DOI:
10.1039/D4QI01306A
(Review Article)
Inorg. Chem. Front., 2024,
11, 5810-5832
New advances in solid-state electrolytes: from halides to oxyhalides
Received
23rd May 2024
, Accepted 19th July 2024
First published on 30th July 2024
Abstract
The emerging area of halide solid electrolytes has garnered significant interest due to its superior ionic conductivity, broad electrochemical stability range, and strong compatibility with positive electrode materials. In recent times, there has been a continuous emergence of diverse halide systems. This study provides a comprehensive review of the synthesis strategies classification schemes, structural attributes, ion transport mechanisms, and modified means of halides. Notably, the paper delves into a detailed analysis of the structural details and ion conduction mechanisms of several representative halides. Furthermore, this review also examines the intrinsic connection between the recently discovered oxyhalides and halides and figures out the primary obstacles and prospective directions for advancement through a thorough review and analysis.
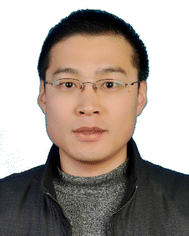 Qingtao Wang | Qingtao Wang received his BSc in 2005 from Shandong University and received his PhD in 2010 from Lanzhou Institute of Chemical Physics, Chinese Academy of Sciences. He joined Northwest Normal University in July 2010. His current research interests include: (1) solid electrolytes of sulfides, halides and oxyhalides; (2) electrocatalysts for energy conversion. |
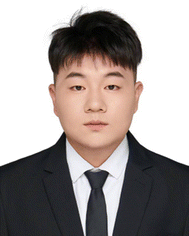 Zhenyang Shen | Zhenyang Shen, a master's student in the group of Associate Professor Qingtao Wang at Northwest Normal University, is currently researching solid electrolytes of halides and oxyhalides. |
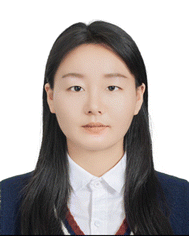 Yongmei Zhou | Yongmei Zhou, a master's student in the group of Associate Professor Qingtao Wang at Northwest Normal University, is currently researching solid electrolytes of halides and oxyhalides. |
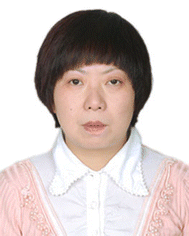 Ying Liu | Ying Liu received her BE in 1996 from Lanzhou university of technology telecommunication department. She joined Northwest Normal University in June 1996. Her current research interests include: (1) chemical automation instrumentation and detection technology, process control; (2) chemical sensors. |
1 Introduction
With the increasingly serious global energy shortage and environmental pollution issues, replacing traditional, non-renewable fossil fuels with clean, sustainable, and renewable energy has become an inevitable trend. Conventional lithium-ion batteries are susceptible to combustion and detonation owing to their limited thermal stability and low ignition threshold. In contrast, solid electrolytes have garnered attention in the realm of battery technology for their enhanced safety, stability, cost-effectiveness, and extended operational lifespan. This has prompted significant research efforts and ongoing advancements within the scientific community in this domain in recent times. As the core component of the new energy storage device, the solid electrolyte needs to meet the ionic conductivity of more than 10−3 S cm−1 at room temperature, while requiring high electrochemical stability and excellent compatibility with the electrode material.1–9 Among the sulfides, oxides, polymers, halides, and oxyhalides electrolytes that have been explored, oxides have high ionic conductivity of 10−3 to 10−4 S cm−1 and have good chemical stability against lithium metal, but the use of solid oxide electrolytes alone cannot provide satisfactory electrochemical performance due to high boundary resistance due to insufficient interfacial contact.10 The ionic conductivity of solid electrolytes based on sulfides is similar to that of liquid electrolytes. However, the practical utility of these solid electrolytes is constrained by their limited electrochemical stability window, high susceptibility to moisture, and tendency to react readily with water in the atmosphere, leading to the generation of hydrogen sulfide gas.11–15 Researchers prefer polymer electrolytes due to their wide electrochemical stability and high flexibility. They are also valued for their cost-effectiveness and straightforward preparation methods, which contribute to their widespread adoption and utilization in commercial settings. However, the ionic conductivity of such electrolytes is generally low at room temperature.16 Although the electrolyte system based on polyethylene oxide is recognized as a promising member of the polymer application, it usually needs to improve its performance by modifying means such as crosslinking and copolymerization, and its excellent electrochemical performance is often only shown at high-temperature conditions.17,18
Compared to the three types of electrolytes mentioned above, research on halide solid electrolytes (HSEs) is still relatively scarce. The focus is on achieving high ionic conductivity (>10−3 S cm−1) at room temperature, a wide electrochemical window, and good compatibility with cathode materials, which has become a popular research direction.19,20 The development timeline of halide solid electrolytes is shown in Fig. 1. In 1930, the ionic conductive behavior of LiX (X = Cl, F, Br, I) was reported, but the low ionic conductivity did not attract much attention.21 In 2018, Asano and colleagues synthesized Li3YCl6 and Li3YBr6 with exceptional ionic conductivity at room temperature using mechanical ball milling and annealing processes. The conductivity achieved was several orders of magnitude higher than that of previously reported HSEs. Subsequent research on HSEs has entered a more in-depth stage.21–23 Although HSEs has excellent electrochemical performance, its widespread application in practical use is hindered by economic barriers and practicality concerns stemming from the expensive metal elements it is composed of. In this context, research on cost control of HSEs is particularly crucial.24 The Li2ZrCl6 raw material reported by Ma et al.25 is several orders of magnitude cheaper than the most advanced HSEs, while also exhibiting a high ionic conductivity (0.81 mS cm−1) at room temperature and good moisture resistance. As of 2022, there is still a significant gap in the reported ion conductivity and sulfide of HSEs, which is worth noting that Tanaka and others26 have studied a new oxyhalide LiMOCl4 (M = Ta, Nb) with an ion conductivity as high as 12.4 mS cm−1. This new material can rival or even surpass the liquid electrolyte in lithium-ion batteries at room temperature. It is considered the most promising candidate for practical applications in all-solid-state batteries (ASSBs).
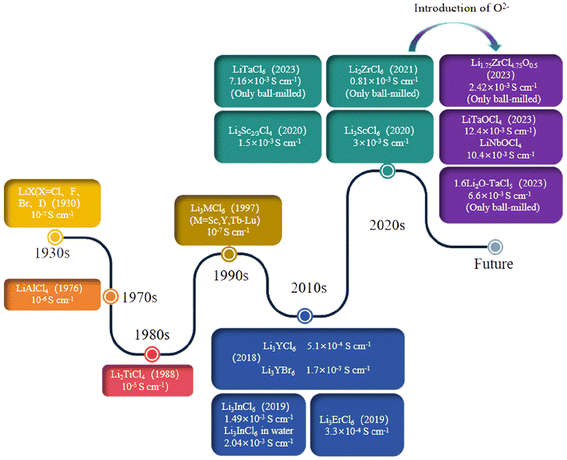 |
| Fig. 1 Development timeline of halide electrolytes and emerging oxyhalides for ASSBs. | |
This article provides an overview of the development of halide solid electrolytes and the innovative evolution from halides to oxyhalides. It then details three common synthesis methods and five major classification systems of halides, and delves into four types of crystal structures and their corresponding ion transport mechanisms. Finally, the article discusses modification strategies for halides and reveals the inherent connection between halides and oxyhalides.
2 Categories of halide solid electrolytes
Regarding the classification of halides, there are currently two main classifications: (1) dividing them into three categories based on different types of metal elements:19 (1) elements from IIIB (M = Sc, Y, La–Lu). (2) Elements from IIIA (M = Al, Ga, Ln). (3) Divalent metal elements (M = Ti, V, Cr, Mn, Fe, Co, Ni, Cu, Zn, Cd, Mg, Pb) in the LiaMXb. (2) According to the amount of transition metal elements substituted for Li ions,3 they are divided into (1) Lia-M-Cl6, (2) Lia-M-Cl4, and (3) Lia-M-Cl8 halides (M = transition metal element). Tuo et al.27 extended the classification of the IV based on the research of Zr and Hf elements. With the research on LiTaCl6 and LiMCl5Xn−1/n (M = Ta, Nb, Xn− = Cl−, F−, O2−) electrolytes28,29 a halide of VB metal element has gained public attention. When classifying based on the degree of Li-ion substitution by transition metal elements, different main groups or subgroup elements can be grouped into the same category. Here, we will continue to further classify based on the diverse types of metal elements.
2.1 Crystal structures of the IIIB metal elements (Sc, Y, La–Lu)
The halides of the IIIB metal elements include Li3MX6 (X = Cl, Br, I) and LiMF4. Meyer et al.19,30,31 confirmed through single-crystal X-ray diffraction data of the ternary chlorides Li3ErCl6, Li3YbCl6, and Li3ScCl6 that they crystallize in three different structural types. They classified (M = Y, Tb–Tm) as a trigonal crystal system with the P
m1 space group, (M = Y, Yb, Lu) as an orthorhombic crystal system with the pnma space group, and Sc as having a cubic close-packed anion sublattice with a crystal structure being monoclinic (space group C2/m). Part of the crystal structure of Li3MCl6 is shown in Fig. 2a.32 YCl3 is a representative of MX3 salts. Its physical properties make it equivalent to the end (rare) member of the lanthanide series,33 which may also be one of the reasons why Y and some lanthanide metal elements have the same space group structure. With the high stability of the YX63− (X = Cl or Br) octahedra and the expectation that each Y can provide two cation vacancies, Asano et al.22,33 synthesized Li3YCl6 (LYC) and Li3YBr6 (LYB) with ionic conductivities reaching 10−3 S cm−1 at room temperature after cold pressing. LYC is composed of hexagonally close-packed (hcp) anions, with a crystal structure of trigonal symmetry (space group P
m1) (Fig. 2b), whereas LYB is composed of cubic close-packed (ccp) anions, with all cations located in octahedral positions, exhibiting a monoclinic structure (space group C2/m) (Fig. 2c). Sun et al.34 synthesized LixScCl3+x using various ratios of LiCl and ScCl3. When x = 3, the room temperature ionic conductivity reached 3.02 mS cm−1. Similar to Y, ScCl63− also has an octahedral structure, but it is based on a monoclinic structure with ccp anion arrangement (Fig. 2d). Subsequently, a disordered spinel structure Li2Sc2/3Cl4 with an ionic conductivity of 1.5 mS cm−1 at room temperature was reported.35 Li is randomly distributed on tetrahedral and octahedral sites available in the lattice. Similar to the previously reported spinel Li2MgCl4,36,37 Li2MgCl4 is composed of edge-sharing (Mg1/Li2)Cl6 octahedra, while Li2Sc2/3Cl4 contains edge-sharing (Sc1/Li4)Cl6 octahedra.
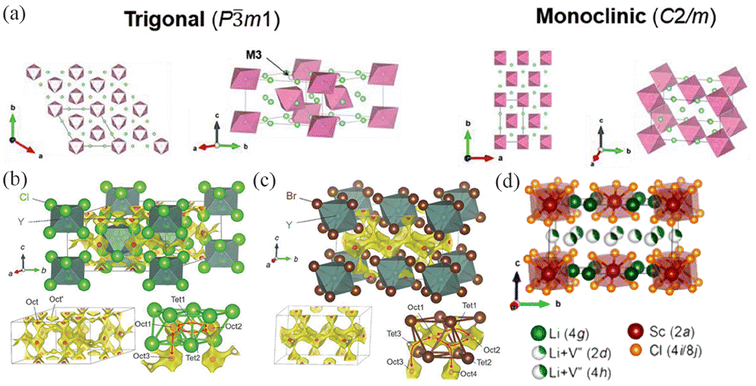 |
| Fig. 2 (a) Crystal structures outlined with the unit cells for trigonal and monoclinic Li3MCl6 (or Li2MCl6).32 (b) The triangular structure of Li3YCl6 and (c) the monoclinic structure of Li3YBr6.22 Reprinted with permission from ref. 22 and 32. Copyright (2018 and 2021) Wiley. (d) Typical crystal structure of Li3ScCl6.34 Reprinted with permission from ref. 34. Copyright (2020) American Chemical Society. | |
Lanthanum (La)38,39 in the lanthanide series has garnered attention as a research hotspot due to its distinctive UCl3-type crystal structure (space group P63/m). This structure sets it apart from the typical hexagonal close-packed (hcp) and face-centered cubic close-packed (ccp) anion frameworks. The fundamental unit of this framework is the lanthanum chloride nine-coordinated “three vertex three prism” unit, distinguished by the abundance of channel sites capable of hosting monovalent cations and offering migration pathways for them.
Xu et al.40 studied the new spinel structure Li3MI6 (M = Sc, Y, La) with octahedral Li occupancy using density functional theory (DFT) calculations. They predicted that Li3MI6 is monoclinic (space group C2) with a layered structure stacked O4 (octahedron 4) along the c-direction (Fig. 3a). There are two main types of stable lithium occupation sites: octahedral sites found in transition metal layers and those within pure lithium layers. The LiI6 octahedra in transition metal layers have a slightly larger volume and longer Li–I bond length than those in pure lithium layers. This setup, with around one-third of the octahedral sites empty, promotes the diffusion of Li+ ions. Zeier et al.31 successfully synthesized Li3ErI6. The Pawley fitting provided information on the lattice and overall symmetry, and only the C2 and C2/c space groups obtained reflections that matched both the observed and predicted data. Although these two space groups are highly similar and have the same polyhedral connectivity, C2/c exhibits superior symmetry. By combining synchrotron X-ray diffraction and neutron diffraction techniques, it was determined that Li3ErI6 belongs to a monoclinic structure (space group C2/c) (Fig. 3b). Compared to Li3ErCl6, it was found that Li3ErI6 has a more flexible lattice and faster ion transport.
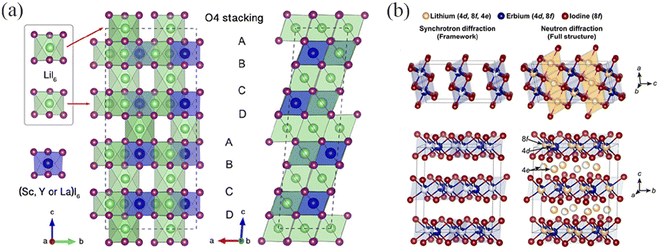 |
| Fig. 3 (a) O4 stacking sequence of Li3MI6 (M = Y, Sc, La) cells.40 (b) Structural frame and full structure of Li3ErI6.31 Reprinted with permission from ref. 31 and 40. Copyright (2019 and 2020) American Chemical Society. | |
All ternary halides of Li3MBr6 exhibit a monoclinic structure.19,40,41 Zeier et al.42 utilized temperature-dependent neutron diffraction to investigate Li3YBr6 with the C2/m space group. This structure is distinct from Li3YCl6 and can be described as having a layered configuration with the (002) crystal plane.
