DOI:
10.1039/D4EN00739E
(Communication)
Environ. Sci.: Nano, 2025,
12, 232-240
Weathering pathways differentially affect colloidal stability of nanoplastics†
Received
13th August 2024
, Accepted 20th October 2024
First published on 21st October 2024
Abstract
Aggregation is the most fundamental process affecting the fate, transport, and risks of nanoplastics in aquatic environments. Weathering of nanoplastics alters their physiochemical properties and, consequently, aggregation behavior. Herein, we show that two weathering pathways, namely, UV irradiation (the primary aging pathway in surface water) and sulfide-induced transformation (a common process in anoxic environments), affect the aggregation and colloidal stability of polystyrene (PS) nanoplastics differentially. Compared to sulfide-induced aging, UV-induced aging introduced more oxygen-containing functional groups on the nanoplastic surface, although significant amounts of O-functional groups were formed during sulfide-induced aging, owing to hydroxyl radicals formed through the spontaneous oxidation of sulfides. Accordingly, UV-aged PS nanoplastics (PS-UV) exhibited higher stability than sulfide-aged PS nanoplastics (PS-S) in a monovalent cation-dominated solution because of enhanced electrostatic repulsion and weakened van der Waals attraction. However, the stability of PS-UV was lower than that of PS-S in a divalent salt solution considering the bridging effects of divalent ions. These results underline the importance of comprehending the effects of diverse environmental weathering processes on nanoplastics' behaviors, particularly those that readily occur in anoxic environments but are insufficiently investigated.
Environmental significance
Nanoplastics are an emerging contaminant of significant ecological and human health concerns owing to their small size, ubiquitous distribution, and persistence in the environment. The colloidal stability of nanoplastics determine their size as well as their fate and transport in aquatic systems. Environmental weathering processes can substantially alter the surface physicochemical properties of nanoplastics, influencing their stability. Therefore, understanding the key factors and mechanisms controlling the stability of nanoplastics in various environmental contexts is essential for accurate risk assessment. This study demonstrates that UV-induced and sulfide-induced aging significantly enhance the stability of nanoplastics, with the extent of this enhancement varying based on solution chemistry conditions. The results of this study may have important implications for assessing the environmental fate and transport of other particulate contaminants.
|
1. Introduction
Micro-/nanoplastics (MNPs) are ubiquitously detected in the environment, even in the most remote places on the earth where no plastic is produced or used because of its high transport ability.1,2 MNPs have been recognized as an emerging global threat because of their persistent and potential health impacts on aquatic and terrestrial organisms.3,4 Because of their small size, nanoplastics are prone to be taken up or ingested by microorganisms or zooplankton and are accumulated in organisms at higher trophic levels, which may induce various toxicological effects, including oxidative stress, energy and metabolic disruption, and immune dysfunction.5–7 Besides, nanoplastics can serve as a carrier of contaminants on account of their large surface area, facilitating the spread of coexisting contaminants.2,8 Notably, the fate and transport of nanoplastics in the environment are significantly influenced by their colloidal stability.9,10 Understanding the factors that govern the colloidal stability of nanoplastics is crucial for predicting their environmental behavior and potential impacts.
The colloidal stability of nanoplastics is governed by their surface physicochemical properties (e.g., surface charge, coating, and morphology). In particular, surface functional groups can dictate the stability of nanoplastics by controlling surface charges in response to varying water chemistry conditions, including the presence of electrolytes, natural organic matter, pH variations, and coexisting particles (e.g., clay and iron oxides).11,12 For instance, nanoplastics with abundant carboxyl or sulfate groups remain highly dispersed in the aqueous solution of hundreds of millimolar of NaCl.13 Nanoplastics undergo aging via mechanical breakdown, photochemical degradation (UV irradiation), heating, and chemical oxidation (e.g., with ozone) in real environments. These weathering processes cause significant alterations to physicochemical properties of nanoplastics, such as surface functional groups, surface charge, surface roughness, and size distribution,14,15 ultimately influencing the colloidal stability of nanoplastics.16,17
UV irradiation-induced aging is a common weathering process for nanoplastics in surface waters, primarily causing surface oxidation.18,19 In contrast, sulfide-induced aging, prevalent in oxygen-deficient and sulfide-rich environments such as sediments and wastewater treatment plants, alters nanoplastics through radical oxidation and sulfide addition.20–22 These two aging processes cause distinctly different alterations in the physicochemical properties of nanoplastics,20,23 which may subsequently influence their colloidal stability in different ways. While the effects of UV-induced aging on the colloidal stability of nanoplastics have been extensively studied,16,24,25 the impact of sulfide-induced aging remains largely unexplored. The specific mechanisms via which surface chemical alterations induced by sulfide-aging affect the colloidal stability of nanoplastics are still unclear. These uncertainties hinder a comprehensive understanding of the behavior of nanoplastics in real environments and accurate assessments of their environmental risks.
In this study, we compared the colloidal stability of PS nanoplastics as affected by sulfide-induced vs. UV irradiation-induced weathering. The physicochemical properties of pristine PS, UV-treated PS (PS-UV), and sulfide-treated PS (PS-S) were characterized, and their aggregation kinetics in aqueous matrices were compared against various metal cation concentrations and pH conditions, as well as in the presence of natural organic matter (NOM).
