DOI:
10.1039/D4TC00965G
(Paper)
J. Mater. Chem. C, 2024,
12, 8944-8951
Self-powered solar-blind ultraviolet-visible Cu2O/Ga2O3 photodetectors†
Received
11th March 2024
, Accepted 22nd May 2024
First published on 22nd May 2024
Abstract
Traditional optical communication using single narrow-band photodetectors (PDs) has poor confidentiality because all information and data are exposed to free space. With the development of science and technology, even the optical communication in the solar-blind ultraviolet (UV) band carries the risk of eavesdropping. Dual-band PDs have potential applications in secure and reliable optical communication through a combination of optical encryption and algorithmic encryption. In this paper, self-powered Cu2O/Ga2O3 heterojunction PDs with solar-blind UV-visible photodetection were fabricated. By adjusting the pH value of the Cu2O electrolyte, the crystallization quality and grain orientation of the Cu2O thin film were improved, and the interface transfer resistance of the heterojunctions was decreased. The Cu2O/Ga2O3 PDs fabricated in Cu2O electrolyte with a pH value of 9.5 demonstrated the optimized solar-blind UV-visible photoresponse characteristics. In the absence of applied bias, the device exhibited a responsivity of 0.12 mA W−1, a rise time of 2.48 ms and a fall time of 11.72 ms at 254 nm. The responsivity reaches 19 mA W−1 when illuminated at 475 nm, and the rise and fall times are 0.96 and 9.12 ms, respectively. Utilizing the excellent photoresponse characteristics of the solar-blind UV-visible band, the device was used to design and demonstrate a proof-of-concept optical communication system for secure data transmission. The proposed system features two independent light channels, utilizing solar-blind UV light as the information carrier and visible light for key transmission. By implementing specific algorithms, this design ensures safe and reliable communication.
1. Introduction
Single narrow-band photodetectors (PDs) cannot meet the requirements of high-precision target detection and information recognition. The dual-band and multi-band PDs can utilize the difference of photoresponse characteristics in the bands to realize mutual verification and mutual compensation of information, and then improve the accuracy, precision and safety of data. Among the dual-band PDs including visible-near-infrared,1–3 UV-near-infrared,4,5 blue-red,6 near-infrared-short infrared,7 and UV-visible,8–10 UV-visible dual-band PDs are widely used in flame detection, memory storage and secure communication.11–16 Compared with the combination of multi-band PDs and line-array multi-band PDs, the PDs that achieve dual-band or even multi-band detection through a single device have the advantages of simple structure and reduced interference. The earliest multi-band single PDs were fabricated using quantum well materials utilizing the sub-band transition formed in the conduction band of doped quantum wells. Devices such as two-color PDs with a GaAlAs/GaAs quantum well structure have advantages such as flexible wavelength control and a simple preparation method.17 The emerging heterojunction PDs constructed using semiconductors with light absorption in different wavelength bands can realize dual-band or multi-band detection. For example, Wang et al. fabricated PDs based on MoS2/Si heterojunctions demonstrating a photoresponse band from the visible to infrared region.18 He et al. constructed the UV-infrared PDs based on β-Ga2O3/BP heterojunctions.5
The direct ultra-wide bandgap Ga2O3 semiconductor material has a large optical absorption coefficient in the solar-blind UV band, good thermal stability and high breakdown voltage resistance, making it one of the preferred materials for solar-blind UV detectors.19–21 A Ga2O3 thin film is generally polycrystalline. Many grain boundaries in Ga2O3 have a strong scattering and blocking effect on carrier transport, resulting in the decrease of photocurrent and responsivity of Ga2O3 PDs. Some strategies have been proposed to solve this problem from the perspectives of the crystalline quality and the control of crystal plane orientation of Ga2O3. Some studies have reported an improvement in the device performance by preparing ordered Ga2O3 nanostructures. The ordered nanorod arrays (NRs) can serve as trapping structures to enhance the light absorption, form interfaces for the separation of photogenerated charge carriers, and provide directional paths to promote carrier transmission.22–25 In order to achieve the photoresponse characteristics in the solar-blind UV-visible band for Ga2O3 based PDs, Ga2O3 is usually compounded with a semiconductor with strong visible light absorption to construct heterojunction PDs, such as Ga2O3/Au/MAPbBr3 sandwich structure PDs26 and Ga2O3/CuSCN core–shell structure PDs.11 Due to a strong absorption in the visible region, simple preparation and easy control of the crystal phase, Cu2O is suitable to construct the visible light PDs. The composite of Cu2O and Ga2O3 can produce heterojunction PDs with photoresponse characteristics in the solar-blind UV-visible band.
The light absorption coefficient and carriers transport capability of Cu2O films are closely related to crystallization and grain orientation, which are key factors that determine the photoelectric characteristics of the device.27 The electrochemical deposition conditions such as the electrolyte substances, concentration and pH value, deposition temperature, time, and current density or voltage can affect the morphology and crystallization of Cu2O films. Herein, Cu2O thin films were electrochemical deposited on Ga2O3 NRs. The morphology and crystal plane orientation of Cu2O crystals was regulated by controlling the pH value of the electrolyte. The self-powered photoresponse characteristics of Cu2O/Ga2O3 heterojunction PDs in the solar-blind UV-visible band were investigated. The self-powered photoresponse characteristics have been elaborated based on the interface contact and optoelectronic properties of Cu2O/Ga2O3 heterojunctions. The PDs have been designed and demonstrated a proof-of-concept optical encryption communication system for secure data transmission utilizing the solar-blind UV light as an information carrier and visible light for key transmission.