2.2 Crystal structures of the IVB metal elements (Zr, Ti, Hf)
Research on the IVB metal elements of HSEs is currently focused on Li2ZrCl6,25 which is popular due to its relatively high ionic conductivity at room temperature and considerable cost-effectiveness. The Li2ZrCl6 (α-LZC) after planetary ball milling has a P
m1 space group that is remarkably similar to Li3YCl6. After annealing at 350 °C, in addition to higher crystallinity, the crystal structure undergoes a change from P
m1 to a structure similar to Li3InCl6 (C2/m) called β-LZC (Fig. 4a). However, the ionic conductivity decreases by two orders of magnitude. Recently reported material Li3TiCl6 (LTC)43 with combined electrolyte, positive electrode, and negative electrode functions, after ball milling treatment, exhibits weaker diffraction peaks compared to LTC after annealing at 300 °C. From the X-ray diffraction (XRD) patterns, there is no fundamental difference between the two samples, indicating their consistency in overall crystal structure. Refinement of the XRD of LTC after ball milling and annealing reveals a close resemblance to the crystal structure of Li3InCl6 in the C2/m space group (Fig. 4b). Li44 and colleagues obtained another ideal layered structure Li2MCl6 (M = Zr, Hf) using the Stochastic Surface Walking global optimization combined with global Neural Network potential (SSW-NN) method. The structure consists of two layers formed by the sharing of a common edge between LiCl65− and Zr/HfCl65− octahedra, displaying a novel layered structure distinct from previous reports (Fig. 4c).
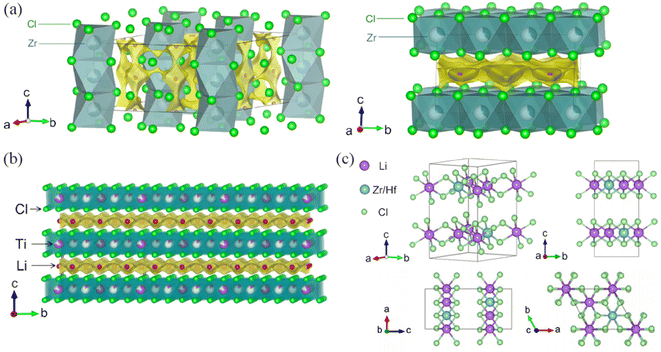 |
| Fig. 4 (a) The crystal structures of α-LZC (left) and β-LZC(right).25 (b) The structural model of LTC superimposed with the Li-ion potential map.43 Reprinted with permission from ref. 25 and 43. Copyright (2021 and 2023) Springer Nature. (c) The ideal layer structure of Li2MCl6 (M = Zr, Hf) crystal.44 Reprinted with permission from ref. 44. Copyright (2022) American Chemical Society. | |
2.3 Crystal structure of the IIIA metal elements (Al, Ga, In)
Research on aluminum halides can be traced back to the 1970s.45 Weppner et al.46 calculated that NaAlCl4 has an orthorhombic structure, while LiAlCl4 and KAlCl4 have monoclinic structures. Gregory et al.47 first synthesized the iodide LiAlI4 (monoclinic, space group P21/c), and room temperature diffraction data confirmed that LiAlI4 and LiAlBr4 are isostructural with chloride analogues. Li+ and Al3+ ions occupy octahedral and tetrahedral interstices, respectively. The extended structure is composed of distorted LiX6 octahedra and AlX4 tetrahedra, with the two octahedra connected at shared edges to form “Li2X10 dimers” (Fig. 5a–c). Regarding the IIIA elements, the current research focus is still on In element. In 1998, Koji Yamada et al.48 first reported Li3InBr6, which undergoes a phase transition at high temperatures with high ionic conductivity and maintains phase stability when returning to low temperatures. For the high-temperature phase Li3InBr6,49 it exhibits a monoclinic structure with space group C2/m, and in the structure, In3+ is in a disordered state at octahedral positions. Yamada et al.50 found that diffusion of In3+ and Li+ occurs throughout the crystal when the sample is kept at 420 K for two hours. One-third of the cation sites are occupied by vacancies due to substitution, indicating that In3+ is a good candidate for introducing vacancies into lithium-based solid electrolytes. Although the structure of the low-temperature phase is unknown, it is believed to be an ordered crystal based on the signals detected in this phase. In 1986, Wolfgang and his team51 determined the crystal structure of LiGaX4 (X = Cl, F, Br) using single crystal X-ray methods. The compound possesses LiX6 octahedra and GaX4 tetrahedra, similar to the structure of LiAlCl4. Interestingly, Jung and others52 designed an amorphous 2LiCl–GaF3 electrolyte, which exhibits a high ionic conductivity of 3.6 mS cm−1 at room temperature and high viscoelasticity. This unique “clay” property is believed to be due to the formation of gallium-containing, mobile, and complex anions Ga-F-X (X representing different halogens).
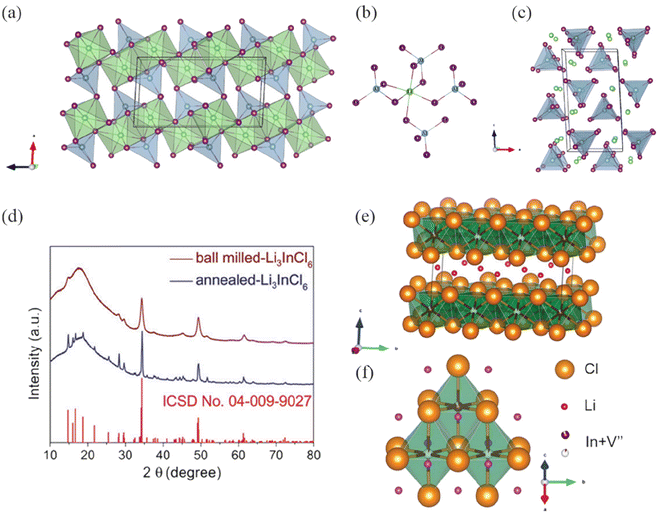 |
| Fig. 5 (a) Crystal structure of LiAlI4 (P21/c) projected along the b-axis. (b) Showing the linkage between an LiX6 octahedron and neighboring AlX4 tetrahedra. (c) Highlighting the positions of the Li+ cations with respect to the isolated haloaluminate anions: Li (4e, green spheres), Al (4e, light blue spheres), and I (4e, purple).47 Reprinted with permission from ref. 47. Copyright (2021) American Chemical Society. (d) X-ray diffraction patterns of the as-prepared ball milled and annealed Li3InCl6 samples. (e) Structure of annealed-Li3InCl6. (f) Schematic unit showing typical Li+ location around the InCl63− octahedra.55 Reprinted with permission from ref. 55. Copyright (2019) Royal Society of Chemistry. | |
The Li3InCl6 (LIC) reported by Lutz et al.53 was the best lithium-ion conductor at that time, with an ionic conductivity of 0.2 mS cm−1 at 300 °C. Schmidt et al.54 obtained colorless single crystals of LIC through the Bridgman-type method using a mixture of LiCl and InCl3 in a 3
:
1 molar ratio, highlighting the potential of indium-based halides. Sun et al.55 reported a high stability and high crystallinity halide electrolyte LIC, with a monoclinic crystal structure (space group C2/m). After annealing, it exhibits a high ionic conductivity of 1.49 mS cm−1 at room temperature. The XRD spectrum after annealing shows higher crystallinity compared to before annealing. The structure is essentially a distorted rock-salt LiCl structure, with octahedral interstices occupied by Li+, In3+, and vacancies in a ratio of 3
:
1
:
2. The anions are arranged in a ccp structure, which has lower symmetry compared to the body-centered cubic sublattice of the anions (Fig. 5d–f). Subsequently, the group prepared LIC with ionic conductivity up to 2.04 mS cm−1 at room temperature in an aqueous solution system.56 The crystal structure of this LIC remains the same as the samples synthesized by ball milling and sintering methods. It is worth noting that the ionic conductivity of this LIC can still be restored after removing the adsorbed water.
2.4 The amorphous state of the VB metal elements (Nb, Ta)
The main molecular formula of the VB metal elements is LiMCl6, and current reports on it are all amorphous. Ishiguro et al.57 found that TaCl5 has the ability to vitrify various lithium salts (LiF, LiCl, LiBr, LiI, Li2O, LiOH, Li2O2, Li2S), and can produce a glassy phase superionic conductor LiTaCl5Xn−1/n with an ion conductivity as high as 10.95 mS cm−1 at room temperature. By observing XRD, it was clearly found that there were no crystalline peaks in LiTaCl6, confirming that the substance is amorphous. In addition, a similar situation was also observed in the XRD of LiNbCl6. Li et al.29 used ab initio molecular dynamics (AIMD) to simulate annealing and create a glassy model of LiTaCl6 (Fig. 6). In the model, high-density Cl− aggregate with Li+ ions bridged by Cl−, with an average of 4.8 Cl− surrounding each Li+. The coordination number of Cl− ions around Ta is 5.7, with most Ta atoms existing in the form of TaCl6− and some in the form of TaCl5. The detailed parameters of the solid electrolytes of the aforementioned four types of halides can be found in Table 1.
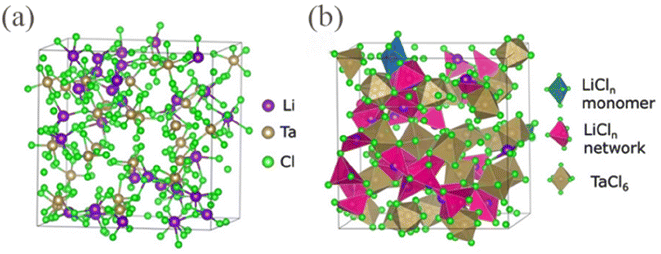 |
| Fig. 6 (a) Structure of glassy LiTaCl6: snapshot from AIMD. (b) Coordination polyhedra and LiCln network in the snapshot.29 Reprinted with permission from ref. 29. Copyright (2024) Wiley. | |
Table 1 Ionic conductivity and crystal structure of some metal halides
Group |
Material |
Structure |
Conductivity (mS cm−1) |
Ref. |
IIIB |
Li3ScCl6 |
Monoclinic, C2/m |
3 at 25 °C |
35
|
Li2Sc2/3Cl4 |
Cubic |
1.5 at 25 °C |
36
|
Li3YCl6 |
Trigonal |
0.51 at 25 °C |
22
|
Li3YCl6 |
Orthorhombic, Pnma |
3.07 at 450 °C (calculate) |
27
|
Li3YBr6 |
Monoclinic, C2/m |
0.72 at 25 °C |
22
|
Li3LaI6 |
Monoclinic, C2 |
1.23 at 25 °C (calculate) |
44
|
Li3ErCl6 |
Trigonal, P m1 |
3 at 25 °C (calculate) |
19
|
Li3ErI6 |
Monoclinic, C2/m |
0.65 at 25 °C |
32
|
IVB |
Li3TiCl6 |
Monoclinic, C2/m |
0.115 at 25 °C |
44
|
Li3TiCl6 |
Monoclinic, C2/m |
1.04 at 300 °C |
44
|
Li2ZrCl6 |
Trigonal, P m1 |
0.81 at 25 °C |
25
|
Li2ZrCl6 |
Monoclinic, C2/m |
5.81 × 10−3 at 350 °C |
25
|
Li2HfCl6 |
— |
∼0.5 at 25 °C (calculate) |
45
|
VB |
LiTaCl6 |
Amorphous |
10.95 at 25 °C |
30
|
LiTaCl6 |
Amorphous |
7.16 at 25 °C |
28
|
LiTaCl6 |
Amorphous |
11 at 25 °C (calculate) |
61
|
LiNbCl6 |
Amorphous |
12.192 at 25 °C (calculate) |
29
|
IIIA |
LiAlCl4 |
Monoclinic |
1.2 × 10−3 at 25 °C |
42
|
LiAlI4 |
Monoclinic, P21/c |
0.012 at 25 °C |
43
|
LiAlBr4 |
Monoclinic, P21/c |
0.033 at 25 °C |
43
|
Li3InCl6 |
— |
0.2 at 300 °C |
47
|
Li3InCl6 |
Monoclinic, C2/m |
1.49 at 25 °C |
48
|
Li3InCl6 |
Monoclinic, C2/m |
2.04 at 25 °C |
49
|
2.5 Crystal structure of divalent metal elements (Fe, Cd, Mg, Co, Zn, V)
In A2BCl4, various structures are generated based on the different ionic radius ratios. Electrolytes composed of divalent metal ions are mainly divided into four crystal systems: spinel system (cubic) with the formula LiaMX4 (M = Ti, V, Cr, Mn, Fe, Cd, Mg), olivine system (orthorhombic) of Li2ZnCl4, distorted Li2MCl4 (M = Cr, Fe, Co), and Suzuki structure (Li6MX8, M = V, Fe, Co). Please refer to Table 2 for more details. Spinel exists in two types: normal and inverse. In the normal spinel, the lithium ion conduction properties mainly depend on specific positions in the octahedral structure, while the inverse spinel utilizes both tetrahedra and octahedra in the lithium ion conduction process. This is also an important factor leading to the lower ionic conductivity of normal spinel compared to inverse spinel. Additionally, an attractive feature of spinel is that various transition metal cations can be stabilized in different distorted structures, offering possibilities for various conduction mechanisms.58
Table 2 Crystal structure and ionic conductivity of some divalent metal halides
Material |
Structure |
Conductivity (mS cm−1) |
Ref. |
Li2MgCl4 |
Inverse spinel |
0.14 at 400 °C |
60
|
Li2CdCl4 |
Inverse spinel |
0.3 at 400 °C |
60 and 65 |
Li1.9Cd1.05Cl4 |
Inverse spinel |
0.35 at 400 °C |
61
|
Li2MnCl4 |
Inverse spinel |
0.14 at 400 °C |
60
|
Li2FeCl4 |
Inverse spinel |
0.63 at 400 °C |
60
|
Li2ZnCl4 |
Normal spinel |
1 × 10−3 at 200 °C |
63
|
Li6FeCl8 |
Suzuki |
2 at 200 °C |
75
|
Li6CoCl8 |
Suzuki |
0.7 at 200 °C |
77
|
Li2ZnCl4 |
Olivine |
0.2 at 280 °C |
64
|
Li2CrCl4 |
Distorted spinel |
1.5 at 400 °C |
71
|
Li2CoCl4 |
Distorted spinel |
1 at 300 °C |
71 and 72 |
Research on Li2MCl4 can be traced back to 1975 when Van Loon et al.59 reported four ternary chlorides Li2MCl4 (M = Mg, Mn, Fe, Cd). Calculations showed that they have a spinel structure, with half of the lithium ions existing in tetrahedra surrounded by chlorine ions within the crystal, while the other half of the lithium ions and M ions are distributed in octahedra. This distribution is believed to resemble a NaCl structure with cationic defects. Lutz et al.60 found that when Li2MgCl4 is heated to 535 °C, Li2MnCl4 (460 °C), and Li2CdCl4 (385 °C), the spinel structure reversibly transforms into a defective cubic structure. In addition, these researchers61–64 measured the ion conductivity of Li2MCl4 between room temperature and 500 °C. At high temperatures, the ternary chlorides become highly disordered, and the ion conductivity continues to increase. For instance, the ion conductivity of Li2CdCl4 increased from 0.12 ± 0.03 at 300 °C to 1.3 ± 0.1 S cm−1 at 500 °C. Li1.9Cd1.05Cl4 exhibits an ion conductivity as high as 0.35 S cm−1 at 400 °C, but its decomposition potential is only 1.1–1.3 V, limiting its application in batteries.
