. Rev., 2022, 472, 213774 Search PubMed
.
- Y. Chen, Y. J. Gao, Y. Chen, L. Liu, A. C. Mo and Q. Peng, J. Controlled Release, 2020, 328, 251–262 CrossRef CAS PubMed
.
- H. M. Hao and X. J. Lang, ChemCatChem, 2019, 11, 1378–1393 CrossRef CAS
.
- B. Chen, K. Guo, X. Zhao, Z. Liu, C. Xu, N. Zhao and F. J. Xu, Exploration, 2023, 20220140 CrossRef PubMed
.
- A. Naskar and K. S. Kim, Pharmaceutics, 2022, 14(11), 2343 CrossRef CAS PubMed
.
- Y. N. Tang, Z. Qin, S. Y. Yin and H. Sun, Nanoscale, 2021, 13, 6373–6388 RSC
.
- L. Argueta-Figueroa, O. Martnez-Alvarez, J. Santos-Cruz, R. Garcia-Contreras, L. S. Acosta-Torres, J. de la Fuente-Hernndez and M. C. Arenas-Arrocena, Mater. Sci. Eng., C, 2017, 76, 1305–1315 CrossRef CAS PubMed
.
- A. Maleki, J. H. He, S. Bochani, V. Nosrati, M. A. Shahbazi and B. L. Guo, ACS Nano, 2021, 15, 18895–18930 CrossRef CAS PubMed
.
- H. C. Han, J. J. Yang, X. Y. Li, Y. Qi, Z. Y. Yang, Z. J. Han, Y. Y. Jiang, M. Stenzel, H. Li, Y. X. Yin, Y. Du, J. R. Liu and F. L. Wang, Nano Res., 2021, 14, 2512–2534 CrossRef CAS PubMed
.
- J. H. Shen, J. L. Liu, X. Y. Fan, H. Liu, Y. Bao, A. P. Hui and H. A. Munir, Biomater. Sci., 2023, 12, 596–620 RSC
.
- H. P. Lee and A. K. Gaharwar, Adv. Sci., 2020, 7(17), 2000863 CrossRef CAS PubMed
.
- S. H. Fan, W. S. Lin, Y. F. Huang, J. J. Xia, J. F. Xu, J. N. Zhang and J. Pi, Front. Pharmacol., 2022, 13, 829712 CrossRef CAS PubMed
.
- S. Yougbaré, C. Mutalik, D. I. Krisnawati, H. Kristanto, A. Jazidie, M. Nuh, T. M. Cheng and T. R. Kuo, Nanomaterials, 2020, 10(6), 1123 CrossRef PubMed
.
- Z. Ahmadian, H. Gheybi and M. Adeli, J. Drug Delivery Sci. Technol., 2022, 73, 103458 CrossRef CAS
.
- Q. X. Song, Y. H. Liu, P. Zhang, W. L. Feng, S. Z. Shi, N. L. Zhou, X. H. Chu and J. Shen, ACS Appl. Nano Mater., 2022, 5, 8621–8630 CrossRef CAS
.
- Y. P. Liu, X. W. Zhang, L. Y. Luo, L. Li, Y. C. He, J. An and D. W. Gao, ACS Biomater. Sci. Eng., 2018, 4, 2911–2921 CrossRef CAS PubMed
.
- R. K. Thapa, J. H. Kim, J. N. Jeong, B. S. Shin, H. G. Choi, C. S. Yong and J. O. Kim, Colloids Surf., B, 2017, 153, 95–103 CrossRef CAS PubMed
.
- T. F. Tian, X. Z. Shi, L. Cheng, Y. C. Luo, Z. L. Dong, H. Gong, L. G. Xu, Z. T. Zhong, R. Peng and Z. Liu, ACS Appl. Mater. Interfaces, 2014, 6, 8542–8548 CrossRef CAS
.
- J. H. He, M. T. Shi, Y. P. Liang and B. L. Guo, Chem. Eng. J., 2020, 394, 124888 CrossRef CAS
.
- X. J. He, S. Koo, E. Obeng, A. Sharma, J. L. Shen and J. S. Kim, Coord. Chem. Rev., 2023, 492, e2400366 CrossRef
.
- K. L. Huang, C. Y. Lv, C. H. Li, H. C. Bai and X. C. Meng, J. Colloid Interface Sci., 2023, 636, 21–32 CrossRef CAS PubMed
.
- S. R. Ye, H. C. Zhang, H. Y. Lai, J. Y. Xu, L. Yu, Z. T. Ye and L. Y. Yang, Front. Bioeng. Biotechnol., 2024, 12, 1338539 CrossRef PubMed
.