2. Results and discussion
2.1 Morphology, crystal structure and optical properties of Cu2O/Ga2O3 heterojunctions
Fig. 1 shows SEM images of Ga2O3 and Cu2O films deposited on Ga2O3 with electrolyte solution of different pH values. As can be seen from Fig. 1(a), Ga2O3 nanorods grow vertically on the FTO substrate with diamond-shaped end faces, a long diagonal length of 100–200 nm and an average length of about 660 nm. The diamond shaped end face is not detected after Cu2O deposition, and pyramid shaped Cu2O particles form a continuous and dense film.28 EDS results of top view and profile view in Fig. S1 (ESI†) suggested that the elements Ga, O and Cu are uniformly distributed throughout the film, indicating that Cu2O grows on the top and side walls of Ga2O3 nanorods. With the increase of electrolyte pH of Cu2O, the pyramid-shaped particles of Cu2O thin films are transformed from tetrahedron to trihedron. Cu2O(9) and Cu2O(9.5) thin films are quadrangular pyramids, and the distribution of the particle size becomes more uniform with the increased pH value, whereas the particles of Cu2O(10) film demonstrate tetrahedral and trihedral pyramid shapes. The pH value of the deposition solution can affect the growth rate of Cu2O crystals on different crystalline surfaces, and then affects the orientation of the crystal structure of the film and the particle shapes. The oxygen atom density of the Cu2O (111) crystalline surface is greater than that of the (200) crystalline surface. The electrolytes with smaller pH provide fewer oxygen atoms, which facilitates the rapid growth of the (200) crystalline surface with tetrahedral pyramid shapes. With the increase of the electrolyte pH value, the growth rate of the (111) crystalline surface with higher oxygen atom density gradually exceeds that of the (200) crystalline surface.29 Therefore, particles of the Cu2O(10) film show trihedral pyramid shapes and tetrahedral pyramid shapes. The SEM profiles demonstrate that the thickness of the Cu2O/Ga2O3 film increases with the increase of the electrolytic pH of Cu2O. The thickness of the Ga2O3 nanorod film is about 660 nm, and that of the Cu2O/Ga2O3 film is 680, 730 and 810 nm for Cu2O(9)/Ga2O3, Cu2O(9.5)/Ga2O3 and Cu2O(10.5)/Ga2O3, respectively. Increase of electrolytic pH of Cu2O is favorable to accelerate the deposition of Cu2O particles.
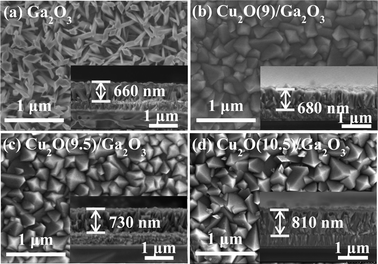 |
| Fig. 1 The top-view and cross-sectional view of SEM images of (a) Ga2O3 NRs and (b) Cu2O(9)/Ga2O3, (c) Cu2O(9.5)/Ga2O3 and (d) Cu2O(10.5)/Ga2O3. | |
Fig. 2(a) shows XRD spectra of Ga2O3 and Cu2O films deposited on Ga2O3 with different pH electrolyte solutions. In the XRD patterns of Ga2O3, the stronger diffraction peak at 36° is attributed to the α-Ga2O3 (110) crystal plane of corundum-type structure. No peaks of the other phase were found except for the diffraction peaks originated from the FTO substrate.30 After the electrochemical deposition of Cu2O thin films on Ga2O3 substrate, Ga2O3 retains a corundum-type structure and presents a (110) crystal plane diffraction peak. The (110), (111), (200) and (311) crystal plane diffraction peaks of Cu2O were observed at 29.6°, 36.5°, 42.4° and 73.6°, while no other phases of Cu and CuxO were found.31,32 With the increase of Cu2O electrolyte pH, the crystal diffraction intensity of the main diffraction peak (200) of Cu2O increases, and the (111) crystal diffraction peak of Cu2O(10) is significantly enhanced compared to the other films, which correlates with the trihedral pyramidal shapes of Cu2O particles.
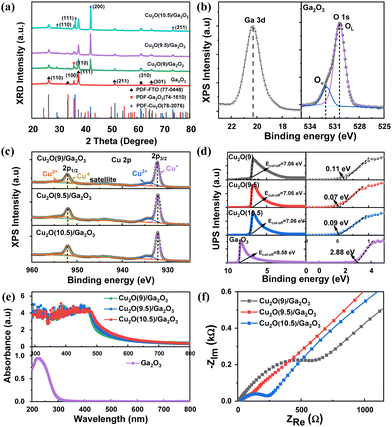 |
| Fig. 2 (a) XRD patterns for Cu2O/Ga2O3 with Cu2O prepared at different pH values. (b) XPS spectra of O 1s and Ga 3d for Ga2O3. (c) XPS spectra of Cu 2p for Cu2O prepared at different pH values. (d) VB spectra and UPS spectra for Ga2O3 and Cu2O prepared at different pH values. (e) UV-vis absorption spectra for Ga2O3 and Cu2O/Ga2O3 with Cu2O prepared at different pH values. (f) EIS curves for Cu2O/Ga2O3 with Cu2O prepared at different pH values. | |
The valence states of the elements in Ga2O3 and Cu2O/Ga2O3 were analysed using XPS spectroscopy. Fig. 2(b) shows the XPS spectra of Ga 3d and O 1s of Ga2O3, and the Ga 3d peak corresponds to a binding energy of 20 eV, which is consistent with the results reported in the literature.33 The peak shape of O1s is asymmetric and can be fitted to two peaks at 530.4 (OL) and 531.9 eV (OV), where OL is related to oxygen ions in the lattice and OV is related to oxygen vacancies.34,35Fig. 2(c) shows the XPS spectra of Cu 2p for Cu2O films deposited on Ga2O3 substrates with different pH electrolytes. Upon fitting, the peaks related to Cu 2p3/2 and Cu 2p1/2 of Cu+ appeared at the binding energies of 932.4 and 952.3 eV, and peaks related to Cu2+ appeared at 934.5 and 954.7 eV, which is due to the oxidation of Cu2O films in air.36 Combined with the Auger spectrum of Cu in Fig. S2 (ESI†), Cu mainly exists in the form of Cu+ in Cu2O films.