Van Loon, Sassmannshausen and others65,66 found that Li2ZnCl4, after low-temperature modification, crystallizes differently from other known halides with a spinel structure. All Li ions are located at octahedral sites, while Zn ions are at tetrahedral sites (normal spinel structure). After high-temperature modification (215 °C), it transforms into an olivine structure. Lutz and others66–68 conducted a more detailed study on this structure. They found that a colorless single crystal Li2ZnCl4 with a normal spinel structure can be obtained by heating a stoichiometric mixture of LiCl and ZnCl2 in a sealed glass ampoule at 403 K for two months. Heating at 532 K for a week followed by quenching in ice water results in olivine-type Li2ZnCl4 (crystal structure shown in Fig. 7). Additionally, the transformation from normal spinel to olivine also changes the space group from Fd3m to Pnma. In the olivine structure,69 Cl ions are densely packed in a hexagonal arrangement around Li ions in the octahedra. The activation energy for conduction through octahedra is slightly lower than in the normal spinel structure. The lower calculated density (d = 1.529) compared to normal spinel (d = 1.601) indicates that the olivine structure is more open.
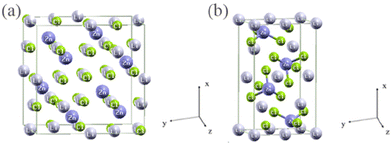 |
| Fig. 7 (a) Spinel (cubic) and (b) olivine (orthorhombic) structures of the Li2ZnCl4 compound.67 Reprinted with permission from ref. 67. Copyright (2022) Elsevier. | |
Kanno and others70 discovered that Li2MCl4 (M = Cr, Fe, Co) has a distorted spinel structure. The distortion is caused by a 1
:
1 ordering of Li and divalent metal ions on the octahedral sites. This distorted structure is defined as a deficient ordered rock-salt type SnMn2S4 (space group Cmmm).71,72 Importantly, the distorted structure results in a significant decrease in ionic conductivity.
The Suzuki phase was proposed by Suzuki in 1960.73 This structure is a rock salt derivative with an upper structure of LiCl type, where cations (Li+ and M2+) are arranged in an ordered manner, and there are vacancies at octahedral positions.74 Unlike the anti-perovskite structure, the MX6 octahedra in the Suzuki structure are isolated from each other. According to reports by H. D. Lutz and others,74 the formation of the Suzuki phase is only possible when the radius ratio RIIM/RIM < 1, such as in the case of Fe, V, and Co. Unlike LiCl,74–76 the presence of vacancies in Li6MCl8 promotes faster conduction of Li+ ions. Experimental results show that when measuring ionic conductivity at 350 °C, the arrangement of cations changes from ordered to disordered, further confirming the critical influence of cation arrangement at octahedral positions on ion migration.
In general, divalent metal electrolytes encompass a wide range of electrolyte types and intricate structures, with overall ion conductivity not being very optimal. The most notable structure is the spinel structure. Unfortunately, research on this particular structure has been exceedingly limited in recent years. Consequently, an urgent challenge currently confronting us is to conduct more thorough and extensive research on these metals.
3 Synthesis method of halide solid electrolytes
Currently, the preparation methods for HSEs typically include solid-phase methods (ball milling or ball milling and sintering) and liquid-phase methods. The two synthesis methods of Li3InCl6 are exemplified in Fig. 8. In addition, there are also a few reports on gas-phase methods77 and ammonia-assisted wet chemical methods.78
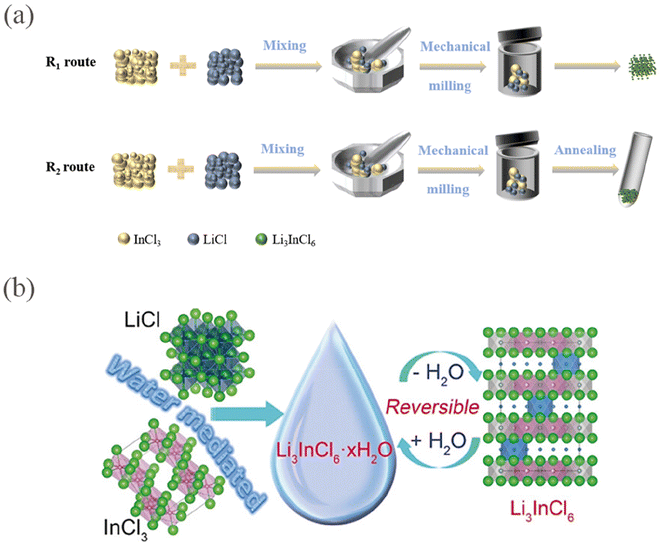 |
| Fig. 8 (a) Schematics of the synthesis of the Li3InCl6 solid electrolytes, including ball-milling (R1) followed by an annealing (R2) step. (b) Illustration of water-mediated synthesis route for Li3InCl6 and the reversible interconversion between the hydrated Li3InCl6·xH2O and dehydrated Li3InCl6.56 Reprinted with permission from ref. 56. Copyright (2019) Wiley. | |
3.1 Solid-phase reaction synthesis (ball milling, ball milling and sintering)
Halides exhibit strong hygroscopic properties, and the majority of solid-phase synthesis methods necessitate an inert gas atmosphere, such as argon or nitrogen. Early synthesis processes often require a significant amount of time, as seen in the case of Li2MCl4 (M = Mg, Mn, Cd),61 where the raw materials must be heated for a week after grinding. Similarly, Li3MBr6 (M = Sm–Lu, Y)41 was synthesized in a quartz ampoule at 400 °C for two weeks. This preparation method greatly limits industrial production.
High-energy ball milling has now become one of the mainstream methods for solid electrolyte synthesis. It refines the raw materials through the impact between mill ball and the original materials, providing the activation energy required for the reaction, and significantly reducing preparation time. Schlem et al.42 optimized the traditional ampoule synthesis method and prepared Li3YCl6 and Li3YBr6 using ball milling technique. There are mainly two types of popular ball milling methods: (1) Direct ball milling method, which does not require any additional processing of the materials. For example, Li2.99Y0.99Zr0.01Br1.6Cl4.479 can be obtained through one-step ball milling, and the non-periodic features induced by intense ball milling lead to high ionic conductivity. (2) In a two-step ball milling method, Li2ZrCl6 prepared by Chen et al.80 was initially ball milled for 12 hours at 600 rpm with a low ratio of ball and material, and in the second step, ball milling was conducted for 48 hours at speeds ranging from 600 to 900 rpm with higher ratios of ball and material. Through comparison, Li2ZrCl6 synthesized using the two-step ball milling method exhibited higher ionic conductivity.
Another common approach is to conduct heat treatment following ball milling. Some halides exhibit poor crystallinity after basic mechanical ball milling, resulting in low ionic conductivity. High-temperature annealing is the most commonly used method to improve crystallinity in materials like Li3InCl6 and Li2Sc2/3Cl6.35,81 Annealing generally increases the crystallinity of the product and enhances ionic conductivity. However, research has found that annealed Li3InCl6 not only increases ionic conductivity but also leads to an increase in impurities in the sample, attributed to the formation of InOCl under the influence of humidity.82 Nevertheless, the proportion of impurities is very small. After annealing, the ion conductivity of Li2ZrCl6 and Li3ErCl625,31 decreased. The reason for this is that the annealing process suppressed the metastable non-periodic features, which resulted in a decrease in ion conductivity as the number of non-periodic features decreased.
3.2 Liquid phase synthesis
A common method for synthesizing halides is solid-phase synthesis, but the products produced through this process are sensitive to moisture. Sun et al.56 reported a method for preparing Li3InCl6 in an aqueous solution system, as shown in Fig. 7b. Li3InCl6·xH2O is generated by reacting LiCl and InCl3 in appropriate molar ratios at room temperature in H2O solution, and pure phase LIC is obtained by vacuum heating at 200 °C. At this point, it is in a highly crystalline state, with an ion conductivity higher than that of LIC produced by solid-phase methods. Additionally, the reversible conversion between LIC and LIC·2H2O ensures resistance to air and humidity. Liu et al.82 tested the effects of high vacuum (HV; 10−3 Torr), low vacuum (LV; 10−1 Torr), Ar, and N2 (1 atm) drying environments on the microstructure and ion conductivity of LIC. The study found that drying under HV conditions was conducive to forming smaller particles. The removal of crystalline water during heating benefited from shorter diffusion lengths and larger surface areas. Additionally, the ion conductivity was improved (2.70 mS cm−1) under reduced oxygen contamination. This study provides insights for synthesizing high-quality LIC and is also beneficial for the synthesis of other water-mediated solid electrolytes.
4 Conduction mechanism of Li+ in halide solid electrolytes
The classic diffusion model describes ion transport as a single ion jumping from one lattice site to another through interconnected diffusion channels within the crystal structure framework. This is currently the basis for understanding ion diffusion in solids. The tetrahedral coordination of Li in sulfides (with a framework of covalent bonds) is geometrically similar to the body-centered cubic (bcc) anion sub-lattice, and the diffusion rate of Li+ in the bcc structure is superior to other closed frameworks. The oxides require covalent doping to achieve high Li+ conduction through cooperative migration. The halides are based on tight anion stacking structures with ionic bonds for Li+ migration, where Cl− and Br− can rapidly transport Li+ in different anion sub-lattices. Compared to sulfides and oxides, halides have fewer lattice restrictions, and the fundamental difference between ionic and covalent bonds in terms of gaining and losing electrons, results in different migration phenomena and diffusion mechanisms for Li+.34,83–85 The structure of halides is the main factor determining ionic conductivity. This section will provide a detailed discussion on the diffusion mechanism of Li+ in four crystal structures and the influencing factors.
4.1 Conduction mechanism of various crystal structures
4.1.1 Monoclinic structure (space group C2/m).
Liang et al.34 discussed the effect of the value of x in LixScCl3+x30 on Li+ diffusion. When x = 3, there is an optimal Li+ diffusion channel and vacancy concentration. Li+ jumps between two adjacent octahedral sites through the tetrahedral interstice, forming a three-directionally isotropic channel (Oct–Tet–Oct) (Fig. 9a). When x > 3, although the structure remains unchanged from Li3ScCl6, the decrease in Sc occupancy leads to an increase in Li+ concentration and a decrease in diffusion vacancies for Li. When x < 3, the Coulomb forces between Sc3+ and Li+ repel each other, blocking the tetrahedral interstice sites near Sc3+, which leads to a decrease in ion jump rate. In Li3YBr6,22 the migration paths of Li+ in all directions are connected through tetrahedral gaps (Oct1–[Tet1 or Tet2]–Oct2, Oct1–Tet3–Oct3) to form a three-dimensional channel (Fig. 9b). Jia et al.86 discovered through AIMD simulation that the highly symmetric ccp structure in Li3InCl6 forms an isotropic three-dimensional channel for the motion trajectory of Li+. The adjacent Li+ ions are restricted to a large octahedral unit due to the longer bond length between In and Cl, while the larger channels along the migration path make it easier for Li+ to diffuse. The highly localized position of In in the unit cell stabilizes the entire structure, enabling Li+ migration while inhibiting Cl− diffusion.
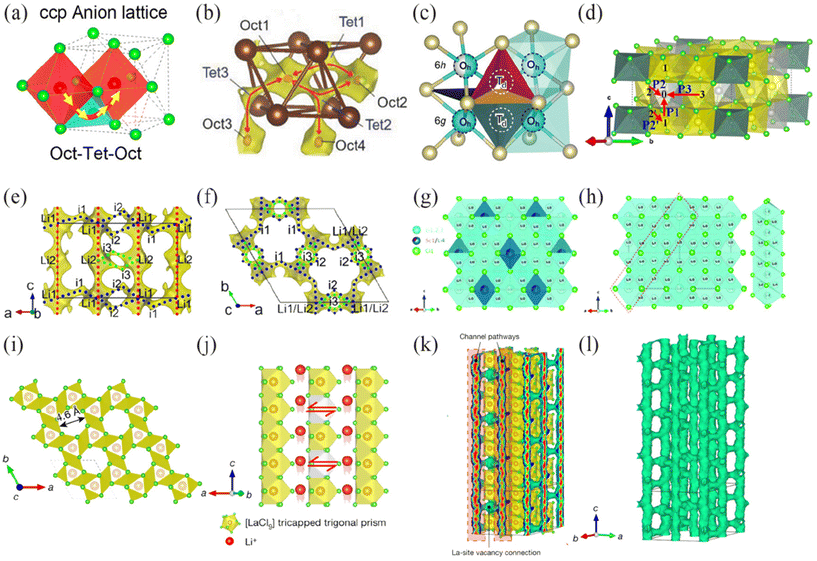 |
| Fig. 9 (a) The Li+ migration pathways in ccp-anion stacking sublattice of Li3ScCl6 structure.34 Reprinted with permission from ref. 34. Copyright (2020) American Chemical Society. (b) Li+ migration path in Li3InBr6.22 Reprinted with permission from ref. 22. Copyright (2018) Wiley. (c) Different transition areas for possible lithium diffusion in Li3ErCl6.87 Reprinted with permission from ref. 87. Copyright (2019) Wiley. (d) Ionic conduction pathway of Li3YCl6. The gray spheres represent Li vacancies.88 Reprinted with permission from ref. 88. Copyright (2021) American Chemical Society. (e) and (f) Li-ion migration pathways of Li2ZrCl6.32 Reprinted with permission from ref. 32. Copyright (2021) Springer Nature. (g) and (h) 3D Li+ ion diffusion pathway of disordered spine Li2/3ScCl4.91 Reprinted with permission from ref. 91. Copyright (2023) Royal Society of Chemistry. (i) Top view of the LaCl3 lattice along the c axis to show the unit cell (dashed square) and the abundant, intrinsically pre-existing channels of inner diameter 4.6 Å. (j) Side view of the vacancy-contained LaCl3 lattice indicating Li+ migration along the 1D channel (red spheres) and between adjacent channels (bidirectional arrows; vacancies are represented by the grey tricapped trigonal prisms). (k) Li+ probability density, represented by green isosurfaces from AIMD simulations at 900 K in the vacancy-contained LaCl3 lattice. (l) Isolated Li+ probability density isosurfaces by removal of all [LaCl9] polyhedrons to show the excellent interconnectivity of the 3D Li+ migration pathways.92 Reprinted with permission from ref. 92. Copyright (2023) Springer Nature. | |
4.1.2 Trigonal structure (space group P
m1).