2. Results and discussion
2.1 Characterization of PS nanoplastics
The physicochemical properties of pristine and aged PS nanoplastics were first compared, and the details of the experiments are described in the ESI.† The weathering processes applied in this study did not alter the morphology or induce significant fragmentation of the nanoplastics. However, these processes did result in differential changes in the surface elemental composition of the nanoplastics. SEM and TEM images (Fig. 1 and S1†) demonstrate that all three types of PS nanoplastics exhibit a smooth surface morphology without cracks or fragments, although the particle size slightly decreased following aging treatments. As illustrated in Fig. 2, the aging processes significantly altered the surface functional groups and the surface elemental composition of the PS nanoplastics. Specifically, the FTIR spectra (Fig. 2a) confirmed the characteristic peaks of PS nanoplastics, including the stretching vibration of O–H at 3437 cm−1, stretching vibration of aromatic C–H at 3068 and 3028 cm−1, stretching vibration of C–H2 in the main chain at 2920 and 2852 cm−1, stretching vibration of aromatic C
C at 1600, 1492, and 1450 cm−1, and bending vibration of aromatic C–H at 754 and 698 cm−1.26–28 The presence of these characteristic peaks indicate that PS remains the primary molecular structure during the aging processes. The broad adsorption peaks in the range of 1680–1830 cm−1 (e.g., 1728 cm−1 attributed to C
O stretching) suggest the oxygen-containing groups of PS nanoplastics.29 Notably, the characteristic peak at 1635 cm−1 was attributed to a small amount of residual aliphatic C
C, which likely originates from the synthesis process of polystyrene.30,31 The disappearance of the peak at 1635 cm−1 for PS-S may be due to the oxidative cleavage of the carbon–carbon double bond via the Michael addition reaction caused by sulfide or polysulfides.20
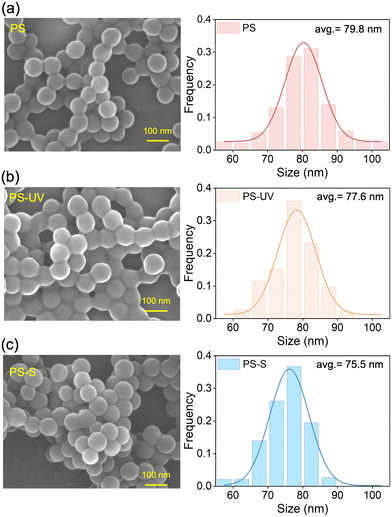 |
| Fig. 1 Characterization of physicochemical properties of nanoplastics. SEM images and size distribution of pristine PS (a), PS-UV (b), and PS-S (c) nanoplastics. | |
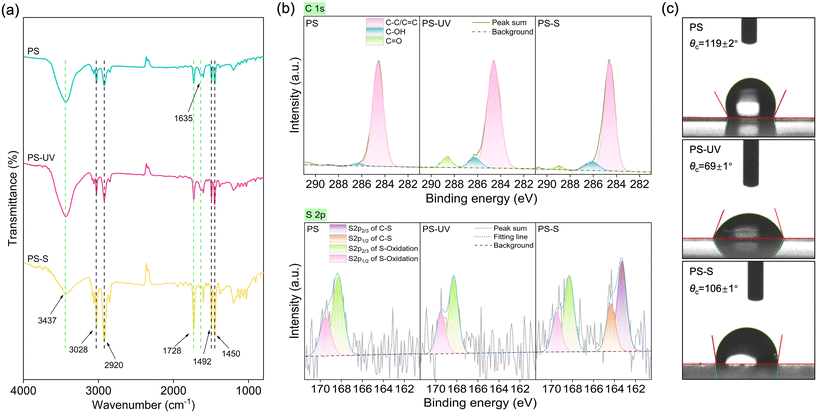 |
| Fig. 2 Characterization of physicochemical properties of nanoplastics. FTIR spectra (a), XPS spectra of C 1s and S 2p (b), and water contact angle (c) of PS, PS-UV, and PS-S nanoplastics. | |
The XPS spectroscopy was further applied to investigate the changes in the chemical compositions of PS during aging processes (Fig. 2b). The increased surface O/C ratio on PS nanoplastics upon aging indicated that PS nanoplastics were oxidized by either UV- or sulfide-treatment (Table S1†). As shown in the deconvolution of the high-resolution C 1s XPS peak (i.e., C–OH at 286.6 eV and O–C
O at 288.8 eV), the aging processes also lead to changes in the distribution of different surface oxygen-functional groups, with a relative abundance following the order of PS-UV > PS-S > PS. Specifically, the C–O and C
O contents accounted for 2.1% and 0% of the surface C for pristine PS nanoplastics, respectively, while those values increased to 7.45% and 6.29% upon UV treatment and 7.80% and 1.9% upon sulfide treatment. (Table S1†). Additionally, two peaks at 164 and 165.2 eV in XPS S 2p spectra of PS-S (Fig. 2b) can be assigned to the C–S species of alkyl sulfides,32 revealing the formation of C–S bonds upon sulfide treatment. Note that the peaks at 168.3 and 169.4 eV for all three types of PS nanoplastics are ascribed to sulfoxides, sulfones or sulfate groups, which may result from the residual sodium dodecyl sulfate or sulfate groups of the initiators used in the polymerization of styrene.12,33 The changes in the distribution of the surface oxygen-containing functional groups and surface elements discussed above also induce alterations in the hydrophobicity and surface charge of PS nanoplastics. After aging treatments, the water contact angle value of nanoplastics decreased (Fig. 2c), indicating the surface of nanoplastics became more hydrophilic. Notably, UV treatment reduced the hydrophobicity of PS nanoplastics more effectively than sulfide treatment. The surface charge negativity of the aged nanoplastics (i.e., PS-UV and PS-S) increased to greater extents compared to pristine nanoplastics with increasing pH (Fig. S2†) due to the significant deprotonation of surface oxygen-containing functional groups.