The band structure of Ga2O3 and Cu2O electrons was analyzed by using UPS valence band (VB) spectra of Fig. 2(d). Through the linear extrapolation of the cut-off edge of the Au standard sample in the low energy region, it is calculated that the Fermi level (EF) is −7.96 eV under the bias of −10 V. The EF values of Ga2O3 and Cu2O are calculated using the semiconductor work function formula Φ = (Ecut-off − EF) − 21.22, in which the cutoff energy (Ecut-off) can be linearly extrapolated from the cutoff edge of the high energy region. The Ecut-off for Ga2O3 and Cu2O are −8.58 and −7.06 eV, respectively. The calculated EF are −4.69 and −6.2 eV for Ga2O3 and Cu2O, respectively. The energy difference between the EF and the valence band maximum (VBM) of Ga2O3 and Cu2O can be analyzed by the intersection of the tangent line at the maximum of the slope and the line along the outer edge of the baseline, which are 2.88, 0.11, 0.07 and 0.09 eV for Ga2O3, Cu2O(9), Cu2O(9.5) and Cu2O(10), respectively. Combining the optical band gap energies of Ga2O3 (4.61 eV) and Cu2O (2.07 eV) calculated from diffuse reflectance spectroscopy (Fig. S4, ESI†), the position of EF relative to vacuum energy levels can be obtained. The EF position of Ga2O3 is far from the VBM, indicating that Ga2O3 is an n-type semiconductor, whereas the EF position of Cu2O is close to the VBM, suggesting that Cu2O is a p-type semiconductor. The UV-vis absorption spectra of Fig. 2(e) shows that Ga2O3 has a strong optical absorption in the deep UV region less than 250 nm, and the absorption edge is broadened to about 298 nm, which is related to the deep-level oxygen vacancy defect in Ga2O3.37,38 Cu2O/Ga2O3 has strong optical absorption from the solar-blind UV to the blue light region, indicating that the composite material can be used for solar-blind UV-visible light detection.
Electrochemical impedance spectroscopy (EIS) can reflect the characteristics of charge transfer at the interface of semiconductor materials. The EIS curves of Cu2O/Ga2O3 tested at open-circuit voltage and frequency in the range of 0.1–106 Hz are given in Fig. 2(f). The radius of the semicircle in the Nyquist plot is related to the interfacial charge transfer process.39 The size of the radius of the semicircle located in the low-frequency region is related to the charge transfer between Cu2O and the electrolyte, and the radius of the semicircle located in the high-frequency region is related to the charge transfer at the Cu2O/Ga2O3 heterojunction interface. With the increase of Cu2O electrolyte pH, the radius of the Cu2O/Ga2O3 heterojunction semicircle firstly decreases and then increases, indicating the interfacial transfer resistance of Cu2O(9.5)/Ga2O3 is the smallest, which is favorable for the transfer of photogenerated carriers.
2.2 Self-powered photoresponse characteristics of Cu2O/Ga2O3 heterojunction PDs
Fig. 3(a) shows a schematic diagram of the PD structure of Cu2O/Ga2O3 PDs, where Cu2O particles are distributed on the top and sidewalls of Ga2O3 nanorod arrays, and FTO and Au are used as the bottom and top electrodes, respectively. Fig. 3(b)–(d) and Fig. S5 (ESI†) show the I–V curves of Cu2O/Ga2O3 heterojunction PDs prepared by Cu2O electrolyte with different pH in the dark state, solar-blind UV light 254, 265 525, 550 and 660 nm illuminations. All PDs show obvious photosensitive characteristics and have photovoltaic effect under solar-blind UV, UV and visible illuminations, indicating that the PD can realize photodetection in the solar-blind UV-visible region under the condition of self-driving without an external electric field.
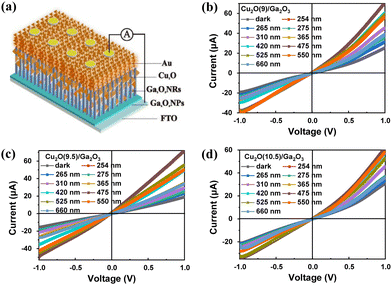 |
| Fig. 3 (a) Schematic diagram of the PD structure of Cu2O/Ga2O3 PDs. Dark-state and different wavelengths light illuminations I–V curves for (b) Cu2O(9)/Ga2O3 PDs, (c) Cu2O(9.5)/Ga2O3 PDs and (d) Cu2O(10.5)/Ga2O3 PDs under an optical power density of 4 mW cm−2 illumination. | |
Fig. 4 shows the I–t curves of the Cu2O/Ga2O3 heterojunction PDs under monochromatic light from solar-blind UV to visible range without external bias. All PDs can demonstrate the self-powered photoresponse in solar-blind UV-visible region with good repeatability and stability under the light switching. The largest photocurrent is obtained under 475 nm light for all the PDs. The Cu2O(9.5)/Ga2O3 PD exhibits an optimized photocurrent under the monochromatic light irradiation compared to other PDs. As revealed from the absorption spectra of Cu2O/Ga2O3 heterojunctions, the photocurrent of the PD in the solar-blind UV band is mainly contributed by photogenerated carriers in Ga2O3 NRs, and that in UV and visible bands is mainly originated from the photogenerated carriers in Cu2O films. The photoresponse time is an important parameter to evaluate the performance of PDs. Fig. S7 (ESI†) shows the photoresponse time of heterojunction PDs with different pH values of Cu2O electrolyte solution under 254 and 475 nm illuminations. The photoresponse time of the PDs is in the order of millisecond. The rise time τrise under illumination is shorter than the decay time τdecay of photocurrent after the light is turned off. The τrise and τdecay of Cu2O(9.5)/Ga2O3 PD are the shortest and the photoresponse speed is the fastest. Table S1 (ESI†) lists the comparison of the photoresponse time of our Cu2O(9.5)/Ga2O3 PD and other Ga2O3 based PDs. The Cu2O/Ga2O3 PD in this work shows a relatively fast photoresponse time.