Li3YCl6, Li3ErCl6, and Li2ZrCl6 all have a trigonal structure (space group P
m1), and are the most extensively studied in this structure. Zeier et al.87 proposed four possible migration pathways for the polyhedra of Li+ diffusion region in Li3ErCl6: (1) Jumping along the z-direction on the shared face of LiCl65− octahedra to form the Oh(6h)–Oh(6g) pathway. (2) Jumping on the (002) plane, Oh(6h)–Td–Oh(6h). (3) Jumping on the (001) plane, Oh(6g)–Td–Oh(6g). (4) Jumping between two adjacent tetrahedra, Td–Td. Complete occupation of the 6g site may lead to faster anisotropic migration of pathways 1 and 2 (Fig. 9c). In Li3YCl6, two adjacent octahedra are connected along the a and b axes by sharing edges,88 and they are connected along the c axis by sharing faces. On the a and b axes, Li+ ions move from octahedral (O) site to the adjacent octahedral (O) site via the tetrahedral (T) site, creating two O–T–O migration paths. On the c axis, Li+ jumps between adjacent octahedral (O) sites, forming an O–O path (Fig. 9d). The climbing image nudged elastic band (CI-NEB) method calculates the migration energy barriers of three paths as follows: two O–T–O migration paths (0.27–0.52 eV), and O–O path (0.19 eV). The results show that in LYC, Li+ is more inclined to migrate along the c-axis. Ertekin et al.89 conducted a more in-depth study on Li+ conduction in LYC. When the anion framework is frozen, Li+ diffusion is almost completely inhibited, confirming that the migration of Li+ is closely related to the vibration of the densely packed anion framework. Li et al.86 demonstrated through calculations that the migration trajectory of the c-axis is more continuous than that of the a or b axes. The difference in migration energy barriers in three directions can be explained by the weaker bonding between Li and Cl in the LiCl6 octahedron, making it easier for Li to leave the host. This anisotropic diffusion pathway leads to an overall decrease in the ionic conductivity of LYC.
Compared to other isomorphic chloride systems, Li2ZrCl625 has smaller lattice parameters and a lower occupancy rate of Zr4+ sites. Calculations using the bond valence site energy (BVSE) method indicate that the Li1–Li2–Li1 path in the [001], direction is the optimal pathway for Li+ migration simultaneously with the Li1–i1–Li1–i2–Li1 and Li2–i3–Li2 paths form an anisotropic three-dimensional network (Fig. 9e). However, this crystal structure does not facilitate Li+ migration. The high ionic conductivity of Li2ZrCl6 at room temperature is attributed to the non-periodic features induced by intense ball milling.
4.1.3 Orthorhombic structure (space group Pnma).
After annealing, Li3YCl6 will exhibit an orthorhombic structure with a space group of Pnma, which differs mainly from P
m1 in the arrangement of octahedra. Additionally, in the Pnma space group, some Li sites are occupied, and there are no vacant sites for Y.90 Although LYC has two different structures, the transport pathways of Li+ all form anisotropic three-dimensional networks and preferentially diffuse along the c-axis.
4.1.4 Cubic structure (spinel).
The common feature of Li2Sc2/3Cl4 and Li2MCl4 (M = Mg, Fe, Cr, Mn) is the disordered distribution of Li+ on the octahedral sites. Li2Sc2/3Cl4 is the first spinel-type superionic halide, with an ion conductivity three orders of magnitude higher than other spinel phases. In the crystal lattice of Li2Sc2/3Cl4, there are multiple Li sites within the unit cell. The Li+ at other sites can migrate through a rigid framework formed by Li/Sc sharing positions, creating a 3D diffusion pathway with the shared octahedral and tetrahedral Li1,2,3 sites (Fig. 9f). The new diffusion pathways and numerous vacancies contribute to the relatively high ionic conductivity of Li2Sc2/3Cl4.35,37,60 The Li2Sc2/3Cl4 computational model established by Han et al.91 suggests that the promotion of fast Li+ transport is due to the small electrostatic repulsion between cations. In addition to the fully connected three-dimensional network, there are additional diffusion pathways at other sites to facilitate Li+ migration. In Li2MgCl4,35 Li+ migrates through the octahedral surface shared with the tetrahedral edges. The lower ionic conductivity is attributed to fewer Li+ vacancies, and the presence of Mg2+ in the main diffusion path of Li+ hinders its migration.
4.1.5 UCl3-type crystal framework.
In the UCl3 lattice,39 chloride ions are arranged in a non-close-packed manner, forming abundant one-dimensional large-sized channels. By inducing lanthanum vacancies, it is possible to achieve continuous conductivity in three-dimensional space, endowing lithium ions with the ability to freely migrate throughout the entire crystal network. Yao et al.92 prepared Li0.388Ta0.238La0.475Cl3 based on the characteristics of the UCl3 lattice, which exhibits a high ionic conductivity of 3.02 mS cm−1 at room temperature. Although the LaCl3 lattice is rich in octahedral positions, its inherent single-dimensional transport path is insufficient to support high ionic conductivity (Fig. 9i). By doping with Ta to introduce lanthanum vacancies, these vacancies trigger strong exchange interactions between large-sized high-speed ion channels and adjacent channels, thereby constructing initial three-dimensional penetrating ion flow channels (Fig. 9j–l). In addition, this lattice has strong tolerance and can accommodate various ions from +1 to +6 without disrupting its basic UCl3-type framework structure.
4.1.6 Amorphous halides.
The current report indicates that amorphous halides can be specifically categorized into two groups: glassy and clay-like states. Yao and his team's28 research on LiTaCl6 through molecular dynamics simulations revealed that the migration of Li ions occurs between the octahedral and tetrahedral channels, involving gap transitions between Li–Ta–Cl. This demonstrates a three-dimensional directional transport mechanism similar to Li2ZrCl6. However, the ion migration path in LiTaCl6 includes more intermediates between Li–Ta–Cl, and all energy barriers during the migration process are lower than in Li2ZrCl6. The reason why the clay-like 2LiCl–GaF393 exhibits high ionic conductivity is due to the presence of mixed anions adjusting the local structure and energy characteristics. In this material, Ga shows enhanced coordination flexibility, mainly attributed to two points: firstly, Cl− creates a relatively spacious space around Ga due to its smaller ionic radius, available for Cl− and F− coordination; secondly, the electronegativity of Cl (3.16) is close to F (3.98), promoting a more uniform electron density distribution in Ga(Cl, F)n, beneficial for maintaining stability under a wider range of coordination numbers (n = 4, 5, 6). The environment in which Li+ is located is defined by the mixed halide anions around Ga, collectively promoting short-range ordered structures in the amorphous framework of 2LiCl–GaF3.
Observing and analyzing the typical crystal structures mentioned above reveals that compounds with monoclinic structures featuring cubic close-packing, such as Li3ScCl6, Li3YBr6, and Li3InCl6, exhibit similar or identical fast ion migration pathways, leading to superior ion conductivity compared to several other types. It is worth noting that although a single UCl3-type lattice does not support fast ion conduction due to its one-dimensional channel characteristics, the introduction of external elements to create lanthanide vacancies and construct a three-dimensional ion migration network enables fast ion conduction. This discovery provides insights for the future development of novel electrolyte materials from the perspective of crystal structure design.
4.2 The impact of defects on Li+ conduction
Defects provide additional diffusion space and storage sites for ions, which are considered the most effective solution for fundamentally improving ion diffusion kinetics.94 In solid halide electrolytes, the primary factor influencing ion conduction is the vacancies created by defects to facilitate their movement. Vacancy is one of the common crystal defects. Ren et al.95 constructed vacancy defect structures of Li3InCl6 and Li3ScCl6 (Fig. 10a and b). The concentration of Li inside the unit cell decreases with the introduction of Li vacancy defects, leading to a significant increase in Li+ diffusion capability. These defects provide Li with transport channels while reducing the migration energy barrier. Research shows that the number of Li site defects within an appropriate range can enhance the migration rate of Li+ in Li3InCl6 and Li3ScCl6. Kim et al.96 established four models, including normal Li3InCl6, In occupying 4g sites (In4g), Cl vacancies (VCl), and the coexistence of In4g and VCl, to study the effect of vacancy defects on the ionic conductivity (Fig. 10c–f). The presence of vacancy defects reduces the migration energy barrier of Li+, promotes partial Li penetration to the vacant octahedral sites in the In layer, increases the concentration of Li layer vacancies, accelerates the diffusion rate of Li+, and results in higher ionic conductivity.
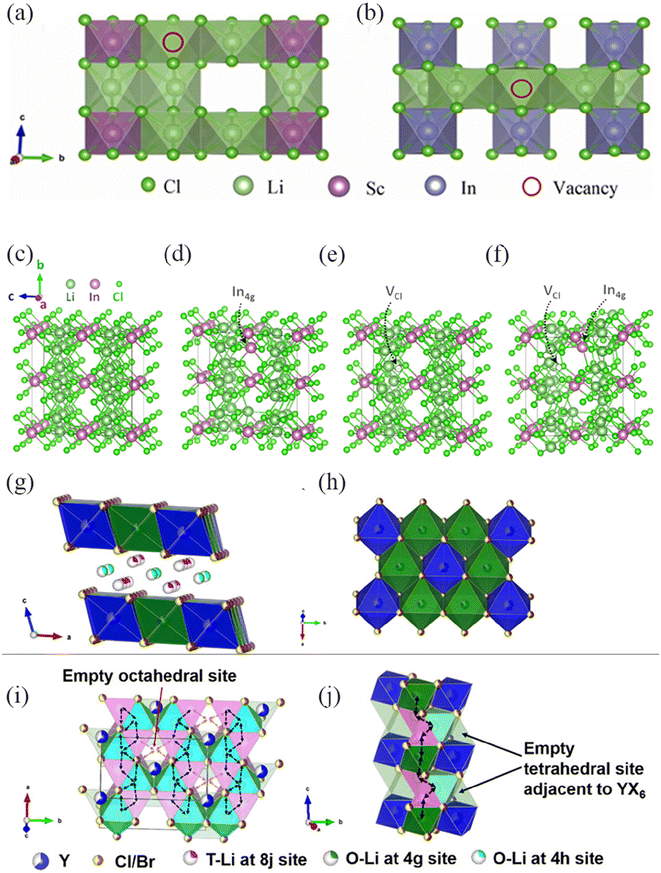 |
| Fig. 10 (a) and (b) Li3ScCl6 and Li3InCl6 defect models constructed according to the vacancy formation energy, where the red part is the distribution of lithium site defects.95 Reprinted with permission from ref. 95. Copyright (2023) Elsevier. (c) Supercell with no point defects introduced (LIC), (d) with a 4g site occupancy by In (LIC_In4g), (e) with a Cl vacancy (LIC_VCl), (f) with both a 4g site occupancy by In and a Cl vacancy (LIC_In4g–VCl).96 Reprinted with permission from ref. 96. Copyright (2022) Royal Society of Chemistry. (g and h) Crystal structure of LYBC-HP viewed along different orientations. (i and j) Proposed Li+ diffusion pathways along ab-plane and c-direction.97 Reprinted with permission from ref. 97. Copyright (2021) American Chemical Society. | |
Stacking faults are a common type of crystal defect. Liu et al.97 obtained tetrahedral (T–Li) and octahedral (O–Li) coexisting Li3Y(Br3Cl3) by mixing Br and Cl ions (Fig. 10g–j). Axial stacking defects result in local order and long-range disorder within the crystal. T–Li creates more vacancies on the octahedra, leading to a cooperative diffusion mechanism, which is one of the reasons for the high ionic conductivity of Li3Y(Br3Cl3). Sebti et al.98 found that the mechanosynthesis of Li3YCl6 (LYC) exhibited a phenomenon of a high concentration of stacking faults and the coexistence of lithium layer defects. The presence of stacking faults and lithium layer defects establishes additional interconnected sites, creates more interlayer channels, and reduces the transport barrier of Li+. However, when exposed to environments above 60 °C, the defect concentration and Li+ migration rate decreased, indicating a strong correlation between defect concentration and the ionic conductivity of LYC. This also demonstrates the important role of defects in promoting the structural conduction of Li+.
The arrangement of cations is a crucial factor that determines the high ionic conductivity of halide superionic conductors. Zhong et al.99 simulated the effect of Zr disorder/order on Li2ZrCl6 ion migration. In their study of the α-hcp and β-fcc phases of LZC, they found that compared to the ground state order, when Zr is completely disordered, Li+ diffusion exhibits Arrhenius behavior at all temperatures. The Li/vacancy disorder caused by Zr disorder promotes the rapid conduction of Li+, but spontaneous disorder of Zr does not occur at any reasonable temperature, only non-equilibrium high-energy processes (such as mechanical ball milling) can achieve a disordered state. This also explains the decrease in ionic conductivity of LZC after high-temperature annealing.
In summary, defects within the crystal structure when designing halide solid electrolytes are an important research focus that cannot be ignored. In addition, multiple factors such as grain boundary characteristics, impurity content, and mechanical-chemical interactions during the synthesis process significantly influence the transport behavior of Li+ at various levels.
4.3 Modified strategy
4.3.1 Different/same-valence cation doping.
Doping with cations of different or same valence is a commonly used method for modifying electrolytes, mainly by enhancing ion conductivity by inducing lattice distortion, increasing vacancies, and altering the crystal structure. The ionic conductivity before and after modification is shown in Table 3.