2.2 Aggregation behavior of PS nanoplastics in the presence of monovalent and divalent salts
Sulfide and UV treatments differentially affect the colloidal stability of PS nanoplastics due to their different alterations in the physicochemical properties of nanoplastics. The surface charge and aggregation behavior of PS nanoplastics were first explored in the presence of monovalent salt (NaCl as a representative). The zeta potential as a function of NaCl concentration for different types of PS nanoplastics is presented in Fig. 3a. Notably, the shape of the plots for the three types of nanoplastics was similar, with the surface charge becoming less negative as the concentration of NaCl increases due to double layer compression resulting from charge screening.34 Furthermore, at a given concentration of NaCl, the zeta potential of PS-UV was more negative than that of pristine PS. This is attributed to the UV treatment introducing additional oxygen-containing functional groups on the surface of PS nanoplastics. This trend aligns with the increased surface oxygen-functional groups (i.e., C–OH) of PS-UV, as confirmed by XPS spectra. In contrast, the zeta potential of PS-S decreased more rapidly with increasing NaCl compared to both PS and PS-UV. This behavior can be attributed to the sulfhydryl groups on the PS-S surface, which have a pKa of approximately 8.0–10.5, making them less ionizable under the experimental conditions at pH 6.35 The weak ionization of these sulfhydryl groups led to a lower surface charge density and a more loosely structured electric double layer. Consequently, this lower surface charge was more easily screened, and the electric double layer was more readily compressed by the added NaCl, leading to a greater decrease in the magnitude of zeta potential (i.e., becoming less negative).36–38 In comparison, PS-UV contained abundant oxygen-rich functional groups, such as carboxyl groups with a pKa of 4.0–5.0,39 are more strongly ionized. This higher surface charge density made PS-UV more resistant to the screening and compression effects of NaCl.
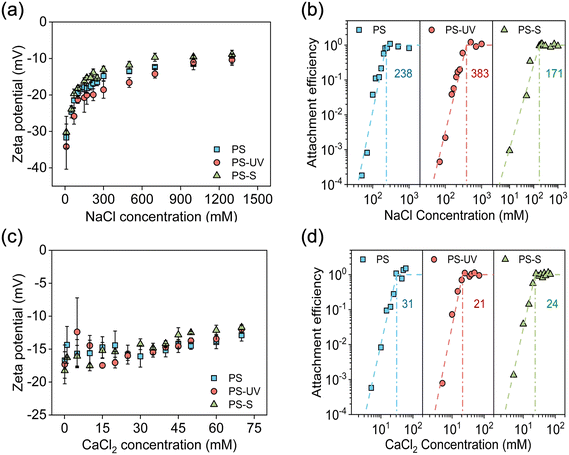 |
| Fig. 3 Charging and aggregation behavior of nanoplastics. Zeta potential (a and c) and attachment efficiencies (b and d) of PS, PS-UV, and PS-S nanoplastics as a function of NaCl and CaCl2 concentrations. Experimental conditions: room temperature (23 ± 1 °C), pH 6, and particle concentration of 10 mg L−1. Note that the starting concentrations for the curves in panels (a) and (c) are 10 mM NaCl and 0.1 mM CaCl2, respectively. | |
Aggregation profiles of PS nanoparticles as a function of NaCl concentration are shown in Fig. S3.† These profiles indicate that the aggregation rate increased as the electrolyte concentration increased, with the aggregation rate reaching its maximum value at high NaCl concentrations due to the eliminated energy barrier between nanoplastics.10,12 By normalizing the initial slopes of the aggregation profiles based on eqn (S1) and (S2),† the attachment efficiency (α), used to quantitatively describe the aggregation kinetics, was obtained (Fig. 3b). All curves exhibited similar distinct slow (α < 1, reaction-limited) and fast (α = 1, diffusion-controlled) aggregation regimes, revealing that all types of PS nanoplastics present the aggregation behavior expected from the classical DLVO theory. More specifically, at low NaCl concentrations, the aggregation rate was slow due to electric double layer (EDL) repulsion, and a small attachment efficiency was obtained. The increasing salt concentration led to charge screening and lowered the energy barrier to be overcome for aggregation to occur.40,41 As a result, the aggregation rate (or the attachment efficiency) increased rapidly, reaching unity. The minimum electrolyte concentration to induce fast aggregation is defined as the critical coagulation concentration (CCC). The CCC values determined from the attachment efficiency curves were 383, 238, and 171 mM for PS-UV, PS, and PS-S nanoplastics, respectively. These CCC values followed the order PS-UV > PS > PS-S, which agrees well with the magnitude of the zeta potential of nanoplastics. As discussed above, the abundant surface oxygen-functional groups on the surface of PS-UV gave rise to a higher negative surface charge density and the highest CCC within these three types of nanoplastics, while the sulfhydryl groups on PS-S were relatively easily screened by the cations, leading to the lower negative surface charge and lower CCC value.