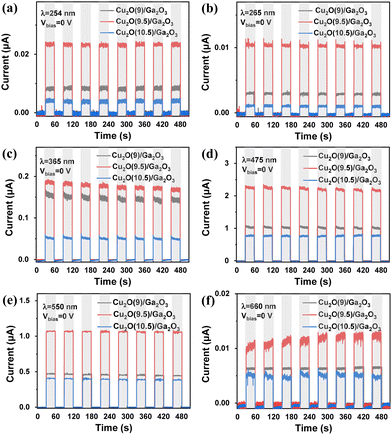 |
| Fig. 4
I–t cycling curves at zero bias for Cu2O/Ga2O3 heterojunction PDs with Cu2O at different pH values under (a) 254, (b) 265, (c) 365, (d) 475, (e) 550 and (f) 660 nm illuminations with P = 4 mW cm−2. | |
Fig. 5(a)–(d) shows the responsivity (R), detectivity (D*), sensitivity (S) and linear dynamic range (LDR) of all PDs under different illumination wavelengths from the solar-blind UV to visible range under self-powered conditions. The PDs have photoresponse characteristics in the illumination range of 254–660 nm. The parameters of the PDs firstly increase and then decrease with the increase of light wavelength, reaching the optimal values at 475 nm. The LDR of Cu2O/Ga2O3 heterojunction PDs is greater than 3 dB in both UV and visible light, which can meet the commercial standard for optical communication.
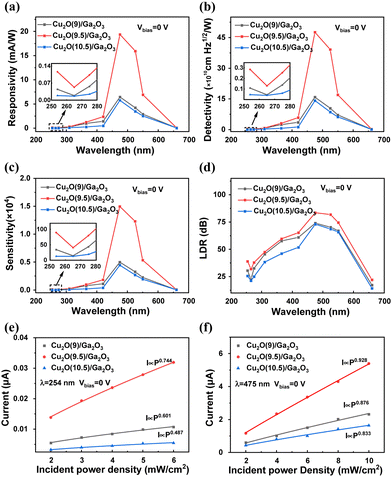 |
| Fig. 5 Performance parameters of (a) R, (b) D*, (c) S and (d) LDR of Cu2O/Ga2O3 heterojunction PDs with Cu2O prepared at different pH values at 0 V bias voltage under solar-blind UV, UV and visible light illuminations with 4 mW cm−2 optical power density. Photocurrent under (e) 254 and (f) 475 nm illuminations of Cu2O/Ga2O3 heterojunction PDs at different optical power densities. | |
Fig. 5(e) and (f) show the curves of the relationship between photocurrent and optical power density of Cu2O/Ga2O3 heterojunction PDs under solar-blind UV 254 nm and visible 475 nm illumination without applied voltage, respectively. The photocurrent of the heterojunction PDs increases with increasing light density, and the heterojunction produce more photogenerated carriers when illuminated by incident light of high optical power density. The nonlinear relationship between the photocurrent (IP) of a PD and the optical power density (P) can be expressed by a power function IP = APθ. A is a constant at a specific wavelength, and θ is related to the kinetic processes of photogenerated carriers including photogenerated carrier production, separation, capture, and complexation. Usually 0 < θ < 1, the closer the θ value is to 1, the more the photogenerated carriers contribute to the photocurrent.40 The Cu2O(9.5)/Ga2O3 PD derives the highest θ values, which are 0.744 and 0.928 at 254 and 475 nm, respectively. The θ values of the Cu2O/Ga2O3 heterojunction PDs during visible light irradiation are larger than those under solar-blind UV illuminations, indicating higher photoelectric conversion efficiency for the heterojunction under the visible light illuminations.41–49
To further analyse the relationship between the self-powered photoresponse characteristics of the PDs and the energy band structure of the heterojunction, the information of the energy band structure based on the XPS, UPS and diffuse reflectance spectroscopy measurements are shown in Fig. 6. Considering that the Fermi level position of Ga2O3 is higher than that of Cu2O, the energy band of Ga2O3 bends upwards and that of Cu2O bends downwards at the interface after the both semiconductors come into contact and reach thermal equilibrium, forming a barrier region of Ga2O3 electrons at the interface. When illuminated at 254 nm, the electrons on the valence band of Ga2O3 and Cu2O are excited to the conduction band. The excited electrons in Ga2O3 need to tunnel through the interface barrier to Cu2O conduction band with the lower energy, and finally are collected by Au electrode. The holes in Cu2O are transferred to the Ga2O3 and then via Ga2O3 to FTO electrode. Considering 254 nm light illumination through Ga2O3 to Cu2O, few photogenerated carriers can be produced in Cu2O. The photocurrent of PDs under solar-blind UV light is mainly contributed by the photogenerated carriers of Ga2O3. When irradiated at 475 nm, Ga2O3 acts as a heterojunction window, and photogenerated carriers in Cu2O contribute to the photocurrent of PDs. Due to the existence of a heterojunction interface barrier, photogenerated electrons of Cu2O are collected by the Au electrode, and photogenerated holes are transferred to the FTO electrode through Ga2O3.
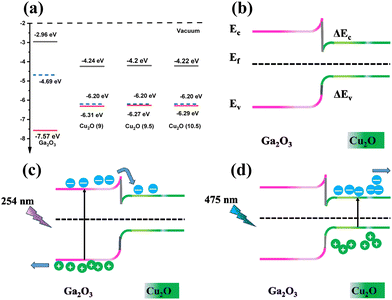 |
| Fig. 6 Energy band structures of Ga2O3 and Cu2O prepared at different pH values (a) before and (b) contact. The schematic diagram of carrier transport of Cu2O/Ga2O3 heterojunction under (c) 254 (d) 475 nm illuminations. | |
Compared with the traditional optical communication system, UV light communication has the unique advantages of high flexibility, low eavesdropping, omni-directional and non-line-of-sight communication, high reliability and good confidentiality, which has wide application prospects in the communication field. With the rapid development of optical communication, there is still a risk of information interception due to the exposure of information data in free space, which poses higher requirements for information security transmission. A proof-of-concept solar-blind UV-visible encrypted communication system is designed based on Cu2O/Ga2O3 heterojunction PDs with the solar-blind UV-visible photoresponse characteristics. The PD can be used as a receiving terminal to decode the information as a binary “1” state for a photocurrent value greater than 0.5 μA and a binary “0” state for a value less than 0.5 μA. Solar-blind UV light is used as a carrier to transmit the encrypted message A, and visible light as a carrier to transmit the key message B with the same number of binary bits. The encryption agreement is defined as follows: when the binary bit in B is “0”, the information corresponding to that of A (“0” or “1”) is false, and its inverse code (“1” or “0”) is output. On the other hand, when the binary bit in B is “1”, the information corresponding to that of A (“0” or “1”) is true, and the original code (“0” or “1”) is output. For example, the solar-blind UV light transmits the encrypted message A as “11011000”, the visible light transmits the same number of binary bit key message B as “01110000”, and the real message decoded by the encryption agreement is “01010111”. The schematic diagram of the calculation process of secure optical communication is shown in Fig. 7. Only PDs with solar-blind UV-visible band photoresponse and agreed-upon encryption algorithms can obtain real information, indicating significant potential for application in safety communication within the realm of secure and efficient data transmission.