Table 3 Comparison of ionic conductivity before and after modification
Modified strategy |
Original sample |
mS cm−1 |
Modified |
mS cm−1 |
Ref. |
Cation doping |
Li2MgCl4 |
0.00019 |
Li1.6Zn0.2MgCl4 |
0.012 |
101
|
Li3ScCl6 |
0.8 |
Li2.375Sc0.375Zr0.625Cl6 |
2.2 |
102
|
Li2ZrCl6 |
0.4 |
Li2.25Zr0.75Fe0.25Cl6 |
1 |
33
|
Li3ErCl6 |
0.33 |
Li2.633Er0.633Zr0.367Cl6 |
1.1 |
104
|
Li3YCl6 |
0.51 |
Li2.5Y0.5Zr0.5Cl6 |
1.4 |
104
|
Li2ZrCl6 |
0.32 |
Li2.25Zr0.75In0.25Cl6 |
1.08 |
105
|
Li2ZrCl6 |
0.5 |
Li2.8Zr0.2In0.8Cl6 |
1.4 |
107
|
Fluoridation |
Li3YCl6 |
0.224 |
Li3YCl5.97F0.03 |
0.239 |
109
|
Li3InCl6 |
0.55 |
Li3InCl5.8F0.2 |
1.2 |
110
|
High-entropy |
Li3InCl6 |
0.849 |
Li2.75Y0.16Er0.16Yb0.16In0.25Zr0.25Cl6 |
1.171 |
111
|
Li3InCl6 |
1.27 |
Li2.8In0.2Sc0.2Yb0.2Lu0.2Zr0.2Cl6 |
2.13 |
112
|
Chen et al.100 reported Zn-doped Li1.6Zn0.2MgCl4, which showed an increase in ion conductivity by two orders of magnitude compared to Li2MgCl4 (1.2 × 10−5 S cm−1). The Li+ is slightly larger than Zn2+, and when Zn enters the Li2MgCl4 lattice, the unit cell volume decreases by 0.89%. However, due to charge balance, the substitution of Zn2+ for Li+ creates Li vacancies (Fig. 11a and b). Element substitution not only affects the vacancy concentration but also smoothens the overall migration energy barrier. Vacancies within a certain range contribute to enhancing Li+ conductivity, but when there are too many vacancies, the ion conductivity decreases instead. Wu et al.101 introduced Zr/Hf into Li3ScCl6 using a strategy of adjusting the vacancy concentration to prepare Li2.375Sc0.375Zr0.625Cl6 and Li2.375Sc0.375Hf0.375Cl6. After successfully substituting Zr4+/Hf4+, due to lattice contraction in the c-direction, the jump distance of Li+ along the axis becomes shorter. Simultaneously, an empty Li site is instantly occupied by another Li ion, resulting in excellent ion conductivity due to the rapid ion exchange rate and larger diffusion bottleneck. Usami and colleagues102 found that doping Li3InCl6 with Nb and Zr resulted in an increase in the volume of the unit cell. The relationship between the sizes of the three ions is as follows: In3+ > Zr4+ > Nb5+, and the unit cell volume replaced by Nb5+ is larger than that of Zr4+. It is not difficult to see that the increase in lattice constant is not directly caused by the substitution of cations. When Nb or Zr enters the unit cell, vacancies are created, causing electrostatic repulsion between internal anions, leading to an increase in unit cell volume as the vacancy space expands. In addition to the impact of vacancies on Li+ transport, first-principles molecular dynamics (FPMD) calculations show that at 373 K–673 K, Li+ transitions from existing only in In-deficient layers to three-dimensional diffusion within and between layers. At 673 K and above, a phase transition occurs where Li/vacancy arrangements become disordered (Fig. 11c and d), and the increase in disorder also facilitates the migration of Li+ to some extent. Replacing Zr with Fe in Li2ZrCl632 not only reduces the Coulomb repulsion between Li+ and other metal cations, lowering the migration energy barrier of Li+ but also, after Fe enters the lattice, the unit cell volume decreases, and the channels between the octahedra being connected along the c-axis direction, making it easier for Li+ to diffuse.
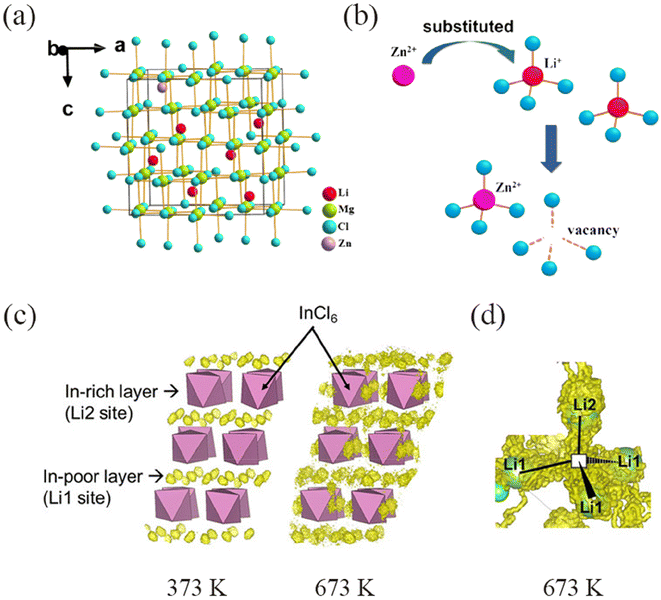 |
| Fig. 11 (a) Crystal structure of Li2-2xZnxMgCl4. (b) The diagram of Li vacancy induced by Zn substitution.100 Reprinted with permission from ref. 100. Copyright (2023) Elsevier. (c) Population density of Li ions in LIC (Li48In16Cl96) derived from FPMD calculations at 373 and 673 K, respectively. (d) Diffusion pathway of Li ions at 673 K.102 Reprinted with permission from ref. 102. Copyright (2023) AIP Publishing. | |
The trigonal structured Li3YCl6 is considered a poor ion conductor. Park et al.103 reported Zr-doped Li3MCl6 (M = Y, Er), where phase transitions occur at higher doping levels. The rearrangement of metal ions and tilting of octahedra transform the trigonal phase into an orthorhombic phase. The additional tetrahedral sites created play a crucial role in altering the overall migration energy barrier. A substantial number of vacancies create three-dimensional channels for Li+ migration, resulting in a significant increase in ionic conductivity at room temperature by an order of magnitude. Based on the theory of hard and soft acids and bases, compounds formed by soft acid In are less likely to react with hard base H2O. Xie et al.104 prepared Li2.25Zr0.75In0.25Cl6, where the covalent substitution strategy effectively increased the ionic conductivity. Excessive substitution and annealing, however, can cause phase transitions and decrease the ionic conductivity. Transmission electron microscopy revealed that the samples after ball milling exhibited a disordered structure, which could be a contributing factor to the high ionic conductivity. Ma and Tu105,106 reported a mixed-phase Li2.8Zr0.2In0.8Cl6 containing P
m1 and C2/m space groups after ball milling, which completely transformed into a monoclinic structure after annealing. When the In substitution amount is between 0.3–0.8, In doping results in lattice expansion in three directions, and the migration barrier for Li+ along all migration paths gradually decreases. When the substitution amount exceeds 0.8, the unit cell abnormally shrinks, and the reason for the decrease in ionic conductivity, in addition to the increase in migration energy barrier, may also be attributed to the insufficient Li vacancies available to facilitate Li+ migration.
Currently, in the methods of doping halides with cations of different or same valence, a very inspiring idea is to alter the crystal structure, transitioning from trigonal to orthorhombic, and from orthorhombic to a monoclinic phase with isotropic ion conduction. The monoclinic phase, through lattice expansion induced by doping, can enhance ionic conductivity, offering a pathway for future modifications to the structure of HSEs.
4.3.2 Fluoridation strategy.
Fluorination is the most commonly used strategy to enhance the stability of electrolytes. In addition, the introduction of fluoride ions into the lattice can lead to local distortion, which can impact the ionic conductivity. Kim et al.107 found additional vacancies in fluorinated Li3InCl6. The migration barrier near F is lower than at other positions, but the Coulomb force of F on Li suppresses the movement range of surrounding Li, affecting Li+ diffusion. Therefore, controlling the concentration of F reasonably can achieve the best doping effect. When Li moves to an empty site in the octahedral layer of In, the initial site forms a new vacancy, which promotes the migration of Li+. This is not only the main migration channel for Li+, but also the key to the entire diffusion process. Zhong et al.108 reported Li3YCl5.97F0.03, where trace amounts of F do not affect the ionic conductivity, but the LiF formed at the interface of electrolyte and negative electrode can not only enhance the oxidation stability of the electrolyte but also inhibit its decomposition. Wang et al.109 prepared Li3InCl5.8F0.2, and found, through the Arrhenius equation, that the introduction of F can significantly reduce the activation energy, leading to a notable increase in ionic conductivity. Additionally, the modified Li3InCl6 exhibited a significant decrease in electronic conductivity, effectively suppressing the growth of lithium dendrites and extending the cycle life of the battery.
4.3.3 High-entropy modification.
High-entropy strategy is an effective approach to promote Li/vacancy disorder and increase configurational entropy. Huang et al.110 reported a new high-entropy halide Li2.75Y0.16Er0.16Yb0.16In0.25Zr0.25Cl16 (HE-LIC). The original Li3InCl6 is a single phase with a monoclinic structure. However, when Y and Zr are introduced, the structure becomes a mixed phase. With the further introduction of Er and Yb, the structure transforms into a monoclinic phase with a small amount of rock salt LiCl. This also indicates that the high-entropy-driven structural stability can promote the solid solution of various cations in LIC. The lattice distortion caused by introducing different cations serves a dual function: on one hand, it can effectively regulate the diffusion kinetics of Li+ and Cl−, on the other hand, this distortion process creates unobstructed three-dimensional migration channels for Li+, thereby achieving high ionic conductivity. Similarly, Zhao et al.111 improved the configurational entropy by increasing the number of main elements, thereby altering the thermodynamic stability and kinetic properties of the material to achieve fast ion conduction in the preparation of Li2.8In0.2Sc0.2Yb0.2Lu0.2Zr0.2Cl6. This material exhibits an ionic conductivity of up to 2.13 mS cm−1.
5 Oxyhalides
The topological structure of a specific anion arrangement determines the inherent mobility of Li+. However, closely packed anions in halides are not conducive to Li+ conduction, which seems to be related to monovalent halide anions. Introducing a mixed double anion strategy in halide matrices can enhance ion conductivity, as demonstrated in Li3Y(Br3Cl3).26,85,97 Emly and others studied oxyhalides with an anti-perovskite structure. Unlike other electrolytes, Li3OCl and Li3OBr exhibit a high number of vacancies for Li+ transport, offering a new avenue for modifying halides.112–115 The emergence of new lithium-ion conductors is largely the result of expanding known superionic compounds into new compositional spaces.85 A mixed anion system oxyhalides based on halides has now been reported.
5.1 Crystalline oxyhalides
Tanaka et al.26 prepared LiMOCl4 (M = Nb, Ta) with a conductivity greater than 10 mS cm−1 at room temperature. The use of high-valence cations to form corner-sharing polyhedra is expected to create pathways for Li+ migration. LiNbOCl4 (LNOC) has an orthorhombic structure (space group Cmc21), consisting of corner-sharing NbO2Cl4 octahedral chains and LiCl tetrahedra located between the chains (Fig. 12a–c). Compared to Li3YCl6 and Li3YBr6, the crystal lattice framework of LNOC is more open, with larger diffusion channels. This is also a significant factor contributing to the high ionic conductivity of LNOC. Further understanding of the diffusion mechanism of LNOC reveals that the moving ions diffuse independently without correlation, following a typical ion hopping mechanism similar to diffusion in liquids. Additionally, its higher oxidation stability enables long cycling and high capacity in batteries, offering new perspectives for solid-state electrolytes.
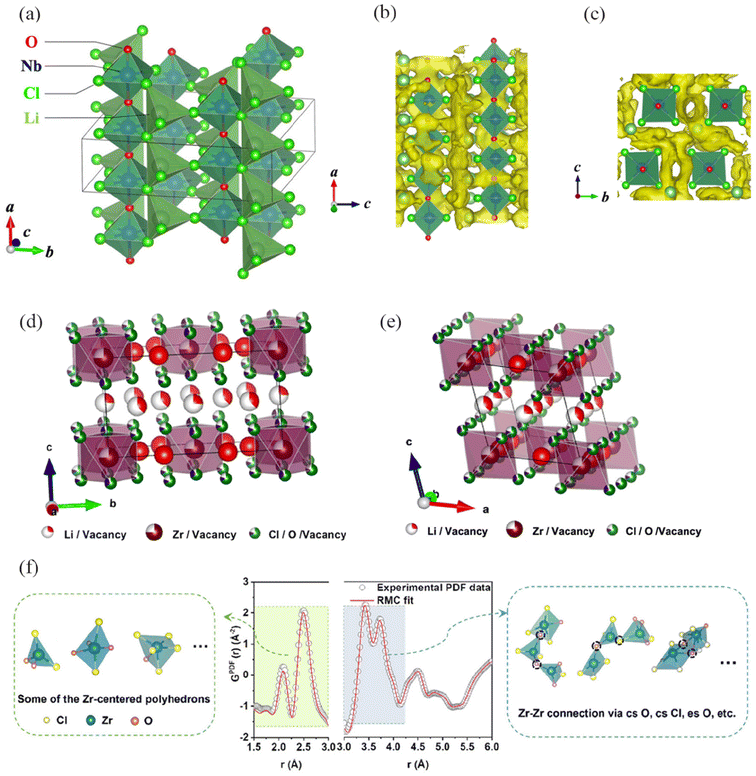 |
| Fig. 12 (a) Crystal structure of the LNOC. The Li-ion probability density map (yellow isosurface) along the (b) b-axis and (c) a-axis direction calculated by AIMD simulations at 800 K.26 Reprinted with permission from ref. 26. Copyright (2023) Wiley. (d and e) Atomic model for the Li3Zr0.75OCl4 materials.116 (f) Reverse Monte Carlo (RMC) fit (red line) to the experimental G(r) (gray circle) of Li3ZrCl4O1.5. Several possible basic building blocks in Li3ZrCl4O1.5 are shown on the left side. On the right side, the schemes show the connectivity that leads to the edge-sharing and corner-sharing Zr-centered polyhedra in Li3ZrCl4O1.5, respectively.120 Reprinted with permission from ref. 116 and 120. Copyright (2023 and 2024) American Chemical Society. | |
Ma et al.116 reported Li3Zr0.75OCl4 with a monoclinic structure. Currently, besides introducing expensive metals such as In and Er in the modification of Li2ZrCl6, it is challenging to achieve an ionic conductivity exceeding 1 mS cm−1 at room temperature. Starting with the monoclinic structure Li3ScCl6, which possesses rapid ion transport characteristics, the product obtained by replacing Sc with 0.75Zr is not a pure phase. Considering that vacancies are needed between transition metals to balance the charge difference between Sc3+ and Zr4+, O is used to replace Cl to introduce anionic vacancies. This results in Li3Zr0.75OCl6 with the same structure as Li3ScCl6 (Fig. 12d and e). In addition, unlike Sc, the Zr sites are not fully occupied, containing a large number of vacancies, which may provide an additional pathway for Li+ migration. BVSE analysis shows that the C2/m structure of Li3Zr0.75OCl6 is more conducive to lithium ion migration compared to the P
m1 structure of Li2ZrCl6. The migration energy barrier for the three-dimensional network pathway is only 0.306 eV, and ion conduction can occur in non-periodic features and crystal phases, both of which collectively determine the overall ionic conductivity.