The surface charge and aggregation kinetics of PS nanoplastics were also investigated in the presence of divalent salts (CaCl2 as a representative). As shown in Fig. 3c, the zeta potential rapidly became less negative with increasing concentration of divalent salts due to strong adsorption of the divalent cations and effective screening of the surface charge.42,43 Compared with the zeta potential results for monovalent salts (Fig. 3a), the divalent salt was much more effective in reducing the zeta potential of nanoplastics since they compress and screen the electrical double layer more effectively. The attachment efficiency of nanoplastics in the presence of divalent salts (Fig. 3d) exhibited the characteristic slow and fast aggregation regimes as expected from the DLVO theory. The CCC values determined from these attachment efficiency curves were 31, 24, and 21 mM for PS, PS-S, and PS-UV nanoplastics, respectively, which were approximately 7–18 times smaller than those for the monovalent salts. The CCC ratios of Ca2+/Na+ are 2–4.2, 2–2.8, and 2–2.9 for PS, PS-S, and PS-UV nanoplastics, respectively, which are generally in line with the Schulze–Hardy rule.42,44 These CCC values in the presence of divalent salt followed the order PS > PS-S > PS-UV, which agrees well with the degree of hydrophobicity of nanoplastics, in other words, reverse to the content of surface oxygen-functional groups (such as carboxyl and carbonyl groups). This trend of CCC for PS-UV and PS-S in CaCl2 solution was opposite to that in NaCl solution in which PS-UV presented a higher CCC value than PS-S. We discuss the mechanisms of these opposite trends of CCC values in detail in the following subsections.
2.3 Effects of pH and NOM on the aggregation behavior of PS nanoplastics
The solution pH and NOM are crucial factors influencing the colloidal stability of nanoplastics during their transport in the environment. To investigate this, the aggregation rates of three types of PS nanoplastics were studied in the presence of 120 mM NaCl at pH levels of 5, 7, and 9 (which fall within the typical environmental pH range), as well as with the addition of NOM. The results showed that the aggregation rate for all three types of nanoplastics decreased rapidly as the pH increased from 5 to 9 (Fig. 4a), which may be attributed to the enhanced dissociation of carboxyl groups and thus increased surface charge at higher pH.45 More specifically, PS-UV nanoplastics exhibited a higher stability at a given pH, compared to the case of PS-S, since UV treatment generated more oxygen-containing functional groups on the nanoplastic surface than sulfide treatment (Fig. 2b and Table S1†). This trend was consistent with that in the presence of NaCl (Fig. 3b). Besides, in the presence of 120 mM NaCl, the addition of NOM (i.e., Suwannee River humic acid (SRHA) and fulvic acid (SRFA)) enhanced the stability of nanoplastics (Fig. 4b). Specifically, for PS-S, the aggregation rate decreased from 3.09 to 0.12 (10−1 nm s−1) or to 0.77 (10−1 nm s−1) after adding 2 mg C per L SRHA or SRFA, respectively. This NOM-enhanced colloidal ability may be attributed to steric repulsion and enhanced electrostatic repulsion resulting from the adsorption of NOM on the nanoplastic surface.46,47 The addition of NOM has a limited effect on the stability of PS-UV since PS-UV nanoplastics themselves remained stable in the presence of 120 mM NaCl. Notably, SRHA exhibited a stronger enhancement on the particle stability than SRFA since a larger amount of SRHA adsorb onto nanoplastic surface due to their higher aromaticity and hydrophobicity compared to SRFA.48 This can be proved by the more negative zeta potential of the nanoplastic after the adsorption of SRHA (Fig. S5†).