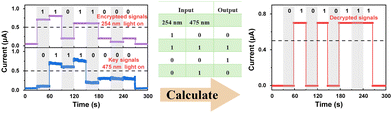 |
| Fig. 7 Schematic diagram of the calculation process of secure optical communication. | |
3. Experimental
3.1 Preparation of Ga2O3 NRs
The Ga2O3 NRs were grown on the seed layer by using a hydrothermal method. Firstly, the Ga2O3 seed layers were prepared by using a sol–gel method on FTO substrates using the precursor solution obtained from 0.3086 g of gallium nitrate hydrate (Aladdin, ≥99%), 12 mL of ethylene glycol methyl ether (Aladdin, ≥99.3%), and 72 μL of ethanolamine (Aladdin, ≥99.5%) stirred in a water bath at 60 °C for 1 h. The as-grown films were annealed for 1 h at 500 °C in air. Secondly, Ga2O3 seed layers were placed in the growth solution of 0.03 M Ga(NO3)3·xH2O, 0.005 M C6H12N4 and 30 mL DI water, and kept for 6 h at 150 °C. Finally, Ga2O3 NRs were obtained by annealing at 500 °C in air for 4 h.
3.2 Preparation of Cu2O/Ga2O3
Cu2O thin films were prepared on Ga2O3 NRs by electrochemical deposition in the solution with different pH values. Firstly, 10 g CuSO4·5H2O (Aladdin, 85–90%) was dissolved in a certain amount of DI water and stirred for 30 min, then 22.4 mL lactic acid was added, and then an appropriate amount of DI water was added to 100 mL. The stirring was continued for 30 min, and the pH value of the solution was adjusted to 9, 9.5 and 10.5 with NaOH to obtain deposition electrolytes. Cu2O thin films were electrochemically deposited at 60 °C in a three-electrode system using Ga2O3 NRs as the working electrode, platinum plated titanium mesh as the counter electrode and Ag/AgCl as the reference electrode. The deposition current was 6 mA and the deposition time was 10 min. According to the different pH values of electrolytes, Cu2O/Ga2O3 samples were named Cu2O(9)/Ga2O3, Cu2O(9.5)/Ga2O3 and Cu2O(10.5)/Ga2O3. Finally, Au electrodes were sputtered on the heterojunction films to construct PDs.
3.3 Characterization and measurements
The surface morphology of the samples was characterized via field emission scanning electron microscopy (SEM, Hitachi S-8010). The crystal structure was studied via X-ray diffractometry (XRD, Rigaku D/max 2500/PC). The elemental composition and chemical state were analyzed through X-ray electron spectroscopy (XPS, Thermo ESCALAB-250), in which the photon energy of the monochromatic source (Al target) was 1846 eV. The band structure of the samples was tested by using He–I (hν = 21.22 eV) UV photoelectron spectroscopy (UPS). The absorption and diffuse reflectance spectra of Cu2O thin films and Ga2O3 NRs were measured using a Hitachi V4100 and a Shimadzu UV-3600i Plus UV-visible spectrophotometer, respectively. Electrochemical impedance spectroscopy (EIS) data were obtained in 1 M Na2SO4 solution with an electrochemical workstation (Autolab PGSTAT 128N) using a three-electrode system with the sample as the working electrode, a platinum wire as the counter electrode, and Ag/AgCl as the reference electrode. The current–voltage (I–V) and current–time (I–t) curves in darkness and illumination were measured using a Keithley 2450 source meter and a light emitting diode (LED). The photoresponse time was obtained using an oscilloscope (Tektronix MDO3014).
4. Conclusions
In summary, Cu2O/Ga2O3 heterojunction PDs with a self-powered solar-blind UV-visible photoresponse have been constructed. By adjusting the pH value of the Cu2O electrolyte, the crystallization quality and grain orientation of Cu2O(9.5) films were improved, and the interface transfer resistance of Cu2O(9.5)/Ga2O3 heterojunction was reduced. The optimized solar-blind UV-visible photoresponse characteristics without the external bias were achieved in Cu2O(9.5)/Ga2O3 PDs, demonstrating a responsivity of 0.12 mA W−1, rise/decay time of 2.48/11.72 ms under 254 nm illumination, and responsivity of 19 mA W−1, the rise/decay time of 0.96/9.12 ms under 475 nm illumination. A secure solar-blind UV-visible optical communication system with two independent optical channels is proposed by using the photoresponse characteristics of solar-blind UV-visible band PDs, in which solar-blind UV is used as the information carrier and visible light as the key. Information can be transmitted through an encryption algorithm via secure and reliable communication. This method has a good application prospects in the field of secure communication.
Author contributions
Xiaodan Wang: conceptualization, data curation, formal analysis, writing – original draft. Jianping Xu: conceptualization, formal analysis, resources, funding acquisition. Shaobo Shi: formal analysis. Lina Kong: formal analysis. Xiangwei He: formal analysis. Jiahang He: formal analysis. Xiaosong Zhang: formal analysis. Lan Li: formal analysis, resources, project administration, supervision.
Conflicts of interest
The authors declare that they have no known competing financial interests or personal relationships that could have appeared to influence the work reported in this paper.