Yu et al.117 enhanced the ionic conductivity of Li2ZrCl6 by substituting hard alkalis. The ionic conductivity of the monoclinic (metastable phase) Li3.1ZrCl4.9O0.1 reached 1.3 mS cm−1. In addition to the fast ion conduction of the monoclinic structure, the energy barrier on the Li+ migration path is lower after introducing O2−. Oxygen substitution stabilizes the Li interstitial sites, but this method is only effective for structures where tetrahedra and metal octahedra share corners. Li et al.118 reported a monoclinic Li2.22Zr1.11Cl5.33O0.67 with a room temperature ionic conductivity exceeding 1 mS cm−1. The lower activation energy is closely related to the changes in crystal structure and the occupation of lithium in different interstitial positions. When Li occupies more tetrahedral sites, the required jump distance for its migration is correspondingly shortened, which is crucial for enhancing high ionic conductivity. Due to the stronger Li+–O2− bonds compared to Li+–Cl−, Li2.22Zr1.11Cl5.33O0.67 exhibits higher structural thermal stability and solvent stability.
5.2 Amorphous oxyhalides
Sun et al.119 constructed an amorphous xLi2O-MCly (M = Nb, Ta) with a mixed anion structure. After optimization, the ion conductivity can reach 6.6 mS cm−1. The disordered and irregular [TaCl5–O] structure enables the formation of Li–Cl sub-lattices in the amorphous region, and the oxygen at bridging sites expands the migration channel radius, facilitating the entry of Li+. Furthermore, the unsaturated Ta–Cl⋯Li bonds between Li–Cl have weaker coulombic forces, enabling Li+ to transition easily between sites. A similar situation is also observed in Hf. In conclusion, the combination of amorphous terminal Cl− with abundant O2− plays a crucial role in the rapid migration of Li+. In addition, the group also controlled the amorphization degree of Li2ZrCl6 by introducing O,120 conducted an in-depth analysis of the Li3ZrCl4O1.5 structure, where the polyhedra centered on Zr are mainly connected by corner-sharing Cl and O, with Zr–X (X = O, Cl) bond lengths of disordered and distorted Zr–O/Cl polyhedra being averaged. The remaining polyhedra exhibit edge-sharing and a small amount of face-sharing (Fig. 12f). This unique structure enables its ionic conductivity to reach (1.35 ± 0.07) mS cm−1.
Li1.75ZrCl4.75O0.5 reported by Ma et al.121 exhibits an ionic conductivity of 2.42 mS cm−1 at room temperature, with the structure mainly being amorphous (where the crystalline phase is less than 20%: trigonal and monoclinic). It is important to emphasize that effective ion transport is achieved through a large amount of amorphous material when the crystal structure transitions to an amorphous state by introducing O into Li2ZrCl6. Hu et al.122 reported a viscoelastic amorphous LiAlCl4-75%O (LACO75) electrolyte, in which the ionic conductivity reached 1 mS cm−1 at 30 °C by replacing chlorine in tetrachloroaluminate with oxygen. This change resulted in an improvement of 3 orders of magnitude compared to LiAlCl4. As the oxygen content increased, the oxygen-containing tetrachloroaluminate transitioned a brittle molten salt a ductile glass. AIMD simulations revealed that the LACO75 structure is completely disordered, with oxygen connecting isolated aluminum atoms to form an Al–O–Al network. In molten the strong Al–O bonds are challenging to break, and during the contraction process of atomic rearrangement, they are further constrained by the Al–O–Al network, leading to the formation of a glassy state. The high ionic conductivity is attributed to the movement/rotation of segments, shorter Li+ hopping paths, and the presence of Al–O–Al chains.
6 Summary and outlook
This article reviews the synthesis methods, structure, corresponding ion conduction mechanisms, modification strategies of halide solid electrolytes, and finally elaborates on the breakthrough from halides to oxyhalides. Firstly, existing halide electrolytes are classified into five categories based on different types of metal elements, and detailed explanations are provided for the crystal structures and space groups of each category. Secondly, the preparation processes of common synthesis methods such as high-energy ball milling, annealing, and liquid-phase method are summarized. Thirdly, starting from four typical structures, the ion conduction mechanisms are organized and summarized, followed by a detailed explanation of three modification methods. Finally, the article describes the connection between halides and oxyhalides and introduces the structures of existing oxyhalides. At present, research on halides primarily focuses on the following aspects:
(1) Ionic conductivity. In order to effectively reduce the migration energy barrier of Li+, researchers use various modification methods: (1) doping with cations of different or same valence to increase Li vacancies. (2) substituting larger radius central metal elements for smaller ones to expand the lattice and enlarge Li+ migration channels. (3) adjusting the crystal structure. For instance, Li3InCl6 with a monoclinic structure demonstrates excellent ionic conductivity at room temperature, whereas economically advantageous Li2ZrCl6 does not exhibit remarkable ionic conductivity at room temperature. By substituting In for Zr to induce a transition from a trigonal to a monoclinic structure to enhance ionic conductivity.
(2) Synthesis process. High-energy ball milling is currently the most widely used synthesis method. The non-periodic characteristics resulting from intense ball milling lead to high ionic conductivity. However, the lengthy processing time and the substantial disparity between theoretical simulations and actual results have yet to be addressed. Recently, the proposed two-step ball milling method has somewhat enhanced the ionic conductivity of Li2ZrCl6, but the 60-hour synthesis process is discouraging. Research has shown that Li3InCl6 prepared by liquid-phase synthesis exhibits excellent electrochemical performance. However, it remains to be verified whether this synthesis method is applicable to other halide electrolytes.
(3) Oxygen anion intervention. Recently, research on the introduction of O2− into halides has been particularly focused on Li2ZrCl6. This modification strategy involves adjusting the structure and chemical properties of materials by introducing oxygen anions, aiming to enhance their ionic conductivity and electrochemical stability, which is particularly crucial for the application of solid-state batteries.
At the current stage, halides exhibit relatively balanced performance compared to sulfides and oxides, indicating promising prospects. However, most central metal atoms are relatively expensive, and there is still a significant gap in ion conductivity of halides and advanced sulfide solid electrolytes at room temperature. Here are some ideas: (1) Develop new low-cost halides. Current research focuses on In, Zr, and Y. Although Zr has low cost, its room temperature ion conductivity is not ideal. In and Y have higher costs, which seriously affects commercialization. Therefore, the exploration of new halide systems is still urgent. (2) Exploration of spinel-type materials. Research on spinel-type electrolytes is still limited, but Li2Sc2/3Cl4 reported by Xueliang Sun and others in 2022, exhibits excellent ion conductivity. We estimate that there is still significant room for improvement in spinel-type halides. (3) Oxygen anion involvement in other systems. Apart from Zr, Nb, Hf, and Ta, there have been no reports on other oxyhalides, but there are numerous existing halide systems. Combining research on the monoclinic structure or coexistence with other phases of Zr-based oxyhalides, can oxygen anions be directly introduced into halides with monoclinic structures to improve electrochemical performance? There has been little exploration of the amorphous state in oxyhalides. It is unknown whether crystalline or amorphous forms will be more advantageous. Further research is needed to understand the unknown properties and issues.
From the research on halide solid electrolytes in 2018 to the excellent performance of oxyhalides shown in 2023, it signifies that this field is in a rapid development stage, indicating that there will be more innovations and breakthroughs in the coming years. The potential applications of halides and oxyhalides in battery technology are particularly noteworthy, as they are expected to address the safety issues and performance limitations brought about by existing liquid electrolytes, and promote the development of battery technology towards higher energy density, longer cycle life, and greater safety.
Data availability
No primary research results, software or code have been included and no new data were generated or analysed as part of this review.
Conflicts of interest
There are no conflicts to declare.
References
- A. Manthiram, X. Yu and S. Wang, Lithium battery chemistries enabled by solid-state electrolytes, Nat. Rev. Mater., 2017, 2, 16103 CrossRef CAS.
- K. Zaghib, M. Dontigny, A. Guerfi, P. Charest, I. Rodrigues, A. Mauger and C. M. Julien, Safe and fast-charging Li-ion battery with long shelf life for power applications, J. Power Sources, 2011, 196, 3949–3954 CrossRef CAS.
- S. Chen, C. Yu, Q. Luo, C. Wei, L. Li, G. Li, S. Cheng and J. Xie, Research Progress of Lithium Metal Halide Solid Electrolytes, Acta Phys.-Chim. Sin., 2022, 39, 2210032 Search PubMed.
- N. Nitta, F. Wu, J. T. Lee and G. Yushin, Li-ion battery materials: present and future, Mater. Today, 2015, 18, 252–264 CrossRef CAS.
- G. Jia, Z. Deng, D. Ni, Z. Ji, D. Chen, X. Zhang, T. Wang, S. Li and Y. Zhao, Temperature-dependent compatibility study on halide solid-state electrolytes in solid-state batteries, Front. Chem., 2022, 10, 952875 CrossRef CAS PubMed.
- Z.-Y. Gu, X.-T. Wang, Y.-L. Heng, K.-Y. Zhang, H.-J. Liang, J.-L. Yang, E. H. Ang, P.-F. Wang, Y. You, F. Du and X.-L. Wu, Prospects and perspectives on advanced materials for sodium-ion batteries, Sci. Bull., 2023, 68, 2302–2306 CrossRef CAS PubMed.
- J.-Z. Guo, Z.-Y. Gu, M. Du, X.-X. Zhao, X.-T. Wang and X.-L. Wu, Emerging characterization techniques for delving polyanion-type cathode materials of sodium-ion batteries, Mater. Today, 2023, 66, 221–244 CrossRef CAS.
- S. Ling, B. Deng, R. Zhao, H. Lin, L. Kong, R. Zhang, Z. Lu, J. Bian and Y. Zhao, Revisiting the Role of Hydrogen in Lithium–Rich Antiperovskite Solid Electrolytes: New Insight in Lithium Ion and Hydrogen Dynamics, Adv. Energy Mater., 2022, 13, 2202847 CrossRef.
- Z. Zeng, X. Shi, M. Sun, H. Zhang, W. Luo, Y. Huang, B. Huang, Y. Du and C.-H. Yan, Stable all-solid-state Li-Te battery with Li3TbBr6 superionic conductor, Nano Res., 2023, 16, 9344–9351 CrossRef CAS.
- P. Jiang, G. Du, J. Cao, X. Zhang, C. Zou, Y. Liu and X. Lu, Solid–State Li Ion Batteries with Oxide Solid Electrolytes: Progress and Perspective, Energy Technol., 2023, 11, 2201288 CrossRef CAS.
- M. Shoji, E. J. Cheng, T. Kimura and K. Kanamura, Recent progress for all solid state battery using sulfide and oxide solid electrolytes, J. Phys. D: Appl. Phys., 2019, 52, 103001 CrossRef.
- R. Rajagopal, Y. Subramanian, Y. J. Jung, S. Kang and K.-S. Ryu, Preparation of Metal-Oxide-Doped Li7P2S8Br0.25I0.75 Solid Electrolytes for All-Solid-State Lithium Batteries, ACS Appl. Mater. Interfaces, 2023, 15, 21016–21026 CrossRef CAS PubMed.
- H. Muramatsu, A. Hayashi, T. Ohtomo, S. Hama and M. Tatsumisago, Structural change of Li2S–P2S5 sulfide solid electrolytes in the atmosphere, Solid State Ionics, 2011, 182, 116–119 CrossRef CAS.
- J. Wu, S. Liu, F. Han, X. Yao and C. Wang, Lithium/Sulfide All–Solid–State Batteries using Sulfide Electrolytes, Adv. Mater., 2021, 33, 2000751 CrossRef CAS PubMed.
- J. Wu, L. Shen, Z. Zhang, G. Liu, Z. Wang, D. Zhou, H. Wan, X. Xu and X. Yao, All-Solid-State Lithium Batteries
with Sulfide Electrolytes and Oxide Cathodes, Electrochem. Energy Rev., 2021, 4, 101–135 CrossRef CAS.
- C. Tang, Z. Xue, S. Weng, W. Wang, H. Shen, Y. Xiang, L. Liu and X. Peng, A Biodegradable Polyester-Based Polymer Electrolyte for Solid-State Lithium Batteries, Nanomaterials, 2023, 13, 3027 CrossRef CAS PubMed.
- K. Xie and L. Shi, Ultrathin PEO based electrolyte for high voltage lithium metal batteries enabled by polymer host-plasticizer interactions, J. Energy Storage, 2023, 68, 107640 CrossRef.
- Z. Yao, K. Zhu, X. Li, J. Zhang, J. Chen, J. Wang, K. Yan and J. Liu, 3D poly(vinylidene fluoride–hexafluoropropylen) nanofiber-reinforced PEO-based composite polymer electrolyte for high-voltage lithium metal batteries, Electrochim. Acta, 2022, 404, 139769 CrossRef CAS.
- X. Li, J. Liang, X. Yang, K. R. Adair, C. Wang, F. Zhao and X. Sun, Progress and perspectives on halide lithium conductors for all-solid-state lithium batteries, Energy Environ. Sci., 2020, 13, 1429–1461 RSC.
- H.-X. Mei, P. Piccardo, A. Cingolani and R. Spotorno, Unconventional solid-state electrolytes for lithium-based batteries: Recent advances and challenges, J. Power Sources, 2023, 553, 232257 CrossRef CAS.
- J. Kendall, E. D. Crittenden and H. K. Miller, A study of the factors influencing compound formation and solubility in fused salt mixtures, J. Am. Chem. Soc., 1923, 45, 963–996 CrossRef CAS.
- T. Asano, A. Sakai, S. Ouchi, M. Sakaida, A. Miyazaki and S. Hasegawa, Solid Halide Electrolytes with High Lithium-Ion Conductivity for Application in 4 V Class Bulk-Type All-Solid-State Batteries, Adv. Mater., 2018, 30, 1803075 CrossRef PubMed.
- B. He, F. Zhang, Y. Xin, C. Xu, X. Hu, X. Wu, Y. Yang and H. Tian, Halogen chemistry of solid electrolytes in all-solid-state batteries, Nat. Rev. Chem., 2023, 7, 826–842 Search PubMed.
- C. Wang, J. Liang, J. T. Kim and X. Sun, Prospects of halide-based all-solid-state batteries: From material design to practical application, Sci. Adv., 2022, 8, eadc9516 CrossRef CAS PubMed.
- K. Wang, Q. Ren, Z. Gu, C. Duan, J. Wang, F. Zhu, Y. Fu, J. Hao, J. Zhu, L. He, C.-W. Wang, Y. Lu, J. Ma and C. Ma, A cost-effective and humidity-tolerant chloride solid electrolyte for lithium batteries, Nat. Commun., 2021, 12, 4410 CrossRef CAS PubMed.