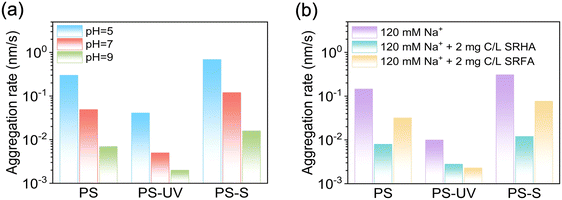 |
| Fig. 4 Aggregation rates of PS, PS-UV, and PS-S nanoplastics in aqueous solutions under varying initial pH conditions (a) and in 120 mM NaCl solutions with or without NOM (i.e., SRHA and SRFA) (b). | |
2.4 Proposed mechanisms influencing the aggregation behavior of PS nanoplastics
To further elucidate the mechanisms by which weathering pathways distinctly affect the colloidal stability of nanoplastics, the Hamaker constant, an intrinsic parameter for evaluating the van der Waals attraction between interacting particles, was derived by comparing the experimental attachment efficiencies in the presence of NaCl with calculated results based on DLVO theory.34,49 The comparison showed a good agreement between the DLVO predictions and the experimental data (Fig. 5a), both in the reaction-limited and diffusion-limited regimes, for PS nanoparticles with different aging treatments. Upon UV-induced aging (PS-UV), the Hamaker constant (which is derived from the fitting of the DLVO predictions and the experimental data) decreased from 3.0 × 10−21 to 2.4 × 10−21 J, while this value increased to 7.8 × 10−21 upon sulfide-induced aging (PS-S) (Fig. 5b), which indicates the distinct surface structural change of nanoplastics caused by the two aging processes. These values of the Hamaker constant are comparable to values (1–10 × 10−21 J) reported in previous studies on PS nanoplastics.12 The reduction in the Hamaker constant for PS-UV leads to diminished van der Waals attractions, thereby enhancing their colloidal stability compared to PS-S, which shows an increased Hamaker constant. Additionally, as mentioned above, PS-S exhibited a more rapid decrease in zeta potential in the presence of NaCl because the weakly ionizable sulfhydryl groups (with a pKa of 8.0–10.5) resulted in a lower surface charge density, leading to a looser electric double layer. This made the electric double layer more easily compressed by Na+ ions. In contrast, PS-UV was more resistant to this compression due to the highly abundant strongly ionized oxygen-containing functional groups (e.g., carboxyl groups with a pKa of 4.0–5.0) on its surface. These factors collectively explain why the CCC of PS-UV in the presence of NaCl is higher than that of PS-S (Fig. 5c).
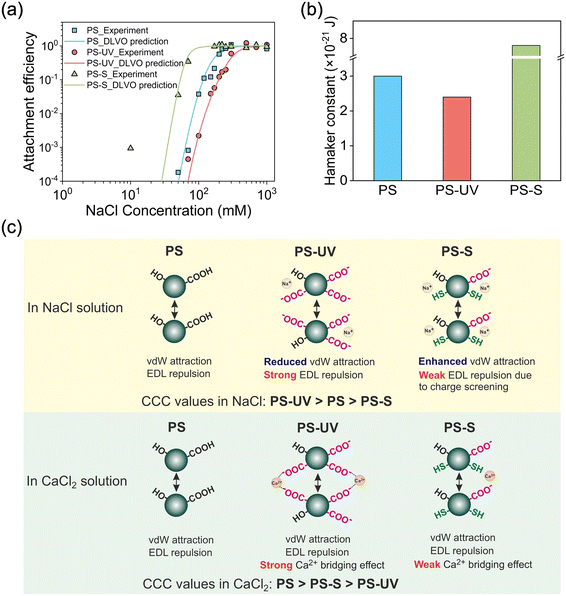 |
| Fig. 5 Comparison of experimentally determined attachment efficiencies with predictions based on DLVO theory for PS, PS-UV, and PS-S in the presence of NaCl (a), and the derived Hamaker constant obtained by fitting DLVO predictions to the experimental data (b). Proposed mechanisms influencing the aggregation behavior of PS nanoplastics in NaCl and CaCl2 solutions (c). | |
Furthermore, in the presence of CaCl2, as nanoplastics approach each other, the Ca2+ ions can specifically “bridge” with these oxygen-containing functional groups (especially carboxyl) on the nanoplastic surface, thereby enhancing their aggregation rates (Fig. 5c).12 This bridging effect of Ca2+ ions is a well-documented mechanism in previous studies and has also been reported for other types of nanoparticles (e.g., fullerene and silicon nanoparticles).48,50 Therefore, PS-UV exhibited a smaller CCC value than PS-S in the presence of a divalent salt since more oxygen-containing functional groups were formed by UV treatment than by sulfide treatment (Fig. 2b and Table S1†). This trend in the CaCl2 solution was opposite to that in the NaCl solution, in which PS-UV presented a higher CCC value than PS-S.
Collectively, we observed that PS-UV exhibited greater stability compared to PS-S in the presence of NaCl but reduced stability in the presence of CaCl2. This increased stability in NaCl can be attributed to the reduced van der Waals attraction between aged PS-UV particles due to their lower Hamaker constants and the enhanced EDL repulsion resulting from the deprotonation of oxygen-containing functional groups. Conversely, in the CaCl2 solution, the decreased stability is likely due to the “bridging” effect of Ca2+ ions with these oxygen-containing functional groups, which promotes aggregation.
3. Conclusion
This study has shown that once PS nanoplastics are released into the environment, natural weathering processes significantly and variably influence their aggregation behavior and environmental fate. In the presence of a monovalent salt, UV-irradiated PS nanoplastics exhibit greater colloidal stability compared to those treated with sulfides. The enhanced stability is attributed to both the increased surface charge from UV-induced incorporation of oxygen-containing functional groups and the reduced van der Waals attractions. Additionally, the abundance of these oxygen-rich functional groups allows PS-UV nanoplastics to remain stable across a wide range of pH levels. However, under conditions involving divalent salts, the stability of PS-UV nanoplastics diminishes due to the bridging interactions between Ca2+ ions and the oxygen-containing functional groups.