- G. H. Liu, Q. S. Xiong, Y. Q. Xu, Q. L. Fang, M. Sang, S. H. Xuan, L. Y. Hao and K. C. F. Leung, Appl. Surf. Sci., 2022, 590, 153125 CrossRef CAS
.
- C. Fang, P. J. Yan, Z. H. Ren, Y. F. Wang, X. J. Cai, X. Li and G. R. Han, Appl. Mater. Today, 2019, 15, 472–481 CrossRef
.
- Y. W. Zheng, Y. L. Yan, L. M. Lin, Q. He, H. H. Hu, R. Luo, D. Y. Xian, J. Y. Wu, Y. Shi, F. P. Zeng, C. B. Wu, G. L. Quan and C. Lu, Acta Biomater., 2022, 142, 113–123 CrossRef CAS PubMed
.
- P. Iravani, S. Iravani and R. S. Varma, Micromachines, 2022, 13(9), 1383 CrossRef PubMed
.
- M. X. Liu, L. Zheng, K. K. Zha, Y. Y. Yang, Y. P. Hu, K. Chen, F. Wang, K. Y. Zhang, W. Liu, B. B. Mi, X. F. Xiao and Q. Feng, Front. Bioeng. Biotechnol., 2023, 11, 1308184 CrossRef PubMed
.
- Z. Z. Yu, L. Jiang, R. Y. Liu, W. D. Zhao, Z. H. Yang, J. Y. Zhang and S. Z. Jin, Chem. Eng. J., 2021, 426, 131914 CrossRef CAS
.
- Y. H. Dong, J. H. Liu, Y. Chen, T. Zhu, Y. H. Li, C. L. Zhang, X. Zeng, Q. M. Chen and Q. Peng, Int. J. Biol. Macromol., 2023, 240, 124482 CrossRef CAS PubMed
.
- Q. Y. Li, W. Wang, H. M. Feng, L. Cao, H. F. Wang, D. Wang and S. G. Chen, J. Colloid Interface Sci., 2021, 604, 810–822 CrossRef CAS PubMed
.
- S. Q. Zhang, J. W. Ye, X. Liu, Y. Wang, C. Li, J. T. Fang, B. N. Chang, Y. Qi, Y. C. Li and G. L. Ning, J. Colloid Interface Sci., 2021, 599, 390–403 CrossRef CAS PubMed
.
- H. M. Feng, W. Wang, T. Wang, Y. A. Pu, C. C. Ma and S. G. Chen, Acta Biomater., 2023, 171, 506–518 CrossRef CAS PubMed
.
- Q. Wu, L. Tan, X. M. Liu, Z. Y. Li, Y. Zhang, Y. F. Zheng, Y. Q. Liang, Z. D. Cui, S. L. Zhu and S. L. Wu, Appl. Catal., B, 2021, 297, 120500 CrossRef CAS
.
- F. M. Lin, Q. Y. Duan and F. G. Wu, ACS Appl. Polym. Mater., 2020, 2, 4331–4344 CrossRef CAS
.
- S. X. Zhu, X. Y. Wang, S. L. Li, L. Liu and L. D. Li, ACS Appl. Mater. Interfaces, 2020, 12, 11063–11071 CrossRef CAS PubMed
.
- E. Y. Bayar, B. Getiren, F. Soysal, Z. Çiplak, N. Yildiz and E. Bayraktar, Mater. Res. Bull., 2023, 166, 112352 CrossRef
.
- Z. R. Li, B. B. Yan, L. L. Wu, B. Xu, L. Cui, Y. Y. Yu and P. Wang, Colloids Surf., A, 2023, 666, 131285 CrossRef CAS
.
- Y. Wang, X. D. He, Y. F. Cheng, L. Li, K. Zhang, E. T. Kang and L. Q. Xu, Colloids Surf., B, 2022, 212, 112381 CrossRef CAS PubMed
.
- H. T. Luo, W. G. Ji, W. X. Guo, P. L. Chen, Z. F. Zhang, X. Xu, B. D. Yue, W. Tan and B. L. Zhou, Microporous Mesoporous Mater., 2022, 346, 112281 CrossRef CAS
.
- B. H. Sun, Z. Q. Ye, M. Zhang, Q. X. Song, X. H. Chu, S. R. Gao, Q. C. Zhang, C. Jiang, N. L. Zhou, C. Yao and J. Shen, ACS Appl. Mater. Interfaces, 2021, 13, 42396–42410 CrossRef CAS PubMed
.