Acknowledgements
This work was supported by the National Natural Science Foundation of China (grant numbers 52271187, 62375205, 51971158 and 51871167); the Natural Science Foundation of Tianjin (grant number 18JCYBJC86200); and the Scientific Developing Foundation of Tianjin Education Commission (grant number 2018ZD09).
References
- M. Dai, H. Chen, R. Feng, W. Feng, Y. Hu, H. Yang, G. Liu, X. Chen, J. Zhang, C.-Y. Xu and P. Hu, A Dual-Band Multilayer InSe Self-Powered Photodetector with High Performance Induced by Surface Plasmon Resonance and Asymmetric Schottky Junction, ACS Nano, 2018, 12, 8739–8747 CrossRef CAS PubMed.
- P. Wang, S. Liu, W. Luo, H. Fang, F. Gong, N. Guo, Z. G. Chen, J. Zou, Y. Huang, X. Zhou, J. Wang, X. Chen, W. Lu, F. Xiu and W. Hu, Arrayed van der Waals Broadband Detectors for Dual-Band Detection, Adv. Mater., 2017, 29, 1604439 CrossRef PubMed.
- Y. Gao, C. Zhao, K. Pu, M. He, W. Cai, M.-C. Tang, F. Kang, H.-L. Yip and G. Wei, Low-voltage-modulated Perovskite/organic Dual-band Photodetectors for Visible and Near-infrared Imaging, Sci. Bull., 2022, 67, 1982–1990 CrossRef CAS PubMed.
- S. Chen, C. Teng, M. Zhang, Y. Li, D. Xie and G. Shi, A Flexible UV-Vis-NIR Photodetector based on a Perovskite/Conjugated-Polymer Composite, Adv. Mater., 2016, 28, 5969–5974 CrossRef CAS PubMed.
- T. He, C. Li, X. Zhang, Y. Ma, X. Cao, X. Shi, C. Sun, J. Li, L. Song, C. Zeng, K. Zhang, X. Zhang and B. Zhang, Metalorganic Chemical Vapor Deposition Heteroepitaxial β-Ga2O3 and Black Phosphorus Pn Heterojunction for Solar-Blind Ultraviolet and Infrared Dual-Band Photodetector, Phys. Status Solidi A, 2019, 217, 1900861 CrossRef.
- B. Huang, J. Liu, Z. Han, Y. Gu, D. Yu, X. Xu and Y. Zou, High-Performance Perovskite Dual-Band Photodetectors for Potential Applications in Visible Light Communication, ACS Appl. Mater. Interfaces, 2020, 12, 48765–48772 CrossRef CAS PubMed.
- C. h Xu, S. H. Luo, Y. Wang, X. F. Shi, C. Fu, J. Wang, C. Y. Wu and L. B. Luo, Bias-Selectable Si Nanowires/PbS Nanocrystalline Film n–n Heterojunction for NIR/SWIR Dual-Band Photodetection, Adv. Funct. Mater., 2023, 33, 2214996 CrossRef CAS.
- F. Cao, Z. Q. Li, X. Y. Liu, Z. F. Shi and X. S. Fang, Air Induced Formation of CsBiBr/CsBiBr Bulk Heterojunction and Its Dual-band Photodetection Abilities for Light Communication, Adv. Funct. Mater., 2022, 32, 2206151 CrossRef CAS.
- M.-M. Fan, K.-L. Xu, X.-Y. Li, G.-H. He and L. Cao, Self-powered Solar-blind UV/visible Dual-band Photodetection based on a Solid-state PEDOT:PSS/α-Ga2O3 Nanorod Array/FTO Photodetector, J. Mater. Chem. C, 2021, 9, 16459–16467 RSC.
- T. T. Yan, Z. Q. Li, L. Su, L. M. Wu and X. S. Fang, Bidirectional and Dual-Mode Organic Photodetector Enables Secure Ultraviolet Communication, Adv. Funct. Mater., 2023, 33, 2302746 CrossRef CAS.
- S. Li, D. Guo, P. Li, X. Wang, Y. Wang, Z. Yan, Z. Liu, Y. Zhi, Y. Huang, Z. Wu and W. Tang, Superhigh Signal-to-Noise Ratio, Self-Powered Solar-blind Photodetector Based on n-Ga2O3/p-CuSCN Core–Shell Microwire Heterojunction, ACS Appl. Mater. Interfaces, 2019, 11, 35105–35114 CrossRef CAS PubMed.
- M. S. Long, P. Wang, H. H. Fang and W. D. Hu, Progress, Challenges, and Opportunities for 2D Material Based Photodetectors, Adv. Funct. Mater., 2019, 29, 1803807 CrossRef.
- X. Yu, Y. Li, X. Hu, D. Zhang, Y. Tao, Z. Liu, Y. He, M. A. Haque, Z. Liu, T. Wu and Q. J. Wang, Narrow Bandgap Oxide Nanoparticles Coupled with Graphene for High Performance Mid-infrared Photodetection, Nat. Commun., 2018, 9, 4299 CrossRef PubMed.
- W. X. Ouyang, F. Teng, J. H. He and X. S. Fang, Enhancing the Photoelectric Performance of Photodetectors Based on Metal Oxide Semiconductors by Charge-Carrier Engineering, Adv. Funct. Mater., 2019, 29, 1807672 CrossRef.
- M. Anaya, J. P. Correa-Baena, G. Lozano, M. Saliba, P. Anguita, B. Roose, A. Abate, U. Steiner, M. Grätzel, M. E. Calvo, A. Hagfeldt and H. Míguez, Optical Analysis of CH3NH3SnxPb1−xI3 Absorbers: A Roadmap for Perovskite-on-perovskite Tandem Solar cells, J. Mater. Chem. A, 2016, 4, 11214–11221 RSC.
- Z. Yang, A. Rajagopal, C. C. Chueh, S. B. Jo, B. Liu, T. Zhao and A. K. Jen, Stable Low-Bandgap Pb-Sn Binary Perovskites for Tandem Solar Cells, Adv. Mater., 2016, 28, 8990–8997 CrossRef CAS PubMed.