Importantly, PS nanoplastics are prevalent across a variety of environmental sites, where physicochemical conditions—such as UV radiation, sulfide levels, and water chemistry (e.g., ion species and contaminants)—can vary substantially. These findings suggest that accurate predictions of the fate, transport, and ecological risks of PS nanoplastics require consideration of the specific environmental conditions at each site. Furthermore, the persistence of PS nanoplastics in the environment, coupled with the potential for prolonged weathering, highlights the need for further research. Future studies should focus on how the evolving chemical and physical properties of PS nanoplastics at various stages of weathering influence their environmental behavior.
Data availability
The data supporting the findings of this study are available from the corresponding author upon reasonable request.
Conflicts of interest
There are no conflicts to declare.
Acknowledgements
This work was supported by the National Natural Science Foundation of China (22125603, 22241602), Tianjin Municipal Science and Technology Bureau (21JCJQJC00060, 23JCQNJC01340), and the Fundamental Research Funds for the Central Universities, Nankai University (63241418).
References
- D. Allen, S. Allen, S. Abbasi, A. Baker, M. Bergmann, J. Brahney, T. Butler, R. A. Duce, S. Eckhardt, N. Evangeliou, T. Jickells, M. Kanakidou, P. Kershaw, P. Laj, J. Levermore, D. Li, P. Liss, K. Liu, N. Mahowald, P. Masque, D. Materić, A. G. Mayes, P. McGinnity, I. Osvath, K. A. Prather, J. M. Prospero, L. E. Revell, S. G. Sander, W. J. Shim, J. Slade, A. Stein, O. Tarasova and S. Wright, Microplastics and nanoplastics in the marine-atmosphere environment, Nat. Rev. Earth Environ., 2022, 3(6), 393–405 CrossRef CAS.
- M. Zhang and L. Xu, Transport of micro- and nanoplastics in the environment: Trojan-Horse effect for organic contaminants, Crit. Rev. Environ. Sci. Technol., 2022, 52(5), 810–846 CrossRef CAS.
- M. Alaraby, D. Abass, J. Domenech, A. Hernández and R. Marcos, Hazard assessment of ingested polystyrene nanoplastics in Drosophila larvae, Environ. Sci.: Nano, 2022, 9(5), 1845–1857 RSC.
- C. Cortés, J. Domenech, M. Salazar, S. Pastor, R. Marcos and A. Hernández, Nanoplastics as a potential environmental health factor: effects of polystyrene nanoparticles on human intestinal epithelial Caco-2 cells, Environ. Sci.: Nano, 2020, 7(1), 272–285 RSC.
- Z. Yang, G. M. DeLoid, H. Zarbl, J. Baw and P. Demokritou, Micro- and nanoplastics (MNPs) and their potential toxicological outcomes: State of science, knowledge gaps and research needs, NanoImpact, 2023, 32, 100481 CrossRef CAS.
- X. Liu, R. Xu, H. Wu, K. Xu, W. Zhang, Z. Wang and W. Gong, Nanoplastics promote the dissemination of antibiotic resistance through conjugative gene transfer: implications from oxidative stress and gene expression, Environ. Sci.: Nano, 2023, 10(5), 1329–1340 RSC.
- H. Gong, R. Li, F. Li, X. Guo, L. Xu, L. Gan, M. Yan and J. Wang, Toxicity of nanoplastics to aquatic organisms: Genotoxicity, cytotoxicity, individual level and beyond individual level, J. Hazard. Mater., 2023, 443, 130266 CrossRef CAS.
- J. Liu, Y. Ma, D. Zhu, T. Xia, Y. Qi, Y. Yao, X. Guo, R. Ji and W. Chen, Polystyrene Nanoplastics-Enhanced Contaminant Transport: Role of Irreversible Adsorption in Glassy Polymeric Domain, Environ. Sci. Technol., 2018, 52(5), 2677–2685 CrossRef CAS PubMed.
- O. S. Alimi, J. Farner Budarz, L. M. Hernandez and N. Tufenkji, Microplastics and nanoplastics in aquatic environments: aggregation, deposition, and enhanced contaminant transport, Environ. Sci. Technol., 2018, 52(4), 1704–1724 CrossRef CAS.
- X. Li, E. He, B. Xia, Y. Liu, P. Zhang, X. Cao, L. Zhao, X. Xu and H. Qiu, Protein corona-induced aggregation of differently sized nanoplastics: impacts of protein type and concentration, Environ. Sci.: Nano, 2021, 8(6), 1560–1570 RSC.
- O. Oriekhova and S. Stoll, Heteroaggregation of nanoplastic particles in the presence of inorganic colloids and natural organic matter, Environ. Sci.: Nano, 2018, 5(3), 792–799 RSC.
- Y. Liu, Y. Hu, C. Yang, C. Chen, W. Huang and Z. Dang, Aggregation kinetics of UV irradiated nanoplastics in aquatic environments, Water Res., 2019, 163, 114870 CrossRef CAS.