- Y. Luo, X. M. Liu, L. Tan, Z. Y. Li, K. W. K. Yeung, Y. F. Zheng, Z. D. Cui, Y. Q. Liang, S. L. Zhu, C. Y. Li, X. B. Wang and S. L. Wu, Chem. Eng. J., 2021, 405, 126730 CrossRef CAS
.
- Y. Xiao, M. R. Xu, N. Lv, C. Cheng, P. Huang, J. B. Li, Y. Hu and M. Sun, Acta Biomater., 2021, 122, 291–305 CrossRef CAS PubMed
.
- J. H. Liu, D. Wu, N. Zhu, Y. N. Wu and G. L. Li, Trends Food Sci. Technol., 2021, 109, 413–434 CrossRef CAS
.
- B. Y. Wu, J. T. Fu, Y. X. Zhou, Y. Shi, J. Wang, X. Q. Feng, Y. T. Zhao, G. L. Zhou, C. Lu, G. L. Quan, X. Pan and C. B. Wu, Pharmaceutics, 2019, 11(9), 463 CrossRef CAS PubMed
.
- D. L. Han, Y. Li, X. M. Liu, B. Li, Y. Han, Y. F. Zheng, K. W. K. Yeung, C. Y. Li, Z. D. Cui, Y. Q. Liang, Z. Y. Li, S. L. Zhu, X. B. Wang and S. L. Wu, Chem. Eng. J., 2020, 396, 125194 CrossRef CAS
.
- E. Naseri and A. Ahmadi, Eur. Polym. J., 2022, 173, 111293 CrossRef CAS
.
- B. Azimi, H. Maleki, L. Zavagna, J. G. De la Ossa, S. Linari, A. Lazzeri and S. Danti, J. Funct. Biomater., 2020, 11(3), 67 CrossRef CAS PubMed
.
- P. I. Campa-Siqueiros, T. J. Madera-Santana, M. M. Castillo-Ortega, J. López-Cervantes, J. F. Ayala-Zavala and E. L. Ortiz-Vazquez, RSC Adv., 2021, 11, 15340–15350 RSC
.
- C. Huang, X. Z. Xu, J. H. Fu, D. G. Yu and Y. B. Liu, Polymers, 2022, 14(16), 3266 CrossRef CAS PubMed
.
- H. Maleki, M. Doostan, S. Shojaei, M. Doostan, H. Stamatis, E. Gkantzou, A. Bonkdar and K. Khoshnevisan, J. Drug Delivery Sci. Technol., 2023, 82, 104367 CrossRef CAS
.
- H. Tian, J. X. Hong, C. G. Li, Y. H. Qiu, M. T. Li, Z. Y. Qin, R. A. Ghiladi and X. Q. Yin, Biomater. Adv., 2022, 142, 12 CrossRef PubMed
.
- H. Fang, J. Xu, H. L. Ma, J. Q. Liu, E. R. Xing, Y. Y. Cheng, H. Wang, Y. Nie, B. Pan and K. D. Song, Int. J. Bioprint., 2023, 9, 166–191 Search PubMed
.
- R. S. de Oliveira, S. S. Fantaus, A. J. Guillot, A. Melero and R. C. R. Beck, Pharmaceutics, 2021, 13(11), 1946 CrossRef CAS PubMed
.
- Y. Shao, K. Y. Dong, X. Y. Lu, B. B. Gao and B. F. He, ACS Appl. Mater. Interfaces, 2022, 14, 56525–56534 CrossRef CAS PubMed
.
- L. T. Xu, Y. Chen, P. Zhang, J. J. Tang, Y. F. Xue, H. S. Luo, R. Dai, J. L. Jin and J. Liu, Biomater. Sci., 2022, 10, 5648–5661 RSC
.
- J. Koehler, F. P. Brandl and A. M. Goepferich, Eur. Polym. J., 2018, 100, 1–11 CrossRef CAS
.
- Z. H. Shen, C. R. Zhang, T. Wang and J. Xu, Polymers, 2023, 15(9), 2000 CrossRef CAS PubMed
.
- Y. Feng, Z. Zhang, W. Tang and Y. Dai, Exploration, 2023, 202200173 Search PubMed
.
- M. Zhang and X. Zhao, Int. J. Biol. Macromol., 2020, 162, 1414–1428 CrossRef CAS PubMed
.
- E. A. Kamoun, E. R. S. Kenawy and X. Chen, J. Adv. Res., 2017, 8, 217–233 CrossRef CAS PubMed
.
- J. Zhao, P. Qiu, Y. Wang, Y. F. Wang, J. N. Zhou, B. C. Zhang, L. H. Zhang and D. X. Gou, Int. J. Biol. Macromol., 2023, 244, 125250 CrossRef CAS PubMed
.