- Y. Tanaka, K. Ueno, K. Mizuno, K. Takeuchi, T. Asano and A. Sakai, New Oxyhalide Solid Electrolytes with High Lithium Ionic Conductivity >10 mS cm−1for All–Solid–State Batteries, Angew. Chem., Int. Ed., 2023, 62, e202217581 CrossRef CAS PubMed.
- K. Tuo, C. Sun and S. Liu, Recent Progress in and Perspectives on Emerging Halide Superionic Conductors for All-Solid-State Batteries, Electrochem. Energy Rev., 2023, 6, 17 CrossRef CAS.
- F. Li, X. Cheng, G. Lu, Y.-C. Yin, Y.-C. Wu, R. Pan, J.-D. Luo, F. Huang, L.-Z. Feng, L.-L. Lu, T. Ma, L. Zheng, S. Jiao, R. Cao, Z.-P. Liu, H. Zhou, X. Tao, C. Shang and H.-B. Yao, Amorphous Chloride Solid Electrolytes with High Li-Ion Conductivity for Stable Cycling of All-Solid-State High-Nickel Cathodes, J. Am. Chem. Soc., 2023, 145, 27774–27787 CrossRef CAS PubMed.
- M. Lei, B. Li, H. Liu and D. E. Jiang, Dynamic Monkey Bar Mechanism of Superionic Li–ion Transport in LiTaCl6, Angew. Chem., Int. Ed., 2023, 63, e202315628 CrossRef PubMed.
- A. Bohnsack, F. Stenzel, A. Zajonc, G. Balzer, M. S. Wickleder and G. Meyer, Ternare chloride der Selten-Erd-Elemente mit lithium, Li3MCl6 (M = Tb-Lu, Y, Sc): Synthese, Kristallstrukturen and Ionenbewegung, Z. Anorg. Allg. Chem., 1997, 623, 1067–1073 CrossRef CAS.
- R. Schlem, T. Bernges, C. Li, M. A. Kraft, N. Minafra and W. G. Zeier, Lattice Dynamical Approach for Finding the Lithium Superionic Conductor Li3ErI6, ACS Appl. Energy Mater., 2020, 3, 3684–3691 CrossRef CAS.
- H. Kwak, D. Han, J. Lyoo, J. Park, S. H. Jung, Y. Han, G. Kwon, H. Kim, S. T. Hong, K. W. Nam and Y. S. Jung, New Cost-Effective Halide Solid Electrolytes for All-Solid-State Batteries: Mechanochemically Prepared Fe3+-Substituted Li2ZrCl6, Adv. Energy Mater., 2021, 11, 2003190 CrossRef CAS.
- G. N. Papatheodorou, Raman spectroscopic studies of yttrium(III) chloride–alkali metal chloride melts and of Cs2NaYCl6 and YCl3 solid compounds, J. Chem. Phys., 1977, 66, 2893–2900 CrossRef CAS.
- J. Liang, X. Li, S. Wang, K. R. Adair, W. Li, Y. Zhao, C. Wang, Y. Hu, L. Zhang, S. Zhao, S. Lu, H. Huang, R. Li, Y. Mo and X. Sun, Site-Occupation-Tuned Superionic LixScCl3 + xHalide Solid Electrolytes for All-Solid-State Batteries, J. Am. Chem. Soc., 2020, 142, 7012–7022 CrossRef CAS PubMed.
- L. Zhou, C. Y. Kwok, A. Shyamsunder, Q. Zhang, X. Wu and L. F. Nazar, A new halospinel superionic conductor for high-voltage all solid state lithium batteries, Energy Environ. Sci., 2020, 13, 2056–2063 RSC.
- C. Cros, L. Hanebali, L. Latié, W. Villeneuve and W. Gang, Structure, ionic motion and conductivity in some solid-solutions of the LiCI-MCI2 systems (M = Mg, V, Mn), Solid State Ionics, 1983, 9, 139–148 CrossRef.
- M. Partik, M. Schneider and H. D. Lutz, Kristallstrukturen von MgCr2O4−Typ Li2VCl4 und Spinell–Typ Li2MgCl4 und Li2CdCl4, Z. Anorg. Allg. Chem., 1994, 620, 791–795 CrossRef CAS.
- X. Li, Y. Xu, C. Zhao, D. Wu, L. Wang, M. Zheng, X. Han, S. Zhang, J. Yue, B. Xiao, W. Xiao, L. Wang, T. Mei, M. Gu, J. Liang and X. Sun, The Universal Super Cation–Conductivity in Multiple–cation Mixed Chloride Solid–State Electrolytes, Angew. Chem., Int. Ed., 2023, 62, e202306433 CrossRef CAS PubMed.
- C. X. Li Feng, L. U. O. Jinda and Y. A. O. Hongbin, Metal chloride solid-state electrolytes and all-solid-state batteries: State-of-the-art developments and perspectives, Energy Storage Sci. Technol., 2024, 13, 193–211 Search PubMed.
- Z. Xu, X. Chen, K. Liu, R. Chen, X. Zeng and H. Zhu, Influence of Anion Charge on Li Ion Diffusion in a New Solid-State Electrolyte, Li3LaI6, Chem. Mater., 2019, 31, 7425–7433 CrossRef CAS.
- A. Bohnsack, G. Balzer, H. U. Güdel, M. S. Wickleder and G. Meyer, Ternäre Halogenide vom Typ A3MX6. VII [1]. Die Bromide Li3MBr6 (M=Sm, Lu, Y): Synthese, Kristallstruktur, Ionenbeweglichkeit, Z. Anorg. Allg. Chem., 1997, 623, 1352–1356 CrossRef CAS.
- R. Schlem, A. Banik, S. Ohno, E. Suard and W. G. Zeier, Insights into the Lithium substructure of the superionic conductors Li3YCl6 and Li3YBr6, Chem. Mater., 2021, 33, 327–337 CrossRef CAS.
- K. Wang, Z. Gu, Z. Xi, L. Hu and C. Ma, Li3TiCl6 as ionic conductive and compressible positive electrode active material for all-solid-state lithium-based batteries, Nat. Commun., 2023, 14, 1396 CrossRef CAS PubMed.
- F. Li, X. Cheng, L.-L. Lu, Y.-C. Yin, J.-D. Luo, G. Lu, Y.-F. Meng, H. Mo, T. Tian, J.-T. Yang, W. Wen, Z.-P. Liu, G. Zhang, C. Shang and H.-B. Yao, Stable All-Solid-State Lithium Metal Batteries Enabled by Machine Learning Simulation Designed Halide Electrolytes, Nano Lett., 2022, 22, 2461–2469 CrossRef CAS PubMed.
- R. A. H. W. Weppner, Ionic conductivity of alkali metal chloroaluminates, Phys. Lett. A, 1976, 58, 245–248 CrossRef.
- W. Weppner and R. A. Huggins, Ionic conductivity of solid and liquid LiAlCl4, J. Electrochem. Soc., 1977, 124, 35–38 CrossRef CAS.
- N. Flores-González, N. Minafra, G. Dewald, H. Reardon, R. I. Smith, S. Adams, W. G. Zeier and D. H. Gregory, Mechanochemical Synthesis and Structure of Lithium Tetrahaloaluminates, LiAlX4 (X = Cl, Br, I): A Family of Li-Ion Conducting Ternary Halides, ACS Mater. Lett., 2021, 3, 652–657 CrossRef PubMed.
- Y. Tomita, A. Fuji-i, H. Ohki, K. Yamada and T. Okuda, New Lithium Ion Conductor Li3InBr6 Studied by 7Li NMR, Chem. Lett., 1998, 27, 223–224 CrossRef.
- K. Yamada, K. Kumano and T. Okuda, Lithium superionic conductors Li3InBr6 and LiInBr4 studied by 7Li, 115In NMR, Solid State Ionics, 2006, 177, 1691–1695 CrossRef CAS.
- K. I. K. Yamada and T. Okuda, Impedance spectroscopy and structural investigation of Li+ conductor Li3InBr6, Solid State Ionics, 2002, 621–628 CAS.
- W. Hönle, B. Hettich and A. Simon, Darstellung und Kristallstrukturen von LiGaCl4 and LiGaI4, Z. Naturforsch B. J. Chem. Sci., 1987, 42, 248–250 CrossRef.
- S.-K. Jung, H. Gwon, G. Yoon, L. J. Miara, V. Lacivita and J.-S. Kim, Pliable Lithium Superionic Conductor for All-Solid-State Batteries, ACS Energy Lett., 2021, 6, 2006–2015 CrossRef CAS.
- H. J. Steiner and H. D. Lutz, Neue schnelle Ionenleiter vom Typ MMIIICl6 (MI = Li, Na, Ag; MIII = In, Y), Z. Anorg. Allg. Chem., 1992, 613, 26–30 CrossRef CAS.
- M. O. Schmidt, M. S. Wickleder and G. Meyer, TernaÈre Halogenide vom Typ A3MX6. VIII Zur Kristallstruktur von Li3InCl6, Z. Anorg. Allg. Chem., 1999, 625, 539–540 CrossRef CAS.
- X. Li, J. Liang, J. Luo, M. N. Banis, C. Wang, W. Li, S. Deng, C. Yu, F. Zhao, Y. Hu, T.-K. Sham, L. Zhang, S. Zhao, S. Lu, H. Huang, R. Li, K. R. Adair and X. Sun, Air-stable Li3InCl6 electrolyte with high voltage compatibility for all-solid-state batteries, Energy Environ. Sci., 2019, 12, 2665–2671 RSC.
- X. Li, J. Liang, N. Chen, J. Luo, K. R. Adair, C. Wang, M. N. Banis, T. K. Sham, L. Zhang, S. Zhao, S. Lu, H. Huang, R. Li and X. Sun, Water-Mediated Synthesis of a Superionic Halide Solid Electrolyte, Angew. Chem., Int. Ed., 2019, 58, 16427–16432 CrossRef CAS PubMed.
- Y. Ishiguro, K. Ueno, S. Nishimura, G. Iida and Y. Igarashib, TaCl5-glassified Ultrafast Lithium Ion-conductive Halide Electrolytes for High-performance All-solid-state Lithium Batteries, Chem. Lett., 2023, 52, 237–241 CrossRef CAS.
- R. Kanno, Y. Takeda and O. Yamamoto, Structure, ionic conductivity and phase transforiviation of double chloride spinels, Solid State Ionics, 1988, 28–30, 1276–1281 CrossRef.
- C. J. J. Van Loon and J. DE Jong, Some chlorides with
the inverse spinel structure: Li2TCl4 (T=Mg,Mn,Fe,Cd), Acta Crystallogr., Sect. B: Struct. Crystallogr. Cryst. Chem., 1975, 31, 2549–2550 CrossRef.
- H. D. Lutz, W. Schmidt and H. Haeuseler, Zur Kenntnis der Chlorid–Spinelle Li2MgCl4, Li2MnCl4, Li2FeCl4, Li2CdCl4, Z. Anorg. Allg. Chem., 1979, 453, 121–126 CrossRef CAS.
- R. Kanno, Y. Takada, K. Takada and O. Yamamoto, Ionic Conductivity and Phase Transition of the Spinel System Li2 − 2x M 1 + x Cl4 (M = Mg, Mn, Cd), J. Electrochem. Soc., 1984, 131, 469–474 CrossRef CAS.
- C. W. R. Nagel, J. Senker and H. D. Lutz,
6Li and 7Li MAS-NMR studies on fast ionic conducting inverse spinel-type Li2 − 2xMg1+x Cl4 and normal spinel-type Li2ZnCl4, Solid State Ionics, 2000, 130, 169–173 CrossRef.
- H. D. Lutz, W. Schmidt and H. Haeuseler, Chloride spinels: a new group of solid lithium electrolytes, J. Phys. Chem. Solids, 1981, 42, 287–289 CrossRef CAS.
- Y. T. R. Kanno and O. Yamamoto, Ionic conductivity of solid lithium ion conductors with the spinel structure: Li2MCl4 (M = Mg, Mn, Fe, Cd), Mater. Res. Bull., 1981, 16, 999–1005 CrossRef.
- C. J. J. Van Loon and D. Visser, Chlorides with the chrysoberyl structure: Na2CoCl4 and Na2ZnCl4, Acta Crystallogr., Sect. B: Struct. Crystallogr. Cryst. Chem., 1977, 33, 188–190 CrossRef.
- M. Sassmannshausen, I. Solinas and H. D. Lutz, Crystal structure of dilithium zinc tetrachloride, Li2ZnCl4 with spinel- and olivine-type structure, Z. Kristallogr. Cryst. Mater., 1996, 211, 819–820 CrossRef CAS.
- A. Vijay and R. D. Eithiraj, Structural, electronic, optical, and thermoelectric properties of Li2ZnCl4 based on density functional theory computations, J. Phys. Chem. Solids, 2022, 171, 111037 CrossRef CAS.
- W. S. P. Kuske and H. D. Lutz, Neutron diffraction studies on spinel type Li2ZnCl4, Mater. Res. Bull., 1988, 23, 1805–1808 CrossRef.
- R. Kanno, Y. Takeda, M. Mort and O. Yamamoto, Ionic conductivity and structure of double chloride Li2ZnCl4 in the Licl-ZnCl2 system, Chem. Lett., 1989, 18, 223–226 CrossRef.
- R. Kanno, K. Takeda and O. Yamamoto, Structure, ionic conductivity and phase transformation of double chloride spinels, Solid State Ionics, 1988, 28–30 Search PubMed.
- H. D. Lutz, Structural Phase Transition and Nonstoichiometry of Li2FeCl4-Neutron Diffraction Studies, J. Solid State Chem., 1993, 107, 245–249 CrossRef CAS.
- H. D. L. M. Schneider, Polymorphie und Pseudosymmetrie von Li2CoCl4, Z. Kristallogr. Cryst. Mater., 1993, 203, 183–197 CrossRef.
- K. Suzuki, X-ray Studies on Precipitation of Metastable Centers in Mixed Crystals NaCl-CdCl2, J. Soc. Jpn., 1961, 16, 67–78 CrossRef CAS.
- H. D. Lutz, Y. KUSK and K. Wussow, Neue Lithiumchlorid-Suzukiphasen: Li6MCI8( M = Fe, Co, N), Z. Anorg. Allg. Chem., 1987, 553, 172–178 CrossRef CAS.
- G. Villeneuve, L. Latié, C. Christian and H. Paul, NMR of 7Li in the paramagnetic “Suzuki-type” phase Li6VCl8, Mater. Res. Bull., 1984, 19, 1515–1526 CrossRef CAS.
- T. M. L. Hanebali, C. Cros and P. Hagenmuller, Sur le systeme LiCl·VCl2, Mater. Res. Bull., 1981, 16, 887–901 CrossRef.
- T. Oi and K. Miyauchi, Amorphous thin film ionic conductors of mLiF.nAlF3, Mater. Res. Bull., 1981, 16, 1281–1289 CrossRef CAS.