- S. Reynaud, A. Aynard, B. Grassl and J. Gigault, Nanoplastics: From model materials to colloidal fate, Curr. Opin. Colloid Interface Sci., 2022, 57, 101528 CrossRef CAS.
- A. Foetisch, M. Filella, B. Watts, M. Bragoni and M. Bigalke, After the sun: a nanoscale comparison of the surface chemical composition of UV and soil weathered plastics, Microplastics and Nanoplastics, 2023, 3(1), 18 CrossRef.
- T. Atugoda, H. Piyumali, H. Wijesekara, C. Sonne, S. S. Lam, K. Mahatantila and M. Vithanage, Nanoplastic occurrence, transformation and toxicity: a review, Environ. Chem. Lett., 2023, 21(1), 363–381 CrossRef CAS.
- J. Su, J. Ruan, D. Luo, J. Wang, Z. Huang, X. Yang, Y. Zhang, Q. Zeng, Y. Li, W. Huang, L. Cui and C. Chen, Differential Photoaging Effects on Colored Nanoplastics in Aquatic Environments: Physicochemical Properties and Aggregation Kinetics, Environ. Sci. Technol., 2023, 57(41), 15656–15666 CrossRef CAS PubMed.
- F. Lian, Y. Han, Y. Zhang, J. Li, B. Sun, Z. Geng, Z. Wang and B. Xing, Exposure Order to Photoaging and Humic Acids Significantly Modifies the Aggregation and Transformation of Nanoplastics in Aqueous Solutions, Environ. Sci. Technol., 2023, 57(16), 6520–6529 CrossRef CAS PubMed.
- X. Li, S. Ji, E. He, W. J. G. M. Peijnenburg, X. Cao, L. Zhao, X. Xu, P. Zhang and H. Qiu, UV/ozone induced physicochemical transformations of polystyrene nanoparticles and their aggregation tendency and kinetics with natural organic matter in aqueous systems, J. Hazard. Mater., 2022, 433, 128790 CrossRef CAS.
- Z. Ouyang, Z. Zhang, Y. Jing, L. Bai, M. Zhao, X. Hao, X. Li and X. Guo, The photo-aging of polyvinyl chloride microplastics under different UV irradiations, Gondwana Res., 2022, 108, 72–80 CrossRef CAS.
- M. Zhao, T. Zhang, X. Yang, X. Liu, D. Zhu and W. Chen, Sulfide induces physical damages and chemical transformation of microplastics via radical oxidation and sulfide addition, Water Res., 2021, 197, 117100 CrossRef CAS.
- L. Duan, Y. Qin, X. Meng, Y. Liu, T. Zhang and W. Chen, Sulfide- and UV-induced aging differentially affect contaminant-binding properties of microplastics derived from commercial plastic products, Sci. Total Environ., 2023, 869, 161800 CrossRef CAS PubMed.
- T. Du, L. Qian, S. Shao, T. Xing, T. Li and L. Wu, Comparison of sulfide-induced transformation of biodegradable and conventional microplastics: Mechanism and environmental fate, Water Res., 2024, 253, 121295 CrossRef CAS.
- J. Lin, D. Yan, J. Fu, Y. Chen and H. Ou, Ultraviolet-C and vacuum ultraviolet inducing surface degradation of microplastics, Water Res., 2020, 186, 116360 CrossRef CAS PubMed.
- Y. Xu, Q. Ou, J. P. van der Hoek, G. Liu and K. M. Lompe, Photo-oxidation of Micro- and Nanoplastics: Physical, Chemical, and Biological Effects in Environments, Environ. Sci. Technol., 2024, 58(2), 991–1009 CrossRef CAS.
- X. Wang, Y. Li, J. Zhao, X. Xia, X. Shi, J. Duan and W. Zhang, UV-induced aggregation of polystyrene nanoplastics: effects of radicals, surface functional groups and electrolyte, Environ. Sci.: Nano, 2020, 7(12), 3914–3926 RSC.
- T. Du, S. Shao, L. Qian, R. Meng, T. Li, L. Wu and Y. Li, Effects of photochlorination on the physicochemical transformation of polystyrene nanoplastics: Mechanism and environmental fate, Water Res., 2023, 243, 120367 CrossRef CAS PubMed.
- C. Campanale, I. Savino, C. Massarelli and V. F. Uricchio, Fourier Transform Infrared Spectroscopy to Assess the Degree of Alteration of Artificially Aged and Environmentally Weathered Microplastics, Polymers, 2023, 15, 911 CrossRef CAS PubMed.
- L. Ding, Y. Luo, X. Yu, Z. Ouyang, P. Liu and X. Guo, Insight into interactions of polystyrene microplastics with different types and compositions of dissolved organic matter, Sci. Total Environ., 2022, 824, 153883 CrossRef CAS.
- J. Almond, P. Sugumaar, M. N. Wenzel, G. Hill and C. Wallis, Determination of the carbonyl index of polyethylene and polypropylene using specified area under band methodology with ATR-FTIR spectroscopy, e-Polym., 2020, 20(1), 369–381 CrossRef CAS.
- J. Fu, W. Liu, Z. Hao, X. Wu, J. Yin, A. Panjiyar, X. Liu, J. Shen and H. Wang, Characterization of a Low Shrinkage Dental Composite Containing Bismethylene Spiroorthocarbonate Expanding Monomer, Int. J. Mol. Sci., 2014, 15(2), 2400–2412 CrossRef.