- W. T. Shu, Y. A. Wang, X. Zhang, C. Y. Li, H. X. Le and F. Chang, Front. Bioeng. Biotechnol., 2021, 9, 788461 CrossRef PubMed
.
- P. Zhao, Y. Zhang, X. A. Chen, C. Xu, J. Z. Guo, M. G. Deng, X. W. Qu, P. S. Huang, Z. J. Feng and J. M. Zhang, Adv. Sci., 2023, 10(11), 2206585 CrossRef CAS PubMed
.
- H. L. Zhang, M. Q. Xu, H. H. Luo, S. Q. Wu, X. F. Gao, Q. Wu, H. Xu and Y. Liu, Int. J. Biol. Macromol., 2023, 243, 124362 CrossRef CAS PubMed
.
- I. Savencu, S. Iurian, A. Porfire, C. Bogdan and I. Tomuta, React. Funct. Polym., 2021, 168, 105059 CrossRef CAS
.
- C. Li, F. Chang, F. C. Gao, Y. D. Wang, Z. Q. Sun, L. Zhao, Y. F. Yang, H. T. Wang, L. Dong, X. R. Zheng and Y. Y. Jiang, Appl. Surf. Sci., 2024, 642 Search PubMed
.
- Y. X. Chen, M. Q. Ye, L. W. Song, J. W. Zhang, Y. Yang, S. Luo, M. J. Lin, Q. W. Zhang, S. Y. Li, Y. J. Zhou, A. Q. Chen, Y. An, W. Huang, T. X. Xuan, Y. G. Gu, H. C. He, J. Wu and X. K. Li, Appl. Mater. Today, 2020, 20, 100756 CrossRef
.
- L. Zheng, B. Gu, S. S. Li, B. C. Luo, Y. M. Wen, M. W. Chen, X. Y. Li, Z. A. Zha, H. T. Zhang and X. Y. Wang, Carbohydr. Polym., 2022, 296, 119924 CrossRef CAS PubMed
.
- X. Zhao, L. Liu, T. Z. An, M. Xian, J. A. Luckanagul, Z. H. Su, Y. Lin and Q. Wang, Acta Biomater., 2020, 104, 85–94 CrossRef CAS PubMed
.
- M. Sadeghi-Aghbash, M. Rahimnejad, H. Adeli and F. Feizi, Curr. Pharm. Biotechnol., 2023, 24, 1079–1093 CAS
.
- S. L. Chen, A. Li, Y. P. Wang, Y. F. Zhang, X. Liu, Z. P. Ye, S. D. Gao, H. Xu, L. D. Deng, A. J. Dong and J. H. Zhang, Acta Biomater., 2023, 171, 428–439 CrossRef CAS PubMed
.
- N. Namuiriyachote, V. Lipipun, Y. Althhatuattananglzul, P. Charoonrut and G. C. Ritthidej, Asian J. Pharm. Sci., 2019, 14, 63–77 CrossRef PubMed
.
- O. M. Alvarez, M. S. Granick, A. Reyzelman and T. Serena, J. Comp. Eff. Res., 2021, 10, 481–493 CrossRef PubMed
.
- T. Yamane, G. Nakagami, S. Yoshino, M. Shimura, A. Kitamura, K. Kobayashi-Hattori, Y. Oishi, Y. Nishijima, T. Minematsu and H. Sanada, Biosci., Biotechnol., Biochem., 2015, 79, 185–189 CrossRef CAS PubMed
.
- A. Buzarovska, S. Dinescu, A. D. Lazar, M. Serban, G. G. Pircalabioru, M. Costache, C. Gualandi and L. Avérous, Mater. Sci. Eng., C, 2019, 104, 10893 CrossRef PubMed
.
- Z. Pahlevanneshan, M. Deypour, A. Kefayat, M. Rafienia, P. Sajkiewicz, R. E. Neisiany and M. S. Enayati, Polymers, 2021, 13(18), 3191 CrossRef CAS PubMed
.
- L. Q. Zhou, F. Chen, Z. S. Hou, Y. W. Chen and X. L. Luo, Chem. Eng. J., 2021, 409, 128224 CrossRef CAS
.
- M. Y. Sun, D. M. Li, Y. Xi, X. T. Qin, Y. T. Liao, X. Z. Liu, S. R. Jia, Y. Y. Xie and C. Zhong, Int. J. Biol. Macromol., 2024, 259(1), 129003 Search PubMed
.
|
This journal is © The Royal Society of Chemistry 2024 |
Click here to see how this site uses Cookies. View our privacy policy here.