- C. H. Wang, J. W. Liang, J. Luo, J. Liu, X. N. Li, F. P. Zhao, R. Y. Li, H. Huang, S. Q. Zhao, L. Zhang, J. T. Wang and X. L. Sun, A universal wet-chemistry synthesis of solid-state halide electrolytes for all-solid-state lithium-metal batteries, Sci. Adv., 2021, 7, eabh1896 CrossRef CAS PubMed.
- X. Wang, E. Zhao and W. Guo, A dual-matrix hybridization strategy for synthesis of cationic-anionic double-doped Li2.99Y0.99Zr0.01Br1.6Cl4.4 halide solid electrolyte, J. Solid State Electrochem., 2023, 27, 3597–3602 CrossRef CAS.
- S. Chen, C. Yu, C. Wei, L. Peng, S. Cheng and J. Xie, Revealing milling durations and sintering temperatures on conductivity and battery performances of Li2.25Zr0.75Fe0.25Cl6 electrolyte, Chin. Chem. Lett., 2023, 34, 107544 CrossRef CAS.
- P. Molaiyan, S. E. Mailhiot, K. Voges, A. M. Kantola, T. Hu, P. Michalowski, A. Kwade, V.-V. Telkki and U. Lassi, Investigation of the structure and ionic conductivity of a Li3InCl6 modified by dry room annealing for solid-state Li-ion battery applications, Mater. Des., 2023, 227, 111690 CrossRef CAS.
- H.-W. Liu, C.-C. Lin, P.-Y. Chang, S.-C. Haw, H.-S. Sheu, J.-M. Chen, C.-C. Chen, R.-J. Jeng and N.-L. Wu, Reducing oxy-contaminations for enhanced Li-ion conductivity of halide-based solid electrolyte in water-mediated synthesis, J. Solid State Electrochem., 2022, 26, 2089–2096 CrossRef CAS.
- X. He, Y. Zhu and Y. Mo, Origin of fast ion diffusion in super-ionic conductors, Nat. Commun., 2017, 8, 15893 CrossRef CAS PubMed.
- S. Wang, Q. Bai, A. M. Nolan, Y. Liu, S. Gong, Q. Sun and Y. Mo, Lithium Chlorides and Bromides as Promising Solid-State Chemistries for Fast Ion Conductors with Good Electrochemical Stability, Angew. Chem., Int. Ed., 2019, 58, 8039–8043 CrossRef CAS PubMed.
- Y. Wang, W. D. Richards, S. P. Ong, L. J. Miara, J. C. Kim, Y. Mo and G. Ceder, Design principles for solid-state lithium superionic conductors, Nat. Mater., 2015, 14, 1026–1031 CrossRef CAS PubMed.
- X. Jia, M. Zhou, R. Zhang and G. Li, Li+ transportation mechanisms in the halide solid-state electrolytes Li3YCl6 and Li3InCl6, Emerging Mater. Res., 2022, 11, 220–227 CrossRef.
- R. Schlem, S. Muy, N. Prinz, A. Banik, Y. Shao-Horn, M. Zobel and W. G. Zeier, Mechanochemical Synthesis: A Tool to Tune Cation Site Disorder and Ionic Transport Properties of Li3MCl6 (M = Y, Er) Superionic Conductors, Adv. Energy Mater., 2019, 10, 1903719 CrossRef.
- J. Qiu, M. Wu, W. Luo, B. Xu, G. Liu and C. Ouyang, Insights into Bulk Properties and Transport Mechanisms in New Ternary Halide Solid Electrolytes: First-Principles Calculations, J. Phys. Chem. C, 2021, 125, 23510–23520 CrossRef CAS.
- B. Ahammed and E. Ertekin, Configurational Disorder, Strong Anharmonicity, and Coupled Host Dynamics Lead to Superionic Transport in Li3YCl6 (LYC), Advanced Materials, 2024, 36, 2310537 CrossRef CAS PubMed.
- L. Hu, J. Zhu, C. Duan, J. Zhu, J. Wang, K. Wang, Z. Gu, Z. Xi, J. Hao, Y. Chen, J. Ma, J.-X. Liu and C. Ma, Revealing the Pnma crystal structure and iontransport mechanism of the Li3YCl6 solid electrolyte, Cell Rep. Phys. Sci., 2023, 4, 101428 CrossRef CAS.
- S. Hyun, H. Chun, M. Hong, J. Kang and B. Han, First-principles study on ultrafast Li-ion diffusion in halospinel Li2Sc2/3Cl4 through multichannels designed by aliovalent doping, J. Mater. Chem. A, 2023, 11, 4272–4279 RSC.
- Y.-C. Yin, J.-T. Yang, J.-D. Luo, G.-X. Lu, Z. Huang, J.-P. Wang, P. Li, F. Li, Y.-C. Wu, T. Tian, Y.-F. Meng, H.-S. Mo, Y.-H. Song, J.-N. Yang, L.-Z. Feng, T. Ma, W. Wen, K. Gong, L.-J. Wang, H.-X. Ju, Y. Xiao, Z. Li, X. Tao and H.-B. Yao, A LaCl3-based lithium superionic conductor compatible with lithium metal, Nature, 2023, 616, 77–83 CrossRef CAS PubMed.
- V. L. Sawankumar, V. Patel, H. Liu, E. Truong, Y. Jin, E. Wang, L. Miara, R. Kim, H. Gwon, R. Zhang, I. Hung, Z. Gan, S.-K. Jung and Y.-Y. Hu, Charge-clustering induced fast ion conduction in 2LiXGaF3: A strategy for electrolyte design, Sci. Adv., 2023, 9, eadj9930 CrossRef PubMed.
- Z. Liu, L. Qin, X. Cao, J. Zhou, A. Pan, G. Fang, S. Wang and S. Liang, Ion migration and defect effect of electrode materials in multivalent-ion batteries, Prog. Mater. Sci., 2022, 125, 100911 CrossRef CAS.
- C. Zhang, H. Zhuang, Z. Qi, X. Liu and Y. Ren, The effect of defects for the ion transport of Li3ScCl6 and Li3InCl6 with the interface of lithium metal anode: A first-principles study, Mater. Today Commun., 2023, 35, 105764 CrossRef CAS.
- E. Kim and Y. Kim, nvestigation of the effect of point defects on the Li-ion conductivity of Li3InCl6, Dalton Trans., 2022, 51, 18159–18168 RSC.
- Z. Liu, S. Ma, J. Liu, S. Xiong, Y. Ma and H. Chen, High Ionic Conductivity Achieved in Li3Y(Br3Cl3) Mixed Halide Solid Electrolyte via Promoted Diffusion Pathways and Enhanced Grain Boundary, ACS Energy Letters, 2020, 6, 298–304 CrossRef.
- E. Sebti, H. A. Evans, H. Chen, P. M. Richardson, K. M. White, R. Giovine, K. P. Koirala, Y. Xu, E. Gonzalez-Correa, C. Wang, C. M. Brown, A. K. Cheetham, P. Canepa and R. J. Clément, Stacking Faults Assist Lithium-Ion Conduction in a Halide-Based Superionic Conductor, J. Am. Chem. Soc., 2022, 144, 5795–5811 CrossRef CAS PubMed.
- P. C. Zhong, S. Gupta, B. W. Deng, K. J. Jun and G. Ceder, The effect of cation-disorder on lithium transport in halide superionic conductors, ACS Energy Letters, 2024, 9, 2775–2781 CrossRef CAS.
- Z. Gao, S. Wang, F. Sun, Z. Yu, H. Song, Z. Liu and H. Chen, Enhanced electrochemical properties of Li2MgCl4 by Zn substitution for all-solid-state batteries, J. Solid State Chem., 2023, 328, 124361 CrossRef CAS.
- R. Li, P. Lu, X. Liang, L. Liu, M. Avdeev, Z. Deng, S. Li, K. Xu, J. Feng, R. Si, F. Wu, Z. Zhang and Y.-S. Hu, Superionic Conductivity Invoked by Enhanced Correlation Migration in Lithium Halides Solid Electrolytes, ACS Energy Letters, 2024, 9, 1043–1052 CrossRef CAS.
- T. Usami, N. Tanibata, H. Takeda and M. Nakayama, Analysis of ion conduction behavior of Nb- and Zr-doped Li3InCl6 -based materials via material simulation, APL Mater., 2023, 11, 121107 CrossRef CAS.
- K.-H. Park, K. Kaup, A. Assoud, Q. Zhang, X. Wu and L. F. Nazar, High-Voltage Superionic Halide Solid Electrolytes for All-Solid-State Li-Ion Batteries, ACS Energy Letters, 2020, 5, 533–539 CrossRef CAS.
- S. Chen, C. Yu, S. Chen, L. Peng, C. Liao, C. Wei, Z. Wu, S. Cheng and J. Xie, Enabling ultrafast lithium-ion conductivity of Li 2 ZrCl 6 by indium doping, Chin. Chem. Lett., 2022, 33, 4635–4639 CrossRef CAS.
- X. Luo, X. Hu, Y. Zhong, X. Wang and J. Tu, Degradation Evolution for Li2ZrCl6 Electrolytes in Humid Air and Enhanced Air Stability via Effective Indium Substitution, Small, 2023, 20, 2306736 CrossRef PubMed.
- K. Wang, Z. Gu, H. Liu, L. Hu, Y. Wu, J. Xu and C. Ma, High–Humidity–Tolerant Chloride Solid–State Electrolyte for All–Solid–State Lithium Batteries, Adv. Sci., 2024, 11, 2305394 CrossRef CAS PubMed.
- Y. Kim and S. Choi, Investigation of the effect of F-doping on the solid-electrolyte property of Li3InCl6, J. Power Sources, 2023, 567, 232962 CrossRef CAS.
- M. Yamagishi, C. Zhong, D. Shibata, M. Morimoto and Y. Orikasa, Effect of Fluorine Substitution in Li3YCl6 Chloride Solid Electrolytes for All-solid-state Battery, Electrochemistry, 2023, 91, 037002–037002 CrossRef CAS.
- Q. Wang, X. Ma, Q. Liu, D. Sun and X. Zhou, Fluorine-doped Li3InCl6 to enhance ionic conductivity and air stability, J. Alloys Compd., 2023, 969, 172479 CrossRef CAS.
- Z. Song, T. Wang, H. Yang, W. H. Kan, Y. Chen, Q. Yu, L. Wang, Y. Zhang, Y. Dai, H. Chen, W. Yin, T. Honda, M. Avdeev, H. Xu, J. Ma, Y. Huang and W. Luo, Promoting high-voltage stability through local lattice distortion of halide solid electrolytes, Nat. Commun., 2024, 15, 1481 CrossRef CAS PubMed.
- Q. Wang, Y. Zhou, X. Wang, H. Guo, S. Gong, Z. Yao, F. Wu, J. Wang, S. Ganapathy, X. Bai, B. Li, C. Zhao, J. Janek and M. Wagemaker, Designing lithium halide solid electrolytes, Nat. Commun., 2024, 15, 1050 CrossRef CAS PubMed.
- A. Emly, E. Kioupakis and A. Van der Ven, Phase Stability and Transport Mechanisms in Antiperovskite Li3OCl and Li3OBr Superionic Conductors, Chem. Mater., 2013, 25, 4663–4670 CrossRef CAS.
- Y. Zhao and L. L. Daemen, Superionic conductivity in lithium-rich anti-perovskites, J. Am. Chem. Soc., 2012, 134, 15042–15047 CrossRef CAS PubMed.
- S. Muy, J. Voss, R. Schlem, R. Koerver, S. J. Sedlmaier, F. Maglia, P. Lamp, W. G. Zeier and Y. Shao-Horn, High-Throughput Screening of Solid-State Li-Ion Conductors Using Lattice-Dynamics Descriptors, iScience, 2019, 16, 270–282 CrossRef CAS PubMed.
- H. Kageyama, K. Hayashi, K. Maeda, J. P. Attfield, Z. Hiroi, J. M. Rondinelli and K. R. Poeppelmeier, Expanding frontiers in materials chemistry and physics with multiple anions, Nat. Commun., 2018, 9, 772 CrossRef PubMed.
- J. Wang, F. Chen, L. Hu and C. Ma, Alternate Crystal Structure Achieving Ionic Conductivity above 1 mS cm−1 in Cost-Effective Zr-Based Chloride Solid Electrolytes, Nano Lett., 2023, 23, 6081–6087 CrossRef CAS PubMed.
- K.-H. Park, S. Y. Kim, M. Jung, S.-B. Lee, M.-J. Kim, I.-J. Yang, J.-H. Hwang, W. Cho, G. Chen, K. Kim and J. Yu, Anion Engineering for Stabilizing Li Interstitial Sites in Halide Solid Electrolytes for All-Solid-State Li Batteries, ACS Appl. Mater. Interfaces, 2023, 15, 58367–58376 CrossRef CAS PubMed.
- B. Li, Y. Li, H.-S. Zhang, T.-T. Wu, S. Guo and A.-M. Cao, Fast Li+-conducting Zr4+-based oxychloride electrolyte with good thermal and solvent stability, Science China Materials, 2023, 66, 3123–3128 CrossRef CAS.
- S. Zhang, F. Zhao, J. Chen, J. Fu, J. Luo, S. H. Alahakoon, L.-Y. Chang, R. Feng, M. Shakouri, J. Liang, Y. Zhao, X. Li, L. He, Y. Huang, T.-K. Sham and X. Sun, A family of oxychloride amorphous solid electrolytes for long-cycling all-solid-state lithium batteries, Nat. Commun., 2023, 14, 3780 CrossRef PubMed.
- S. Zhang, F. Zhao, L.-Y. Chang, Y.-C. Chuang, Z. Zhang, Y. Zhu, X. Hao, J. Fu, J. Chen, J. Luo, M. Li, Y. Gao, Y. Huang, T.-K. Sham, M. D. Gu, Y. Zhang, G. King and X. Sun, Amorphous Oxyhalide Matters for Achieving Lithium Superionic Conduction, J. Am. Chem. Soc., 2024, 146, 2977–2985 CrossRef CAS PubMed.
- L. Hu, J. Wang, K. Wang, Z. Gu, Z. Xi, H. Li, F. Chen, Y. Wang, Z. Li and C. Ma, A cost-effective, ionically conductive and compressible oxychloride solid-state electrolyte for stable all-solid-state lithium-based batteries, Nat. Commun., 2023, 14, 3807 CrossRef CAS PubMed.
- T. Dai, S. Wu, Y. Lu, Y. Yang, Y. Liu, C. Chang, X. Rong, R. Xiao, J. Zhao, Y. Liu, W. Wang, L. Chen and Y.-S. Hu, Inorganic glass electrolytes with polymer-like viscoelasticity, Nat. Energy, 2023, 8, 1221–1228 CrossRef CAS.
|
This journal is © the Partner Organisations 2024 |
Click here to see how this site uses Cookies. View our privacy policy here.