- Y. Zhang, D. S. Jiang, J. B. Xia, L. Q. Cui, C. Y. Song, Z. Q. Zhou and W. K. Ge, A Voltage-controlled Tunable Two-color Infrared Photodetector using GaAs/AlAs/GaAlAs and GaAs/GaAlAs Stacked Multiquantum Wells, Appl. Phys. Lett., 1996, 68, 2114–2116 CrossRef CAS.
- L. Wang, J. Jie, Z. Shao, Q. Zhang, X. Zhang, Y. Wang, Z. Sun and S. T. Lee, MoS2/Si Heterojunction with Vertically Standing Layered Structure for Ultrafast, High-Detectivity, Self-Driven Visible–Near Infrared Photodetectors, Adv. Funct. Mater., 2015, 25, 2910–2919 CrossRef CAS.
- D. Y. Guo, H. Z. Shi, Y. P. Qian, M. Lv, P. G. Li, Y. L. Su, Q. Liu, K. Chen, S. L. Wang, C. Cui, C. R. Li and W. H. Tang, Fabrication of β-Ga2O3/ZnO Heterojunction for Solar-blind Deep Ultraviolet Photodetection, Semicond. Sci. Technol., 2017, 32, 03LT01 CrossRef.
-
A. Hierro, G. Tabares, M. Lopez-Ponce, J. M. J. M. Ulloa, A. Kurtz, E. Muñoz, V. Marín-Borrás, V. Muñoz-Sanjosé and J.-M. Chauveau, ZnMgO-based UV Photodiodes: a Comparison of Films Grown by Spray Pyrolysis and MBE, San Francisco, CA, February, 2016 Search PubMed.
- T. Onuma, S. Saito, K. Sasaki, T. Masui, T. Yamaguchi, T. Honda and M. Higashiwaki, Valence Band Ordering in β-Ga2O3 Studied by Polarized Transmittance and Reflectance Spectroscopy, Jpn. J. Appl. Phys., 2015, 54, 112601 CrossRef.
- Q.-M. Fu, D.-C. He, Z.-C. Yao, J.-L. Peng, H.-Y. Zhao, H. Tao, Z. Chen, Y.-F. Tu, Y. Tian, D. Zhou, G. Zheng and Z.-B. Ma, Self-powered Ultraviolet Photodetector based on ZnO Nanorod Arrays Decorated with Sea Anemone-like CuO Nanostructures, Mater. Lett., 2018, 222, 74–77 CrossRef CAS.
- D. Chen, L. Wei, L. Meng, D. Wang, Y. Chen, Y. Tian, S. Yan, L. Mei and J. Jiao, High-Performance Self-Powered UV Detector based on SnO2-TiO2 Nanomace Arrays, Nanoscale Res. Lett., 2018, 13, 92 CrossRef PubMed.
- Y. Zhang, J. Xu, S. Shi, Y. Gao, C. Wang, X. Zhang, S. Yin and L. Li, Development of Solution-Processed ZnO Nanorod Arrays Based Photodetectors and the Improvement of UV Photoresponse via AZO Seed Layers, ACS Appl. Mater. Interfaces, 2016, 8, 22647–22657 CrossRef CAS PubMed.
- Z. Xi, Z. Liu, L. Yang, K. Tang, L. Li, G. Shen, M. Zhang, S. Li, Y. Guo and W. Tang, Comprehensive Study on Ultra-Wide Band Gap La2O3/ε-Ga2O3 p–n Heterojunction Self-Powered Deep-UV Photodiodes for Flame Sensing, ACS Appl. Mater. Interfaces, 2023, 15, 40744–40752 CrossRef CAS PubMed.
- W. Gong, J. Yan, F. Gao, S. Ding, G. He and L. Li, High-Performance UV–Vis Broad-Spectra Photodetector Based on a β-Ga2O3/Au/MAPbBr3 Sandwich Structure, ACS Appl. Mater. Interfaces, 2022, 14, 47853–47862 CrossRef CAS PubMed.
- S. K. Baek, Y. H. Kwon, J. H. Shin, H. S. Lee and H. K. Cho, Low-Temperature Processable High-Performance Electrochemically Deposited p-Type Cuprous Oxides Achieved by Incorporating a Small Amount of Antimony, Adv. Funct. Mater., 2015, 25, 5214–5221 CrossRef CAS.
- L. L. Wu, L. K. Tsui, N. Swami and G. Zangari, Photoelectrochemical Stability of Electrodeposited CuO Films, J. Phys. Chem. C, 2010, 114, 11551–11556 CrossRef CAS.
- Y. Zhou and J. A. Switzer, Electrochemical Deposition and Microstructure of Copper(I) Oxide Films, Scr. Mater., 1998, 38, 1731–1738 CrossRef CAS.
- T. Kobayashi, T. Gake, Y. Kumagai, F. Oba and Y.-I. Matsushita, Energetics and Electronic Structure of Native Point Defects in α-Ga2O3, Appl. Phys. Express, 2019, 12, 091001 CrossRef CAS.
- P. Grez, F. Herrera, G. Riveros, A. Ramírez, R. Henríquez, E. Dalchiele and R. Schrebler, Morphological, Structural, and Photoelectrochemical Characterization of n-type CuO Thin Films Obtained by Electrodeposition, Phys. Status Solidi A, 2012, 209, 2470–2475 CrossRef CAS.
- Y.-C. Chen, Y.-J. Chen, P.-H. Dong and Y.-K. Hsu, Benchmarked Photoelectrochemical Water Splitting by Nickel-Doped n-Type Cuprous Oxide, ACS Appl. Energy Mater., 2020, 3, 1373–1380 CrossRef CAS.
- Y. Wang, N. Li, P. Duan, X. Sun, B. Chu and Q. He, Properties and Photocatalytic Activity of β-Ga2O3 Nanorods under Simulated Solar Irradiation, J. Nanomater., 2015, 2015, 191793 Search PubMed.