- B. N. Jang and C. A. Wilkie, The thermal degradation of polystyrene nanocomposite, Polymer, 2005, 46(9), 2933–2942 CrossRef CAS.
- M. Kozłowski, XPS study of reductively and non-reductively modified coals, Fuel, 2004, 83(3), 259–265 CrossRef.
- S. Lu, K. Zhu, W. Song, G. Song, D. Chen, T. Hayat, N. S. Alharbi, C. Chen and Y. Sun, Impact of water chemistry on surface charge and aggregation of polystyrene microspheres suspensions, Sci. Total Environ., 2018, 630, 951–959 CrossRef CAS.
- T. Cao and M. Elimelech, Colloidal stability of cellulose nanocrystals in aqueous solutions containing monovalent, divalent, and trivalent inorganic salts, J. Colloid Interface Sci., 2021, 584, 456–463 CrossRef CAS.
- A. V. Glushchenko and D. W. Jacobsen, Molecular Targeting of Proteins by L-Homocysteine: Mechanistic Implications for Vascular Disease, Antioxid. Redox Signaling, 2007, 9(11), 1883–1898 CrossRef CAS PubMed.
- V. Agmo Hernández, An overview of surface forces and the DLVO theory, ChemTexts, 2023, 9(4), 10 CrossRef.
- G. Trefalt, S. H. Behrens and M. Borkovec, Charge Regulation in the Electrical Double Layer: Ion Adsorption and Surface Interactions, Langmuir, 2016, 32(2), 380–400 CrossRef CAS PubMed.
-
J. N. Israelachvili, Intermolecular and surface forces, Academic press, 2015 Search PubMed.
- M. Müller, L. Wirth and B. Urban, Determination of the Carboxyl Dissociation Degree and pKa Value of Mono and Polyacid Solutions by FTIR Titration, Macromol. Chem. Phys., 2021, 222(4), 2000334 CrossRef.
- S. Stenberg and J. Forsman, Overcharging and Free Energy Barriers for Equally Charged Surfaces Immersed in Salt Solutions, Langmuir, 2021, 37(49), 14360–14368 CrossRef CAS PubMed.
- A. M. Smith, M. Borkovec and G. Trefalt, Forces between solid surfaces in aqueous electrolyte solutions, Adv. Colloid Interface Sci., 2020, 275, 102078 CrossRef CAS PubMed.
-
M. Elimelech, J. Gregory and X. Jia, Particle deposition and aggregation: measurement, modelling and simulation, Butterworth-Heinemann, 2013 Search PubMed.
- T. Cao, T. Sugimoto, I. Szilagyi, G. Trefalt and M. Borkovec, Heteroaggregation of oppositely charged particles in the presence of multivalent ions, Phys. Chem. Chem. Phys., 2017, 19(23), 15160–15171 RSC.
- T. Cao, I. Szilagyi, T. Oncsik, M. Borkovec and G. Trefalt, Aggregation of colloidal particles in the presence of multivalent co-ions: The inverse Schulze–Hardy rule, Langmuir, 2015, 31(24), 6610–6614 CrossRef CAS PubMed.
- Z. Chen, X. Xiao, B. Chen and L. Zhu, Quantification of Chemical States, Dissociation Constants and Contents of Oxygen-containing Groups on the Surface of Biochars Produced at Different Temperatures, Environ. Sci. Technol., 2015, 49(1), 309–317 CrossRef CAS PubMed.
- I. Ali, X. Tan, J. Li, C. Peng, I. Naz, Z. Duan and Y. Ruan, Interaction of microplastics and nanoplastics with natural organic matter (NOM) and the impact of NOM on the sorption behavior of anthropogenic contaminants – A critical review, J. Cleaner Prod., 2022, 376, 134314 CrossRef CAS.
- Z. Li, S. Shakiba, N. Deng, J. Chen, S. M. Louie and Y. Hu, Natural Organic Matter (NOM) Imparts Molecular-Weight-Dependent Steric Stabilization or Electrostatic Destabilization to Ferrihydrite Nanoparticles, Environ. Sci. Technol., 2020, 54(11), 6761–6770 CrossRef CAS.
- W. Zhang, U. S. Rattanaudompol, H. Li and D. Bouchard, Effects of humic and fulvic acids on aggregation of aqu/nC60 nanoparticles, Water Res., 2013, 47(5), 1793–1802 CrossRef CAS PubMed.
- K. L. Chen and M. Elimelech, Aggregation and deposition kinetics of fullerene (C60) nanoparticles, Langmuir, 2006, 22(26), 10994–11001 CrossRef CAS PubMed.
- X. Liu, M. Wazne, T. Chou, R. Xiao and S. Xu, Influence of Ca2+ and Suwannee River Humic Acid on aggregation of silicon nanoparticles in aqueous media, Water Res., 2011, 45(1), 105–112 CrossRef CAS PubMed.
|
This journal is © The Royal Society of Chemistry 2025 |
Click here to see how this site uses Cookies. View our privacy policy here.