- D. Y. Guo, Z. P. Wu, Y. H. An, P. G. Li, P. C. Wang, X. L. Chu, X. C. Guo, Y. S. Zhi, M. Lei, L. H. Li and W. H. Tang, Unipolar Resistive Switching Behavior of Amorphous Gallium Oxide Thin Films for Nonvolatile Memory Applications, Appl. Phys. Lett., 2015, 106, 042105 CrossRef.
- D. Y. Guo, Z. P. Wu, Y. H. An, X. C. Guo, X. L. Chu, C. L. Sun, L. H. Li, P. G. Li and W. H. Tang, Oxygen Vacancy Tuned Ohmic-Schottky Conversion for Enhanced Performance in β-Ga2O3 Solar-blind Ultraviolet Photodetectors, Appl. Phys. Lett., 2014, 105, 023507 CrossRef.
- Y. Liu, J. Zhu, L. Cai, Z. Yao, C. Duan, Z. Zhao, C. Zhao and W. Mai, Solution-Processed High-Quality Cu2O Thin Films as Hole Transport Layers for Pushing the Conversion Efficiency Limit of Cu2O/Si Heterojunction Solar Cells, Sol. RRL, 2019, 4, 1900339 CrossRef.
- D.-W. Jeon, H. Son, J. Hwang, A. Y. Polyakov, N. B. Smirnov, I. V. Shchemerov, A. V. Chernykh, A. I. Kochkova, S. J. Pearton and I.-H. Lee, Electrical Properties, Structural Properties, and Deep Trap Spectra of Thin α-Ga2O3 Films Grown by Halide Vapor Phase Epitaxy on Basal Plane Sapphire Substrates, APL Mater., 2018, 6, 121110 CrossRef.
- T. Zhang, J. Lin, X. Zhang, Y. Huang, X. Xu, Y. Xue, J. Zou and C. Tang, Single-crystalline Spherical β-Ga2O3 Particles: Synthesis, N-doping and Photoluminescence Properties, J. Lumin., 2013, 140, 30–37 CrossRef CAS.
- X. Ren, P. Gao, X. Kong, R. Jiang, P. Yang, Y. Chen, Q. Chi and B. Li, NiO/Ni/TiO2 Nanocables with Schottky/p-n Heterojunctions and the Improved Photocatalytic Performance in Water Splitting under Visible Light, J. Colloid Interface Sci., 2018, 530, 1–8 CrossRef CAS PubMed.
- Z. Long, X. Xu, W. Yang, M. Hu, D. V. Shtansky, D. Golberg and X. Fang, Cross-Bar SnO2-NiO Nanofiber-Array-Based Transparent Photodetectors with High Detectivity, Adv. Electron. Mater., 2019, 6, 1901048 CrossRef.
- P. Li, H. Shi, K. Chen, D. Guo, W. Cui, Y. Zhi, S. Wang, Z. Wu, Z. Chen and W. Tang, Construction of GaN/Ga2O3 p-n Junction for an Extremely High Responsivity Self-powered UV Photodetector, J. Mater. Chem. C, 2017, 5, 10562–10570 RSC.
- Y. Wang, C. Wu, D. Guo, P. Li, S. Wang, A. Liu, C. Li, F. Wu and W. Tang, All-Oxide NiO/Ga2O3 p–n Junction for Self-Powered UV Photodetector, ACS Appl. Electron. Mater., 2020, 2, 2032–2038 CrossRef CAS.
- Z. Yan, S. Li, Z. Liu, Y. Zhi, J. Dai, X. Sun, S. Sun, D. Guo, X. Wang, P. Li, Z. Wu, L. Li and W. Tang, High Sensitivity and Fast Response Self-powered Solar-blind Ultraviolet Photodetector with a β-Ga2O3/spiro-MeOTAD p–n Heterojunction, J. Mater. Chem. C, 2020, 8, 4502–4509 RSC.
- S. Li, Z. Yan, Z. Liu, J. Chen, Y. Zhi, D. Guo, P. Li, Z. Wu and W. Tang, A Self-powered Solar-blind Photodetector with Large Voc Eenhancing Performance Based on the PEDOT:PSS/Ga2O3 Organic–inorganic Hybrid Heterojunction, J. Mater. Chem. C, 2020, 8, 1292–1300 RSC.
- C. Wu, F. Wu, L. Deng, S. Li, S. Wang, L. Cheng, A. Liu, J. Wang, W. Tang and D. Guo, Solution-processed Y-doped SnSrO3 Electron Transport Layer for Ga2O3 Based Heterojunction Solar-blind Photodetector with High Sensitivity, Vacuum, 2022, 201, 111064 CrossRef CAS.
- K. Arora, N. Goel, M. Kumar and M. Kumar, Ultrahigh Performance of Self-Powered β-Ga2O3 Thin Film Solar-Blind Photodetector Grown on Cost-Effective Si Substrate Using High-Temperature Seed Layer, ACS Photonics, 2018, 5, 2391–2401 CrossRef CAS.
- L. Wu, X. Jiao, D. Wang, W. Guo, L. Li, F. Li and W. Tang, A Self-powered Deep-ultraviolet Photodetector Based on an Epitaxial Ga2O3/Ga:ZnO Heterojunction, J. Mater. Chem. C, 2017, 5, 8688–8693 RSC.
- J. Yu, M. Yu, Z. Wang, L. Yuan, Y. Huang, L. Zhang, Y. Zhang and R. Jia, Improved Photoresponse Performance of Self-Powered β-Ga2O3/NiO Heterojunction UV Photodetector by Surface Plasmonic Effect of Pt Nanoparticles, IEEE Trans. Electron. Devices, 2020, 67, 3199–3204 CAS.
- D. Guo, H. Liu, P. Li, Z. Wu, S. Wang, C. Cui, C. Li and W. Tang, Zero-Power-Consumption Solar-Blind Photodetector Based on β-Ga2O3/NSTO Heterojunction, ACS Appl. Mater. Interfaces, 2017, 9, 1619–1628 CrossRef CAS PubMed.
|
This journal is © The Royal Society of Chemistry 2024 |
Click here to see how this site uses Cookies. View our privacy policy here.